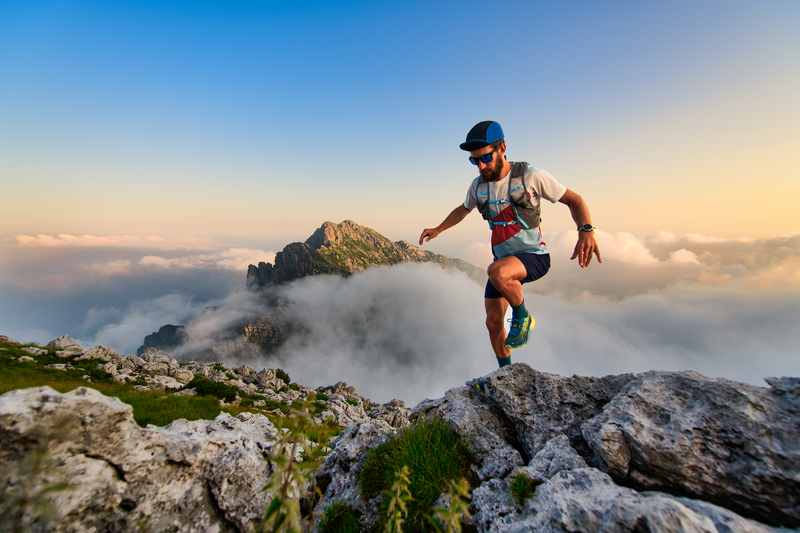
94% of researchers rate our articles as excellent or good
Learn more about the work of our research integrity team to safeguard the quality of each article we publish.
Find out more
ORIGINAL RESEARCH article
Front. Environ. Sci. , 31 July 2023
Sec. Environmental Systems Engineering
Volume 11 - 2023 | https://doi.org/10.3389/fenvs.2023.1241397
This article is part of the Research Topic Environmental Impacts of Materials and their Life Cycle View all 9 articles
The construction industry plays a significant role in contributing to various environmental impacts, including climate change, acidification, and ecotoxicity, among others. This research paper presents a scientific analysis focused on the environmental evaluation of a masonry residential house by life cycle assessment (LCA) methodology. Specifically, it investigates the environmental impacts associated with the production phase of materials using the SimaPro software, which employs the Ecoinvent database. The ReCiPe method is utilized to calculate the comprehensive environmental impacts, with particular emphasis on climate change, terrestrial acidification, ecotoxicity, land use, and water use. To accurately determine the environmental impacts of the house materials, an allocation approach is employed, taking into account the function and location of the materials within individual structures. The calculated share of climate change per house materials amounted to 21.59 kg CO2 eq/m2/year, while the share on water scarcity was estimated at 15.87 m3/m2/year. The research findings reveal that vertical structures exhibit the highest environmental impacts across all impact categories, while horizontal structures demonstrate comparatively lower values.
Construction activity is a crucial driver of national development, as it caters to the growing population’s need for shelter, resulting in an expansion of the built-up area and subsequent environmental impacts (Pamu et al., 2022). The construction sector plays a significant role in energy consumption, accounting for over one-third of final energy consumption and both direct and indirect energy-related emissions. These emissions are quantified in terms of carbon dioxide equivalents (CO2 eq), as highlighted in a report coordinated by the United Nations Environment Program (Chapa, 2019; Globalabc, 2020). In Europe, it is estimated that the construction sector utilizes approximately 50% of all materials and generates more than one-third of total waste (Herczeg, 2014). To mitigate the adverse effects of climate change, the construction industry needs to achieve “carbon neutrality” by 2050, aligning with the International Energy Agency’s recommendations to limit global average temperature rise to 1.5°C and prevent severe climate-related catastrophes.
There is a growing need for standardized tools and databases that facilitate the harmonized application of life cycle assessment (LCA) methodologies in the field of building analysis. These tools and databases are essential for ensuring transparent and consistent monitoring of environmental impacts across the entire life cycle of buildings (Sassanelli et al., 2022; De Wolf et al., 2023). LCA is a well-established technique utilized to evaluate the environmental implications associated with a product throughout its entire life cycle. By employing LCA, it becomes possible to enhance resource utilization efficiency (Curran, 2017; Sassanelli and Terzi, 2022). The primary objective of LCA is to provide a comprehensive assessment of resource consumption and potential environmental impacts across all phases of a product’s life cycle. Rather than focusing solely on specific issues like acidification or climate change, LCA encompasses a broad spectrum of potential environmental impacts (Füchsl et al., 2022). LCA enables the comprehensive assessment and quantification of material and energy flows, encompassing the entire life cycle of a system or product. This assessment aids in the evaluation of the associated environmental impacts (Valencia–Barbara et al., 2023). The construction sector follows the guidelines outlined in ISO 15804, which specifies the life cycle stages considered for analysis. These stages include the production stage (A1-A3), the construction process stage (A4-A5), the use stage (B1-B7), and the end-of-life stage (C1-C4). Additionally, it is possible to incorporate external factors, benefits, and burdens that lie beyond the system boundaries (D) (European Committee for Standardization, 2019).
Historically, LCA studies on buildings have predominantly focused on the environmental impacts associated with the production and construction phases, often neglecting or providing limited detail on other crucial phases such as use, maintenance, repair, replacement, renewal, and end of life (Pomponi and Moncaster, 2018). However, previous research has demonstrated that incorporating environmental parameters into the design phase, alongside conventional selection criteria, can effectively reduce the environmental impact of a building (Dixit et al., 2012; Kylili and Fokaides, 2017; Figueiredo et al., 2021). While there is a growing body of literature on LCA of buildings, the attention is typically focused on major environmental impacts, such as energy consumption and global warming, associated with climate change (Asdrubaki et al., 2013; Rodriguez et al., 2022; Scherz et al., 2023).
This research paper aims to comprehensively identify and assess the climate change and other often overlooked environmental impacts such as land and water use, soil and water acidification, and ecotoxicity, associated with a single-family house. The assessment is conducted using the life cycle assessment approach, allowing for a holistic evaluation of the house’s environmental performance. Environmental impacts are allocated based on the specific functions of materials within the building, considering individual structures as the basis for analysis.
For the purpose of conducting the environmental analysis, a masonry ground-floor house was chosen as the subject of study. This particular house type represents a common example of family-oriented construction prevalent in the Central European region (Figure 1). The characteristics and attributes of the analysed ground-floor family house are presented in Table 1.
The inventory analysis section provides a detailed specification of the building materials utilized in the construction of the examined house.
According to the ISO 14040 standard, the LCA methodology consists of four phases: 1) definition of goal and scope, 2) analysis of life cycle inventory (LCI), 3) life cycle impact assessment (LCIA) and 4) interpretation (International Organization For Standardization, 2006).
The goal and scope of LCA define the purpose, boundaries, and specific objectives of the assessment. These elements provide a clear framework for conducting an LCA study and guide the selection of appropriate methodologies, data collection, and analysis.
The goal of the study was to evaluate the overall environmental impact of a family house in terms of the materials used in its construction. The scope of the life cycle assessment was specifically focused on evaluating the environmental impacts related to global warming potential, terrestrial acidification potential, ecotoxicity potential, agricultural land occupation potential, and water consumption potential. These impact categories were carefully selected to ensure a comprehensive and accurate analysis of the environmental challenges associated with the family house.
The declared unit specified in this study pertains to a single building representing 339,158.5 kg of materials. The projected lifespan of the building was determined considering factors such as the construction type, assemblies, and prevailing climatic conditions, with an estimated duration of 50 years for all long-term elements and 25 years for short-term construction elements (Sartori and Hestnes, 2007).
This research focused on the calculation of environmental impacts specifically related to the production phase (A1-A3, as defined by EN 15804) of building materials.
The SimaPro software (Sustainability, 2020) used for the analysis, has been among the leading LCA software solutions for over 30 years used in more than 80 countries (Xu et al., 2008; Brecheisen and Theis, 2015; Manjunatha et al., 2021; Katebi et al., 2023). The software provides a range of LCIA methods, categorized into European, Global, North American, Others, Single issue, and Water footprint, among others (Feng et al., 2023). These methods differ in the number of impact categories they encompass. For instance, Ecological Scarcity includes 19 impact categories, Traci includes 10, and Cumulative Energy Demand includes 6. Therefore, selecting the most suitable impact assessment method is crucial. However, no specific method is universally recommended, and the decision is often reliant on the subjective judgment of the researcher. In this research, the ReCiPe (Relevance-based Impact Assessment on Characterization Factors) method was selected as the chosen evaluation method. The ReCiPe method is widely recognized and frequently employed in life cycle assessment studies. The method combines inventory data, which quantifies the inputs and outputs of a product or system, with impact assessment factors to calculate the potential environmental impacts. These impact assessment factors are derived through a consensus-building process involving experts from various fields. By employing a consensus-based approach and providing a broad range of impact categories, the ReCiPe method enables a comprehensive and standardized assessment of environmental impacts, allowing for more informed decision-making and sustainable development practices. It was first developed in 2008 and the last version was updated in 2016 (Huijbregts et al., 2016).
The LCI step is a fundamental component of LCA analysis. It involves the compilation and quantification of all inputs and outputs associated with the analysed life cycle stages of a product, process, or system. The purpose of LCI is to develop an inventory database that captures the flow of materials, energy, and emissions throughout the entire life cycle. During the LCI step, data is collected on various inputs and outputs, including raw material extraction, energy consumption, emissions to air, water, and soil, waste generation, transportation, and other relevant factors. This data is typically collected from multiple sources, including industry data, published databases, and direct measurements. This inventory serves as the foundation for subsequent phases of LCA, such as LCIA and interpretation.
In this study, the Life Cycle Inventory (LCI) phase involved summarizing the types and quantities of materials employed in the construction of the family house. The materials were categorized into six structures based on their specific locations within the building: substructure and foundations, vertical structures, horizontal structures, roof structure, surface and finishing materials, and insulation (see Figure 2). The unit data for construction materials were sourced from the Ecoinvent database, taking into account the typical manufacturing processes of the materials, including the associated energy consumption. The electricity consumption was calculated using the EU energetic mix as the basis for assessment.
The main goal of LCIA was to provide a comprehensive and scientifically rigorous assessment of the potential environmental burdens and impacts associated with a product or system. Classification and characterization are the mandatory steps and in frame of those the collected inventory data is translated into a set of impact categories. The impact categories represent specific environmental concerns, such as climate change, human toxicity, ozone depletion, ecosystem quality, etc. Characterization factors, which are derived from scientific models and databases, are used to convert the inventory data into impact scores for each category. LCIA methodologies can vary in terms of the impact categories and impact indicators considered, the characterization factors used, and the specific modeling approaches employed. In LCIA, midpoint and endpoint indicators are two types of metrics used to quantify and evaluate the environmental impacts associated with a product or system’s life cycle. Mid-point indicators represent specific environmental factors or intermediate impacts that occur during the life cycle stages of a product or system. They provide a more detailed and specific assessment of the potential environmental effects. End-point indicators, also known as damage or final impact indicators, are broader and more aggregated metrics that represent the ultimate consequences or end-points of the assessed environmental impacts. They provide a higher-level view of the overall environmental damage or potential effects on human health or ecosystem quality.
In the paper, calculations were performed using 8.3.3. version of the SimaPro software. The chosen method, ReCiPe, provides results at both the end and mid-point indicator levels. In this study’s life cycle impact assessment analysis, mid-point categories were utilized to characterize the environmental impacts (Huijbregts et al., 2016).
The LCI data were compiled to evaluate the environmental impacts associated with selected impact categories, including climate change, terrestrial acidification, ecotoxicity, land use, and water consumption (Table 2).
Interpretation step involves analyzing and communicating the results of the assessment, drawing conclusions, and making informed decisions based on the findings.
The findings of this LCA study are interpreted and discussed in the Results and discussion section of the manuscript. The overall environmental impacts have been normalized per unit of built-up floor area of the house, employing Formula (1) as a reference.
In Eq. 1, the symbol REIi denotes the environmental impact per unit of built-up floor area, specifically for each individual mid-point impact category (i). On the other hand, EIi refers to the environmental impact per unit of built-up floor area calculated by the software. The variable FA represents the floor area of the house measured in square meters, as indicated in Table 1.
Table 3 presents the computed outcomes of the comprehensive environmental impact assessment for the examined residential dwelling across various impact categories expressed by EI and REI values. The EI and REI values have also been calculated on an annual basis, taking into account the 50-year service life of the building (Table 3).
The global warming potential (GWP) of the materials used in the family house indicates the contribution of these materials to climate change, and it was found to be lower (21.59 kg CO2 eq/m2/y) compared to the values reported in Turkey (39 kgCO2eq/m2/y) by Atmaca and Atmaca. (2022) or in Korea (49.4 kg of CO2 eq/m2/y) reported by Roh and Tae. (2017). However, it is comparable to the findings reported by Bastos et al. (2014) or Motuziene et al. (2016) (28.6 kg CO2 eq/m2/y). According to Atmaca and Atmaca. (2022), American and European electricity grids have significantly lower global warming potential (GWP) compared to the Asian grid, largely due to the substantial contribution of renewable and nuclear energy sources. In 2019, the CO2 intensity of electrical energy in European countries was reported to be 2 t CO2eq/toe, whereas Asian countries measured a higher value of 2.7 t CO2eq/toe. Additionally, residential houses in urban areas of Asian countries commonly rely on coal for space heating, resulting in elevated CO2 emissions into the atmosphere. The acidification potential was determined to be 3.75 kg SO2 eq/m2, slightly higher than the range of 0.633–3.25 kg SO2 eq/m2 reported by Wrålsen et al. (2018) for building materials in a Norwegian apartment building.
Comparing the ecotoxicity results for building components can be challenging due to the prevailing influence of technical and economic factors, as emphasized by Kobetičová and Černý (2017). This dominance often leads to an underestimation of the potential impact of materials on human health and the wellbeing of other organisms. Ecotoxicity, which refers to the harmful effects of materials on ecosystems, is typically considered as an additional aspect in LCA, if it is even assessed at all.
The water use per production phase of materials was calculated to 15.86 m3/m2 per year for the analysed house. Rivero-Camacho et al. (2023) notice that large part of water use is carried out indirectly through the production processes of materials and equipment, which is usually called virtual water. Crawford and Pullen (2011) study water in residential building over a period of 50 years and conclude that virtual water in building materials is greater than direct household consumption. Regarding the water embodied in construction, Mannan and Al-Ghamdi. (2020) and Meng et al. (2014) found values between 18.9 and 54.1 m3 of virtual water per m2 of gross floor area.
The allocated referenced environmental impacts (REI) for the individual categories, including foundation structures (FM), vertical structures (VS), horizontal structures (HS), roof structures (RS), surface materials (SM), and insulation material (IM), are documented in Table 4.
TABLE 4. Referenced environmental impacts per 1 m2 of built-up flooring area per individual structures.
The results demonstrate that vertical structures (VS) exhibit the highest environmental impact across all categories, followed by surface materials (SM), foundation materials (FM), roof construction (RC), insulation materials (IM), and horizontal structures (HS), which display the lowest impact (Figure 3).
The primary factor responsible for the highest environmental impact of vertical structures is the utilization of bricks as the primary construction material for walls. Bricks account for over 80% of the overall calculated environmental impacts, with significant contributions across various categories: 90% to global warming (GWP), 88% to acidification (TAP), 89% to ecotoxicity (ETP), 80% to land use (LOP), and 91% to water scarcity (WCP). In a study conducted by Caruso et al. (2018) and Eštoková and Porhinčák (2012), an analysis based on life cycle assessment was performed to compare the environmental impact of different structural materials. The results indicated that concrete structures exhibited higher carbon emissions compared to steel structures. However, Alshamrani (2016) presented contrasting findings, suggesting that concrete buildings actually have a lower environmental impact. In another study by Skullestad et al. (2016), an attributional LCA approach was employed to evaluate the climate change impact of timber structures in comparison to masonry structures. The results showed that timber structures resulted in a climate change impact that was 34%–84% lower than that of masonry structures. Furthermore, Pierobon et al. (2019) discovered that hybrid cross-laminated timber (CLT) buildings achieved an average reduction of 26.5% in global warming potential when compared to masonry buildings.
The same pattern of a dominant material driving the observed environmental impacts was also observed for the foundations and roof structure (Ondova and Estokova, 2016). In both cases, concrete was the primary material utilized. When considering concrete in foundations, it contributed to 93%–99% of the total environmental burden across all environmental categories. As for the roof structure, concrete roofing accounted for a range of 70% (ETP) to 85% (LOP) of the overall environmental impact.
The scenario differed when it came to insulation and surface materials, as multiple materials played a role in the environmental burden. Take, for instance, lime mortars and ceramic tiles, which served as surface materials. They shared a similar responsibility in contributing to global warming, with rates of approximately 38% and 37% respectively. However, in the remaining environmental categories, ceramic tiles took the lead, accounting for contributions ranging from 43% (WCP) to 56% (ETP).
Figures 4–8 depict the percentage distribution of individual building structures contributing to each impact category. The percentage contributions of vertical structures, identified as having the highest impact, varied from 39% to 71% across each impact category. As mentioned above, the significant contribution of vertical structures to overall environmental impacts can be attributed to the predominant use of brick as the primary masonry material for perimeter walls. In contrast, horizontal structures exhibited a minimal contribution to the overall environmental impact of the building, falling within the range of 0%–3%.
When considering materials for the primary structural elements such as vertical and horizontal structures, as well as foundations, it can be observed that these components have the most significant impact on the global warming potential (GWP), accounting for 67% of the total GWP. This finding aligns with the results presented by Paleari et al. (2016), who reported that primary structures’ materials used in the structure contribute to 61% of the total greenhouse gas emissions. Dimoudi and Tompa (2008) also noted that building materials for the primary structure constitute 73%–75% of the total GWP in building construction.
The contribution of surface materials was slightly higher, accounting for 23% of the total GWP, compared to the figures calculated by Paleari et al. (2016) for finishes and plasters, which amounted to 18%. Regarding insulation materials, including mineral wool and polystyrene, the results from the present study and the Italian study Paleari et al. (2016) are comparable, representing a 5% share of the total GWP in this study compared to 3% in the Italian study.
It is worth mentioning that surface materials account for 21% of the total acidification potential (TAP). This finding aligns with the results reported by Paleari et al. (2016) who also observed a similar contribution of surface materials to acidification.
The ecotoxicological research conducted on the manufacturing of building materials has primarily concentrated on cement, concrete, and ceramics, as indicated in the studies (Souza et al., 2015; Souza et al., 2016; Jang et al., 2022). Interestingly, no significant differences were found in the impact categories of human toxicity and ecotoxicity among these materials. During the production of concrete bricks, the ecosystem quality was primarily affected due to the release of aluminum and zinc from drilling waste generated during the construction of crude oil wells, which is necessary for extracting crude oil used in the production process. In comparison, ceramic bricks emerged as the most environmentally friendly option among the materials investigated.
The order of results for the environmental impact categories of ecotoxicity and land use aligns with that of the global warming category. In terms of terrestrial acidification, insulating materials exhibit a slightly higher impact on the environment compared to foundation materials, albeit with a minimal difference. The most notable differences in ranking are observed in the water consumption category. Vertical structures display the highest impact, followed by surface materials, roof construction, foundation materials, horizontal structures, and insulation materials, which exhibit the lowest impact. This observation is logical since the production of bricks and surface materials entails significant water consumption, particularly on construction sites. Conversely, the production of insulating materials or horizontal elements involves considerably less water consumption.
Afterward, an analysis was conducted to examine the interdependent relationship among the calculated values of each impact category (Figure 9).
A linear correlation was observed between the GWP (global warming potential) and several other environmental indicators, with the exception of LOP (land use). This suggests that water use, eutrophication, and total acidification resulting from building materials can be estimated based on GWP values since they tend to increase proportionally with an increase in GWP. Similar correlation for water use/GWP was found in the paper investigating exclusively the building envelope (Estokova et al., 2022). Total ecotoxicity comprises three components: terrestrial, marine, and freshwater ecotoxicity, with freshwater ecotoxicity contributing the most to the overall ecotoxicity. Consequently, it can be concluded that a substantial dependence exists between global warming and freshwater ecotoxicity. Dong et al. (2021) found that the results of acidification during the production stage were correlated with energy depletion. However, no significant correlations were observed between acidification and other impact categories according to their study. In a separate analysis by Lasvaux et al. (2016), it was determined that four to six impact categories would be sufficient to explain at least 90%–95% of the variance for each set of indicators. Notably, the impact categories of climate change, energy depletion, and acidification were identified as belonging to a single cluster with strong correlations.
A different correlation type was detected between the LOP and GWP which indicates that the relationship between the variables is not linear but instead follows a logarithmic pattern. As one variable increases or decreases, the corresponding changes in the other variable become progressively smaller. This implies that the impact of GWP on the LOP diminishes as the values increase or decrease.
The correlation types were examined by calculating and comparing the correlation coefficients (R) for specific dependencies. While several pairs of variables, in addition to LOP/GWP, could display both linear and logarithmic patterns, e.g., ETP/GWP and ETP/TAP, the linear correlation was selected as the primary one due to its higher R value. The correlation coefficients between the parameters, determining the strength and direction of the correlations, are displayed in the correlation matrix (Table 5).
Generally, the values in the correlation matrix range from −1 to 1, where 1 represents a strong positive correlation, −1 represents a strong negative correlation, and 0 represents no correlation. Examining the correlation matrix reveals robust positive correlations among all parameters, suggesting a high level of association. The most noteworthy correlation (R = 0.991) was observed between terrestrial acidification and water consumption. Conversely, the lowest correlation coefficient (R = 0.819) was found in the case of the land use/terrestrial acidification dependence. The correlation between the GWP results and the other impact categories is notably strong, indicating the potential to estimate environmental burdens of masonry houses in those categories based on the GWP calculations of the materials. However, it is important to recognize the limitations associated with the system boundaries under evaluation. The estimation of the impact of building materials would only be applicable within the context of a product phase assessment of materials.
This contribution pertains to the LCA analysis conducted on a typical masonry family house. The LCA analysis employed the SimaPro software to identify building elements with the most notable environmental impact. The analysis revealed that house materials contribute by 21.59 kg CO2 eq/m2/year to climate change, 35.9 kg 1,4-DCB/m2/year to ecotoxicity, 0.07 kg SO2 eq/m2/year to terrestrial acidification, and 0.24 m2a crop eq/m2/year to land use. Additionally, the impact on water scarcity is estimated to be 172.74 m3/m2/year. Based on the findings presented, the following conclusions can be drawn:
• The analysis indicates that vertical structures (VS) have the highest environmental impact across all categories, followed by surface materials (SM), foundation materials (FM), roof construction (RC), insulation materials (IM), and horizontal structures (HS), which have the lowest impact. This highlights the significance of vertical structures, primarily due to the use of bricks for walls, which accounts for a substantial portion of the overall environmental impacts.
• The percentage contributions of vertical structures to overall environmental impacts vary from 39% to 71% across each impact category. Horizontal structures exhibit a minimal contribution, falling within the range of 0%–3%. This confirms the significant role of vertical structures in determining the overall environmental impact of the building.
• Bricks, concrete in foundations, and concrete roofing are identified as the primary materials driving the observed environmental impacts. Bricks contribute significantly to global warming, acidification, ecotoxicity, land use, and water scarcity. Concrete in foundations and roof structures also have a major role in overall environmental burden across various categories.
• In contrast to the dominant material-driven impacts, insulation and surface materials involve multiple materials sharing the responsibility for the environmental burden. Lime mortars and ceramic tiles, as surface materials, contribute significantly to global warming. Ceramic tiles have a higher contribution across other environmental categories.
• A linear correlation is observed between GWP and several other environmental indicators, suggesting that water scarcity, eutrophication, and total acidification can be estimated based on GWP values. A logarithmic pattern is observed between land use and GWP, indicating a diminishing impact of GWP on land use as values increase or decrease.
• The correlation matrix reveals robust positive correlations among all parameters, indicating a high level of association. The strongest positive correlation is found between terrestrial acidification and water consumption, while the lowest correlation coefficient is observed in the case of land use and terrestrial acidification dependence.
• The strong correlation between GWP results and other impact categories suggests the potential to estimate environmental burdens of masonry houses based on GWP calculations.
However, it is important to consider the limitations of the research outcomes including scope and generalizability, system boundaries and data availability and quality. The findings are specific to the examined residential dwelling and may not be directly applicable to other types of buildings or regions with different construction practices and material availability. Future research should aim to expand the scope by including a wider range of building types and locations to enhance the generalizability of the findings. In addition, the findings are limited to the product phase assessment of building materials. To obtain a comprehensive understanding of the environmental impacts throughout the entire life cycle of a building, including the construction, operation, and end-of-life phases, a more holistic life cycle assessment approach should be considered in future studies. The findings consider the environmental impacts over the 50-year service life of the building. However, it would be valuable to investigate the long-term performance of different materials and their adaptability to changing environmental conditions, such as climate change and resource availability.
The study primarily addresses the environmental impacts, and other important factors such as social aspects, economic considerations, and occupant behaviour are not fully taken into account. Future research should aim to integrate social and economic dimensions to provide a more comprehensive assessment of sustainable building practices.
The research findings have several managerial and policy implications that can guide decision-making and promote sustainable practices in the construction industry. The findings emphasize the importance of considering the environmental implications of different building structures. Managers and policymakers can encourage design practices that minimize the use of high-impact elements, such as vertical structures, and promote alternative construction techniques that reduce the reliance on resource-intensive materials. Policymakers can introduce stricter environmental requirements for construction projects, promoting the use of low-impact materials, energy-efficient designs, and sustainable construction practices. This can drive industry-wide adoption of sustainable building practices and contribute to achieving broader sustainability goals.
The research highlights the importance of considering the entire life cycle of a building. Managers and policymakers can encourage the adoption of LCA methodologies in decision-making processes. By considering not only the environmental impacts during construction but also the operation and end-of-life phases, a more comprehensive and sustainable approach to building design and construction can be achieved.
The raw data supporting the conclusion of this article will be made available by the authors, without undue reservation.
MF: Substantial contributions to design of the work and analysis, drafting the work
AE: Contributions to the conception of the work, interpretation of data for the work, revising of content. All authors contributed to the article and approved the submitted version.
This study was conducted within the framework of the project solution and received support from the Scientific Grant Agency of the Ministry of Education, Science, Research, and Sport of the Slovak Republic, and the Slovak Academy of Sciences, under project VEGA Grant Nos. 1/0230/21 and APVV-20-0140.
The authors declare that the research was conducted in the absence of any commercial or financial relationships that could be construed as a potential conflict of interest.
All claims expressed in this article are solely those of the authors and do not necessarily represent those of their affiliated organizations, or those of the publisher, the editors and the reviewers. Any product that may be evaluated in this article, or claim that may be made by its manufacturer, is not guaranteed or endorsed by the publisher.
Alshamrani, O. S. (2016). Life cycle assessment of low-rise office building with different structure–envelope configurations. Can. J. Civ. Eng. 43 (3), 193–200. doi:10.1139/cjce-2015-0431
Asdrubaki, F., Baldassarri, C., and Fthenakis, V. (2013). Life cycle analysis in the construction sector: Guiding the optimization of conventional Italian buildings. Energy Build. 64, 73–89. doi:10.1016/j.enbuild.2013.04.018
Atmaca, A., and Atmaca, N. (2022). Carbon footprint assessment of residential buildings, a review and a case study in Turkey. J. Clean. Prod. 340, 130691. doi:10.1016/j.jclepro.2022.130691
Bastos, J., Batterman, S. A., and Freire, F. (2014). Life-cycle energy and greenhouse gas analysis of three building types in a residential area in Lisbon. Energy Build. 69, 344–353. doi:10.1016/j.enbuild.2013.11.010
Brecheisen, T., and Theis, T. (2015). “Life cycle assessment as a comparative analysis tool for sustainable brownfield redevelopment projects: Cumulative energy demand and greenhouse gas emissions,” in Assessing and measuring environmental impact and sustainability (Butterworth-Heinemann), 323–365.
Caruso, M. C., Menna, C., Asprone, D., and Prota, A. (2018). LCA-based comparison of the environmental impact of different structural systems. IOP Conf. Ser. Mater Sci. Eng. 442 (1), 012010. doi:10.1088/1757-899x/442/1/012010
Chapa, J. (2019). “Bringing embodied carbon upfront,” in Built environment economist: Australia and New Zealand, 38–41.
Crawford, R. H., and Pullen, S. (2011). Life cycle water analysis of a residential building and its occupants. Build. Res. Inf. 39 (6), 589–602. doi:10.1080/09613218.2011.584212
Curran, M. A. (2017). “Overview of goal and scope definition in life cycle assessment,” in Goal and scope definition in life cycle assessment. LCA compendium – the complete world of life cycle assessment. Editor M. A. CURRAN (Netherlands, Dordrecht: Springer), 1–62. 978-94-024-0855-3.
De Wolf, C., Cordella, M., Dodd, N., Byers, B., and Donatello, S. (2023). Whole life cycle environmental impact assessment of buildings: Developing software tool and database support for the EU framework Level (s). Resour. Conservation Recycl. 188, 106642. doi:10.1016/j.resconrec.2022.106642
Dimoudi, A., and Tompa, C. (2008). Energy and environmental indicators related to construction of office buildings. Resour. Conservation Recycl. 53 (1-2), 86–95. doi:10.1016/j.resconrec.2008.09.008
Dixit, M. K., Fernández-Solís, J. L., Lavy, S., and Culp, C. H. (2012). Need for an embodied energy measurement protocol for buildings: A review paper. Renew. Sustain. energy Rev. 16 (6), 3730–3743. doi:10.1016/j.rser.2012.03.021
Dong, Y., Ng, S. T., and Liu, P. (2021). A comprehensive analysis towards benchmarking of life cycle assessment of buildings based on systematic review. Build. Environ. 204, 108162. doi:10.1016/j.buildenv.2021.108162
European Committee for Standardization (2019). 2012 + A2: 2019, sustainability of construction works—environmental product declarations—core rules for the product category of construction products. Brussels, Belgium: European Committee for Standardization.
Eštoková, A., and Porhinčák, M. (2012). Reduction of primary energy and CO2 emissions through selection and environmental evaluation of building materials. Theor. Found. Chem. Eng. 46 (6), 704–712. doi:10.1134/s0040579512060085
Eštoková, A., Wolfová Fabianová, M., and Ondová, M. (2022). Concrete structures and their impacts on climate change and water and raw material resource depletion. Int. J. Civ. Eng. 20, 735–747. doi:10.1007/s40999-022-00701-8
Feng, H., Zhao, J., Hollberg, A., and Habert, G. (2023). Where to focus? Developing a LCA impact category selection tool for manufacturers of building materials. J. Clean. Prod. 405, 136936. doi:10.1016/j.jclepro.2023.136936
Figueiredo, K., Pierott, R., Hammad, A. W., and Haddad, A. (2021). Sustainable material choice for construction projects: A life cycle sustainability assessment framework based on bim and fuzzy-AHP. Build. Environ. 196, 107805. doi:10.1016/j.buildenv.2021.107805
Füchsl, S., Röder, H., Rheude, F., and Röder, H. (2022). Life cycle assessment (LCA) of thermal insulation materials: A critical review. Clean. Mater. 5, 100119. doi:10.1016/j.clema.2022.100119
Globalabc, I. E. A. U. N. E. P. (2020). GlobalABC roadmap for buildings and constructio n 2020-2050, towards a zero-emission, efficient and resilient buildings and construction sector. Paris, France: International Energy Agency.
Herczeg, M. (2014). Resource efficiency in the building sector: Final report to DG environment. Rotterdam: European Commission.
Huijbregts, M. A., Steinmann, Z. J., and Elshout, P. (2016). ReCiPe 2016: A harmonized life cycle impact assessment method at midpoint and endpoint level report I: Characterization.
International Organization For Standardization (2006). Environmental management: Life cycle assessment; requirements and guidelines, 14044. Geneva, Switzerland: ISO.
Jang, H. J., Ahn, Y. H., and Tae, S. H. (2022). Proposal of major environmental impact categories of construction materials based on life cycle impact assessments. Materials 15 (14), 5047. doi:10.3390/ma15145047
Katebi, A., Tushmanlo, H. S., and Asadollahfardi, G. (2023). Environmental life cycle assessment and economic comparison of different roof systems. J. Build. Eng. 76, 107316. doi:10.1016/j.jobe.2023.107316
Kobetičová, K., and Černý, R. (2017). Ecotoxicology of building materials: A critical review of recent studies. J. Clean. Prod. 165, 500–508. doi:10.1016/j.jclepro.2017.07.161
Kylili, A., and Fokaides, P. A. (2017). Policy trends for the sustainability assessment of construction materials: A review. Sustain. Cities Soc. 35, 280–288. doi:10.1016/j.scs.2017.08.013
Lasvaux, S., Achim, F., Garat, P., Peuportier, B., Chevalier, J., and Habert, G. (2016). Correlations in Life Cycle Impact Assessment methods (LCIA) and indicators for construction materials: What matters? Ecol. Indic. 67, 174–182. doi:10.1016/j.ecolind.2016.01.056
Manjunatha, M., Preethi, S., Mounika, H. G., Niveditha, K. N., and Ravi, (2021). Life cycle assessment (LCA) of concrete prepared with sustainable cement-based materials. Mater. Today Proc. 47, 3637–3644. doi:10.1016/j.matpr.2021.01.248
Mannan, M., and Al-Ghamdi, S. G. (2020). Environmental impact of water-use in buildings: Latest developments from a life-cycle assessment perspective. J. Environ. Manag. 261, 110198. doi:10.1016/j.jenvman.2020.110198
Meng, J., Chen, G. Q., Shao, L., Li, J. S., Tang, H. S., Hayat, T., et al. (2014). Virtual water accounting for building: Case study for E-town, beijing. J. Clean. Prod. 68, 7–15. doi:10.1016/j.jclepro.2013.12.045
Motuzienė, V., Rogoža, A., Lapinskienė, V., and Vilutienė, T. (2016). Construction solutions for energy efficient single-family house based on its life cycle multi-criteria analysis: A case study. J. Clean. Prod. 112, 532–541. doi:10.1016/j.jclepro.2015.08.103
Ondova, M., and Estokova, A. (2016). Environmental impact assessment of building foundation in masonry family houses related to the total used building materials. Environ. Prog. Sustain. Energy 35 (4), 1113–1120. doi:10.1002/ep.12307
Paleari, M., Lavagna, M., and Campioli, A. (2016). The assessment of the relevance of building components and life phases for the environmental profile of nearly zero-energy buildings: Life cycle assessment of a multifamily building in Italy. Int. J. Life Cycle Assess. 21, 1667–1690. doi:10.1007/s11367-016-1133-6
Pamu, Y., Kumar, V., Shakir, M. A., and Ubbana, H. (2022). Life cycle assessment of a building using open-LCA software. Mater. Today Proc. 52 (3), 1968–1978. doi:10.1016/j.matpr.2021.11.621
Pierobon, F., Huang, M., Simonen, K., and Ganguly, I. (2019). Environmental benefits of using hybrid CLT structure in midrise non-residential construction: An LCA based comparative case study in the US Pacific Northwest. J. Build. Eng. 26, 100862. doi:10.1016/j.jobe.2019.100862
Pomponi, F., and Moncaster, A. (2018). Scrutinising embodied carbon in buildings: The next performance gap made manifest. Renew. Sustain. Energy Rev. 81, 2431–2442. doi:10.1016/j.rser.2017.06.049
Pré Sustainability B.V. (2023). SimaPro database manual methods library. Pré Sustainability B.V. web. Available at: https://simapro.com/wp-content/uploads/2021/07/DatabaseManualMethods920.pdf (Accessed July 10, 2023).
Rivero-Camacho, C., Martín-Del-Río, J. J., and Marrero-Meléndez, M. (2023). Evolution of the life cycle of residential buildings in Andalusia: Economic and environmental evaluation of their direct and indirect impacts. Sustain. Cities Soc. 93, 104507. doi:10.1016/j.scs.2023.104507
Rodríguez, L., Martínez, L., Panameño, R., París, O., Muros, A., Rodríguez, R., et al. (2022). LCA of the NZEB El Salvador building, a model to estimate the carbon footprint in a tropical country. J. Clean. Prod. 408, 137137. doi:10.1016/j.jclepro.2023.137137
Roh, S., and Tae, S. (2017). An integrated assessment system for managing life cycle CO2 emissions of a building. Renew. Sustain. Energy Rev. 73, 265–275. doi:10.1016/j.rser.2017.01.139
Sartori, I., and Hestnes, A. G. (2007). Energy use in the life cycle of conventional and low-energy buildings: A review article. Energy Build. 39 (3), 249–257. doi:10.1016/j.enbuild.2006.07.001
Sassanelli, C., Arriga, T., Zanin, S., D’Adamo, I., and Terzi, S. (2022). Industry 4.0 driven result-oriented PSS: An assessment in the energy management. Int. J. Energy Econ. Policy 12 (4), 186–203. doi:10.32479/ijeep.13313
Sassanelli, C., and Terzi, S. (2022). The D-BEST Based digital innovation hub customer journey analysis method: Configuring DIHs unique value proposition. Int. J. Eng. Bus. Manag. 14, 184797902211246. doi:10.1177/18479790221124634
Scherz, M., Kreiner, H., and Passer, A. (2023). Sustainable procurement for carbon neutrality of buildings: A life cycle assessment (LCA)-based bonus/malus system to consider external cost in the bid price. Dev. Built Environ. 14, 100161. doi:10.1016/j.dibe.2023.100161
Skullestad, J. L., Bohne, R. A., and Lohne, J. (2016). High-rise timber buildings as a climate change mitigation measure–A comparative LCA of structural system alternatives. Energy Procedia 96, 112–123. doi:10.1016/j.egypro.2016.09.112
Souza, D. M. de, Lafontaine, M., Charron-Doucet, F., Bengoa, X., Chappert, B., Duarte, F., et al. (2015). Comparative Life Cycle Assessment of ceramic versus concrete roof tiles in the Brazilian context. J. Clean. Prod. 89, 165–173. doi:10.1016/j.jclepro.2014.11.029
Souza, D. M. de, Lafontaine, M., Charron-Doucet, F., Chappert, B., Kicak, K., Duarte, F., et al. (2016). Comparative life cycle assessment of ceramic brick, concrete brick and cast-in-place reinforced concrete exterior walls. J. Clean. Prod. 137, 70–82. doi:10.1016/j.jclepro.2016.07.069
Sustainability, P. (2020). SimaPro database manual Methods library. The Netherlands: Pré Sustainability: Amersfoort.
Valencia-Barba, Y. E., Gómez-Soberón, J. M., and Gómez-Soberón, M. C. (2023). Dynamic life cycle assessment of the recurring embodied emissions from interior walls: Cradle to grave assessment. J. Build. Eng. 65, 105794. doi:10.1016/j.jobe.2022.105794
Wrålsen, B., O'Bornr, , and Skaar, C. (2018). Life cycle assessment of an ambitious renovation of a Norwegian apartment building to nZEB standard. Energy Build. 177, 197–206. doi:10.1016/j.enbuild.2018.07.036
Keywords: life cycle assessment (LCA), environmental impact, ecosystems, building structure, climate change
Citation: Fabianova M and Estokova A (2023) Environmental evaluation of family house materials impacts on climate change, land and water use, acidification and ecotoxicity. Front. Environ. Sci. 11:1241397. doi: 10.3389/fenvs.2023.1241397
Received: 16 June 2023; Accepted: 20 July 2023;
Published: 31 July 2023.
Edited by:
Jose Navarro Pedreño, Miguel Hernández University of Elche, SpainReviewed by:
Gregorio Garcia, Polytechnic University of Cartagena, SpainCopyright © 2023 Fabianova and Estokova. This is an open-access article distributed under the terms of the Creative Commons Attribution License (CC BY). The use, distribution or reproduction in other forums is permitted, provided the original author(s) and the copyright owner(s) are credited and that the original publication in this journal is cited, in accordance with accepted academic practice. No use, distribution or reproduction is permitted which does not comply with these terms.
*Correspondence: Adriana Estokova, YWRyaWFuYS5lc3Rva292YUB0dWtlLnNr
Disclaimer: All claims expressed in this article are solely those of the authors and do not necessarily represent those of their affiliated organizations, or those of the publisher, the editors and the reviewers. Any product that may be evaluated in this article or claim that may be made by its manufacturer is not guaranteed or endorsed by the publisher.
Research integrity at Frontiers
Learn more about the work of our research integrity team to safeguard the quality of each article we publish.