- 1Institute of Fruit Tree Research, Guangdong Academy of Agricultural Sciences, Key Laboratory of South Subtropical Fruit Biology and Genetic Resource Utilization (MOA), Guangdong Province Key Laboratory of Tropical and Subtropical Fruit Tree Research, Guangzhou, China
- 2Wuhan Zhihuiyuan Environmental Protection Technology Co., Ltd., Wuhan, China
- 3Zhanjiang Experimental Station of Chinese Academy of Tropical Agricultural Sciences, Zhanjiang, China
- 4College of Biology and Agricultural Engineering, Jilin University, Jilin, China
- 5Wuhan Ecological Environmental Technology Center, Wuhan, China
Introduction: The application of Fe- (BC-Fe) and Mg-modified (BC-Mg) biochars for the remediation of heavy-metal-contaminated soil has become a research hotspot in recent years. It is critical to select suitable biochar for soil rejuvenation under the same pollution condition.
Methods: In this study, the biochars were characterized by Fourier transform infrared (FTIR) spectroscopy, X-ray diffraction (XRD), and scanning electron microscopy (SEM). In addition, the adsorption and passivation effects of BC-Fe and BC-Mg were comprehensively evaluated through batch adsorption experiments and soil incubation experiments, and the effects of Cd-contaminated soil on plant growth were also demonstrated by pot experiments.
Results: SEM, FTIR, and XRD showed that MgO and Fe3O4 nanoparticles were successfully loaded on the biochar surface. The maximum adsorption efficiencies of BC-Fe and BC-Mg for Cd were 52.63 and 66.23 mg g−1, which was 9.05 and 7.19 times higher than that of the original biochar (7.32 mg g−1), respectively. Soil culture experiment showed that 5% BC-Fe and BC-Mg significantly reduced soil DTPA-Cd content by 38.86% and 50.85% at 120 days, respectively. In addition, BC-Fe and BC-Mg promoted the conversion of acid-soluble Cd to the oxidizable and residual states. Pot experiments revealed that BC-Fe and BC-Mg reduced the Cd content in shoots of the maize by 3.97 and 6.09 times, respectively, and both significantly increased the dry and fresh weights of the shoots and roots of the maize plants. Moreover, BC, BC-Fe, and BC-Mg provided nutrients required for plant growth to the soil and increased the activities of soil dehydrogenase, urease, and peroxidase. The adsorption–passivation mechanism of BC-Fe on Cd mainly included electrostatic attraction, while the adsorption–passivation mechanism of BC-Mg on Cd included precipitation and ion exchange.
Discussion: Overall, the results showed that BC-Mg can be used as an efficient functional material for heavy-metal pollution remediation, and this study provided guidance on the selection of passivator materials for heavy-metal-contaminated soil remediation.
1 Introduction
Soil pollution by heavy metals is currently a prevalent environmental issue in China. According to the 2014 National Soil Pollution Status Survey data, approximately 16.1% of soil-contaminated sites, encompassing both agricultural and industrial areas, exceeded the permissible limit of the secondary standard for soil environmental quality. Among these contaminated sites, heavy metals accounted for 82.8% of the pollution. Specifically, the levels of Cd, Hg, As, Cu, Pb, Cr, Zn, and Ni exceeded the standard by 7.0%, 1.6%, 2.7%, 2.1%, 1.5%, 1.1%, 0.9%, and 4.8%, respectively. The impact of heavy metals on soil fertility, microbial activity, crop yield, and their potential accumulation in vegetables, thereby posing a risk to human health, has been extensively studied (Bolan et al., 2014; Ma et al., 2015). In particular, significant research efforts have been dedicated to investigating the contamination of soils by Cd in recent decades (Culbard et al., 1988; Chlopecka, 1996). It has been observed that Cd exhibits a higher bioavailability in soil than other metal contaminants, such as Cu, Mg, Ni, Pb, and Zn (Prokop et al., 2003). Despite the presence of low concentrations of Cd, plants can still exhibit toxicity toward Cd. Furthermore, there exists a possibility that plants may have the ability to take higher amount of Cd from soils with low Cd concentrations (Li et al., 2006). Furthermore, it is worth noting that the presence of Cd in soil exhibits a persistent nature. Scholars have made significant advancements in devising various technological strategies to address the issue of Cd-contaminated soil, including thermal treatment, soil drenching, and phytoremediation (Ghosh, 2005; Chou et al., 2010; Zhang et al., 2010). Nevertheless, the considerable expenses associated with equipment commissioning and maintenance in industrial Cd-contaminated soil treatment, coupled with the propensity to induce secondary contamination, render it unsuitable for practical remediation of wastewater or soil (Chen et al., 2015; Natarajan et al., 2018).
Biochar (BC), a solid carbon material derived from the pyrolysis of animal or plant residual waste under oxygen-limited conditions at temperatures ranging from 200°C to 700°C, exhibits promising potential as an environmentally friendly and cost-effective remediation material (Qiu et al., 2021). In addition to its ability to efficiently remove Cd from wastewater, biochar also demonstrates a favorable in situ passivation effect on Cd-contaminated soil (Xu et al., 2013). Notably, the utilization of biochar derived from Vetiveria zizanioides as feedstock resulted in a remarkable adsorption efficiency of 69.92% for Cd within the 90-min timeframe (Tyagi, 2022). The utilization of biochar derived from rice husks has been found to effectively decrease the bioavailability of heavy metals in soil, as well as reduce the uptake of heavy metals by lettuce (Kim et al., 2015). However, it should be noted that original biochar has certain limitations in its ability to adsorb Cd from both Cd-contaminated wastewater and soil environments. Consequently, it fails to meet the demands of progressively stricter environment regulations. Consequently, scholars have endeavored to enhance and incorporate biochar media by means of modifications aimed at improving its efficacy in removing heavy metals. These modifications include acid–base treatment to augment heavy-metal adsorption by enhancing the surface area and increasing the presence of oxygen-containing functional groups in biochar, as well as the loading of metal oxides to enhance the specific adsorption capacity of heavy metals onto biochar (Hemavathy et al., 2020; Lonappan et al., 2020; Amusat et al., 2021; Ma et al., 2021). In recent years, research on Mg-based and Fe-based biochars has attracted considerable attention, particularly in their composite materials with MgO and Fe3O4 nanoparticles. This increased attention can be attributed to their effective adsorption and passivation capabilities in Cd-contaminated soil. The pyrolysis of MgO-BC at 500°C resulted in an adsorption capacity of 104.68, 173.22, 104.38, and 47.02 mg g−1 for Cd(II), Cu(II), Zn(II), and Cr(VI), respectively. Additionally, it reduced CaCl2-Cd by 70.0% and facilitated the conversion of Cd to a stable fraction (Li et al., 2022a). Zahedifar et al. (2021) revealed that the magnetic biochar synthesized from date leaves exhibited a notable maximum adsorption capacity of 53.75 mg g−1 for Cd in wastewater, surpassing the adsorption capacity of pristine biochar. Furthermore, Fu et al. (2021) demonstrated that magnetic biochar derived from wheat straw effectively mitigated the presence of Cd in soil, resulting in a reduction of available soil Cd by 61.38%.
As described previously, different biomass raw materials, different biochar preparation conditions, and different physical and chemical properties of the soil can significantly affect the adsorption and passivation effects of the biochar, which seriously hinders the selection of biochar modification methods and the practical applications. Certain biochar derived from the pyrolysis of raw biomass demonstrated notable adsorption capacity for Cd-contaminated soil. Notably, the biochar derived from pine cones exhibited a maximum adsorption capacity of 92.7 mg·g−1 of Cd, surpassing the adsorption capacities of most of the majority of modified biochars designed for heavy-metal removal (Teng et al., 2020). There are few studies on the comprehensive comparison and evaluation of the adsorption and passivation capacities of Fe-based and Mg-based biochars for Cd. This study aimed to assess the adsorption and passivation effects of Fe-based and Mg-based biochars on Cd and to determine the most suitable modified biochar for practical applications. To achieve this, Fe3O4 and MgO nanoparticle BC composites were prepared using wheat straw. The adsorption kinetics and isotherm realization were employed to evaluate the maximum amount and adsorption characteristics of Cd adsorbed by BC-Fe and BC-Mg. Subsequently, soil culture experiments were conducted for 120 days to assess the passivation characteristics of soil Cd by BC-Fe and BC-Mg. To further evaluate the effects of BC-Fe and BC-Mg on the growth and development of maize plants and their physiological mechanisms in alleviating Cd stress, pot experiments were conducted using this soil for a period of 100 days. The study comprehensively evaluated the following: 1) the adsorption and passivation characteristics and mechanisms of BC-Mg and BC-Fe; 2) the impact of BC-Mg and BC-Fe on soil physicochemical properties; and 3) the effects and mechanisms of BC-Mg and BC-Fe in mitigating plant Cd stress. Hence, the importance of this study resides in offering guidance on the selection of passivator materials for the purpose of remediating heavy metals in soil.
2 Materials and methods
All solutions used in this study were prepared with ultrapure water (18.25 Ω). All reagents used were of analytical grade and were used without purification.
2.1 Preparation and characterization of MgO- and Fe3O4-loaded biochar nanocomposites
2.1.1 Preparation of MgO- and Fe3O4-loaded biochar nanocomposites
To prepare the original biochar using wheat straw as the raw biomass material, the wheat straw was initially cut into 1–2-cm fragments. Subsequently, it was washed three times, first with tap water and then with deionized water. The washed fragments were then dried in an oven at a temperature of 60°C and then ground. The resulting wheat straw fragments were placed inside a crucible, which was then wrapped and sealed with tinfoil. The samples were subjected to a temperature of 500°C in a muffle furnace for 2 h to induce pyrolysis. Following the pyrolysis process, biochar was carefully removed from the furnace and allowed to cool down to room temperature. The resulting product was identified as the original biochar, referred to as BC. The synthesis of BC-MgO nanocomposites involved several steps. Initially, 50 g of wheat straw powder was added to 1 L solution of 1.5 M KOH. The resulting mixture was subjected to magnetic stirring for 5 h, yielding the KOH-biomass precursor. Subsequently, 20 g of the precursor biomass was combined with 500 mL solution containing 0.04 M magnesium acetate. The resulting mixture was then subjected to magnetic stirring at a temperature of 60°C for 24 h. The pyrolysis conditions utilized were consistent with those previously described for the original BC. The resulting sample was designated as BC-Mg. The BC-Fe3O4 nanocomposites were prepared by dissolving 10 g FeSO4·7H2O and 10 g Fe2(SO4)3 in 200 mL of deionized water, followed by the addition of 10 g BC to Fe3+/Fe2+ mixed solution. The mixture was then subjected to magnetic stirring for 30 min, and the pH was adjusted to 10 using 0.1 M KOH. The stirring was continued for an additional 30 min until the mixed solution reached a stable state, resulting in the formation of the Fe-biomass precursor. Subsequently, the precursor samples were heated in a muffle furnace at 500°C for 2 h. The resulting sample was designated as BC-Fe.
2.1.2 Characterization of original and modified biochars
To analyze the crystallographic structure of both original and modified BCs, a Bruker D8 X-ray diffractometer (Cu Kα, 40/thinsp; kV, 20/thinsp; mA, λ = 1.54056”˚) was used. The morphology of powdered BC was examined using a scanning electron microscope (JEOL JSM-6510). Furthermore, the presence of functional groups in both the original and modified BCs was determined using a Fourier transform infrared (FTIR) analyzer (Bruker Tensor 27, Bruker AXS GmbH, Karlsruhe, Germany). BC samples were analyzed for multipoint BET surface area using nitrogen as the adsorbing gas. The surface areas of BC, BC-Mg, and BC-Fe were 90.33, 62.45, and 53.59 m2·g−1, respectively.
2.2 Batch adsorption experiments
2.2.1 Adsorption kinetics experiments
A measure of 20 mg of BC, BC-Fe, and BC-Mg was collected in a 50-mL centrifuge tube, and 20 mL of 200 mg·L−1 Cd(NO3)2 solution was mixed with 20 mg BC, and then the centrifuge tube was shaken. The pH of the mixed solution was adjusted to 5 ± 0.1. Subsequently, the corresponding centrifuge tube was taken out at different times: 0, 15, 30, 60, 150, 300, and 600 min. After collection and filtering using a 0.45-μm filter membrane, the supernatant was collected, and the Cd concentration of the supernatant was determined by AAS (Huang et al., 2022).
In order to investigate potential adsorption behavior, quasi-first-order and quasi-second-order kinetic models were fitted for obtaining the relationship between reaction time and sample adsorption capability. The quasi-first-order equation is expressed as follows:
The quasi-second-order equation is expressed as follows:
where qe (mg·g−1) represents the adsorption amount at adsorption equilibrium; qt (mg·g−1) represents the adsorption amount at time t; K1 (1·min−1) is the quasi-first rate constant; and K2 (g/mg/min) is the quasi-second rate number.
2.2.2 Isothermal adsorption experiment
A measure of 20 mg original and modified BCs was collected in a 50-mL centrifuge tube, and different concentrations of Cd(NO3)2 solution (10, 20, 50, 100, 200, and 300 mg·L−1) were mixed with 20 mg BC. The pH of the mixed solution was adjusted to 5 ± 0.1. The tube was shaken (280 rpm) at room temperature in a thermostatic oscillator. After 24 h, the mixture samples were filtered through 0.45-μm filter membranes, and the Cd concentration of the supernatant was determined by AAS.
Sorption isotherms were modeled using Langmuir and Freundlich equations (Hu et al., 2011; Kołodyńska et al., 2012). The equation of the Langmuir model is expressed as follows:
The equation of the Freundlich model is expressed as follows:
where Ce (mg·L−1) represents the concentration of the adsorbate in the solution at adsorption equilibrium; Qe (mg·g−1) represents the adsorption capacity of the adsorbent at adsorption equilibrium; Qm (mg·g−1) represents the maximum saturated adsorption capacity of the adsorbent; KL is the Langmuir constant or equilibrium constant, which is a parameter characterizing the affinity between the adsorbent and adsorbate; KF represents the Freundlich constant; and N refers to the adsorption intensity constant and the intensity of the adsorption amount increasing with the concentration.
2.3 Soil experiment
The tested soil was collected from the surface tillage layer (about 20 cm deep) from the test field in Huazhong Agricultural University, Wuhan, China. The total Cd content of the soil was supplemented to 12 mg·kg−1 with CdCl2 solution, aged for 45 days. After completion of the aging process, physicochemical properties of the aged soil were as follows: the pH was 7.4; the content of DTPA-Cd was 5.63 mg·kg−1; the content of available phosphorus was 28.33 g·kg−1; the total Cd content was 11.28 mg·kg−1; and the content of organic matter was 24.3 g·kg−1. Soil organic matter encompasses all carbon-based organic substances present in soil, manifesting in diverse forms such as animal and plant remnants, microorganisms, and various decomposed and synthesized organic materials within the soil.
Soil incubation and pot experiment: A measure of 3 kg Cd-contaminated soil was collected in a plastic basin, which was homogeneously mixed with 5% BC, BC-Fe, and BC-Mg. Therefore, treatments applied in this experiment are as follows: CK (no original and modified BCs), BC, BC-Fe, and BC-Mg, and each treatment was processed in three replicates. The soil moisture content was kept at 60% during incubation using the weighing method. A measure of 20 g of soil samples were taken from plastic pots on 30th, 60th, and 120th days, dried and ground, and passed through 2- and 0.15-mm sieve holes. After the completion of soil culture, the maize seeds sterilized using sodium hypochlorite were sown in each plastic pot, and three maize seedlings were planted in each plastic pot after 1 week of growth. The maize seedlings were irrigated with ultrapure water during the growth period, and the maize plants were harvested after 30 days. Finally, 50 g of maize rhizosphere soil samples was collected for subsequent index determination. After 30 days of growth, the fresh and dry weights of the roots and shoots (within this report, the term “shoot” encompasses all aboveground tissues of the maize plants) of one part of the plant samples were measured. The second part of the plant samples was immediately frozen in liquid nitrogen for quenching for evaluating physiological index measurements. The last part of the plants samples was washed three times with 20 mM Na2-EDTA and then with deionized water for several times. The Cd content of plant samples was determined after drying.
2.4 Determination of soil and plant samples
2.4.1 Plant determination
During a microwave oven digestion procedure, plant samples were digested with 10 mL concentrated nitric acid, and then the ICP-MS was used to determine the Cd concentrations in plant samples.
Using the MDA detection kit and H2O2 detection kit, we measured the levels of MDA and H2O2 in the plant tissue homogenates at different concentrations (KeyGen Biotech). Furthermore, APX activity was measured using the Plant APX Elisa Kit (Xiamen Huijia Biotechnology Institute).
2.4.2 Soil determination
DTPA-extractable Cd was determined by mixing 20 g of soil with 50 mL DTPA-TEA solution (0.005 M DTPA, 0.1 M TEA, and 0.01 M CaCl2, pH = 7.3) (Hamid et al., 2018). The soil Cd fraction was determined using the BCR three-step continuous extraction method (Sun et al., 2023). The soil pH was determined as follows: soil and water was taken at a ratio of 1:2.5 (w/v), shaken for 1 min, and then allowed to settle for 30 min, and the pH of the supernatant was determined using a pH meter. The soil samples were subjected to digestion using a mixture of 5 mL of 1 M K2Cr2O7-H2SO4 and 5 mL of concentrated H2SO4, followed by boiling at 185°C for 5 min. The content of soil organic carbon was determined through the ferrous sulfate titration process, resulting in a red coloration. The soil organic matter was then calculated by multiplying the soil organic carbon value by a factor of 1.7 (Chen et al., 2019a). Soil phosphorus was extracted using a solution of 50.0 mL of 0.5 M NaHCO3 (pH = 8.5) added to 2.50 g of soil. The resulting mixture was shaken for 30 min and then filtered. The phosphorus content was quantified using the colorimetric molybdenum blue method, and the absorbance at 880 nm of the antimony–phospho-molybdate complex formed through the reaction of phosphate with acidic molybdate in the presence of antimony was measured. This complex was subsequently reduced by ascorbic acid, resulting in the emission of a blue color (Bar-Yosef and Akiri, 1978). Soil exchangeable potassium, calcium, and magnesium were determined by ammonium acetate leaching using AAS (CHENG and BRAY, 1951). By using the diethylenetriaminepentaacetic (DTPA) method, Fe, Cu, and Zn contents in the soil were measured (Lindsay and Norvell, 1978). Soil urease was determined by incubating the soil with urea at 37°C for 24 h and then analyzing the ammonia released from the soil (Tabatabai and Bremner, 1972). Soil peroxidase was determined using 0.002 mol·L−1 of potassium permanganate (Zhang et al., 2023). Soil dehydrogenase was determined by measuring the rate of reduction of tetrazolium chloride to triphenylmethanol in soil incubated at 30°C for 6 h (Casida et al., 1964). The soil samples underwent digestion using a combination of acids (HF–HCl–HNO3) at a ratio of 1:10. Specifically, 1 g of the soil sample was combined with 2.0 mL of HF, 2.0 mL of concentrated HCl, and 6.0 mL of HNO3 and subjected to microwave digestion at 160°C for 2 h. The concentrations of Cd in the resulting digestion solution were subsequently determined using ICP-MS (Huang et al., 2023).
2.5 Statistical analysis
One-way ANOVA was performed using SPSS 26.0 software with Duncan’s method for significance testing (p < 0.05). The underlying data were collated using Excel 2019 and plotted using Origin 2022 software.
3 Results
3.1 Biochar surface morphology and functional groups
In this study, SEM was utilized to examine the alterations in the surface morphology of the modified biochar. As shown in Figure 1, the surface of the unmodified biochar (BC) exhibited a relatively uneven texture, lacking distinct pore structure and harboring some impurities. However, upon loading the biochar with nanoparticles of MgO and Fe3O4, the surfaces of BC-Fe and BC-Mg became even rougher, displaying a more pronounced pore structure. Furthermore, the nano-MgO and -Fe3O4 particles were uniformly dispersed on the biochar surface and within the voids formed during pyrolysis.
The properties of the modified biochar were initially examined by FTIR and XRD (Figure 2). Obviously, the absorption peak at 3,600 cm−1 was attributed to the stretching vibration of -OH (Novak et al., 2010), and the absorption peak at 1,630 cm−1 was attributed to the -COO vibration of carboxylate. Additionally, the presence of -C=O at 1,320 cm−1 was found to be interconnected with the -CH at 1,120 cm−1 (Wu et al., 2019). Following the loading of MgO and Fe3O4 nanoparticles onto the biochar, distinctive peaks were observed at 560 cm−1 for BC-Fe, corresponding to Fe-O bonds (Xie et al., 2021), and at 740 cm−1 for BC-MgO, corresponding to Mg-O bonds (Wu et al., 2019), indicating the successful loading of the desired functional groups onto the modified BC. Additionally, XRD analysis was utilized to investigate the fraction of Mg and Fe present on the surfaces of BC-Fe and BC-Mg. The XRD results clearly indicated the presence of Fe3O4 on the surface of BC-Fe, while Mg was observed in the form of Mg-O on the surface of BC-Mg. In conclusion, successful loading of MgO and Fe3O4 onto the biochar surface was achieved.
3.2 Isothermal adsorption and adsorption kinetics experiments
This study investigated the adsorption performance of BC, BC-Fe, and BC-Mg on Cd using an isothermal adsorption model. The experimental data were analyzed using the Langmuir and Freundlich models as depicted in Figures 3B, C, respectively. The obtained model parameters are presented in Table 1. Notably, the R2 value of the Langmuir model surpassed that of the Freundlich model, indicating that the adsorption processes of BC, BC-Fe, and BC-Mg on Cd exhibited a higher degree of conformity to the Langmuir adsorption model. The maximum adsorption capacities for Cd by BC, BC-Fe, and BC-Mg were determined to be 7.32, 66.23, and 52.63 mg·g−1, respectively. Additionally, the maximum adsorption capacities of BC-MgO and BC-Fe were found to be 9.05 and 7.19 times greater than that of BC, respectively. Notably, BC-MgO demonstrated the highest KL value, suggesting a more robust and stable adsorption capacity for Cd.
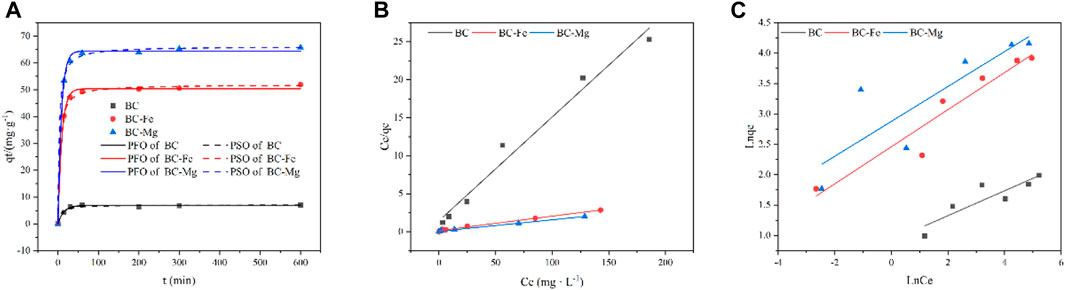
FIGURE 3. Curves of adsorption kinetics and adsorption isotherms. (A) Pseudo-first-order adsorption kinetics plot and pseudo-second-order adsorption kinetics plot. (B) Langmuir adsorption isotherm. (C) Freundlich adsorption isotherm.

TABLE 1. Parameters of Langmuir and Freundlich adsorption isotherm models of Cd adsorption on biochar.
The adsorption kinetics refers to the temporal change in the adsorption quantity of the adsorbent. As depicted in Figure 3A, the adsorption process of several BCs on Cd can be divided into two periods. Within the initial 0–60 min, the adsorption capacity of these biochars for Cd exhibited a rapid increase, reaching over 90% of the maximum adsorption capacity; after 60 min, the adsorption equilibrium was attained, signifying that the active adsorption sites on the biochar surface had reached saturation. Notably, no desorption of Cd by BC, BC-Fe, and BC-Mg was observed during the 600 min duration. The R2 values obtained from comparing the pseudo-first-order kinetic model and pseudo-second-order kinetic model for Cd adsorption by BC, BC-Fe, and BC-Mg suggest that BC-Fe and BC-Mg exhibit a stronger correlation with the pseudo-second-order kinetic model. Conversely, BC demonstrates a stronger correlation with the pseudo-first-order kinetic model (Table 2). This observation implies that the adsorption process of Cd by BC-Fe and BC-Mg is primarily governed by chemisorption.
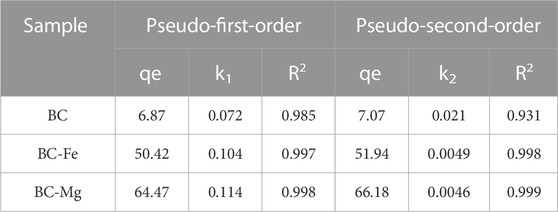
TABLE 2. Model parameters of pseudo-first-order kinetic and pseudo-second-order kinetic models of Cd adsorption on biochar.
3.3 Soil culture experiment
3.3.1 Soil DTPA-Cd and Cd fraction
As shown in Figure 4, it can be seen that BC, BC-Fe, and BC-Mg exhibit significant reductions in soil DTPA-Cd content, with BC-Mg demonstrating the lowest DTPA-Cd content at day 60. Furthermore, the passivation efficiency of BC and BC-Fe on soil Cd displays an initial increase followed by a decrease, indicating that BC-Mg possesses the timeliest effectiveness for Cd. At day 120, BC, BC-Fe, and BC-Mg reduce the DTPA-Cd content by 27.33%, 38.86%, and 50.85%, respectively (Figure 4A). Additionally, the passivation efficiency of BC-Fe and BC-Mg surpasses that of BC in the range of 5.6%–11.52% and 10.64%–23.51%, respectively.
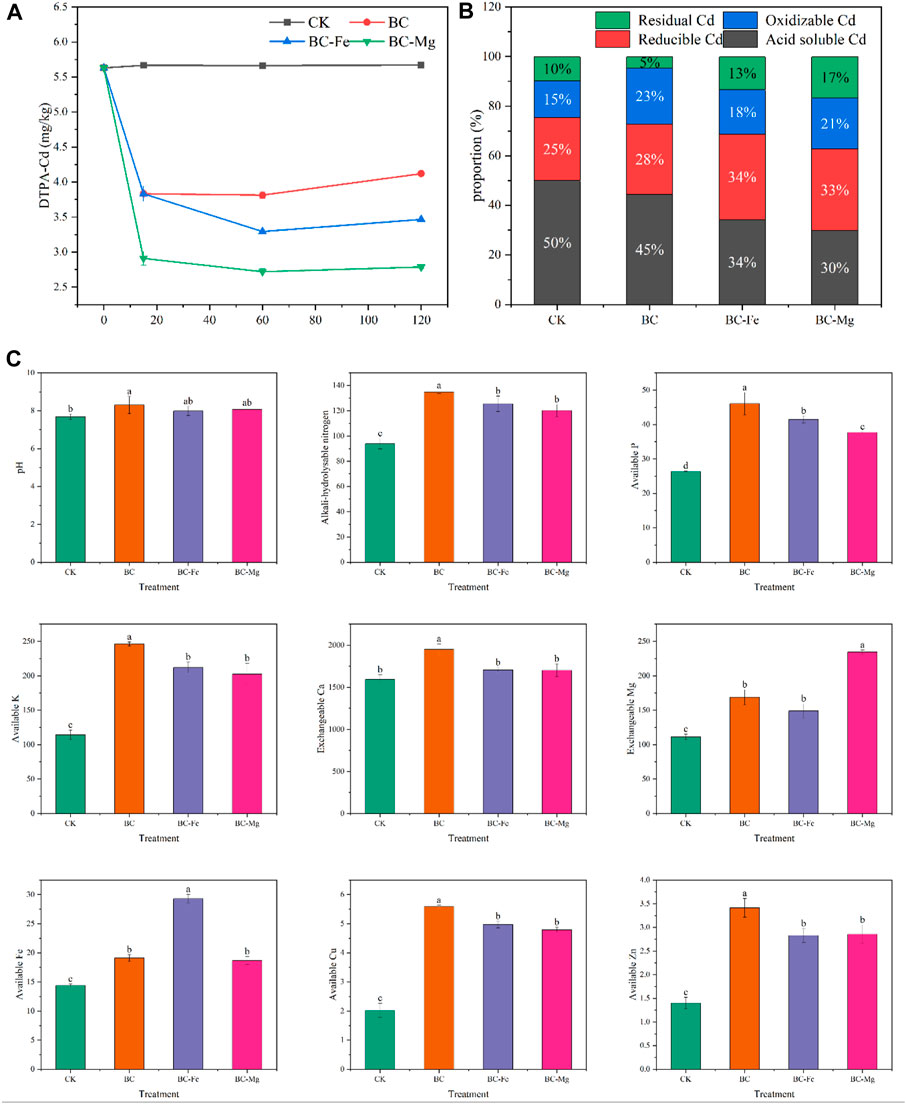
FIGURE 4. Effect of biochar on soil DTPA-Cd, Cd fraction, and soil physicochemical properties. (A) Dynamic changes in soil DTPA-Cd. (B) Soil Cd fraction at 120th day. (C) Soil physicochemical properties at 120th day.
Morphologically, BC, BC-Fe, and BC-Mg demonstrated reductions in soil acid-soluble Cd by 21.32%, 39.34%, and 47.34%, respectively (Figure 4B). It is worth noting that the oxidizable and residual fractions of soil Cd are considered to possess relatively stable characteristics and are less prone to absorption by plants (Chen et al., 2022). These fractions accounted for 28%, 31%, and 39% in BC, BC-Fe, and BC-Mg, respectively. Consequently, it can be concluded that BC-Mg exhibited the highest efficacy in passivating Cd.
3.3.2 Physicochemical properties of soil
The objective of this experiment was to investigate the impact of different BCs on the pH and physical and chemical properties of weakly alkaline soil. The results showed that BC significantly increased the soil pH value to 8.31, while BC-Fe and BC-Mg had no significant effect on soil pH (Figure 4C). Furthermore, all biochars demonstrated varying degrees of improvement in physical and chemical properties of soil. BC treatment exhibited the highest levels of alkali-hydrolyzed nitrogen, available phosphorus, available potassium, exchangeable calcium, available copper, and available zinc. These values were 44.65%, 74.38%, 115.50%, 22.28%, 176.11%, and 143.81% higher than those in CK treatment, respectively. BC-Fe exhibited the highest available iron content, which is 103.68% higher than that in CK. BC-Mg exhibited the highest exchangeable Mg content, which is 111.05% higher than that in CK (Figure 4C).
3.4 Pot experiment
3.4.1 The maize biomass and Cd content
The evaluation of biochar passivation efficacy can be effectively conducted by considering the biomass of plants, which serves as an intuitive indicator. As depicted in Figure 5A, all biochars demonstrated a significant increase in the dry and fresh weights of maize shoots and roots compared to the control group (CK). However, there were no notable differences in maize biomass observed among BC, BC-Fe, and BC-Mg. Specifically, the application of BC, BC-Fe, and BC-Mg resulted in significant increases in shoot fresh weight, with increments of 27.37%, 30.46%, and 38.11%, respectively. Furthermore, these treatments led to notable enhancements in the fresh weight of roots, with increments of 47.50%, 43.32%, and 64.28%, respectively. Additionally, the dry weight of shoots exhibited increases of 29.14%, 27.75%, and 52.97%, while the dry weight of roots experienced increments of 115.75%, 127.56%, and 134.60%.
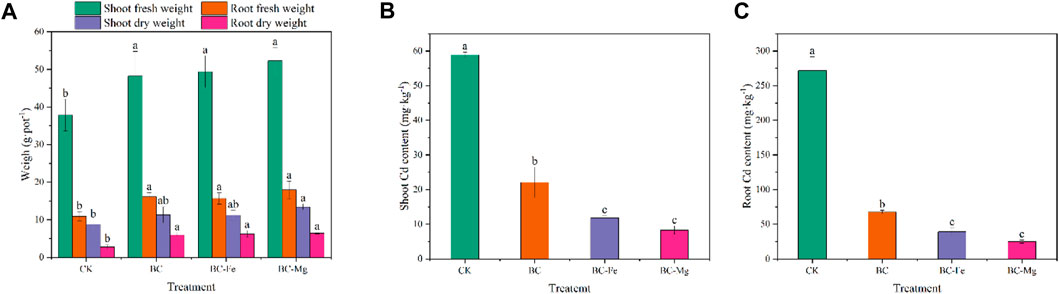
FIGURE 5. Effect of biochar on maize biomass and Cd content. (A) Dry and fresh weights of shoots and roots of maize. Cd content in shoots (B) and roots (C) of maize.
BC, BC-Fe, and BC-Mg demonstrated significant reductions in Cd contents in the shoot and root of maize. Specifically, the Cd content in the shoots decreased by 1.67, 3.97, and 6.09 times, while in the roots, the reductions were 3.01, 5.97, and 9.86 times, respectively. Furthermore, BC-Mg exhibited superior efficacy in reducing Cd absorption by crops to BC and BC-Fe.
3.4.2 The content of MDA and H2O2 and APX activity
To assess the presence of oxidative stress, the levels of H2O2 and MDA were quantified (Figure 6). Remarkably, the application of BC resulted in the lowest concentrations of H2O2 and MDA in both the shoot and root of maize, followed by BC-Mg treatment. Specifically, BC, BC-Fe, and BC-Mg treatments led to a reduction in the MDA content in the shoot by 189.33%, 79.28%, and 144.92% and in the root by 253.51%, 51.52%, and 107.99%, respectively. Additionally, the content of H2O2 in the shoot decreased by 123.46%, 24.35%, and 40.64% with BC, BC-Fe, and BC-Mg treatments, and in the root by 244.14%, 79.80%, and 26.38%, respectively.
This study aimed to assess the impact of Cd stress on the antioxidant system in maize plants. Specifically, the activity of APX was measured in both the shoots and roots of maize plants (Figure 6). The finding clearly observed that the APX activity in the BC, BC-Fe, and BC-Mg treatments was significantly lower than that in the CK treatment. This decrease in APX activity can be attributed to the lower levels of reactive oxygen species (ROS) in the biochar treatments, which consequently reduces the need for APX activity. Furthermore, the original biochar exhibited higher APX activity and demonstrated a greater ability to remove ROS than the modified biochar, aligning with the results obtained for ROS content in maize plants.
3.4.3 Soil enzyme activities, DTPA-Cd content, and Cd content
In order to assess the influence of biochar on the health of soil contaminated with Cd, the levels of soil dehydrogenase, catalase, and urease were quantified (Figures 7A–C, respectively). The application of BC, BC-Fe, and BC-Mg resulted in significant enhancements in soil urease activity, with increases of 67.81%, 39.46%, and 60.99%, respectively. Similarly, the application of BC, BC-Fe, and BC-Mg led to notable increases in dehydrogenase activity, with improvements of 76.31%, 75.73%, and 46.71%, respectively. It is noteworthy that the application of BC and BC-Fe resulted in a substantial increase in soil catalase activity by 43.22% and 17.92%, respectively, whereas BC-Mg led to a significant decrease in soil catalase activity by 20.69%. These findings clearly demonstrate that BC exhibits the most pronounced effect in enhancing soil enzyme activity, followed by BC-Fe, indicating that various biochar types elicit distinct biochemical responses within the soil.
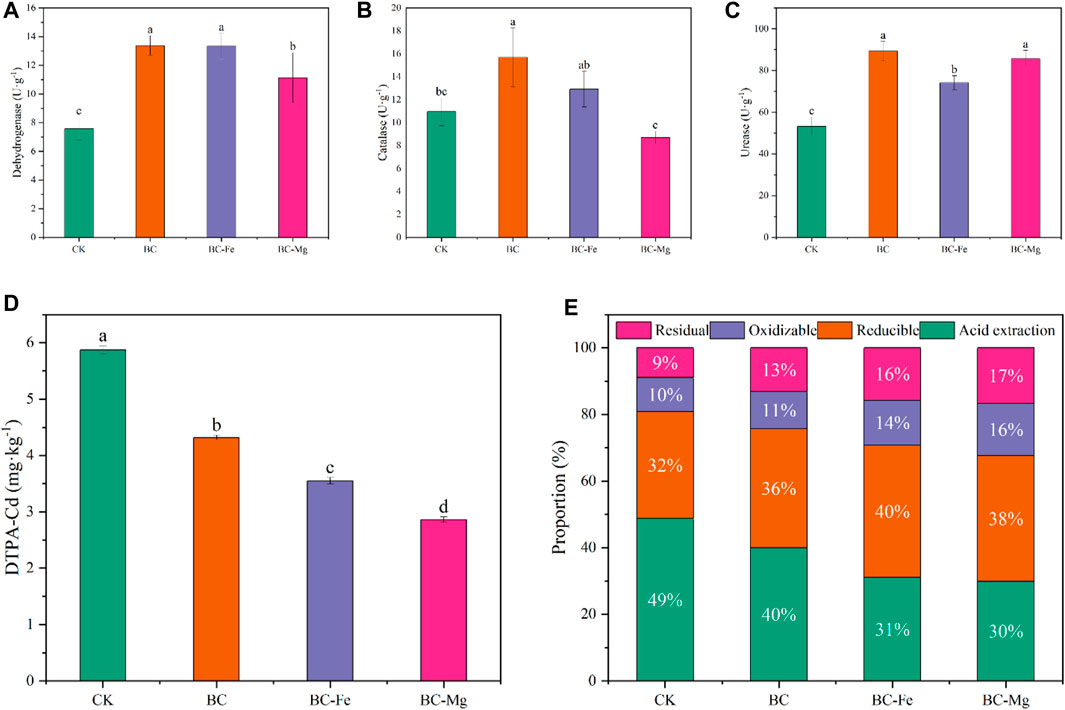
FIGURE 7. Soil enzyme activity, DTPA-Cd content, and Cd fraction of the pot experiment. (A) Soil dehydrogenase activity. (B) Soil catalase activity. (C) Soil urease activity. (D) Content of DTPA-Cd. (E) Proportion of the Cd fraction.
After a period of 120 days of soil culture and 30 days of pot planting, it was observed that BC, BC-Fe, and BC-Mg continued to exhibit significant reductions in DTPA-Cd levels by 26.41%, 39.52%, and 51.22% and acid-soluble Cd levels by 16.84%, 34.90%, and 37.98%, respectively. Additionally, both the original biochar and modified biochar were found to have the ability to convert Cd into a stable fraction. Specifically, BC, BC-Fe, and BC-Mg demonstrated increases in the oxidizable Cd levels by 10.94%, 35.16%, and 55.47%, and in the residual Cd by 49.11%, 79.46%, and 88.39%, respectively (Figures 7D, E).
4 Discussion
4.1 Mechanism of adsorption and passivation of Cd by modified biochar
Biochar has been found to be a highly effective adsorbent for the treatment of heavy metals in wastewater and for the passivation of soil in situ. Comparative analysis reveals that BC-Fe and BC-Mg exhibit superior performance in terms of Cd adsorption from aqueous solutions and the passivation of soil Cd compared to BC alone. Specifically, the maximum adsorption of Cd by BC-Fe and BC-Mg was found to be 9.05 and 7.19 times higher than that of BC, respectively. Furthermore, the passivation efficiencies of BC-Fe and BC-Mg for soil Cd were, respectively, 5.6%–11.52% and 10.64%–23.51% higher than BC. These findings suggest that BC-Mg exhibits the highest adsorption and passivation effects on Cd under the same environmental conditions. The mechanisms of Cd adsorption and passivation by biochar have been reported extensively, specifically, including physical adsorption (Zhang et al., 2019), electrostatic attraction (Wang et al., 2021b), complexation (Huang et al., 2019), ion exchange (Wu et al., 2021), and precipitation (Zhou et al., 2020). The different raw materials utilized in biomass can result in distinct dominant mechanisms pertaining to the adsorption or passivation of Cd. For example, biochar derived from municipal waste exhibits heightened electronegativity and ion-exchange capacity, whereas biochar sourced from plant residues possesses a more abundant array of the surface functional group (Zhao et al., 2015). The adsorption or passivation of Cd by pristine biochar involves a combination of several mechanisms, and the poor selective adsorption, barren pore structure, and unabundant surface functional groups lead to poor adsorption of Cd by pristine biochar, which often fails to achieve the desired results of researchers (Liu et al., 2023). For BC-Mg and BC-Fe, the loading of MgO and Fe3O4 nanoparticles on biochar can lead to a substantial enhancement in the adsorption capability of Cd. Following the introduction of MgO nanoparticles, in comparison to BC and BC-Fe, BC-Mg exhibited remarkable efficacy in adsorption and passivation of Cd in both aqueous solutions and soil. The hydrolysis of Mg2+ from the BC-Mg surface facilitates ion exchange with Cd. Furthermore, Cd can be precipitated through the ionization of OH- and CO32− by Mg(OH)2, resulting in a notable increase in the ion-exchange capacity and precipitation of BC-Mg for Cd (Wu et al., 2019; Wang et al., 2021a; Li et al., 2022a; Cheng et al., 2022). In addition to this main adsorption mechanism, isomorphous substitution and functional group complexation are also potential adsorption mechanisms of BC-Mg for Cd (Li et al., 2022b). The biochar loaded with Fe3O4 exhibits a potential of −0.44 V for Fe on the BC-Fe surface and possesses a strong electron-donating capacity (Mandal et al., 2021), which usually adsorbs and passivates Cd in the form of electrostatic attraction, which is in agreement with Chen et al. (2019b), Zhang et al. (2020), and Duan et al. (2022). In summary, the adsorption passivation mechanism of BC-Mg on Cd primarily involves surface precipitation, whereas that of BC-Fe primarily relies on electrostatic attraction. The analysis clearly indicated that the adsorption of Cd and the passivation of soil Cd by BC-Mg are considerably greater than those achieved by BC-Fe, as depicted in Figures 4, 7. This discrepancy in adsorption and passivation efficacies can be primarily attributed to distinct passivation mechanisms. Notably, the precipitation and ion exchange of Cd with BC-Mg demonstrate enhanced stability compared to electrostatic attraction. The potential desorption of Cd from the biochar surface should be taken into account, as it provides an explanation for the superior adsorption passivation capability of BC-Mg toward Cd.
4.2 Improvement of Cd-contaminated soil by the modified biochar
In order to facilitate the successful implementation of BC for the remediation of Cd-contaminated soils, it is crucial to consider the impact on the physicochemical properties of tested soils as a significant evaluation criterion. The assessment of soil properties in this study encompassed two primary dimensions. First, the influence of biochar on the nutrient composition of Cd-contaminated soil was examined to determine its potential to supply essential elements for plant growth. Second, the effect of biochar on the enzymatic activity of Cd-contaminated soil was evaluated as an indicator of soil health. The results of this study showed that BC, BC-Fe, and BC-Mg significantly increased soil alkali-hydrolyzed nitrogen, available phosphorus, available potassium, exchangeable calcium, exchangeable magnesium, exchangeable iron, exchangeable copper, and exchangeable zinc, indicating that pristine biochar and modified biochar can provide the nutrients required for plant growth (Figure 4C), laying the foundation for the practical application of biochar. The augmentation of maize plant biomass through biochar treatment provides additional evidence to support this notion. Moreover, the utilization of BC as a slow-release fertilizer not only diminishes the dispersion of soil nutrients but also curtails the reliance on chemical fertilizers, thereby emphasizing the practical significance of BC. Hussain et al. (2016) reported that the utilization of biochar has the potential to enhance the agricultural productivity of severely degraded lands characterized by low nutrient content and poor soil fertility. It is worth mentioning that the unmodified biochar demonstrated superior outcomes in terms of enhancing various nutrients, with the exception of BC-Mg, which displayed the highest level of exchangeable Mg, and BC-Fe, which exhibited the highest level of exchangeable Fe. This can be attributed to the incorporation of Fe and Mg elements during the biochar modification process, which led to the depletion of other elements from the biochar surface.
The activity of soil microorganisms can be assessed through the measurement of soil dehydrogenases (Bartkowiak et al., 2017). Additionally, the presence of soil urease is closely associated with the soil’s capacity to supply nitrogen (Chen et al., 2018). Furthermore, soil catalase plays a crucial role in the decomposition and conversion of peroxides within the soil, thereby mitigating the negative impact of ROS on soil health (Bian et al., 2020). Based on the findings of a comprehensive analysis, it was observed that the addition of BC, BC-Fe, and BC-Mg significantly enhanced the activity of soil dehydrogenase, catalase, and urease (Figures 7A–C). This suggests that BC, BC-Fe, and BC-Mg have the potential to improve the soil microbial environment and create a more favorable growth environment for crops in Cd-contaminated soils. It is noteworthy that BC demonstrated superior effects on enhancing enzyme activity in Cd-contaminated soils. Previous studies have revealed that the impact of biochar on soil enzymes is multifaceted and can even yield contradictory outcomes. On the one hand, the favorable pore structure of biochar facilitates the attachment of reaction substrates to its surface, thereby promoting the reaction. On the other hand, enzymes also become adsorbed onto the biochar surface, leading to a reduction in the enzymatic reaction and subsequently diminishing soil enzymatic activity (Lehmann et al., 2011). Hence, the aforementioned factors contributed to the observed variations in soil enzyme activity among BC, BC-Fe, and BC-Mg. Furthermore, it was observed that BC-Mg exhibited a reduction in soil peroxidase activity. This reduction can be attributed to the enhanced adsorption and passivation capabilities of BC-Mg toward soil Cd, as depicted in Figures 3, 4, 7. Consequently, BC-Mg effectively decreased the bioavailability of Cd and facilitated its conversion into a more stable form, thereby leading to a decline in soil peroxide levels and subsequently resulting in diminished peroxidase activity in BC-Mg-treated soil. In general, the findings of this investigation indicate that BC, BC-Mg, and BC-Fe have the potential to enhance soil nutrient levels to different extents, aligning with Ali et al. (2017), Adhikari et al. (2022), Tran et al. (2023), and Zhao et al. (2023). Nevertheless, taking into account the existence of the hazardous element Cd in the soil, BC-Mg emerged as the most favorable option following a comprehensive assessment of the outcomes derived from adsorption experiments, soil culture experiments, and pot experiments.
4.3 Effect and mechanism of biochar in alleviating Cd stress in plants
The passivation of Cd adsorption by MgO and Fe3O4 nanoparticle-loaded biochar has been previously documented, although there is limited research on its impact on crop growth. This study investigates the effects of BC, BC-Fe, and BC-Mg on maize plant biomass. Surprisingly, there were no significant differences in maize biomass between treatments with pristine biochar and BC-Fe and BC-Mg (Figure 5A). However, the concentrations of Cd in the shoot and root tissues were found to be significantly lower in the BC-Fe and BC-Mg treatments than in the BC treatment. This suggests that enhancing the soil environment may have a greater positive impact on plant growth and development in Cd-contaminated soil. Furthermore, this study also aimed to investigate the physiological mechanisms underlying the alleviation of Cd stress in plants by BC, BC-Fe, and BC-Mg, by measuring the levels of MDA, H2O2, and APX enzyme activities. The original biochar treatment exhibited the most notable reduction in MDA and H2O2 levels in both the shoots and roots of maize plants, surpassing the BC-Fe and BC-Mg treatments. This suggests that the original biochar treatment is more effective in facilitating the elimination of ROS from maize plants and safeguarding cells against oxidative damage, thereby enhancing the overall growth and development of plants. It is worth noting that APX, as highlighted by Su et al. (2016), plays a crucial role in scavenging ROS in plants. An intriguing discovery emerged from the study, as the CK treatment demonstrated the highest APX activity, contradicting the findings of several recent investigations (Kamran et al., 2020; Awad et al., 2022; Hussain et al., 2022; Pandey et al., 2022). This discrepancy could potentially be attributed to the elevated bioavailability of Cd in the CK treatment (Figures 7D, E), prompting the activation of the maize plants’ self-defense mechanism and triggering a more pronounced oxidative stress response, ultimately leading to a noteworthy augmentation in APX activity. In contrast, the application of BC, BC-Fe, and BC-Mg treatments resulted in a significant decrease in the bioavailability of Cd in the soil (as depicted in Figures 4, 7). This reduction in the soil bioavailability of Cd not only mitigated the oxidative stress response in maize plants but also potentially facilitated the elimination of ROS from the plant or regulated APX activity. These findings suggest that further investigation into the regulation of APX activity and its potential role in Cd removal is a promising avenue for future research. An additional significant observation was that, in comparison to BC-Fe and BC-Mg, pristine biochar exhibited the highest APX activity. This implies that biochar stimulated plants to enhance APX enzyme activity, consequently efficiently eliminating ROS. This phenomenon also accounts for the lowest levels of MDA and H2O2 in the BC treatment. When considering the collective results, it can be observed that the initial biochar treatment yielded the most favorable outcomes in terms of diminishing oxidative stress and augmenting the antioxidant system of maize plants. However, the levels of Cd content in the BC-Fe and BC-Mg treatments of maize plants were significantly lower than that of the original biochar treatment. This suggests that the oxidative stress experienced by maize plants in Cd-contaminated soil, resulting from the original biochar treatment, BC-Fe, and BC-Mg, fell within an acceptable range for the plants themselves. Consequently, it can be concluded that the ability of soil Cd passivation is ranked as follows: BC-Mg > BC-Fe > BC.
5 Conclusion
In this research, composites of BC-Fe and BC-Mg were synthesized, and their ability to adsorb and passivate Cd was thoroughly examined. Additionally, the mechanisms through which these composites alleviate Cd-induced stress in plants were investigated. The findings from both adsorption and soil incubation experiments confirmed that BC-Fe and BC-Mg exhibited significant enhancements in Cd adsorption and passivation effects. The observed Cd removal capacities were ranked as follows: BC-Mg > BC-Fe > BC. BC-Fe primarily removed Cd through the dominant mechanism of electrostatic attraction, while BC-Mg accomplished Cd removal through ion exchange and precipitation. The results of pot experiments revealed that BC-Fe and BC-Mg exhibited significant efficacy in the immobilization of Cd, concurrently enhancing soil fertility and ameliorating the soil environment. Consequently, the Cd concentration in both the shoots and roots of maize plants was reduced, while the dry and fresh weights of maize plants were augmented. Furthermore, BC-Fe and BC-Mg were found to facilitate the elimination of excessive ROS from the plant, thereby bolstering the antioxidant system and mitigating the adverse effects of Cd stress. This study contributes by conducting batch adsorption, soil culture, and pot experiments to compare the adsorption and passivation abilities of heavy metals between BC-Fe and BC-Mg. The findings lay a theoretical foundation for the practical implementation of BC-Mg.
Data availability statement
The data analyzed in this study is subjected to the following licenses/restrictions: contact the corresponding author to provide access to datasets. Requests to access these datasets should be directed to HD, ZGluZ2h1YXpoeUAxNjMuY29t.
Author contributions
HD: conception and design, drafting of the manuscript, and analysis and interpretation of the data. JL: critical revision of the manuscript for important intellectual content. QL: provision of study materials. ZL: statistical expertise. KX, LH, and XW: investigation. QY: obtaining funding and final approval of the manuscript. All authors contributed to the manuscript and approved the submitted version.
Funding
This study was supported by the National Natural Science Foundation of China (No. 32271643).
Conflict of interest
Authors HD, JL, and KX were employed by the company Wuhan Zhihuiyuan Environmental Protection Technology Co., Ltd.
The remaining authors declare that the research was conducted in the absence of any commercial or financial relationships that could be construed as a potential conflict of interest.
Publisher’s note
All claims expressed in this article are solely those of the authors and do not necessarily represent those of their affiliated organizations, or those of the publisher, the editors, and the reviewers. Any product that may be evaluated in this article, or claim that may be made by its manufacturer, is not guaranteed or endorsed by the publisher.
References
Adhikari, S., Timms, W., and Mahmud, M. A. P. (2022). Optimising water holding capacity and hydrophobicity of biochar for soil amendment – a review. Sci. Total Environ. 851, 158043. doi:10.1016/j.scitotenv.2022.158043
Ali, S., Rizwan, M., Qayyum, M. F., Ok, Y. S., Ibrahim, M., Riaz, M., et al. (2017). Biochar soil amendment on alleviation of drought and salt stress in plants: A critical review. Environ. Sci. Pollut. Res. 24, 12700–12712. doi:10.1007/s11356-017-8904-x
Amusat, S. O., Kebede, T. G., Dube, S., and Nindi, M. M. (2021). Ball-milling synthesis of biochar and biochar–based nanocomposites and prospects for removal of emerging contaminants: A review. J. Water Process Eng. 41, 101993. doi:10.1016/j.jwpe.2021.101993
Awad, M., Moustafa-Farag, M., Liu, Z., and El-Shazoly, R. M. (2022). Combined effect of biochar and salicylic acid in alleviating heavy metal stress, antioxidant enhancement, and Chinese mustard growth in a contaminated soil. J. Soil Sci. Plant Nutr. 22, 4194–4206. doi:10.1007/s42729-022-01018-0
Bar-Yosef, B., and Akiri, B. (1978). Sodium bicarbonate extraction to estimate nitrogen, phosphorus, and potassium availability in soils. Soil Sci. Soc. Am. J. 42, 319–323. doi:10.2136/sssaj1978.03615995004200020024x
Bartkowiak, A., Lemanowicz, J., and Hulisz, P. (2017). Ecological risk assessment of heavy metals in salt-affected soils in the Natura 2000 area (Ciechocinek, north-central Poland). Environ. Sci. Pollut. Res. Int. 24, 27175–27187. doi:10.1007/s11356-017-0323-5
Bian, X., Xiao, S., Zhao, Y., Xu, Y., Yang, H., and Zhang, L. (2020). Comparative analysis of rhizosphere soil physiochemical characteristics and microbial communities between rusty and healthy ginseng root. Sci. Rep. 10, 15756. doi:10.1038/s41598-020-71024-8
Bolan, N., Kunhikrishnan, A., Thangarajan, R., Kumpiene, J., Park, J., Makino, T., et al. (2014). Remediation of heavy metal(loid)s contaminated soils – to mobilize or to immobilize? J. Hazard. Mater. 266, 141–166. doi:10.1016/j.jhazmat.2013.12.018
Casida, L. E. J., Klein, D. A., and Santoro, T. (1964). Soil dehydrogenase activity. Soil Sci. 98, 371–376. doi:10.1097/00010694-196412000-00004
Chen, J., Li, J., Shen, W., Xu, H., Li, Y., and Luo, T. (2019a). The structure and species Co-occurrence networks of soil denitrifying bacterial communities differ between A coniferous and A broadleaved forests. Microorganisms 7, 361. doi:10.3390/microorganisms7090361
Chen, L., Guo, L., Liao, P., Xiong, Q., Deng, X., Gao, H., et al. (2022). Effects of biochar on the dynamic immobilization of Cd and Cu and rice accumulation in soils with different acidity levels. J. Clean. Prod. 372, 133730. doi:10.1016/j.jclepro.2022.133730
Chen, M., Tao, X., Wang, D., Xu, Z., Xu, X., Hu, X., et al. (2019b). Facilitated transport of cadmium by biochar-Fe3O4 nanocomposites in water-saturated natural soils. Sci. Total Environ. 684, 265–275. doi:10.1016/j.scitotenv.2019.05.326
Chen, T., Zhou, Z., Xu, S., Wang, H., and Lu, W. (2015). Adsorption behavior comparison of trivalent and hexavalent chromium on biochar derived from municipal sludge. Bioresour. Technol. 190, 388–394. doi:10.1016/j.biortech.2015.04.115
Chen, Y., Xu, X., Jiao, X., Sui, Y., Liu, X., Zhang, J., et al. (2018). Responses of labile organic nitrogen fractions and enzyme activities in eroded mollisols after 8-year manure amendment. Sci. Rep. 8, 14179. doi:10.1038/s41598-018-32649-y
Cheng, K. L., and Bray, R. H. (1951). Determination of calcium and magnesium in soil and plant material. Soil Sci. 72, 449–458. doi:10.1097/00010694-195112000-00005
Cheng, S., Zhao, S., Guo, H., Xing, B., Liu, Y., Zhang, C., et al. (2022). High-efficiency removal of lead/cadmium from wastewater by MgO modified biochar derived from crofton weed. Bioresour. Technol. 343, 126081. doi:10.1016/j.biortech.2021.126081
Chlopecka, A. (1996). Assessment of form of Cd, Zn and Pb in contaminated calcareous and gleyed soils in Southwest Poland. Sci. Total Environ. 188, 253–262. doi:10.1016/0048-9697(96)05182-0
Chou, J.-D., Wey, M.-Y., and Chang, S.-H. (2010). Study on Pb and PAHs emission levels of heavy metals- and PAHs-contaminated soil during thermal treatment process. J. Environ. Eng. 136, 112–118. doi:10.1061/(ASCE)EE.1943-7870.0000133
Culbard, E. b., Thornton, I., Watt, J., Wheatley, M., Moorcroft, S., and Thompson, M. (1988). Metal contamination in British urban dusts and soils. J. Environ. Qual. 17, 226–234. doi:10.2134/jeq1988.00472425001700020011x
Duan, L., Wang, Q., Li, J., Wang, F., Yang, H., Guo, B., et al. (2022). Zero valent iron or Fe3O4-loaded biochar for remediation of Pb contaminated sandy soil: Sequential extraction, magnetic separation, XAFS and ryegrass growth. Environ. Pollut. 308, 119702. doi:10.1016/j.envpol.2022.119702
Fu, H., Ma, S., Xu, S., Duan, R., Cheng, G., and Zhao, P. (2021). Hierarchically porous magnetic biochar as an efficient amendment for cadmium in water and soil: Performance and mechanism. Chemosphere 281, 130990. doi:10.1016/j.chemosphere.2021.130990
Ghosh, M. (2005). A review on phytoremediati ON OF heavy metals and utilizatiON OF its byproducts. Appl. Ecol. Env. Res. 3, 1–18. doi:10.15666/aeer/0301_001018
Hamid, Y., Tang, L., Wang, X., Hussain, B., Yaseen, M., Aziz, M. Z., et al. (2018). Immobilization of cadmium and lead in contaminated paddy field using inorganic and organic additives. Sci. Rep. 8, 17839. doi:10.1038/s41598-018-35881-8
Hemavathy, R. V., Kumar, P. S., Kanmani, K., and Jahnavi, N. (2020). Adsorptive separation of Cu(II) ions from aqueous medium using thermally/chemically treated Cassia fistula based biochar. J. Clean. Prod. 249, 119390. doi:10.1016/j.jclepro.2019.119390
Hu, X., Wang, J., Liu, Y., Li, X., Zeng, G., Bao, Z., et al. (2011). Adsorption of chromium (VI) by ethylenediamine-modified cross-linked magnetic chitosan resin: Isotherms, kinetics and thermodynamics. J. Hazard. Mater. 185, 306–314. doi:10.1016/j.jhazmat.2010.09.034
Huang, C., Guo, Z., Li, T., Xu, R., Peng, C., Gao, Z., et al. (2023). Source identification and migration fate of metal(loid)s in soil and groundwater from an abandoned Pb/Zn mine. Sci. Total Environ. 895, 165037. doi:10.1016/j.scitotenv.2023.165037
Huang, K., Hu, C., Tan, Q., Yu, M., Shabala, S., Yang, L., et al. (2022). Highly efficient removal of cadmium from aqueous solution by ammonium polyphosphate-modified biochar. Chemosphere 305, 135471. doi:10.1016/j.chemosphere.2022.135471
Huang, M., Li, Z., Luo, N., Yang, R., Wen, J., Huang, B., et al. (2019). Application potential of biochar in environment: Insight from degradation of biochar-derived DOM and complexation of DOM with heavy metals. Sci. Total Environ. 646, 220–228. doi:10.1016/j.scitotenv.2018.07.282
Hussain, S., Irfan, M., Sattar, A., Hussain, S., Ullah, S., Abbas, T., et al. (2022). Alleviation of cadmium stress in wheat through the combined application of boron and biochar via regulating morpho-physiological and antioxidant defense mechanisms. Agronomy 12, 434. doi:10.3390/agronomy12020434
Hussain, S., Khan, F., Cao, W., Wu, L., and Geng, M. (2016). Seed priming alters the production and detoxification of reactive oxygen intermediates in rice seedlings grown under sub-optimal temperature and nutrient supply. Front. Plant Sci. 7, 439. doi:10.3389/fpls.2016.00439
Kamran, M., Malik, Z., Parveen, A., Huang, L., Riaz, M., Bashir, S., et al. (2020). Ameliorative effects of biochar on rapeseed (Brassica napus L) growth and heavy metal immobilization in soil irrigated with untreated wastewater. J. Plant Growth Regul. 39, 266–281. doi:10.1007/s00344-019-09980-3
Kim, H.-S., Kim, K.-R., Kim, H.-J., Yoon, J.-H., Yang, J. E., Ok, Y. S., et al. (2015). Effect of biochar on heavy metal immobilization and uptake by lettuce (Lactuca sativa L) in agricultural soil. Environ. Earth Sci. 74, 1249–1259. doi:10.1007/s12665-015-4116-1
Kołodyńska, D., Wnętrzak, R., Leahy, J. J., Hayes, M. H. B., Kwapiński, W., and Hubicki, Z. (2012). Kinetic and adsorptive characterization of biochar in metal ions removal. Chem. Eng. J. 197, 295–305. doi:10.1016/j.cej.2012.05.025
Lehmann, J., Rillig, M. C., Thies, J., Masiello, C. A., Hockaday, W. C., and Crowley, D. (2011). Biochar effects on soil biota – a review. Soil Biol. Biochem. 43, 1812–1836. doi:10.1016/j.soilbio.2011.04.022
Li, A., Xie, H., Qiu, Y., Liu, L., Lu, T., Wang, W., et al. (2022a). Resource utilization of rice husk biomass: Preparation of MgO flake-modified biochar for simultaneous removal of heavy metals from aqueous solution and polluted soil. Environ. Pollut. 310, 119869. doi:10.1016/j.envpol.2022.119869
Li, A., Zhang, Y., Ge, W., Zhang, Y., Liu, L., and Qiu, G. (2022b). Removal of heavy metals from wastewaters with biochar pyrolyzed from MgAl-layered double hydroxide-coated rice husk: Mechanism and application. Bioresour. Technol. 347, 126425. doi:10.1016/j.biortech.2021.126425
Li, J. T., Qiu, J. W., Wang, X. W., Zhong, Y., Lan, C. Y., and Shu, W. S. (2006). Cadmium contamination in orchard soils and fruit trees and its potential health risk in Guangzhou, China. Environ. Pollut. 143, 159–165. doi:10.1016/j.envpol.2005.10.016
Lindsay, W. L., and Norvell, W. A. (1978). Development of a DTPA soil test for zinc, iron, manganese, and copper. Soil Sci. Soc. Am. J. 42, 421–428. doi:10.2136/sssaj1978.03615995004200030009x
Liu, Q., Deng, W.-Y., Zhang, L.-Y., Liu, C.-X., Jie, W.-W., Su, R.-X., et al. (2023). Modified bamboo charcoal as a bifunctional material for methylene blue removal. Materials 16, 1528. doi:10.3390/ma16041528
Lonappan, L., Liu, Y., Rouissi, T., Brar, S. K., and Surampalli, R. Y. (2020). Development of biochar-based green functional materials using organic acids for environmental applications. J. Clean. Prod. 244, 118841. doi:10.1016/j.jclepro.2019.118841
Ma, L., Zhong, H., and Wu, Y.-G. (2015). Effects of metal-soil Contact time on the extraction of mercury from soils. Bull. Environ. Contam. Toxicol. 94, 399–406. doi:10.1007/s00128-015-1468-x
Ma, Y., Qi, Y., Yang, L., Wu, L., Li, P., Gao, F., et al. (2021). Adsorptive removal of imidacloprid by potassium hydroxide activated magnetic sugarcane bagasse biochar: Adsorption efficiency, mechanism and regeneration. J. Clean. Prod. 292, 126005. doi:10.1016/j.jclepro.2021.126005
Mandal, S., Pu, S., Adhikari, S., Ma, H., Kim, D.-H., Bai, Y., et al. (2021). Progress and future prospects in biochar composites: Application and reflection in the soil environment. Crit. Rev. Environ. Sci. Technol. 51, 219–271. doi:10.1080/10643389.2020.1713030
Natarajan, R., Al Fazari, F., and Al Saadi, A. (2018). Municipal waste water treatment by natural coagulant assisted electrochemical technique—parametric effects. Environ. Technol. Innovation 10, 71–77. doi:10.1016/j.eti.2018.01.011
Novak, J. M., Busscher, W. J., Watts, D. W., Laird, D. A., Ahmedna, M. A., and Niandou, M. A. S. (2010). Short-term CO2 mineralization after additions of biochar and switchgrass to a Typic Kandiudult. Geoderma 154, 281–288. doi:10.1016/j.geoderma.2009.10.014
Pandey, B., Suthar, S., and Chand, N. (2022). Effect of biochar amendment on metal mobility, phytotoxicity, soil enzymes, and metal-uptakes by wheat (Triticum aestivum) in contaminated soils. Chemosphere 307, 135889. doi:10.1016/j.chemosphere.2022.135889
Prokop, Z., Cupr, P., Zlevorova-Zlamalikova, V., Komarek, J., Dusek, L., and Holoubek, I. (2003). Mobility, bioavailability, and toxic effects of cadmium in soil samples. Environ. Res. 91, 119–126. doi:10.1016/S0013-9351(02)00012-9
Qiu, B., Tao, X., Wang, H., Li, W., Ding, X., and Chu, H. (2021). Biochar as a low-cost adsorbent for aqueous heavy metal removal: A review. J. Anal. Appl. Pyrolysis 155, 105081. doi:10.1016/j.jaap.2021.105081
Su, Y., Wang, Z., Xu, L., Peng, Q., Liu, F., Li, Z., et al. (2016). Early selection for smut resistance in sugarcane using pathogen proliferation and changes in physiological and biochemical indices. Front. Plant Sci. 7, 1133. doi:10.3389/fpls.2016.01133
Sun, L., Zhang, G., Li, X., Zhang, X., Hang, W., Tang, M., et al. (2023). Effects of biochar on the transformation of cadmium fractions in alkaline soil. Heliyon 9, e12949. doi:10.1016/j.heliyon.2023.e12949
Tabatabai, M. A., and Bremner, J. M. (1972). Assay of urease activity in soils. Soil Biol. Biochem. 4, 479–487. doi:10.1016/0038-0717(72)90064-8
Teng, D., Zhang, B., Xu, G., Wang, B., Mao, K., Wang, J., et al. (2020). Efficient removal of Cd(II) from aqueous solution by pinecone biochar: Sorption performance and governing mechanisms. Environ. Pollut. 265, 115001. doi:10.1016/j.envpol.2020.115001
Tran, H.-T., Bolan, N. S., Lin, C., Binh, Q. A., Nguyen, M.-K., Luu, T. A., et al. (2023). Succession of biochar addition for soil amendment and contaminants remediation during co-composting: A state of art review. J. Environ. Manag. 342, 118191. doi:10.1016/j.jenvman.2023.118191
Tyagi, U. (2022). Enhanced adsorption of metal ions onto Vetiveria zizanioides biochar via batch and fixed bed studies. Bioresour. Technol. 345, 126475. doi:10.1016/j.biortech.2021.126475
Wang, Y.-P., Liu, Y.-L., Tian, S.-Q., Yang, J.-J., Wang, L., and Ma, J. (2021b). Straw biochar enhanced removal of heavy metal by ferrate. J. Hazard. Mater. 416, 126128. doi:10.1016/j.jhazmat.2021.126128
Wang, Y., Wang, L., Li, Z., Yang, D., Xu, J., and Liu, X. (2021a). MgO-laden biochar enhances the immobilization of Cd/Pb in aqueous solution and contaminated soil. Biochar 3, 175–188. doi:10.1007/s42773-020-00080-0
Wu, J., Wang, T., Wang, J., Zhang, Y., and Pan, W.-P. (2021). A novel modified method for the efficient removal of Pb and Cd from wastewater by biochar: Enhanced the ion exchange and precipitation capacity. Sci. Total Environ. 754, 142150. doi:10.1016/j.scitotenv.2020.142150
Wu, L., Wei, C., Zhang, S., Wang, Y., Kuzyakov, Y., and Ding, X. (2019). MgO-modified biochar increases phosphate retention and rice yields in saline-alkaline soil. J. Clean. Prod. 235, 901–909. doi:10.1016/j.jclepro.2019.07.043
Xie, J., Lin, R., Liang, Z., Zhao, Z., Yang, C., and Cui, F. (2021). Effect of cations on the enhanced adsorption of cationic dye in Fe3O4-loaded biochar and mechanism. J. Environ. Chem. Eng. 9, 105744. doi:10.1016/j.jece.2021.105744
Xu, X., Cao, X., Zhao, L., Wang, H., Yu, H., and Gao, B. (2013). Removal of Cu, Zn, and Cd from aqueous solutions by the dairy manure-derived biochar. Environ. Sci. Pollut. Res. 20, 358–368. doi:10.1007/s11356-012-0873-5
Zahedifar, M., Seyedi, N., Shafiei, S., and Basij, M. (2021). Surface-modified magnetic biochar: Highly efficient adsorbents for removal of Pb(ΙΙ) and Cd(ΙΙ). Mater. Chem. Phys. 271, 124860. doi:10.1016/j.matchemphys.2021.124860
Zhang, J.-Y., Zhou, H., Gu, J.-F., Huang, F., Yang, W.-J., Wang, S.-L., et al. (2020). Effects of nano-Fe3O4-modified biochar on iron plaque formation and Cd accumulation in rice (Oryza sativa L). Environ. Pollut. 260, 113970. doi:10.1016/j.envpol.2020.113970
Zhang, J., Shao, J., Jin, Q., Li, Z., Zhang, X., Chen, Y., et al. (2019). Sludge-based biochar activation to enhance Pb(II) adsorption. Fuel 252, 101–108. doi:10.1016/j.fuel.2019.04.096
Zhang, W., Tong, L., Yuan, Y., Liu, Z., Huang, H., Tan, F., et al. (2010). Influence of soil washing with a chelator on subsequent chemical immobilization of heavy metals in a contaminated soil. J. Hazard. Mater. 178, 578–587. doi:10.1016/j.jhazmat.2010.01.124
Zhang, Y., Yang, S., Yang, J., Wu, Z., Liu, H., Nie, Z., et al. (2023). Temporal hormetic response of soil microbes to cadmium: A metagenomic perspective. Sci. Total Environ. 891, 164190. doi:10.1016/j.scitotenv.2023.164190
Zhao, L., Cao, X., Zheng, W., Wang, Q., and Yang, F. (2015). Endogenous minerals have influences on surface electrochemistry and ion exchange properties of biochar. Chemosphere 136, 133–139. doi:10.1016/j.chemosphere.2015.04.053
Zhao, Y., Li, X., Li, Y., Bao, H., Xing, J., Zhu, Y., et al. (2023). Biochar acts as an emerging soil amendment and its potential ecological risks: A review. Energies 16, 410. doi:10.3390/en16010410
Keywords: Fe3O4- and MgO-loaded biochars, adsorption, soil remediation, maize growth, heavy metal
Citation: Ding H, Liu J, Li Q, Liu Z, Xia K, Hu L, Wu X and Yan Q (2023) Highly effective adsorption and passivation of Cd from wastewater and soil by MgO- and Fe3O4-loaded biochar nanocomposites. Front. Environ. Sci. 11:1239842. doi: 10.3389/fenvs.2023.1239842
Received: 14 June 2023; Accepted: 26 July 2023;
Published: 09 August 2023.
Edited by:
Jing Ding, Harbin Institute of Technology, ChinaReviewed by:
Xianqiang Yin, Northwest A&F University, ChinaXiaokai Zhang, Jiangnan University, China
Lixin Li, Heilongjiang University of Science and Technology, China
Copyright © 2023 Ding, Liu, Li, Liu, Xia, Hu, Wu and Yan. This is an open-access article distributed under the terms of the Creative Commons Attribution License (CC BY). The use, distribution or reproduction in other forums is permitted, provided the original author(s) and the copyright owner(s) are credited and that the original publication in this journal is cited, in accordance with accepted academic practice. No use, distribution or reproduction is permitted which does not comply with these terms.
*Correspondence: Qian Yan, eWFucWlhbkBnZGFhcy5jbg==