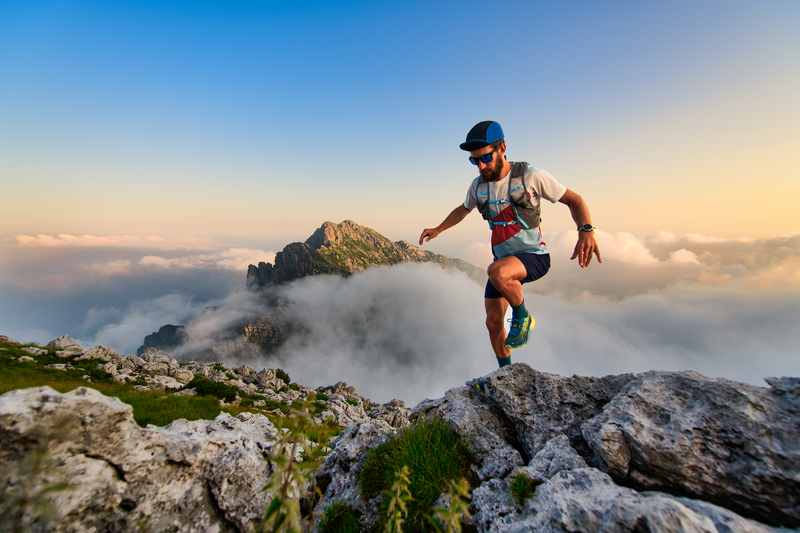
94% of researchers rate our articles as excellent or good
Learn more about the work of our research integrity team to safeguard the quality of each article we publish.
Find out more
ORIGINAL RESEARCH article
Front. Environ. Sci. , 25 September 2023
Sec. Toxicology, Pollution and the Environment
Volume 11 - 2023 | https://doi.org/10.3389/fenvs.2023.1252305
This article is part of the Research Topic Recent Research Advances on Heavy Metals, Microplastics, Persistent Organic Pollutants, and Solid Waste in Aquatic and Terrestrial Ecosystems View all 11 articles
Farmers in resource-poor areas of the Guinea Savanna zone of Ghana often face declining soil fertility due to the continuous removal of nutrient-rich harvested produce from their fields. This study focuses on the Lawra Municipality in the Guinea Savanna zone of Ghana, where low soil fertility, specifically, limits phosphorus (P) bioavailability and hinders crop production. The objective of this research is to formulate P-enhanced biochar-compost from maize stover (MS) and groundnut husk, which abound in the area, to close the nutrient loop. MS was co-composted with groundnut husk biochar at varying rates of 0, 10, 20, 30, and 40% by volume. To facilitate decomposition using the windrow system, the composting heaps were inoculated with decomposing cow dung, and the moisture content was kept at 60% throughout the monitoring period. The addition of biochar shortened the lag phase of composting. However, rates above 20% resulted in reduced degradation of MS. Biochar incorporation enriched the available phosphorus content in the final compost from 286.7 mg kg−1 in the non-biochar-compost to 320, 370, 546, and 840.0 mg kg−1 in the 10, 20, 30, and 40% biochar-compost, respectively.
Soil organic matter plays an important role in soil fertility and crop yield improvement via the geochemical cycling of plant nutrients such as P (Duarte and Duarte, 2019; Ch’Ng et al., 2014). Compost is an accepted form of organic matter applied to soils (Diaz and Bertoldi, 2007). However, in tropical regions where there is rapid mineralization, compost application has had limited long-term beneficial results (Gan et al., 2020; Oliveira et al., 2020). Consequently, desired benefits, including soil bulk density reduction, nutrient availability enhancement, and overall soil productivity improvements, have been minimal (Oorts et al., 2003; Ahad et al., 2015; Tsai and Chang, 2019). Considerable research attention has, therefore, been devoted to compost stabilization in recent years (Gabhane et al., 2012; Bazrafshan et al., 2016). One promising approach is the co-application of biochar and compost, which has shown the potential of synergistically enhancing soil quality and promoting plant growth (Fischer and Glaser, 2012; Gan et al., 2020; Antonangelo et al., 2021). Biochar as a feedstock in composting has had positive effects on compost stability, reducing composting time, and minimizing the leaching of exchangeable cations such as NH4+ (Gabhane et al., 2012; Vandecasteele et al., 2016; Akumah et al., 2021). The use of biochar in co-composting has mainly been in the context of managing municipal and market organic waste (Bazrafshan et al., 2016; Akumah et al., 2021; Sulemana et al., 2021). Limited studies have investigated the use of biochar as a co-composting feedstock to specifically harness nutrients from farm residues. Most farm residues in the Guinea Savanna zone of sub-Saharan Africa are either burnt or removed from farm sites to be fed to animals. Harnessing these residues into biochar-compost for application to the farms from which the produce is harvested will minimize nutrient mining and ultimately close the nutrient loop. Farm residues are classified as bulking agents (Batham et al., 2013), thereby limiting their prospects for composting. These residues have, however, sequestered nutrients that, upon composting, could be made available, especially in the Guinea Savanna zone, where soil organic carbon levels are very low.
Most compost plants are sited close to urban centers to primarily manage municipal waste and, thus, serve as a major boost to urban and peri-urban agriculture. However, the cost involved in transporting the amendment to the Guinea Savanna zone, where organic carbon contents of soils are very low, is a disincentive. In Ghana’s Guinea Savanna zone, where low soil fertility continues to constrain crop production (Tetteh et al., 2016; IFDC, 2017; UNDP, 2018), the limited availability of household waste restricts the production of biochar-compost (Amoah and Kosoe, 2014). However, there is an opportunity to harness the potential of readily available farm residues such as maize (Zea mais) stover and groundnut (Arachis hypogea) husk, which have limited competing uses. Maize stover (MS) is abundantly generated in the zone due to the surge in maize production, surpassing that of millet and sorghum (Raheem et al., 2021). Approximately 10.47 tons/ha and 8.07 tons/ha of maize and groundnut, respectively, were produced in the Guinea Savanna zone of Ghana in the year 2020 (SRID, 2021). The cob and husk after shelling are used as feed for livestock and fuel for cooking, which creates competition for their use as composting feedstock. Similarly, groundnut husk offers potential as a composting material due to its large volume compared to waste from cowpea (Vigna unguiculata) (SRID, 2021). The transportation of tubers and vegetables outside of the Guinea Savanna zone results in limited quantities of waste available for compost production. Therefore, MS and groundnut husk are readily available feedstocks with limited competing uses for composting.
Groundnut is a legume and, thus, has a high demand for P. Being a pod, the husk is likely to sequester high levels of P. Groundnut husk has been found to contain, on average, 105.5 mg kg-1 of P (Mokolopi, 2022). Charred groundnut husk could, therefore, liberate P, making it a very good co-composting feedstock with MS, a readily available farm waste in the Guinea Savanna zone of Ghana. Organic acids produced from MS during composing would help minimize surface precipitation of P onto biochar, thereby making the nutrient readily available should the compost product be applied to the generally P-deficient soils of the Guinea Savanna zone of northern Ghana and sub-Saharan Africa in general. Such a biochar-compost should help recycle P in northern Ghana and decrease the import bill of Ghana for inorganic P fertilizer, especially in this era of high inorganic fertilizer costs. Carbon is highly recalcitrant in biochar-compost (Sulemana et al., 2019) and could, thus, be sequestered into the impoverished and fragile soils of the Guinea Savanna and invariably improve their productivity.
The ideal ratio of groundnut husk biochar (GHB) to MS and the protocol to be used in formulating an ideal P-rich biochar-compost are yet to be ascertained. It is in light of this that the present study seeks to exploit the potential of MS and groundnut husk as feedstocks to produce a P-rich organic amendment. This study also aims to provide practical solutions for closing the nutrient loop by harnessing farm waste, which would have otherwise been burnt in resource-limited farming systems.
Groundnut husk and MS were collected from farmers’ fields in Lawra, located in the Upper West Region of the Guinea Savanna agro-ecological zone of Ghana. Parts of these feedstocks were oven-dried at 70°C to attain constant weight. The samples were then ground and saved for chemical characterization.
Part of the groundnut husk was sun-dried, weighed (W1), and pyrolyzed using a locally fabricated retort stove (Kuntan kiln). Temperature readings were recorded at 10-min intervals using the Extech Dual Laser Infrared Thermometer (model 42,570) until pyrolysis was complete. The mean temperature of the readings (454.4 ± 19.8°C) was taken as the pyrolysis temperature. The product, GHB, was collected from the hot kiln after intermittent turning and then cooled with clean water. The cooled biochar was air-dried, weighed (W2), and then, bagged in jute sacks for storage. Biochar yield, which represents the quantity of biochar produced from a quantity of feedstock charred, was expressed as a percentage. Samples of the biochar produced were then ground to pass through a 2 mm sieve for characterization.
The MS was chopped with machetes and then sieved to obtain fraction of length <5 cm. The sieved fraction of the MS was mixed with GHB on volume basis to attain varying MS-to-biochar ratios of 10:0, 9:1, 8:2, 7:3, and 6:4, representing 0, 10, 20, 30, and 40% biochar concentrations. Thus, five different composting heaps, each 2 m in diameter at the base and 1 m in height, were formulated, replicated three times, and arranged in a completely randomized design. To each compost heap, 1 kg of decomposing cow dung (nitrogen = 2.3%, organic carbon = 25.6%, available p = 0.38%, and pHwater = 8.2) was added as an inoculant to introduce decomposing microorganisms. The heaps were uniformly mixed on a cemented compost platform with a slope of about 0% and moistened to approximately 60% moisture content. Perforated polyvinyl chloride (PVC) pipes of 7 cm diameter with grooves at the top end were inserted into each of the heaps, making sure that the base of each of the PVC pipes touched the cemented platform. With the aid of ropes fastened onto twigs, thermometers were inserted into each of the compost heaps by hanging the twigs into the PVC grooves. Heat emanating from the heaps during the composting process passed through the perforations to be measured by the thermometers inside the PVC pipes. Daily temperature readings were recorded, and the compost moistened and turned when the temperature readings became stable. The maturity of the various heaps was determined when temperatures did not increase after compost moistening and turning.
The maturity of the various compost types was assessed using the germination index (GI) method as described by Zucconi et al. (1981). Total humic substances were determined based on their relative solubility in acid and alkaline extractants according to the method of Serra-Wittling et al. (1996). The CEC of the compost samples was determined using the method described by Harada and Inoko (1980a).
The biochar was analyzed for its ash content according to the method prescribed by the ASTM D1762-84 (2007) for wood charcoal. The pH and EC of the milled groundnut husk and MS feedstocks, the GHB, and different compost types were determined electrometrically in a ratio of 1:10 (dried samples:deionized water). Total carbon and nitrogen in the samples were determined by dry combustion with a Leco Trumac version 1.3 Carbon–Nitrogen–Sulfur Analyzer. The hydrogen contents of the groundnut husk and its biochar derivative were determined using the Leco Trumac H analyzer, and their oxygen contents were determined by the difference method, i.e., [100% − (%H+%C+%N)] as sulfur was not detected by the analyzer. Soluble Ca, Mg, and K in the biochar were extracted with distilled water, and their concentrations were read on a PerkinElmer AAnalyst 800 spectrophotometer.
Total P, Ca, Mg, K, and Na in the matured compost types were determined by digesting 0.5 g of homogenized samples with 5 mL of concentrated H2SO4. The color development of phosphorus in the digest was carried out by the ascorbic acid method of Murphy and Riley (1962), and the concentrations of the element were read on a Cole–Palmer UV spectrophotometer. Calcium, Mg, K, and Na concentrations were read on a PerkinElmer AAnalyst 800 atomic absorption spectrophotometer. The available phosphorus contents of biochar and compost were determined according to the method of Watanabe and Olsen (1965). Available N was determined by Kjeldahl distillation after extraction with 2 M KCl. The cation exchange capacity of biochar and compost types was determined using a BaCl2/triethanolamine (TEA) solution buffered at pH 8.2. The presence of surface functional groups was determined using the PerkinElmer Spectrum Two FT-IR Spectrometer fitted with MIRacle Accessory. The concentrations of carboxylic, lactonic, and phenolic functional groups in biochar and compost types were determined by Boehm titration (Boehm, 1994).
All the data were subjected to normality tests and thereafter subjected to both univariate ANOVA and multivariate analyses using GenStat Edition 12. Treatment means were compared using Fischer’s protected LSD test at p < 0.05. Graphs from FT-IR analyses were redrawn using the Origin 2021 software.
Table 1 shows selected properties of the MS, groundnut husk, and GHB used for the study. The yield of biochar from groundnut husk using the Kuntan kiln was 35.1%. The biochar had an ash content of 52.35 g/kg via proximate analysis. The pH in water of the groundnut husk was 7.06, 1.31 pH units higher than that of the MS (5.75). The total P content of the groundnut husk (921.7 mg P kg−1) was 6.2-folds that of the MS (147.8 mg P kg−1). The total C and N contents of the groundnut husk were 373.65 g kg−1 and 7.36 g kg−1, respectively, whereas in MS, they were 333.1 g kg−1 and 6.02 g kg−1, respectively. These results yielded a C:N ratio of 50.8 for groundnut husk, which was lower than that of MS, which was 55.2. The superior concentrations of total P and C in the legume justified its choice for use as feedstock for biochar production for enhanced P availability and C sequestration.
TABLE 1. Some properties of the two feedstocks and the groundnut husk biochar derivativea.
Pyrolysis liberated bases from the groundnut husk to levels at which the concentrations of water-soluble Ca, Mg, and K in the biochar were 2.29 g kg−1, 0.43 g kg−1, and 5.32 g kg−1, respectively (Table 1). Consequently, the pH of the groundnut husk, which was neutral (7.06), increased to strongly alkaline (9.74) in the biochar derivative. Electrical conductivity also increased to 1.3 dS m−1 in the resultant biochar. The CEC of the GHB was 49.63 cmolc kg−1. Total phosphorus, which was 921 mg kg−1 in the feedstock, increased 3.7-folds to 3,400 mg P kg−1 upon charring, with approximately 22% of that being in the available form. Pyrolysis of the groundnut husk feedstock did not have any significant reduction in total N content, as the feedstock N, which was 7.36 g kg−1, was similar to the 7.13 g kg−1 (p < 0.05) of the biochar derivative. Less than 0.02% of the total N in the biochar was in the available form. Pyrolysis of the groundnut husk increased the total carbon content from 373.7 g kg−1 in the feedstock to 671.3 g kg−1, which culminated in an increased C:N ratio of 94.2 from the 50.8 of the feedstock.
The concentration of elemental O decreased from 422.7 g kg−1 to 291.7 g kg−1. Elemental H also decreased from 52.9 g kg−1 to 24.7 g kg−1. Thus, aromaticity (H/C) and stability (O/C) in the feedstock were 0.14 and 1.32, respectively. Aromaticity and stability were also 0.04 and 0.43, respectively, for the biochar. The total acidic functional groups contained in a gram of biochar were 77.91 mmol. The concentration of carboxylic group was very low (1.05 mmol g−1), lower than that of phenolic and lactone groups, which were 38.92 and 37.95 mmol g−1, respectively.
Functional groups present on the surface of the groundnut husk and the resultant biochar, as revealed by ATR-FTIR spectra, in the range of 4,000–500 cm-1 are shown in Figure 1. For the groundnut husk, the presence of a broad band around 3,320 cm−1 assigned to intermolecular -OH stretching vibration was indicative of alcoholic, phenolic, and carboxylic or N–H stretching of amino functional groups (Ossman et al., 2014; Eder et al., 2021). The intensity of this peak increased upon pyrolysis and re-centered around 3,350 cm−1. The spectra further revealed a band at 2,920 cm−1 for the groundnut husk, which was ascribed to C–H asymmetrical stretching of methyl, methylene, and amine groups (Chun et al., 2004; Schwanninger et al., 2004). This band was lost upon pyrolysis. Similarly, the presence of strongly bonded C=O stretching vibration at 1739.40 cm−1 representing ester, aldehyde, and δ-lactone was also lost upon pyrolysis (Smith, 2011). The transmission band around 1,630 cm−1, which indicates the presence of C=C stretching in alkenes and N–H bending in amines, was intensified upon pyrolysis. Strong N–O stretching for a nitro compound assigned to the peak around 1,508 cm−1 was lost in the biochar. The band at 1419.49 cm−1, indicative of the medium O–H bend of carboxylic groups (Yang et al., 2006; Smith, 2011), was also lost upon pyrolysis. The band at 1379.64 cm−1 was retained upon pyrolysis and was assigned to nitrate ions. Strong C-O stretch and medium C-N stretch bonds were assigned to the band at 1215.65 cm−1. This band was retained in the biochar. The bands at 1096.85 and 873.18 are indicative of phosphate ions and out-of-plane bending for carbonate ions, respectively (Smith, 2023).
The composting lag phase varied for the various decomposing heaps. The initial increase in temperature occurred after 3 days in the compost without any biochar and in the 10% biochar heaps (Figure 2). Increasing the biochar rate to 20% shortened the lag phase to 2 days, while further increasing the biochar rate to 30% and 40% prolonged the lag phase to 4 days. The temperature in the various compost heaps had risen from 28°C to 32°C by day 7, except for the 40% biochar heap, which saw a marginal increase to 29°C (Figure 2). The temperature remained constant between days 8 and 9, and the heaps were turned on day 9. Except for the 40% biochar heap, which recorded no increase in temperature, the heaps showed an increase in temperature a day after turning. The temperature in the 0% biochar and 10% biochar heaps rose to 34°C, while that of the 20% biochar heap increased to 32°C. Temperatures in the 30% and 40% biochar heaps were 31°C and 27°C, respectively.
After the first turning (10 days), a decline in maximum weekly temperature was observed up to maturity (Figure 3). The weekly temperature was highest in the 0% biochar decomposing heap (28°C–36°C) and lowest in the 40% biochar heap (23°C–28°C), with that of the 10, 20, and 30% biochar heaps being similar (26°C–34°C). By week 8, the temperature in the 40% biochar compost heap (23°C) was not different from that of the ambient air and was lower than the temperature at the start of the composting. The 0% biochar and 10% biochar compost heap temperatures were 28°C, while the temperatures for the 20% and 30% biochar compost heaps were 27°C and 26°C, respectively, by the end of the eighth week. In general, the temperatures recorded in the heaps were not thermophilic.
The indices used to assess the maturity of the composts produced are summarized in Table 2. The germination index is a measure of the effect of phytotoxic substances from compost on the germination rate and radicle elongation of seedlings. The germination index increased as the biochar percentage increased and ranged from 103.5 to 108.6. Biochar addition significantly increased the humin content with values ranging from 71.8% in the 0% biochar compost type to 78.5% in the 30% biochar compost. The humin content of the biochar compost types with biochar contents of 10% and 20% was similar (p < 0.05) to the non-biochar compost type. However, the compost types with higher biochar contents (30% and 40%) had statistically higher humin content compared to their non-biochar counterparts, albeit with similar content as the lower biochar (10% and 20%) types. Compost types with high biochar content (30% and 40%) had the least humic acid (HA) content with the acid content in the 40% biochar compost type being less than half of that in the non-biochar compost type. Similarly, fulvic acid (FA) concentration was highest in the 0% biochar compost and decreased to 9.5 in the 30% biochar compost. The ratio of HA:FA, which indicates the degree of polymerization of humification products, was 1.24 in the 0% biochar compost and increased slightly to 1.36 in the 10% and 20% biochar compost types. A further increase in the biochar content decreased the ratio to 1.26 in the 30% biochar type. The ratio of HA:FA dropped to as low as 0.61 with a further increase in the biochar rate to 40%. There seems to be no influence on the concentration of biochar on CEC as the various compost types had statistically similar CECs that ranged from 62.5 to 71.7 cmolc kg−1.
TABLE 2. Maturity indices of the biochar-compost typesa.
Table 3 shows the electrochemical properties and total basic cations of the compost types. Compost pH tended to increase with increasing biochar addition from 6.5 in the 0% biochar compost to 7.5 in the 40% biochar compost. The addition of at least 20% biochar resulted in at least a significant 0.5 pH unit increase over the non-biochar compost type. Electrical conductivity of the 0% biochar compost was 3.69 dS m−1 and decreased with an increase in biochar percentage to as low as 1.36 dS m−1 in the 40% biochar compost. Total Ca and Mg contents of the compost types increased with an increase in biochar additions and, consequently, the sum of these two basic cations. Total Na and total K, on the contrary, decreased with an increase in biochar percentage. The concentration of K was the highest among the basic cations and ranged from 65.7 g kg−1 in the 0% biochar compost to 44.37 g kg−1 in the 40% biochar compost.
Total and available phosphorus contents of the compost types increased with an increase in biochar percentage (Table 4). Total P was lowest (6,083 mg kg−1) in the 0% biochar compost and increased with an increase in biochar percentage up to 7,530 mg kg−1 in the 40% biochar compost. The available phosphorus content of the compost types was high, ranging from 286.67 mg kg−1 in the 0% biochar compost to 840 mg kg−1 in the 40% biochar compost. The percentage of available phosphorus in total phosphorus was 4.2% in the 0% biochar compost and increased with an increase in biochar percentage up to 11.2% in the 40% biochar compost. It is worthy of note that 30% and 40% addition of GHB to the MS improved P availability by 1.9- and 2.9-folds, respectively, over the non-biochar compost type.
The 0% biochar compost had the lowest concentration of total organic carbon (TOC) (128.1 g kg−1). Total C increased with increasing biochar addition with an approximately 3.2-fold increment in the 40% biochar compost type over the non-biochar type. The total nitrogen contents of the biochar compost types were higher than those of their non-biochar counterparts, with the 40% biochar compost type being almost 2.4 times higher in total N than in the non-biochar compost. Notably, there was no significant difference in total C and N content among the 10, 20, and 30% biochar composts. The carbon:nitrogen (C:N) ratio was lowest in the 0% biochar compost and increased with an increase in biochar percentage up to 24.7 in the 20% biochar compost. Further addition of biochar lowered the C:N ratios. It is worthy of note that among the compost types, only the non-biochar compost and the 40% biochar compost had C:N ratios below 20.
Nitrate-N and ammonium-N and, hence, the available N were highest in the non-biochar compost and decreased significantly with an increase in biochar percentage. The concentrations of NO3−-N in the various composts were higher than those of NH4+-N. The difference in the concentration of the N forms resulted in NH4+/NO3− ratios being below 0.5.
The FT-IR spectra of the various compost types are shown in Figure 4. In the non-biochar compost, two sharp bands were observed at 3695.65 cm−1 and 3621.80 cm−1 of medium-bonded O–H stretch of free alcohols (Hagemann et al., 2018). These bands were absent in the biochar-containing compost types. The band at 3310.74 cm−1, which was present in all the compost types, was assigned to strong broad, intermolecularly bonded O–H stretching of carboxylic, phenolic, and alcohol groups and N–H stretching of aliphatic amines (Yang et al., 2006; Hagemann et al., 2018; Bong et al., 2020). The intensity of this band was highest in the non-biochar compost and lowest in the 10% and 20% biochar composts. The bands at 2917.47 cm−1 and 2851.40 cm−1 were assigned to the C–H stretch of aliphatic methyl and methylene groups (Carballo et al., 2008; Hagemann et al., 2018). The band at 2917.47 cm−1 was present in all the composts, albeit with reduced intensities in the biochar compost types. The band at 2851.40 cm−1 in the non-biochar compost was lost upon biochar addition. On the other hand, a band at 2968.29 cm−1 in biochar compost types, which was also assigned to aliphatic methylene groups (Carballo et al., 2008), appeared. A band at 2,650 cm−1 was present in all the compost types with similar intensity and was assigned to strong intermolecular O–H bonding of carboxylic acids (Sahoo et al., 2012). A medium-bond C=C stretch, indicative of conjugated and unsubstituted alkene, and C=O stretch of carboxylic acids, esters, ketones, and quinones (Carballo et al., 2008), was assigned to the band at 1634.59 cm−1 for the non-biochar compost. In the biochar compost types, this band (1634.59 cm−1) re-centered at 1588.44 cm−1 and was assigned to the medium N–H bend of amine and the medium C=C stretch for cyclic alkene. The band at 1402.80 cm−1 was assigned to O–H deformation and C=O vibration of phenols and COO− vibration of aliphatic deformation (Abouelwafa et al., 2008; Carballo et al., 2008). This band (1402.80 cm−1) shifted and re-centered at 1372.44 cm−1 in the biochar composts. The band at 1034.47 cm−1 in the non-biochar compost was assigned to the strong S=O stretch of sulfoxide. The band re-centered at 1039.32, 1037.08, 1036.67, and 1036.67 cm−1 for the 10, 20, 30, and 40% biochar composts, respectively, indicating a chemical shift. The band at 1005.75 cm−1 in the non-biochar compost could be a strongly bonded C=C for mono-substituted alkene. This band disappeared in the biochar compost types. In all the compost types, the presence of strongly bonded C–H bends, suspected to be mono-, 1-3-di-, and 1,2,3-tri-substituted, was assigned to the bands at 779.73 cm−1 and 690.22 cm−1. Moreover, the band at 690.22 cm−1 was again assigned to a strong C=C band indicative of a benzene derivative. This band (690.22 cm−1) re-centered at higher wavenumbers with the addition of biochar.
Feedstock properties and pyrolysis conditions can influence the properties of biochar and, subsequently, biochar application (Kloss et al., 2012; Spokas et al., 2012). Legumes accumulate high concentrations of N and P because they have a high demand for these nutrients compared to non-leguminous crops under similar cropping systems (Adamu et al., 2014; Anguria et al., 2017; Romanyà and Casals, 2020). Nitrogen and P absorbed by plants are translocated to reproductive regions (Logah et al., 2013; Bender et al., 2015). It, therefore, stands to reason that groundnut husk, which is part of the legume reproductive organ, accumulated higher N and P than the MS. The higher C, N, and P levels in the groundnut husk compared to the MS justified the former’s choice as an ideal feedstock for pyrolysis. With higher C and P, groundnut husk upon charring should give elevated concentrations of these elements under similar pyrolysis conditions. The elevated P could be a source for organic fertilizer formulation. The lower N content of the MS may have contributed to its higher C:N ratio, as noted elsewhere by Ruan et al. (2019) and Kumar and Singh (2018).
Loss of acid functional groups such as the carboxylic groups after charring of groundnut husk as depicted by the FT-IR, which is consistent with the very low content of 1.05 mmol/g carboxylic functional group from the Boehm titration with a concomitant release of soluble basic cations such as K+, Ca2+, and Mg2+, accounted for a higher biochar pH compared to the groundnut feedstock, as noted elsewhere by Novak et al. (2009) and Bourke et al., 2007. With most soils in humid sub-Saharan Africa being acidic, any amendment with a liming potential and high P levels should improve the availability of the macronutrient. The higher pH of the biochar than its feedstock is certainly attributable to the release of approximately 8.04 g/kg soluble K+, Ca2+, and Mg2+ upon charring.
The biochar yield in this study is indicative of biomass reduction, which can be attributed to the loss of volatile constituents such as CO, CO2, H2O, HCN, and NH3, from the thermal degradation of cellulose, hemicellulose, and lignin (Lee et al., 2016; Pawar and Panwar, 2020). The loss of volatile constituents is evident in the decreased O and H contents of the biochar derivative compared with the groundnut husk feedstock. This finding is consistent with the biochar yield from slow pyrolysis reported by Tomczyk et al. (2020) and Fidel et al. (2017). Alkali salts such as carbonates, oxides, and hydroxides of Ca, Mg, Na, and K, together with nutrients such as P, S, N, Fe, and Zn, released upon separation of mineral matter from the organic matrix, constitute the mineral ash content of biochar (Fungai and Sanjai, 2016). Hence, the mineral ash content of biochar is closely correlated with pH, EC, and nutrient status (Lehmann et al., 2011; Uras et al., 2012; Fungai and Sanjai, 2016). The high concentrations of Ca, Mg, and K observed in the present study and the presence of carbonate ions revealed by FT-IR may account for the high pH of the biochar and suggest that the biochar could be used as a liming material in acidic soils (Eduah et al., 2019; Frimpong-Manso et al., 2019). The high pH of the GHB, coupled with its high soluble base content, is a positive attribute that could be exploited in composting. When co-composted with MS, the high pH and soluble bases would mitigate the inhibitory effect of low initial pH on decomposing organisms and promote a shorter composting duration.
High salt concentrations that reflect a high EC can inhibit seed germination and plant growth through water stress, salt stress, and nutrient imbalances (Al-Wabel et al., 2013; Tomczyk et al., 2020). The release of Ca, Mg, and K from the organic matrix may have accounted for the higher GHB EC, which was twice that of the feedstock. The EC of the biochar, being below 2 dS m−1, is considered non-saline (Hazelton and Murphy, 2016). Thus, the biochar produced can be used as a growth medium, soil amendment, or feedstock to produce non-saline compost.
Oxygen-containing surface functional groups account for the CEC of biochar (Sohi et al., 2010; Briggs et al., 2012). Carboxylic acids (COOH) have pKa values lower than 5.0, above which deprotonation occurs to yield carboxylate (COO−) ions, one of the main sources of CEC. Similarly, phenolic groups deprotonate at pH around 9.0 and contribute to negative charges for increased CEC (Banik et al., 2018; Eduah et al., 2019; Tomczyk et al., 2020). Considering the fact that the concentration of the carboxylic functional groups (1.05 mmol g−1) in the biochar was just a paltry 2.7% of the phenolic group, the contribution toward negative charge development (CEC) is more attributable to the phenolic groups. At the biochar pH of 9.74, more than half of the phenolic group will deprotonate for negative charge development. When GHB is applied to acidic soils, the equilibrium pH would be lower than the pKa (9.8) of the phenolic group (Evangelou, 1998), and this may not favor the deprotonation of the phenolic group. As such, the CEC of the amended soils will only be marginally improved owing to the low content of carboxylic functional groups.
The soluble bases, 52% ash content, and high pH of the biochar would promote surface precipitation of liberated P, and that may, in part, account for the very high total P of 3,400 mg kg−1, of which only 22% is available. Nevertheless, the available P content of the biochar is high enough to sustain crop cultivation should the amendment be applied in the appropriate form. The MS with its inherent lower pH (5.75) during co-composting with GHB will release more organic acids to minimize surface precipitation of P onto biochar by complexing with the cations in the ash of the biochar. The decomposing MS will, in addition, provide more carboxylate ions to repel phosphate anions into the soil solution to increase the availability of P when the soil is amended with the biochar compost. Co-composting GHB with MS should, therefore, provide a better P amendment than sole biochar or sole MS compost.
The increase in C concentration can be attributed to carbonization, whereas losses of H and O atoms may be due to the dehydration of groundnut husk. These two processes subsequently concentrate carbon in the biochar (Guo and Rockstraw, 2007). The ratios of H:C and O:C have been used to predict the stability of biochar in soil (Spokas, 2010; Budai et al., 2013; 2016). The reduction in the H:C ratio is an indication of the loss of alkyl C groups and the formation of aromatic C compounds in the biochar. According to Budai et al. (2013), approximately 70% of biochar would persist for more than 100 years in soil if the H:C ratio is at most 0.4. The H:C ratio of 0.04 reported in this study implies that the biochar is stable and that more than 70% would remain for at least 100 years when applied to soil. The O:C ratio of 0.43 suggests that the biochar produced has a half-life of between 100 and 1,000 years (Spokas, 2010), which will promote carbon sequestration in the fragile soils of sub-Saharan Africa.
Pyrolysis of biomass results in the conversion of organically bound phosphorus in feedstocks into plant-available forms at temperatures below 400°C (Xu et al., 2016). The plant-available forms of P are, however, converted to unavailable forms at temperatures between 400°C and 600°C (Figueiredo et al., 2021; Jiang et al., 2019; G; Xu et al., 2016). Phosphorus in biochar may become completely unavailable when pyrolyzed at temperatures above 700°C (Figueiredo et al., 2021; Jiang et al., 2019; G; Xu et al., 2016). In the present study, biochar was produced at a temperature of approximately 450°C, accounting for the very high total P, of which only 22% is in the available form.
The high C:N ratio of the biochar can be attributed to a high increase in C because of carbonization. Application of biochar with such a high C:N ratio would result in immobilization of N and possibly N starvation of plants, especially in N-deficient soils (Fungai and Sanjai, 2016). In the present study, nitrogen from decomposing cow dung was, thus, added to the farm residue composting heaps, with or without biochar, to facilitate decomposition.
According to Keiluweit et al. (2010) and Rutherford et al. (2012), decomposition of aliphatic carboxylic groups and aromatization of carbon lead to the formation of phenolic and/or amine groups of higher bond energy. Thus, the increased intensity and chemical shift of the broad OH-stretching vibration can be attributed to increased phenolic and/or amine groups formed from dehydration and decarboxylation reaction products of C–H, C=O, and O–H groups (Angın, 2013). This assertion was confirmed by the results of the Boehm titration, which revealed a relatively higher concentration of the phenolic and lactonic groups than the carboxylic group. The intensification of 1,630 cm−1 upon pyrolysis suggests an enrichment in aromatic groups containing amines. Thus, inorganic N released may have reacted with C=O groups to form more stable products (Chen et al., 2017; 2018). The loss of the bands at 2,920 cm−1 and 1739.40 cm−1 upon pyrolysis can be attributed to the decomposition of aliphatic carboxylic groups. Similar results have been reported by Rutherford et al. (2012) and Keiluweit et al. (2010). The retention of C–O and C–N groups can be attributed to the lignin component as biochar was produced at a temperature lower than the ceiling for lignin decomposition (Babu, 2008). Inorganic phosphate, carbonate, and nitrate ions identified are attributed to the presence of these anions in the mineral ash of the biochar produced (Montes-Morán et al., 2004; Carrier et al., 2012). Inorganic phosphates have been reported to be completely absent at temperatures above 700°C (Christel et al., 2014). Thus, considering the temperature (454 °C) at which the biochar was produced in this study, the presence of inorganic phosphate and carbonate was expected.
The initial increase in temperature during composting is indicative of active microbial decomposition in the various heaps. Consequently, the early initial increase in temperature of the heap with 20% biochar can be attributed to the neutralization of organic acids produced at the initial decomposition stage by the alkalinity of biochar (Akumah et al., 2021). The 1-day delay of the 0% and 10% biochar heaps suggests that the alkalinity of the 10% was not enough to neutralize the organic acids produced during MS decomposition and that similar microbial activities prevailed in both heaps. The delayed increase in temperature of the 30% and 40% biochar heaps can be attributed to the higher proportions of unavailable N associated with these higher biochar loading rates. The high N concentration in the 30% and 40% biochar heaps may have lowered the C:N ratio. However, these N forms are recalcitrant to microbial attack, culminating in lowered microbial activity in these heaps. Moreover, the absorption of moisture at these high biochar rates may have lowered the moisture at the interface between decomposing microbes and the MS, thereby reducing microbial activity (Antonangelo et al., 2021). Thus, in the composting of stover–biochar mix, moistening of heaps to higher contents (>60%) may be required. The temperature profiles of the compost heaps in this study (Figure 2; Figure 3) suggest that the composting process occurred in the mesophilic range. The dry form of the maize stover may have suppressed microbial activity and, consequently, the composting process.
GI, a measure of compost phytotoxicity, is mainly used to determine compost maturity (Luo et al., 2018). Mature composts must have very little or no phytotoxic effect on germinating seeds, in addition to their ability to supply nutrients. When cress or tomato seeds are used, values of 100 and above are accepted for commercially produced composts intended for use as growth media. Lower values are acceptable for composts to be used as soil amendments (Hase and Kawamura, 2012; Warman, 2013; Luo et al., 2018). In the present study, all the composts scored more than 100 for germination index, which indicates that the various compost types were non-phytotoxic and, hence, matured.
The humification process during composting is often used to ascertain compost maturity. Humic acid-to-fulvic acid ratio (HA:FA) is an index of humification that describes the progress of HA-to-FA transformation during composting. A ratio ranging from 3.6 to 6.2 has been recommended for mature composts (Hase and Kawamura, 2012; Zhou et al., 2014). The ratio in the present study (0.61–1.36) suggests that the composts contain nearly equal amounts of fulvic and humic acids and that they would undergo further humification when applied to soils. The results also indicate that the 40% biochar-compost was the least humified. Perhaps, the predominant recalcitrant biochar N and C in the 40% biochar heap accounted for low humification. This compost type, when applied to the degraded soils of the Guinea Savanna zone, is likely to improve their carbon status as it will persist in the soil relatively longer.
Humic acid and fulvic acid contain surface functional groups, of which carboxylic groups dominate in the latter. The complete dissociation of protons in carboxyl and phenolic groups results in the formation of negatively charged surfaces. The negative charges developed constitute the CEC of the compost. Thus, the high CEC (62.5–66.7 cmol kg−1) of the compost types can be attributed to deprotonation of the abundant carboxyl group as the pH was below the pKa of phenolic functional groups. According to the CEC rating by Harada and Inoko (1980b), mature composts have CEC >60 cmol kg−1. Thus, the composts from this study with CEC >60 cmol kg−1 can be considered mature. The statistically similar CEC of the five compost types despite the varying proportion of biochar applied implies that the contribution of biochar to charge development in the compost was negligible. Perhaps with the aging of the biochar in soils, the positive effects on CEC would manifest. The fact that the compost types are still undergoing humification implies that, with time, the CEC would increase to improve upon cationic nutrient storage. The use of these biochar compost types holds promise as P-enriched organic amendments in sub-Saharan Africa. With humification, the availability of P may increase with time, implying that residual P may be higher in subsequent seasons. Therefore, there may not be a need for annual applications, which is the main drawback of organic farming in the tropical environment. It is, however, imperative for trials to be carried out to determine when to reapply the amendment for sustainable crop growth. Again, with the high available P contents but very low available N, these biochar compost types could be exploited for use in legume production in the P-deficient ferruginous soils of the Guinea Savanna zone of Ghana. Phosphorus would be made more available, and the legumes would experience N deficiency, which could induce N fixation.
However, co-application of these compost types with cationic micronutrients such as Zn, Cu, and Mn into soils is not encouraged, as the readily available P may promote precipitation of the metals. The high CEC of these compost types will repel H2PO4− and HPO42- in the compost. These anions will then persist in the soil solution to precipitate any metal that may be available. Co-application will rather promote metallic micronutrients and P deficiency. Preferably, in the use of biochar compost amendments, micronutrients should be applied as foliar fertilizers.
The pH of compost types in the present study increased with an increase in biochar percentage and was within the acceptable range (6.5–7.5) for crop production (Anthonis, 1994). The increase in pH with an increase in biochar percentage can be attributed to the release of alkaline compounds present in the biochar, as was revealed by a strong positive correlation between biochar pH and the sum of Ca and Mg (Supplementary Figure S1). According to the regression analysis, the sum of Ca and Mg accounted for 75% of the increase in compost pH (Supplementary Figure S1).
The aeration of the heaps in this study may have contributed to increased nitrification and consequently reduced NH4+ accumulation in the final composts. Although the composts produced in the present study were not alkaline, they can be applied to extremely acidic soils, such as Oxisols and Ultisols, to serve as liming materials. Application of these compost types to neutral soils would increase their pH buffering capacity due to their high CEC, as noted elsewhere by Antonangelo et al. (2021). The neutral nature of the compost types will minimize the volatilization of ammonium to preserve the already low N in the various amendments. However, it is expected that with further humification, the available N contents will increase. The neutral pH of the compost types is a result of the high nitrification rates of the amendments, as reflected in the very low NH4+:NO3− ratios of less than 0.4. High nitrification will culminate in the release of protons, which will nullify alkalinity.
Another parameter used to assess compost maturity is the nitrification index (NH4+–N:NO3−1–N ratio) (Rashad et al., 2010). Composts with NH4+:NO3− lower than 1 are free of the phytotoxic effect of NH4+ (Abouelwafa et al., 2008; Tumuhairwe et al., 2009; Vergnoux et al., 2009). Final compost ratings of NH4+–N:NO3−–N ratio of <0.5, between 0.5 and 3.0, and >3.0 are indicative of a very mature, mature, and immature compost, respectively (Das et al., 2011). All the composts had NH4+–N/NO3−1–N ratios <0.5, confirming that all the composts were very mature.
A significant positive correlation existed between EC and K (0.96**) and EC and Na (0.92**) in the compost types, as evident in Supplementary Figure S2, S3, respectively, indicating that the EC of the amendments was largely controlled by their K and Na contents. Approximately 92% and 85% of the EC can be attributed to K and Na, respectively. Thus, the Na and K contents of the MS contributed to the high EC of the non-biochar compost. The lower EC of the biochar-compost types could be attributed to the dilution effect of the GHB. Compost EC is one of the important properties that determines its quality and, therefore, class. An EC value of at most 4.0 dS m−1 is recommended for general-purpose composts and for landscaping (Lasaridi et al., 2006; Gondek et al., 2020). The various composts had EC values lower than the critical value of 4.0 dS m−1, suggesting that they can be applied as soil amendments. The non-biochar-compost and the 10% biochar compost types, which have ECs of 3.69 dS m−1 and 3.54 dS m−1, respectively, close to the critical limit, could be used with moderation for landscaping and not for the cultivation of crops to avoid salt injury and destruction of soil structure. The other biochar-compost types, particularly those with 30% and 40% biochar loading rates, could be used for crop production without fear of salt injury or salinity problems. The similarity in the EC values for the non-biochar compost and the 10% biochar compost and the fact that their P contents are marginally different implies that in the preparation of the latter, cost must be factored in. Would the additional cost incurred in charring groundnut husk to co-compost with MS compensate for the additional available P compared to the non-biochar compost type?
Increasing the biochar loading rate resulted in a high total organic C content of the compost due to the high levels of C in the biochar. In addition, the reduced decomposition upon incorporation of biochar into the compost was evident in the higher C:N ratios of the biochar-compost as compared with the non-biochar counterparts.
Total N retention in the groundnut husk biochar vis-à-vis the increasing loading rate resulted in the high total N content of the biochar-compost. The ratio of carbon to nitrogen is used as an indicator of the stability of mature composts (Bernal et al., 2009). Values around 20 show a satisfactory maturity of composts (Li et al., 2015). The slightly higher values for the biochar-containing compost can be attributed to the recalcitrant C content of the biochar added. The differences in the C:N ratio further suggest that the various composts would be mineralized to varying extents when applied to soil. For early-maturing crops such as corchorus, which is a leafy vegetable popular in the diet of people in the Guinea Savanna zone of Ghana, the compost of choice should be the non-biochar types as they would mineralize faster to release N for plant uptake. The non-biochar compost type would be characterized by faster release of N because it had the highest available N and the least C:N ratio of 12.8. It should, however, be used with regular monitoring of the soil’s EC to avoid destruction of the structure of the soil. For tree crops and long-duration crops, the biochar compost types should be preferred. The biochar-composts, particularly the 20% biochar type, would be more appropriate for carbon sequestration because of their relatively high C:N ratio. The decrease in the available N content of the compost types with an increase in biochar percentages can be attributed to the effect of biochar on reducing degradation (Khan et al., 2014). The high proportion of carbon in the biochar may have accounted for the reduced mineralization of the MS and the resultant lower available N with an increased biochar percentage.
Biochar addition resulted in the loss of free hydroxyl groups in the final compost, which can be attributed to the loss of water caused by aeration in the biochar-containing compost types. The loss of the aliphatic groups can be attributed to microbial degradation due to their low recalcitrance (Barje et al., 2012). The chemical shifts observed in the bands of the biochar-compost types suggest surface modifications, which can be attributed to the adsorption of NH4+ onto biochar, as mentioned by Barje et al. (2012).
This study showed that MS and groundnut husk, which abound in the Guinea Savanna zone of Ghana, could be harnessed into sustainable production of P-enriched organic amendments to reduce the import bill of fertilizers while closing the nutrient loop. This may also reduce nutrient miming to minimize soil fertility decline in the soils of the Guinea Savanna, particularly when amendments are applied to soils from which the feedstocks are harvested.
Biochar addition affected the composting process. Germination index, HA:FA, and CEC showed that all the compost types were non-phytotoxic after 10 weeks of composting. Excessive dissipation of heat associated with the bulky nature of the feedstocks favored mesophilic activities during composting. The addition of GHB to the MS improved P availability by 1.9- and 2.9-folds, respectively, over the non-biochar compost type.
This study indicates that composting MS alone or co-composting MS with 10% GHB is not ideal for producing good-quality compost. Good-quality compost with low EC, very high available P, and high CEC for crop cultivation and the ability to sequester carbon could be produced for use in the Guinea Savanna zone of northern Ghana by co-composting 40% groundnut husk charred in the Kuntan kiln with MS.
The raw data supporting the conclusion of this article will be made available by the authors, without undue reservation.
The first author, DF, carried out the investigation as part of his Doctor of Philosophy thesis and prepared the original draft. Conceptualization, grant acquisition, methodology, supervision, writing, and reviewing and editing were performed by the corresponding author, EN. MA, TA, and IL were also supervisors and assisted in the methodology, curation of data, and the original write-up. CA assisted with grant acquisition, data curation, review and editing of the manuscript, and project administration. SA-B, AA, NS, and MB assisted with review and editing of the manuscript. All authors contributed to the article and approved the submitted version.
This research work was funded by the USAID/University of Ghana Feed the Future project (grant number 641-002-FY14-IL#02).
The authors acknowledge the assistance of the Director and staff of the Ministry of Food and Agriculture office in the Lawra Municipality, Ghana.
The authors declare that the research was conducted in the absence of any commercial or financial relationships that could be construed as a potential conflict of interest.
All claims expressed in this article are solely those of the authors and do not necessarily represent those of their affiliated organizations, or those of the publisher, the editors, and the reviewers. Any product that may be evaluated in this article, or claim that may be made by its manufacturer, is not guaranteed or endorsed by the publisher.
The Supplementary Material for this article can be found online at: https://www.frontiersin.org/articles/10.3389/fenvs.2023.1252305/full#supplementary-material
Abouelwafa, R., Amir, S., Souabi, S., Winterton, P., Ndira, V., Revel, J. C., et al. (2008). The fulvic acid fraction as it changes in the mature phase of vegetable oil-mill sludge and domestic waste composting. Bioresour. Technol. 99 (14), 6112–6118. doi:10.1016/J.BIORTECH.2007.12.033
Adamu, U. K., Almu, H., Adam, I. A., and Sani, S. A. (2014). Evaluation of nutrient composition of some cereals and legumes crops residues as compost materials. Bayero J. Pure Appl. Sci. 7 (2), 52–54. doi:10.4314/bajopas.v7i2.10
Ahad, T., Kanth, T. A., and Nahi, S. (2015). Soil bulk density as related to texture, organic matter content and porosity in Kandi soils of district Kupwara (Kashmir valley), India. Int. J. Sci. Res. 4 (1), 198–200.
Akumah, A. M., Nartey, E. K., Ofosu-Budu, G. K., Ewusie, E. A., Offei, B. K., and Adamtey, N. (2021). Innovations in market crop waste compost production: Use of black soldier fly larvae and biochar. Int. J. Recycl. Org. Waste Agric. doi:10.30486/ijrowa.2021.1899111.1071
Al-Wabel, M. I., Al-Omran, A., El-naggar, A. H., Nadeem, M., and Usman, A. R. A. A. (2013). Pyrolysis temperature induced changes in characteristics and chemical composition of biochar produced from conocarpus wastes. Bioresour. Technol. 131, 374–379. doi:10.1016/j.biortech.2012.12.165
Amoah, S. T., and Kosoe, E. A. (2014). Solid waste management in urban areas of Ghana: Issues and experiences from wa. J. Environ. Pollut. Hum. Health 2 (5), 110–117. doi:10.12691/jephh-2-5-3
Angın, D. (2013). Effect of pyrolysis temperature and heating rate on biochar obtained from pyrolysis of safflower seed press cake. Bioresour. Technol. 128, 593–597. doi:10.1016/j.biortech.2012.10.150
Anguria, P., Chemining’wa, G. N., Onwonga, R. N., and Ugen, M. A. (2017). Decomposition and nutrient release of selected cereal and legume crop residues. J. Agric. Sci. 9 (6), 108. doi:10.5539/jas.v9n6p108
Anthonis, G. (1994). Standards for organic fertilizer. Agro-Chemicals News Brief (ESCAP/FAO/UNIDO 17 (2), 12–15.
Antonangelo, A., Sun, X., and Zhang, H. (2021). The roles of co-composted biochar (COMBI) in improving soil quality, crop productivity, and toxic metal amelioration ☆. J. Environ. Manag. 277, 111443. doi:10.1016/j.jenvman.2020.111443
ASTM D1762-84 (2007). Standard test method for chemical analysis of wood charcoal. doi:10.1520/D1762-84R07
Babu, B. V. (2008). Biomass pyrolysis: A state-of- the-art review. Hoboken, New Jersey, United States: Wiley, 393–414. doi:10.1002/bbb.92
Banik, C., Lawrinenko, M., Bakshi, S., and Laird, D. A. (2018). Impact of pyrolysis temperature and feedstock on surface charge and functional group chemistry of biochars. J. Environ. Qual. 47 (3), 452–461. doi:10.2134/JEQ2017.11.0432
Barje, F., El Fels, L., El Hajjouji, H., Amir, S., Winterton, P., and Hafidi, M. (2012). Molecular behaviour of humic acid-like substances during co-composting of olive mill waste and the organic part of municipal solid waste. Int. Biodeterior. Biodegrad. 74, 17–23. doi:10.1016/J.IBIOD.2012.07.004
Batham, M., Gupta, R., and Tiwari, A. (2013). Implementation of bulking agents in composting: A review. J. Bioremediation Biodegrad. 4 (205). doi:10.4172/2155-6199.1000205
Bazrafshan, E., Zarei, A., Mostafapour, F. K., Poormollae, N., Mahmoodi, S., and Zazouli, M. A. (2016). Maturity and stability evaluation of composted municipal solid wastes. Health Scope 5 (1). doi:10.17795/jhealthscope-33202
Bender, R. R., Haegele, J. W., and Below, F. E. (2015). Nutrient uptake, partitioning, and remobilization in modern soybean varieties. Agron. J. 107 (2), 563–573. doi:10.2134/AGRONJ14.0435
Bernal, M. P., Alburquerque, J. A., and Moral, R. (2009). Composting of animal manures and chemical criteria for compost maturity assessment. A review. Bioresour. Technol. 100 (22), 5444–5453. doi:10.1016/j.biortech.2008.11.027
Bong, C. P. C., Lim, L. Y., Lee, C. T., Ong, P. Y., Klemeš, J. J., Li, C., et al. (2020). Lignocellulosic biomass and food waste for biochar production and application: A review. Chem. Eng. Trans. 81, 427–432. doi:10.3303/CET2081072
Bourke, J., Manley-Harris, M., Fushimi, C., Dowaki, K., Nunoura, T., and Antal, M. J. (2007). Do all carbonized charcoals have the same chemical structure? 2. A model of the chemical structure of carbonized charcoal. Industrial Eng. Chem. Res. 46 (18), 5954–5967. doi:10.1021/ie070415u
Briggs, C., Breiner, J. M., and Graham, R. C. (2012). Physical and chemical properties of Pinus ponderosa charcoal: Implications for soil modification. Soil Sci. 177 (4), 263–268. doi:10.1097/SS.0B013E3182482784
Budai, A., Rasse, D. P., Lagomarsino, A., Lerch, T. Z., and Paruch, L. (2016). Biochar persistence, priming and microbial responses to pyrolysis temperature series. Biol. Fertil. Soils 52 (6), 749–761. doi:10.1007/s00374-016-1116-6
Budai, A., Zimmerman, A. R., Cowie, A. L., Webber, J. B. W., Singh, B. P., Glaser, B., et al. (2013). Biochar Carbon Stability Test Method: An assessment of methods to determine biochar carbon stability. Int. Biochar Initiat., 1–10.
Carballo, T., Gil, M. V., Gómez, X., González-Andrés, F., and Morán, A. (2008). Characterization of different compost extracts using Fourier-transform infrared spectroscopy (FTIR) and thermal analysis. Biodegradation 19 (6), 815–830. doi:10.1007/s10532-008-9184-4
Carrier, M., Hardie, A. G., Uras, Ü., Görgens, J., and Knoetze, J. (2012). Production of char from vacuum pyrolysis of South-African sugar cane bagasse and its characterization as activated carbon and biochar. J. Anal. Appl. Pyrolysis 96, 24–32. doi:10.1016/J.JAAP.2012.02.016
Chen, W., Yang, H., Chen, Y., Li, K., Xia, M., and Chen, H. (2018). Influence of biochar addition on nitrogen transformation during copyrolysis of algae and lignocellulosic biomass. Environ. Sci. Technol. 52 (16), 9514–9521. doi:10.1021/acs.est.8b02485
Chen, W., Yang, H., Chen, Y., Xia, M., Chen, X., and Chen, H. (2017). Transformation of nitrogen and evolution of N-containing species during algae pyrolysis. Environ. Sci. Technol. 51 (11), 6570–6579. doi:10.1021/acs.est.7b00434
Ch’Ng, H. Y., Ahmed, O. H., and Majid, N. M. A. (2014). Improving phosphorus availability in an acid soil using organic amendments produced from agroindustrial wastes. Sci. World J. 2014, 1–6. doi:10.1155/2014/506356
Christel, W., Bruun, S., Magid, J., and Jensen, L. S. (2014). Phosphorus availability from the solid fraction of pig slurry is altered by composting or thermal treatment. Bioresour. Technol. 169, 543–551. doi:10.1016/J.BIORTECH.2014.07.030
Chun, Y., Sheng, G., Chiou, G. T., and Xing, B. (2004). Compositions and sorptive properties of crop residue-derived chars. Environ. Sci. Technol. 38 (17), 4649–4655. doi:10.1021/ES035034W
Das, M., Uppal, H. S., Singh, R., Beri, S., Mohan, K. S., Gupta, V. C., et al. (2011). Co-composting of physic nut (Jatropha curcas) deoiled cake with rice straw and different animal dung. Bioresour. Technol. 102 (11), 6541–6546. doi:10.1016/J.BIORTECH.2011.03.058
Duarte, R. M. B. O., and Duarte, A. C. (2019). “Geochemistry | Soil, organic components,” in Encyclopedia of analytical science. Third Edit (Amsterdam, Netherlands: Elsevier). doi:10.1016/B978-0-12-409547-2.14328-8
Eder, S., Torko, M., Montalbetti, A., Azzari, P., and Nyström, L. (2021). Pigeon pea husk for removal of emerging contaminants trimethoprim and atenolol from water. Molecules 26 (11), 3158. doi:10.3390/MOLECULES26113158
Eduah, J. O., Nartey, E. K., Abekoe, M. K., Breuning-Madsen, H., and Andersen, M. N. (2019). Phosphorus retention and availability in three contrasting soils amended with rice husk and corn cob biochar at varying pyrolysis temperatures. Geoderma 341, 10–17. doi:10.1016/j.geoderma.2019.01.016
El Fels, L., Zamama, M., El Asli, A., and Hafidi, M. (2014). Assessment of biotransformation of organic matter during co-composting of sewage sludge-lignocelullosic waste by chemical, FTIR analyses, and phytotoxicity tests. Int. Biodeterior. Biodegrad. 87, 128–137. doi:10.1016/J.IBIOD.2013.09.024
Evangelou, V. P. (1998). Environmental soil and water chemistry: Principles and applications. Hoboken, New Jersey, United States: John Wiley & Sons, Inc.
Fidel, R. B., Laird, D. A., Thompson, M. L., and Lawrinenko, M. (2017). Characterization and quantification of biochar alkalinity. Chemosphere 167, 367–373. doi:10.1016/J.CHEMOSPHERE.2016.09.151
Figueiredo, C. C. de, Reis, A. de S. P. J., Araujo, A. S. de, Blum, L. E. B., Shah, K., and Paz-Ferreiro, J. (2021). Assessing the potential of sewage sludge-derived biochar as a novel phosphorus fertilizer: Influence of extractant solutions and pyrolysis temperatures. Waste Manag. 124, 144–153. doi:10.1016/J.WASMAN.2021.01.044
Fischer, D., and Glaser, B. (2012). Synergisms between compost and biochar for sustainable soil amelioration. Manag. Org. Waste. doi:10.5772/31200
Frimpong-Manso, E., Adjadeh, T. A., and Darko, D. A. (2019). Use of corn cob and rice husk biochar as liming materials in acid soils. West Afr. J. Appl. Ecol. 27 (2), 32–50.
Fungai, M. N. D., and Sanjai, P. J. (2016). “Chemical, physical, and surface characterization of biochar,” in Biochar production, characterization, and applications. Editors Y. S. Ok, S. M. Uchimiya, S. X. Chang, and N. Bolan (Oxfordshire United Kingdom: Taylor & Francis Group), 68–96.
Gabhane, J., William, S. P., Bidyadhar, R., Bhilawe, P., Anand, D., Vaidya, A. N., et al. (2012). Additives aided composting of green waste: Effects on organic matter degradation, compost maturity, and quality of the finished compost. Bioresour. Technol. 114, 382–388. doi:10.1016/j.biortech.2012.02.040
Gan, H. Y., Schöning, I., Schall, P., Ammer, C., and Schrumpf, M. (2020). Soil organic matter mineralization as driven by nutrient stoichiometry in soils under differently managed forest stands. Front. For. Glob. Change 3, 1–15. doi:10.3389/ffgc.2020.00099
Gondek, M., Weindorf, D. C., Thiel, C., and Kleinheinz, G. (2020). Soluble salts in compost and their effects on soil and plants: A review. Compost Sci. Util. 28 (2), 59–75. doi:10.1080/1065657X.2020.1772906
Guo, Y., and Rockstraw, D. A. (2007). Physicochemical properties of carbons prepared from pecan shell by phosphoric acid activation. Bioresour. Technol. 98 (8), 1513–1521. doi:10.1016/J.BIORTECH.2006.06.027
Hagemann, N., Subdiaga, E., Orsetti, S., de la Rosa, J. M., Knicker, H., Schmidt, H. P., et al. (2018). Effect of biochar amendment on compost organic matter composition following aerobic composting of manure. Sci. Total Environ. 613–614, 20–29. doi:10.1016/j.scitotenv.2017.08.161
Harada, Y., and Inoko, A. (1980a). Relationship between cation-exchange capacity and degree of maturity of city refuse composts. Soil Sci. Plant Nutr. 26 (3), 353–362. doi:10.1080/00380768.1980.10431220
Harada, Y., and Inoko, A. (1980b). The measurement of the cation-exchange capacity of composts for the estimation of the degree of maturity. Soil Sci. Plant Nutr. 26 (1), 127–134. doi:10.1080/00380768.1980.10433219
Hase, T., and Kawamura, K. (2012). Evaluating compost maturity with a newly proposed index based on a germination test using Komatsuna (Brassica rapa var. peruviridis) seeds. J. Material Cycles Waste Manag. 14, 220–227. doi:10.1007/s10163-012-0063-z
Hazelton, P., and Murphy, B. (2016). Interpreting soil test results: What do all the numbers mean? 3rd ed. Canberra, Australia: CSIRO.
IFDC (2017). Feed the future soil fertility technology (SFT) adoption, policy reform and knowledge management project. https://ifdc.org/projects/feed-the-future-soil-fertility-technology-sft-adoption-policy-reform-and-knowledge-management-project/.
Jiang, Y., Ren, C., Guo, H., Guo, M., and Li, W. (2019). Speciation transformation of phosphorus in poultry litter during pyrolysis: Insights from X-ray diffraction, fourier transform infrared, and solid-state NMR spectroscopy. Environ. Sci. Technol. 53 (23), 13841–13849. doi:10.1021/acs.est.9b03261
Keiluweit, M., Nico, P. S., Johnson, M., and Kleber, M. (2010). Dynamic molecular structure of plant biomass-derived black carbon (biochar). Environ. Sci. Technol. 44 (4), 1247–1253. doi:10.1021/es9031419
Khan, N., Clark, I., Sánchez-monedero, M. A., Shea, S., Meier, S., and Bolan, N. (2014). Maturity indices in co-composting of chicken manure and sawdust with biochar. Bioresour. Technol. 168, 245–251. doi:10.1016/j.biortech.2014.02.123
Kloss, S., Zehetner, F., Dellantonio, A., Hamid, R., Ottner, F., Liedtke, V., et al. (2012). Characterization of slow pyrolysis biochars: Effects of feedstocks and pyrolysis temperature on biochar properties. J. Environ. Qual. 41 (4), 990–1000. doi:10.2134/JEQ2011.0070
Kumar, D., and Singh, V. (2018). “Bioethanol production from corn,” in Corn: Chemistry and Technology. 3rd Edition (Amsterdam, Netherlands: Elsevier Inc). doi:10.1016/B978-0-12-811971-6.00022-X
Lasaridi, K., Protopapa, I., Kotsou, M., Pilidis, G., Manios, T., and Kyriacou, A. (2006). Quality assessment of composts in the Greek market: The need for standards and quality assurance. J. Environ. Manag. 80 (1), 58–65. doi:10.1016/J.JENVMAN.2005.08.011
Lee, J. W., Hawkins, B., Kidder, M. K., Evans, B. R., Buchanan, A. C., and Day, D. (2016). Characterization of biochars produced from peanut hulls and pine wood with different pyrolysis conditions. Bioresour. Bioprocess. 3, 15. doi:10.1186/s40643-016-0092-x
Lehmann, J., Rillig, M. C., Thies, J., Masiello, C. A., Hockaday, W. C., and Crowley, D. (2011). Biochar effects on soil biota - a review. Soil Biol. Biochem. 43 (9), 1812–1836. doi:10.1016/j.soilbio.2011.04.022
Li, R., Wang, Q., Zhang, Z., Zhang, G., Li, Z., Wang, L., et al. (2015). Nutrient transformation during aerobic composting of pig manure with biochar prepared at different temperatures. Environ. Technol. (United Kingdom) 36 (7), 815–826. doi:10.1080/09593330.2014.963692
Logah, V., Atobrah, V., Essel, B., Bosomtwe, A., and Acquah, A. (2013). Phosphorus uptake and partitioning in maize as affected by tillage on Dystric Cambisol and Ferric Acrisol in Ghana. J. Ghana Sci. Assoc. 15 (1), 23. https://www.researchgate.net/publication/311494336.
Luo, Y., Liang, J., Zeng, G., Chen, M., Mo, D., Li, G., et al. (2018). Seed germination test for toxicity evaluation of compost: Its roles, problems and prospects. Waste Manag. 71, 109–114. doi:10.1016/j.wasman.2017.09.023
Mokolopi, B. G. (2022). Groundnut shells as a potential feed supplement for ruminants on pastures: A review. Indian J. Animal Res. 56 (5), 521–524.
Montes-Morán, M. A., Suárez, D., Menéndez, J. A., and Fuente, E. (2004). On the nature of basic sites on carbon surfaces: An overview. Carbon 42 (7), 1219–1225. doi:10.1016/J.CARBON.2004.01.023
Murphy, J., and Riley, J. P. (1962). A modified single solution method for the determination of phosphate in natural waters. Acta, Anal. Chim. 27, 31–36. doi:10.1016/s0003-2670(00)88444-5
Novak, J., Lima, I., Xing, B., Gaskin, J., Steiner, C., Das, K., et al. (2009). Characterization of designer biochar produced at different temperatures and their effects on a loamy sand. Ann. Environ. Sci. 3 (1), 195–206.
Oliveira, M., Rebac, D., Coutinho, J., Ferreira, L., and Trindade, H. (2020). Nitrogen mineralization of legume residues: Interactions between species, temperature and placement in soil. Span. J. Agric. Res. 18 (1), e1101. doi:10.5424/sjar/2020181-15174
Oorts, K., Vanlauwe, B., and Merckx, R. (2003). Cation exchange capacities of soil organic matter fractions in a Ferric Lixisol with different organic matter inputs. Agric. Ecosyst. Environ. 100 (2–3), 161–171. doi:10.1016/S0167-8809(03)00190-7
Ossman, M., Mansour, M., Fattah, M., Taha, N., and Kiros, Y. (2014). Peanut shells and talc powder for removal of hexavalent chromium from aqueous solutions. Bulg. Chem. Commun. 46 (3), 629–639.
Pawar, A., and Panwar, N. L. (2020). Experimental investigation on biochar from groundnut shell in a continuous production system. Biomass Convers. Biorefinery, March 12, 1093–1103. doi:10.1007/s13399-020-00675-4
Raheem, D., Dayoub, M., Birech, R., and Nakiyemba, A. (2021). The contribution of cereal grains to food security and sustainability in Africa: Potential application of UAV in Ghana, Nigeria, Uganda, and Namibia. Urban Sci. 5 (1), 8. doi:10.3390/urbansci5010008
Rashad, F. M., Saleh, W. D., and Moselhy, M. A. (2010). Bioconversion of rice straw and certain agro-industrial wastes to amendments for organic farming systems: 1. Composting, quality, stability and maturity indices. Bioresour. Technol. 101 (15), 5952–5960. doi:10.1016/J.BIORTECH.2010.02.103
Romanyà, J., and Casals, P. (2020). Biological nitrogen fixation response to soil fertility is species-dependent in annual legumes. J. Soil Sci. Plant Nutr. 20 (2), 546–556. doi:10.1007/s42729-019-00144-6
Ruan, Z., Wang, X., Liu, Y., and Liao, W. (2019). “Corn,” in Integrated processing technologies for Food and agricultural by-products (Amsterdam, Netherlands: Elsevier Inc). doi:10.1016/B978-0-12-814138-0.00003-4
Rutherford, D. W., Wershaw, R. L., Rostad, C. E., and Kelly, C. N. (2012). Effect of formation conditions on biochars: Compositional and structural properties of cellulose, lignin, and pine biochars. Biomass Bioenergy 46, 693–701. doi:10.1016/J.BIOMBIOE.2012.06.026
Sahoo, S., Ck, C., Pk, B., and Sc, M. (2012). FTIR and Raman spectroscopic investigations of a norfloxacin/carbopol934 polymerie suspension. J. Young Pharm. 4 (3), 138–145. doi:10.4103/0975-1483.100017
Schwanninger, M., Hinterstoisser, B., Gierlinger, N., Wimmer, R., and Hanger, J. (2004). Application of Fourier transform near infrared spectroscopy (FT-NIR) to thermally modified wood. Holz Als Roh - Und Werkst. 62 (6), 483–485. doi:10.1007/s00107-004-0520-z
Serra-Wittling, C., Barriuso, E., and Houot, S. (1996). Impact of composting type on composts organic matter characteristics. Sci. Compost., 262–273. doi:10.1007/978-94-009-1569-5_26
Smith, B. C. (2011). “Fundamentals of fourier transform infrared spectroscopy,” in Fundamentals of fourier transform infrared spectroscopy. Second Edition (Boca Raton, Florida, United States: CRC Press).
Smith, B. C. (2023). Infrared spectroscopy of polymers, XI: Introduction to organic nitrogen polymers. Spectroscopy, 14–18. doi:10.56530/SPECTROSCOPY.VD3180B5
Sohi, S. P., Krull, E., Bol, R., Lopez-Capel, E., and Bol, R. (2010). A review of biochar and its use and function in soil. Adv. Agron. 105 (10), 47–82. doi:10.1016/S0065-2113(10)05002-9
Spokas, K. A., Cantrell, K. B., Novak, J. M., Archer, D. W., Ippolito, J. A., Collins, H. P., et al. (2012). Biochar: A synthesis of its agronomic impact beyond carbon sequestration. J. Environ. Qual. 41 (4), 973–989. doi:10.2134/JEQ2011.0069
Spokas, K. A. (2010). Review of the stability of biochar in soils: Predictability of O:C molar ratios. Carbon Manag. 1 (2), 289–303. doi:10.4155/cmt.10.32
Statistics, Research and Information Directorate (SRID) (2021).Statistics, research and information directorate (SRID) Ghana, West Africa: Ministry of Food and Agriculture.
Sulemana, N. (2019).Biochar-compost as Phosphorus source and its effects on maize yield and labile carbon in a ferric lixisol MPhil Thesis. Ghana, West Africa: Department of Soil Science, University of Ghana.
Sulemana, N., Nartey, E. K., Abekoe, M. K., Adjadeh, T. A., and Darko, D. A. (2021). Use of biochar-compost for phosphorus availability to maize in a concretionary ferric lixisol in northern Ghana. Agronomy 11 (2), 359. doi:10.3390/agronomy11020359
Tetteh, F. M., Larbi, A., Nketia, K. A., Senayah, J. K., Hoeschle-Zeledon, I., Abdul-Rahman, N., et al. (2016). Suitability of soils for cereal cropping in Northern Ghana. Ibadan, Nigeria: International Institute of Tropical Agriculture, 5. doi:10.13140/RG.2.2.34455.73122
Tomczyk, A., Sokołowska, Z., and Boguta, P. (2020). Biochar physicochemical properties: Pyrolysis temperature and feedstock kind effects. Rev. Environ. Sci. Biotechnol. 19 (1), 191–215. doi:10.1007/s11157-020-09523-3
Tsai, C. C., and Chang, Y. F. (2019). Carbon dynamics and fertility in biochar-amended soils with excessive compost application. Agronomy 9 (9), 511. doi:10.3390/agronomy9090511
Tumuhairwe, J. B., Tenywa, J. S., Otabbong, E., and Ledin, S. (2009). Comparison of four low-technology composting methods for market crop wastes. Waste Manag. 29 (8), 2274–2281. doi:10.1016/J.WASMAN.2009.03.015
UNDP (2018). Northern Ghana human development report 2018. New York, NY, United States: United Nations Development Programme.
Uras, Ü., Carrier, M., Hardie, A. G., and Knoetze, J. H. (2012). Physico-chemical characterization of biochars from vacuum pyrolysis of South African agricultural wastes for application as soil amendments. J. Anal. Appl. Pyrolysis 98, 207–213. doi:10.1016/J.JAAP.2012.08.007
Vandecasteele, B., Sinicco, T., Hose, T. D., Vanden, T., and Mondini, C. (2016). Biochar amendment before or after composting affects compost quality and N losses, but not P plant uptake. J. Environ. Manag. 168, 200–209. doi:10.1016/j.jenvman.2015.11.045
Vergnoux, A., Guiliano, M., Le Dréau, Y., Kister, J., Dupuy, N., Doumenq, P., et al. (2009). Monitoring of the evolution of an industrial compost and prediction of some compost properties by NIR spectroscopy. Sci. Total Environ. 407 (7), 2390–2403. doi:10.1016/J.SCITOTENV.2008.12.033
Warman, P. R. (2013). Evaluation of seed germination and growth tests for assessing compost maturity. Compost Sci. Util. 7, 33–37. doi:10.1080/1065657X.1999.10701972
Watanabe, F. S., and Olsen, S. R. (1965). Test of an ascorbic acid method for determining phosphorus in water and NaHCO3 extracts from soil. Soil Sci. Soc. Am. J. 29 (6), 677–678. doi:10.2136/SSSAJ1965.03615995002900060025X
Xu, G., Zhang, Y., Shao, H., and Sun, J. (2016). Pyrolysis temperature affects phosphorus transformation in biochar: Chemical fractionation and 31P NMR analysis. Sci. Total Environ. 569–570, 65–72. doi:10.1016/j.scitotenv.2016.06.081
Yang, H., Yan, R., Chen, H., Zheng, C., Lee, D. H., and Liang, D. T. (2006). In-depth investigation of biomass pyrolysis based on three major components: Hemicellulose, cellulose and lignin. Energy & Fuels 20 (1), 388–393. doi:10.1021/EF0580117
Zhou, Y., Selvam, A., and Wong, J. W. C. (2014). Evaluation of humic substances during co-composting of food waste, sawdust and Chinese medicinal herbal residues. Bioresour. Technol. 168, 229–234. doi:10.1016/J.BIORTECH.2014.05.070
Keywords: agroecology, farm residues, compost production, phosphorus availability, nutrient mining, carbon sequestration, pyrolysis
Citation: Fianko DA, Nartey EK, Abekoe MK, Adjadeh TA, Lawson IYD, Amoatey CA, Sulemana N, Akumah AM, Baba ME and Asuming-Brempong S (2023) Sustainable P-enriched biochar-compost production: harnessing the prospects of maize stover and groundnut husk in Ghana’s Guinea Savanna. Front. Environ. Sci. 11:1252305. doi: 10.3389/fenvs.2023.1252305
Received: 03 July 2023; Accepted: 11 September 2023;
Published: 25 September 2023.
Edited by:
Zhenming Zhang, Guizhou University, ChinaReviewed by:
Khaled D. Alotaibi, King Saud University, Saudi ArabiaCopyright © 2023 Fianko, Nartey, Abekoe, Adjadeh, Lawson, Amoatey, Sulemana, Akumah, Baba and Asuming-Brempong. This is an open-access article distributed under the terms of the Creative Commons Attribution License (CC BY). The use, distribution or reproduction in other forums is permitted, provided the original author(s) and the copyright owner(s) are credited and that the original publication in this journal is cited, in accordance with accepted academic practice. No use, distribution or reproduction is permitted which does not comply with these terms.
*Correspondence: Eric K. Nartey, ZW5hcnRleUB1Zy5lZHUuZ2g=
Disclaimer: All claims expressed in this article are solely those of the authors and do not necessarily represent those of their affiliated organizations, or those of the publisher, the editors and the reviewers. Any product that may be evaluated in this article or claim that may be made by its manufacturer is not guaranteed or endorsed by the publisher.
Research integrity at Frontiers
Learn more about the work of our research integrity team to safeguard the quality of each article we publish.