- Division of Sustainable Development, College of Science and Engineering, Hamad Bin Khalifa University, Qatar Foundation, Doha, Qatar
Introduction: As a result of the rapid increase in population and depletion of natural resources, particularly energy and water, approximately 200 million people are expected to face hunger by 2030. Going forward, the sustainability of energy, water, and food (EWF) resources can be enhanced by considering a nexus approach, which supports effective resource management by identifying synergies and trade-offs. Furthermore, the regeneration of biomass into value-added products, such as biochar (BC), can reduce cross-sectoral environmental impacts and support the EWF nexus.
Methodology: This research investigates the optimum options for utilizing BC within the context of the EWF nexus for both wastewater treatment and soil applications whilst considering the optimal blending ratio of various biomass (camel manure, date pits, sewage sludge, and coffee waste) for both single-end use and multi-end use stages to fulfill various objectives within defined scenarios based on maximum savings in energy, water, cost, and emissions.
Results and discussion: The single-end use stage considered using BC for wastewater treatment (WWT) and as a soil amendment (SA) individually, and 18 optimal solutions were collected for this stage. The optimization of the multi-end use stage resulted in 70 optimal solutions, where BC was applied for both WWT and SA. The solutions that leaned toward SA application suggested that BC should consist of 97%–99% of date pits with relatively smaller proportions of the other biomass. On the other hand, the other solutions that leaned toward the WWT pathway suggested that the optimum biomass mix should consist of relatively equal proportions of camel manure, sewage sludge, and coffee waste of 29%—33% and smaller amounts of date pits of approximately 2%–5%.
1 Introduction
Solid waste generation increased from 6.94 million tons in 2021 to 7.39 million tons in 2022, in which 3.83 and 4.19 million tons were recycled globally in 2021 and 2022, respectively (National Environment Agency, 2019). Furthermore, global municipal solid waste generation is expected to increase by approximately 70% to reach 3.4 billion metric tons in 2050 due to population growth, urbanization, and economic growth (Tiseo, 2021). Production, incineration, and transportation for the removal of waste result in greenhouse gas emissions, whereas global waste output contributes to 3.2% of global CO2 emissions (Ritchie and Roser, 2020).
After decades of economic growth and technological advances in agriculture, achieving global food security remains a growing challenge (Campi et al., 2021; D. Poudel and Gopinath, 2021). The World Food Summit conceptualized that “food security exists when all people, at all times, have physical, social and economic access to sufficient, safe and nutritious food to meet their dietary needs and food preferences for an active and healthy life” (Summit, 1996). The Food and Agriculture Organization (FAO) estimated that between 720 and 811 million people in the world faced hunger in 2020, where nearly one in three did not have access to adequate food, resulting in 118 million more people facing hunger in 2020 compared to 2019. Furthermore, due to the lasting effects of the COVID-19 pandemic on global food security, approximately 300 million people may still face hunger in 2030 (FAOSTAT, 2022).
Food security is very tightly linked with water security. Water is vital for all forms of life, essential to public health and human wellbeing, and smoothens the path toward ending poverty (Irannezhad et al., 2022). High water scarcity impacts approximately 3.2 billion rural people, where one-sixth of the world’s population live in agricultural areas (FAOSTAT, 2022) and 40% live in dryland (Stringer et al., 2021). In addition, more than 170 million hectares, which is approximately 60% of irrigated croplands, are subject to high water stress (FAOSTAT, 2022). Water scarcity has led to economic losses, social conflicts, and an increasing international competition for water resources (Kumar et al., 2022), which in turn affects both the food and energy sectors. Planet Earth has limited freshwater resources. The total global volume of water is 1.4 billion km3, but only 35 million km3 of this resource is freshwater, and much of it is locked in the form of ice. Thus, only 105,000 km3 of freshwater is accessible for use as water supply, and 70% of all withdrawals of freshwater are currently used for agriculture, primarily for food production (Khokhar, 2017; Falconer, 2021). For example, in 2020, 48% of Afghanistan’s population had access to water supplies, while only 28% had access to clean water free of contaminants. Meanwhile, in Canada, approximately 99% of its population has access to clean water supplies (WHO/UNICEF, 2021), which highlights the disproportionate distribution of water resources around the world and, thus, hinders the progression toward achieving global water and food security.
The increase in water and food insecurities will create barriers that restrict the development of modern society (Falconer, 2021; Yuxi et al., 2021; Vieira da Silva Filho et al., 2022). Attaining food and water security requires the management of these natural resources through a comprehensive policy structure. The United Nations introduced the Sustainable Development Goals (SDGs) in September 2015 as targets to be achieved by 2030; these goals seek to reconcile economic growth, environmental balance, and social progress, ensuring that all people have the same opportunities. In this regard, SDG 2 focuses on achieving zero hunger by ending all forms of malnutrition (Herrmann and Rundshagen, 2020), whereas SDG 6 aims to provide clean and sanitized water, addressing the quality and sustainability of water resources (Requejo-Castro et al., 2020; Partzsch et al., 2021), which are critical to the survival of people and the planet (Haas and Ivanovskis, 2022).
Today, resources are stressed because of rapid population growth, the increasing demand and consumption of various products and services, scarcity of resources, and elevated greenhouse gas (GHG) emissions leading to climate change (Hao et al., 2022; Schmidt et al., 2022). Therefore, it is necessary to manage these resources while considering the external pressures and the inherent interdependencies that exist between them in what is known as the nexus of the three resources: energy, water, and food (Bazilian et al., 2011; Al-Ansari et al., 2015; Allan et al., 2015; De Laurentiis et al., 2016; Mannan et al., 2018; Ghiat and Al-Ansari, 2021). The energy–water–food (EWF) nexus was first recognized in 1983 by the United Nations University in their Food–Energy Nexus Program and was first proposed at the Bonn conference held in Germany in 2011 (Sachs and Silk, 1990). The nexus alludes to the inextricable linkages, synergies, and trade-offs between energy, water, and food sectors and technology sub-systems (Bonn 2011 Conference, 2012). The management of EWF resources leads to optimum consumption of resources, minimizes environmental impacts (Endo et al., 2017; Owen et al., 2018), enhances resilience (Govindan and Al-Ansari, 2019; Sukhwani et al., 2019), and ultimately promotes security of food, water, and energy resources (Wong, 2015).
An important feature within the EWF nexus and the broader sustainability agenda is the role of waste in value-added products (Fouladi et al., 2021). Numerous waste-to-energy technologies have been established, including gasification and pyrolysis thermochemical processes, where the former mainly produces syngas whilst the latter mainly produces biochar. These processes can be influenced by several factors, including the composition of the feedstock and the process parameters (Al-Rumaihi et al., 2021; Al-Rumaihi, Shahbaz, et al., 2022; AlNouss et al., 2022; Mariyam et al., 2022; Parthasarathy, Al-Ansari, et al., 2022). In pyrolysis, biomass, which is a carbon-based renewable organic material generated from animals and plants, is converted into value-added products in the absence of oxygen: biogas, bio-oil, and biochar (Elkhalifa et al., 2019; Shahbaz et al., 2021). BC is utilized in modern society for multiple agricultural and environmental purposes in the framework of circular economy (CE) (Jindo et al., 2020), where different strategies for BC utilization have been considered: a) as a soil amendment, increasing the crop yield, reducing chemical fertilizer (CF) requirements, sequestering carbon in the soil by absorbing it from the atmosphere to mitigate climate change, and enhancing the water retention due to its porous structure (Mukherjee and Lal, 2013; Mylavarapu et al., 2013; Smith, 2016); b) as a means for water treatment with an approximate 85% recovery of wastewater of high purity (Gupta et al., 2022); and c) as a source of energy replacing non-renewable resources. Evidently, biochar demonstrates potential in reducing environmental impacts and climate change, contributing toward optimizing EWF nexus systems while contributing toward a circular economy (Alherbawi et al., 2020; Kua et al., 2020; Almanassra et al., 2021; Hu et al., 2021).
Considering an EWF approach requires the identification of the existing synergies and trade-offs between the energy, water, and food sectors (Al-Ansari et al., 2015; Alherbawi et al., 2021). Analysis of the interactions between the EWF resource system will facilitate integrated planning and decision making (Rosales-Asensio et al., 2020). Applying one of the different levels of decision-making tools—strategical, tactical, or operational—will ensure continuous access and enable efficient management of spatially distributed EWF resources toward the development of a resilient and sustainable EWF nexus system (Namany et al., 2019; Haji et al., 2022). Examples of decision-making tools include life cycle assessment (LCA), optimization, machine learning, and agent-based modeling (Namany and Al-Ansari, 2021). Optimization can solve large problems concisely and analyze the solutions using linear and non-linear formulations, which identify the best fit solutions (Singh, 2012). In terms of EWF nexus studies, optimization was implemented to achieve several objectives: maximizing the resilience of EWF systems, minimizing CO2 emissions, optimizing the regulation of water, soil, and energy resources, improving regional cropland management techniques for developing sustainable circular agriculture, and boosting the economic development of disadvantaged rural communities (Cansino-Loeza et al., 2021; H; Li et al., 2022; M; Li et al., 2022; Núñez-López et al., 2022). Optimization models aim to quantify the interactions within the EWF nexus and analyze trade-offs between the three sectors in terms of their utilization efficiency, economic benefits, and environmental impact (M. Li et al., 2021).
In this study, a multi-objective optimization tool is used to assess the utilization of BC from four different types of biomass: camel manure, date pits, sewage sludge, and coffee waste. Two pathways were considered for the end-use: biochar-based fertilizer for SA and biochar-based adsorbent for WWT. Novel to this study is the objective of investigating the best biomass blend and end-use mix for the production and application of biochar that contributes the largest toward achieving EWF resource security within the highest energy, water, cost, and global warming potential (GWP) savings possible.
The structure of this study is as follows: Section 2 reviews previous EWF nexus studies in addition to reviewing biochar and its various applications. Section 3 introduces the methodology, which includes three main steps: BC production, BC utilization analysis, and multi-objective optimization. Section 4 discusses the results obtained from each step, and finally, Section 5 concludes the study.
2 Literature review
As this research paper is built on a solid background from the literature, this section reviews EWF nexus studies in addition to water, energy, and food resource security. It also reviews the production of biochar, its applications, and its relation with the EWF nexus economy and circular economy.
2.1 Energy–water–food nexus
The world is challenged by freshwater scarcity, drying of groundwater resources, increased food consumption, and the depletion of non-renewable resource availability (Mannan et al., 2018; Al-Enazi et al., 2021; Bilal et al., 2021). Adopting a nexus approach to the management of critical resources may support alleviating some of the burdens on the environment and society, in addition to enhancing the security of energy, water, and food resources. In the literature, the EWF nexus is multi-dimensional, covering various objectives, case studies, methodologies, and approaches. Bieber et al. (2018) developed a methodology to support resilient and sustainable planning at the city level for multiple sectors and applications within urban energy systems and the EWF nexus. The results highlighted that investment to supporting Ghana’s infrastructure and agriculture intensification will effectively increase the share of renewable energy and carbon emissions. Rasul and Sharma (2016) discussed the potential synergies and trade-offs between the three sectors, which provided a conceptual framework for effective adaptation responses to climate change. Highlighting the impact of global environmental and economic changes on Brazil, Mercure et al. (2019) identified governance shortcomings in the context of the EWF nexus and analyzed the complex interdependence of developments at both the global and local levels. Namany et al. (2021) developed a methodology to assess the performance of EWF sectors in Qatar based on the analytical hierarchy process. The results unveiled the vulnerability of the three sectors to internal and external risks, which is represented by integrated resilience indexes of 0.21, 0.17, and 0.10 for the food, water, and energy sectors, respectively. Compared to the other sectors, the water system displayed the lowest security level with a high criticality score of 0.14, whilst the food system was found to be the least sustainable. Al-Ansari et al. (2015, 2017) integrated greenhouse gas control technologies within an EWF nexus tool and evaluated the environmental impact of a hypothetical food product system of 40% food self-sufficiency. As a result of this study, the global warming potential was reduced by approximately 98% for the different scenarios through the integration of bio-energy carbon capture and storage (BECCS) and solar energy. Ghiat et al. (2021) evaluated the effectuality of BECCS or utilization pathways by utilizing carbon dioxide (CO2) for enriching food systems and reducing GHG emissions within the EWF nexus concept. The results of this study revealed that the CO2 captured from an energy sub-system is utilized to enhance the food sub-system by increasing the yield by 13.8% and reducing crop water requirements by 28%, therefore achieving negative CO2 emissions of 24.6 kg/m2 year.
Whilst many researchers focused on attaining the security of food, water, and energy resources, some researchers were more involved in obtaining the security of only one of the aforementioned sectors. For instance, Namany et al. (2019) assessed both technology alternatives and environmental performance for a domestic food production case in Qatar, and the study indicated that diversifying the energy and water mix introduces more than 70% of renewable energy technologies, which can be used as a sustainable alternative by the food sector. De Laurentiis et al. (2016) studied the potential of LCA in assessing the performance of food service providers by applying an EWF approach. The study identified improvement measures in line with the promotion of shifts in both production and consumption patterns. Al-Thani et al. (2020) evaluated the possibility of maximizing the nutritional value of agricultural output through the optimal allocation of water and energy resources. The study concluded that dates and fish are strategic crops due to their contribution to food security, whilst poultry and meat groups are not recommended due to their high water and energy requirements.
Marttunen et al. (2019) developed a structured and systematic method to assess water security and its future trends in Finland via a framework that established a criteria hierarchy for water security consisting of four main themes; this enabled the analysis of relationships between the water security criteria as well as between EWF security. Mirzaei et al. (2019) estimated groundwater withdrawal from electric-powered irrigation wells, examined agronomic productivity in Iran through the lens of the EWF nexus, and shed light on some of the root causes of water shortening problems of the nation. Nepal et al. (2021) identified promoting the development of Nepal’s hydropower potential to provide energy for pumping, where only 1.4 billion cubic meters of groundwater reserves were pumped to irrigate 613 K ha of agricultural lands with a potential economic gain of $1.1 billion annually. This study concluded that a nexus-based approach is required for effective water management, promotion of energy-based industry, food security, and local employment.
Ogbolumani and Nwulu (2022) proposed a meta-model-based EWF nexus system for addressing the issue of natural resource allocation for food and energy security with maximum economic benefit as its objective function. The study determined that utilizing the EWF resources for food production in open fields and undercover greenhouses was more profitable than the production of electrical energy from bio-energy systems. Fetanat et al. (2021) assessed different technologies for energy recovery from wastewater treatment processes, considering energy, water, and food security principles, and concluded that the up-flow anaerobic sludge blanket reactor technology was the most appropriate option. Tan and Yap (2019) investigated the impact of different energy scenarios in Malaysia within the context of the EWF nexus. The key findings of this study included the importance of allowing a reasonable penetration of 20% of renewable energy for the long term, in addition to proper consideration for nuclear energy due to its low running costs and high power density.
2.2 Circular economy and the energy–water–food nexus
Moving toward an EWF nexus approach requires reducing waste, keeping products and materials at the highest value at all times, and extracting all possible by-products from resources. Hence, this approach can be made possible when applying the principle of circular economy, as the two concepts share common aims of resource sustainability and waste minimization (Del Borghi et al., 2020; Al-Thani and Al-Ansari, 2021; Parsa et al., 2021). CE uses theory and principles from industrial ecology; it aims to close the loop of materials and substances to reduce the cross-sectoral environmental impacts of the EWF nexus (Jurgilevich et al., 2016; Del Borghi et al., 2020). Its main principle is to manage and utilize the generated waste of any process and integrate it within the system as this will a) minimize the environmental impacts and reduce pollution (Ferronato et al., 2019), b) keep the products and materials in use (Stahel, 2013), c) secure the availability of natural resources (Khajuria et al., 2022), and d) improve resilience through diversity (Jurgilevich et al., 2016). CE can be applied to water through the reclamation of water in wastewater treatment plants for reuse within irrigation for food production, industrial processes, groundwater recharge, and potable water supply (Sgroi et al., 2018; Smol et al., 2020). It also applies to the food sector through the redistribution of surplus edible food to people in need and the utilization of the inedible food as a feedstock for other processes (i.e., organic fertilizers) (Stabnikova et al., 2005).
Many researchers have highlighted the importance of the linkages between the CE and EWF nexus. Brandoni & Bo njakovic (2018) indicated that a CE framework can support interlinkages between energy, water, and food resources within the European Union (EU). Paiho et al. (2020) denoted that in a circular city, a nexus approach can identify the synergies and trade-offs amongst diverse urbanization challenges by providing a broader and more holistic analysis. D’Odorico et al. (2018) conceptualized that for enhancing the resilience of global water, energy, and food security, it is critical to analyze the interlinkages of the EWF nexus in a CE paradigm. Laso et al. (2018) indicated that circularity without nexus thinking does not necessarily propose the best possible options for environment and natural resource management; thus, the application of CE needs a deeper analysis from the nexus approach. Slorach et al. (2020) quantified the impact of four treatment options on resource recovery from household food waste within the context of the CE and EWF nexus. The results showed that anaerobic digestion is environmentally the most sustainable option with the lowest overall impact on the nexus, while in-vessel composting is the worst option overall. Rodias et al. (2021) summarized the benefits of reusing resources on the EWF nexus; this includes natural resource savings, reductions in GHG emissions due to the production of synthetic fertilizers, and reductions in fossil fuel use. Haitsma Mulier et al. (2022) identified the interactions and interdependencies in the energy–water–nutrients–food nexus to formulate options for circularity in urban farming, where the results indicated that the reuse of resources in urban waste has the potential to reduce stormwater nuisances, energy needs for water, nutrients, and food transport, irrigation, and wastewater pumping.
2.3 Biomass and the energy–water–food nexus
Biomass, which is the organic matter derived from plants and animals, accounts for 9.4% of the global energy supply and 10% of chemical feedstock (IEA, 2020). Nowadays, biomass is the only large-scale organic carbon source that can be a source of organic fuels and chemicals (Deng et al., 2022). It is considered a renewable energy and carbon-neutral source with the highest potential to contribute to the energy needs of modern society (Seow et al., 2022).
The modern industry uses technologies that require high energy consumption. However, this rapid and dependent consumption of petroleum resources has led to an increase in the concentration of GHG in the atmosphere (Ke et al., 2022). Deployment of alternative energy resources, including biomass, is highly favored as it has widely contributed to attaining sustainability in the EWF sectors. Zheng et al. (2022) proposed the co-optimization of cropland distribution and biomass utilization pathways based on the impacts of water shortage and the implementation of carbon prices; the obtained results showed that corn and wheat are the primary crops, and for economic purposes, bioethanol production was advised. Fouladi et al. (2021) developed a systematic approach for the integration of industrial parks across the EWF nexus in which wastewater and biomass were integrated. In this study, the optimized scenario resulted in a 30% reduction in the total GWP with an exergy efficiency of 28% at a 3% cost increase within the economic objective. AlNouss et al. (2019) analyzed the utilization strategy of biomass feedstock in gasification for the poly-generation of different products from an EWF nexus perspective. The results demonstrated that urea production and power generation are the most viable utilization techniques that maximize sustainability indices for EWF resources. An EWF nexus tool was developed by Al-Ansari et al. (2015, 2017) to evaluate the different pathways for a hypothetical 40% food self-sufficiency in Qatar. The sub-systems in this study consisted of a biomass-integrated gasification combined cycle and a carbon capture sub-system. This integration resulted in a 7.8 × 107 kg/year reduction in natural gas consumption, and the maximum achievable negative emission was 1.09 × 109 kg CO2-eq/year.
Different types of biomass—including camel manure, date pits, sewage sludge, and coffee waste—were used in several studies. Camel manure is the organic camel waste that can be found in hot and arid regions, and it was found to contribute to 18% of GHG emissions (Parthasarathy et al., 2023). However, it is mainly composed of protein, lignocellulose, lipid, and inorganic ash content; it can also be utilized as an organic fertilizer due to being rich in nutrients, such as nitrogen, potassium, and phosphorus (Parthasarathy et al., 2021; Liu et al., 2023). Al-Rumaihi et al. (2021) compared the pyrolysis degradation characteristics of camel dung waste with its gasification decomposition behavior; the results showed that Gibbs free energy and enthalpy values of the pyrolysis degradation were lower than in the case of the gasification decomposition. Date pits are hard-coated seeds, ventrally grooved with a small embryo, that are mainly found in arid regions. They represent a vital biomass resource due to their low ash and high carbon contents (Al-Rumaihi, Alherbawi, et al., 2022). As a byproduct of the date processing industry, date pits are frequently abundant and easily accessible locally in Qatar. Due to this, obtaining this type of biomass can have less of an impact on the environment and on transportation expenses. Ouedrhiri et al. (2022) investigated the adsorption performance of methyl orange dye from wastewater by using activated carbon derived from date pits, which resulted in a maximum adsorption capacity of 334 mg/g of methyl orange dye. Sewage sludge is the residue resulting from the treatment of wastewater released from various sources, such as homes and medical facilities (Zuloaga et al., 2012). Converting this type of waste to biochar can reduce the burden on landfills and incineration in Qatar, as significant amounts of sewage sludge are produced as a result of the wastewater treatment processes locally. Moreover, utilizing this locally available resource can reduce transportation expenses and the dependency on importing other types of biomass, which promotes sustainable resource utilization. K. Li et al. (2022) derived biochar from feedstock mixtures, where each was made from different ratios of pine sawdust and sewage sludge. This biochar was used for the adsorption of CO2 gas to mitigate GHG emissions. Coffee is one of the most important traded agricultural products and the most consumable beverage worldwide (Campos-Vega et al., 2015). Its waste is produced as a result of brewing coffee (spent coffee grounds). This waste contains valuable organic materials such as lipids, amino acids, polysaccharides, minerals, and other valuable materials. Therefore, its direct disposal at landfill sites can create ecotoxicological and environmental problems (Atabani et al., 2022). Ahn et al. (2021) evaluated the removal of formaldehyde (an air pollutant) in indoor environments by the co-pyrolysis of spent coffee waste with acid mine drainage sludge. The results showed that the addition of coffee waste increased the removal of formaldehyde by 18.4-fold higher than before using it.
2.4 Biochar
Biochar is the solid product of biomass pyrolysis (Weber and Quicker, 2018). It has received increasing attention due to its unique features, such as its high carbon content and cation exchange capacity, large specific surface area, and stable structure (S. Li and Skelly, 2023; Lu et al., 2023; Wang and Wang, 2019). These features helped it to be widely used for the generation of heat and power, as a good additive to soil as fertilizer and carbon sequestration agent, and for adsorption applications in the form of activated carbon (Amin et al., 2016; Yang et al., 2023). Biochar has been recently used as a modern and sustainable solution to achieve lower environmental impacts and lower GHG emissions by being utilized in different applications (Parthasarathy, Sajjad, et al., 2022; Al-Rumaihi et al., 2023; Liberati et al., 2023), as discussed in the following.
2.4.1 Biochar-based fertilizers
Chemical fertilizers are substances added to the soil to increase crop productivity due to the presence of essential nutrients (including nitrogen, phosphorous, and potassium) that are required by plants. Therefore, fertilizers have been widely used in conventional agricultural systems (Naher et al., 2018). For example, the total annual quantity of CF applied increased from 1.82 × 108 tons in 2002 to 2.45 × 108 tons in 2017, and correspondingly, the total annual amount of grain yield increased from 76.7 × 108 tons to 11.0 × 109 tons during the same period (FAOSTAT, 2020). However, this increase in CF utilization intensity leads to a series of environmental burdens due to the high carbon and water footprint, high non-renewable resource input, and high cost of production (Hasler et al., 2015; Jiang et al., 2021; S. Li et al., 2020). A study was conducted by Guo et al. (2021) using the LCA tool to analyze the environmental benefits under optimized fertilizer and pesticide inputs (i.e., reduction of 24.7% nitrogen, 35.6% phosphorus pentoxide, 18.8% potassium oxide, 17.1% organic fertilizer, and 30.9% pesticides) compared to traditional farmer inputs. The results illustrated that the optimized inputs reduced energy depletion by 24.7%, water depletion by 6.4%, and global warming by 28.8%. Today, biochar-based fertilizers have been widely used to replace CF due to several advantages: i) improves soil structure and its sorption capacity, ii) enhances soil nutrient retention and water-holding capacity, iii) immobilizes contaminants from soil (sorption), and iv) reduces GHG emissions and soil nutrient leaching losses while stimulating the growth of a plant (Rombel et al., 2022; Samoraj et al., 2022). In an investigation conducted by Mikos-Szymańska et al. (2019), biochar and brown coal were used as raw materials for fertilizer production, whereas BC was used for the coating purposes of the urea superphosphate granules. This application had a positive impact on the grain yield per plant of spring wheat, with an increase of 55% compared to when applying nitrogen–phosphorus–potassium (NPK) fertilizers. A meta-analysis conducted by Bai et al. (2022) to investigate the effect of applying biochar and the co-application of biochar with inorganic fertilizers on crop yield showed an increase of 25.3% and 35.3%, respectively. Pradhan et al. (2020) assessed the conversion of four different vegetable wastes (cauliflower, cabbage, banana peels, and corn cob residues) into BC to be used for soil amendment. The best feedstocks determined were cauliflower and banana peels, which produced biochar at an optimum temperature of 400 °C for pyrolysis. This study demonstrates that pyrolysis of dried vegetable wastes is a suitable waste valorization approach to producing biochar with good agricultural properties.
2.4.2 Biochar-based adsorbents
Water contamination is a global problem that threatens the environment and human life and ultimately contributes to water scarcity. Clean water and sanitation is one of the United Nations SDGs that needs to be achieved by 2030 (Wennersten and Qie, 2018; Sun et al., 2021), and therefore, new technologies continue to be developed to provide clean water. Adsorption technology has received increased attention for the removal of organic pollutants and contaminants from wastewater (Anderson et al., 2022). Activated carbon (AC) adsorbents and biochar-based adsorbents have been widely used for wastewater treatment applications due to their high adsorption capacity of contaminants (X. Huang et al., 2018). Chung et al. (2022) used AC derived from sucrose, melamine, and urea for the removal of methylene blue from wastewater; the adsorption capacity of methylene blue reached a maximum of 454.57 mg/g with an adsorption cost of 4.42$/g. AC is extensively used not only for removing pollutants from wastewater streams but also for adsorbing contaminants from drinking water sources, e.g., groundwater, rivers, lakes, and reservoirs. However, the widespread use of AC is restricted due to its high cost and high energy consumption (Thompson et al., 2016; Crini et al., 2019). Malik (2004) developed an effective carbon adsorbent from mahogany sawdust for the removal of dyes from wastewater; the results indicated that the resultant adsorbent had a lower cost compared to the commercial AC adsorbent. Biochar technology represents a new, cost-effective, and environmentally friendly solution for WWT (Xiang et al., 2020). An investigation conducted by Patra et al. (2017) indicated that using BC under optimum conditions removed 99% of cadmium (Cd), lead (Pb), and zinc (Zn) from wastewater.
To the best of our knowledge, many papers have focused on investigating sustainable methods for biochar production. However, there is a gap in investigating the optimum and sustainable biochar production and utilization pathway in the context of the EWF nexus. As such, this study investigates various types of biomass used to produce biochar, namely, camel manure, date pits, sewage sludge, and coffee waste, including determining the optimal blending ratio when using the resultant biochar for either WWT, SA, or both. This helps ensure the highest energy, water, cost savings, and carbon emission reductions. The overall scope of the study is illustrated in Figure 1, where its main objective is to investigate the most optimal utilization option of biochar prepared from the best optimal biomass mix that includes camel manure, date pits, sewage sludge, and coffee waste. The aforementioned wastes, which are rich in nutrients, were selected because of their abundance in hyper-arid regions, which include countries in the Gulf Cooperation Council (GCC) such as Qatar. These end-use utilization options will include WWT only, SA only, and the application of the two pathways. First, a comparison is conducted between the application of BC and NPK fertilizer to the soil, as the energy, water, cost, and emissions required for the production and application were collected from the literature and used to find the savings when applying BC.
Then, AC and BC are evaluated as adsorbents for WWT application in terms of energy, water, cost, and emission savings when considering both their production and application. These objectives are followed to compare the two end-use applications and the different biomass used in the preparation of BC in order to subsequently determine the optimal solution.
3 Methodology
In this study, the biomass blend prepared from camel manure, date pits, sewage sludge, and date pits is pyrolyzed, as illustrated in Figure 2. The rationale for favoring pyrolysis over other treatment processes, such as gasification, is due to the high BC yield produced, which is the main product that is required in this study to be assessed for both SA and WWT pathways. The methodology adopted in this study consists of three steps and is described in the following.
3.1 Biochar production
The product distribution of the pyrolysis process based on multiple biomass sources and the char’s biogenic carbon composition are estimated using the empirical prediction model of Neves et al. (2011). However, the process energy requirement is estimated according to literature reports (Cheng et al., 2020). The former model is utilized to evaluate the production of biochar using four different types of biomass: camel manure, date pits, sewage sludge, and coffee waste. The aforementioned biomass feedstocks are selected due to their local availability in the GCC region. The process is conducted at an operating temperature of 300 °C, a drying efficiency of 90%, a 5L/min.tonne nitrogen flowrate, and a reaction time of 30 min. The elemental analysis for each biomass is collected from the literature, as detailed in Table 1, where the analysis is on a dry ash and sulfur-free basis. Meanwhile, the system’s economics based on multiple feedstocks are evaluated considering Qatar’s case study. The key operating and equipment costs are derived from technical reports (Wright et al., 2010; Humbird et al., 2011) and then scaled up and inflated to the base year of analysis (2019) according to the Chemical Engineering Plant Cost Index (CEPCI) using Eqs. 1, 2. On the other hand, the levelized cost of biochar is estimated using Eq. 3.
The prices of camel manure, date pits, sewage sludge, and coffee waste from the literature are 25$/tonne, 15$/tonne, and 88$/tonne, respectively (Marufuzzaman et al., 2015; Kamil et al., 2019; Alherbawi et al., 2021). However, the price of date pits is quoted locally at 100$/tonne.
In addition, a life cycle assessment is conducted as per the ISO 14044 standard (Finkbeiner et al., 2006). The assessment is performed from “cradle to gate,” covering the key biochar production stages from the acquisition of biomass to transportation, plant construction, pre-processing, and finally, the key processing stage “pyrolysis.” The selected impact categories of interest include the global warming potential, energy consumption, and water footprint. The impact categories are evaluated per 1 kg of biochar, which represents the functional unit (FU) of the analysis. Furthermore, GWP is evaluated according to the second assessment report released by the Intergovernmental Panel on Climate Change (IPCC, 1995).
At the acquisition and transportation stage, biomass is assumed to be transported to the biorefinery for a distance of 100 km via heavy diesel trucks. The water consumed at this stage is negligible. However, the emissions released are calculated as a function of transportation distance and biomass weight (Beeson, 2018). On the other hand, the energy consumed is calculated based on the heating value of the consumed diesel (36 MJ/L) throughout the trip.
For the plant’s construction stage, the land area required is estimated according to the refinery siting workbook as a function of the plant’s capacity (Refinery Siting Workbook: Appendices A and B, 1980), whereas the required energy, water, and associated emissions per construction area unit are adapted from the literature (Klufallah et al., 2014; European Commission, 2020). However, the embodied energy, water, and emissions of machinery are neglected.
At the pre-processing and processing stages, which are meant to dry and then pyrolyze biomass, the energy required in the form of heat and electricity and their associated emissions are calculated based on Cheng et al.’s (2020) energy model, assuming that natural gas is used as an energy carrier. Moreover, the emissions released during the processing stage are calculated based on the product distribution, as previously elaborated. In addition, the cooling water per product mass unit is estimated based on a technical report (Jones et al., 2013).
3.2 Pathway utilization analysis
Figure 3 illustrates conceptually the manifestation of BC within the EWF nexus, where it can be used to provide green energy, enhance water retention, and enhance soil properties for agricultural purposes. Simultaneously, all three sectors contribute to the production of BC as water and energy are needed for the production process, whereas the food sector provides its waste to be utilized as a precursor for this process.
The selection of the most sustainable utilization pathway depends on the analysis of each end-use. A comparison is conducted between BC and its substitute in each pathway to evaluate the possible positive outcomes of utilizing BC in terms of energy, water, cost, and GWP. Table 2 represents the variables used in the equations for the calculations of this section.
3.2.1 Pathway 1: wastewater treatment
A comparison is conducted between AC and BC as adsorbents used for the wastewater treatment process. The data collected from the literature showed that the amount of AC adsorbent used to treat 1 m3 of wastewater is equal to 0.076 kg; this value was used in the equations as the adsorbent requirement (Jeswani et al., 2015). An amount of 1.12 kg BC is found to be equivalent to 1 kg AC (Jjagwe et al., 2021) when considering the adsorption capacity of Pb2+ ions as a reference. The equations in Table 3 present the total requirements of the different variables when a) applying 100% AC and b) reducing the application of AC with an increase in the application of BC. The data from the literature showed that high amounts of carbon emissions are released when producing AC from sewage sludge, with a value equal to 11.096 kg CO2-eq for each kg of AC produced (C. Huang et al., 2022). Alternatively, relatively equal amounts are released when applying AC and BC for treating 1 m3 of wastewater, equal to 0.399 kg CO2-eq/m3 and 0.318 kg CO2-eq/m3 (Jeswani et al., 2015), respectively. In addition, 2.57$ and 167.63 MJ are required to produce each 1 kg of AC adsorbent, which are relatively high compared to the cost and energy needed to produce biochar that are provided in Table 7; the energy needed to apply the AC adsorbent is 5.009 MJ/m3. However, for the BC adsorbent, it was found to be equal to 0.36 MJ/m3 (Jeswani et al., 2015; Rayburn, 2012). Notably, the water requirements for AC production are much lower than the one for BC, which is approximately 0.202 m3/kg AC (C. Huang et al., 2022), where it is only needed for washing. The data are inserted into the requirement equations (eqs. 4–7), and then, the mean is calculated and used to determine the savings when applying each different biomass.
3.2.2 Pathway 2: soil amendment
In this pathway, the application of biochar for soil enhancement is evaluated based on the comparison with NPK fertilizer application. To achieve this objective, tomato is selected as the reference crop, where it is found that the amount of NPK fertilizer needed to grow tomato per each m2 is equal to 0.05 kg/m2, and whilst applying it, the water requirement is found to be equivalent to approximately 1.5 m3/m2 (Ziane et al., 2021). The data from the literature indicate that the GWP when producing and applying NPK fertilizer is much higher than when using BC, with values equal to 0.2 (Walling and Vaneeckhaute, 2020), 0.069 (Brentrup and Palliere, 2008), and 0.024 kg CO2-eq/m2 (Majumder et al., 2019), respectively. It requires 0.8$/kg, 7.7 × 10−3 m3/kg, and 12.54 MJ/kg to produce NPK fertilizer (Gellings and Parmenter, 2004; Wu et al., 2021; Samoraj et al., 2022); these values are greater compared to the cost, water, and energy required to produce BC, as detailed in Table 7. Meanwhile, for the application segment, water and energy are found to be slightly lower when using biochar of approximately 0.545 m3/m2 and 0.434 MJ/m2 than when using NPK of values equal to 0.771 m3/m2 and 0.615 MJ/m2 (Gellings and Parmenter, 2004; Ji et al., 2020; Jiang et al., 2021). The data are then inserted into the equations presented in Table 4, where a reduction in fertilizer usage is considered. C1, E1, W1, and GWP1 are calculated when considering the 100% application of NPK fertilizer only. Subsequently, C2, E2, W2, and GWP2 represent the utilization of BC combined with a reduction in the utilization of the fertilizer. Finally, the savings are calculated and used for the optimization component.
3.3 Optimization
Based on the analysis conducted in the previous sections, a multi-objective mathematical optimization is performed using the genetic algorithm tool within MATLAB. This optimization considers the following objectives: a) maximum GWP reduction, b) maximum cost saving, c) maximum water saving, and d) maximum energy saving. The equations used to achieve these objectives are presented in Table 5, in addition to the constraints that are adopted to provide equal relative weights to the four objectives.
Genetic algorithms are heuristic adaptive search techniques based on the mechanisms of natural selection and natural genetics (Kanyilmaz et al., 2022). These algorithms aim to explore part of the set of possible solutions to determine the most optimal solution by selecting a narrower set of solutions at each step and using them as parents to produce the following solutions (Alherbawi et al., 2021; Estran et al., 2022). First, thousands of solutions are initially generated randomly as individuals, and the fitness level of each solution is calculated. Then, a second generation is obtained using two operators for generating the offspring population: crossover and mutation. The crossover operator combines two selected solutions to derive a new solution that is close to both parent solutions, while the mutation operator alters the existing solutions to create new solutions. This process is repeated until the optimal solutions meet the acquired objectives. This multi-objective optimization was conducted in two stages. The first relates to the biomass mix when treating each pathway individually fulfilling the four objectives at once. On the other hand, the other stage is more broadly focused on the most optimal mix of biomass for multi-end use, providing a broader insight to decision makers. The second stage is conducted based on seven scenarios, where each stage is concerned with two or more objectives, as detailed in Table 6.
4 Results and discussion
For each step followed in the methodology, the results are collected and discussed in the following subsections.
4.1 Biochar production
The biochar yield, as shown in Figure 4, is the highest for date pits and the lowest for sewage sludge with values equal to 43% and 6%, respectively. BC produced, composed of 67.9% carbon, 4.5% hydrogen, and 27.6% oxygen, has a heating value equal to 24.54 MJ/kg and the empirical formula C H0.79 O0.30 for all biomasses. The total electricity required to produce biochar is 1.824, 1.731, 3.614, and 1.820 MJ/kg when using camel manure, date pits, sewage sludge, and coffee waste, respectively. GWP, cost, water footprint, and energy footprint are quantified for each type of biomass, as detailed in Table 7. The resulting data illustrate that despite the fact that sewage sludge has the lowest water footprint of 0.063 L/kg biochar, it is highest in terms of highest carbon emissions, cost, and energy footprint, whilst coffee waste has the lowest values and is the most sustainable biomass to be used for BC production.
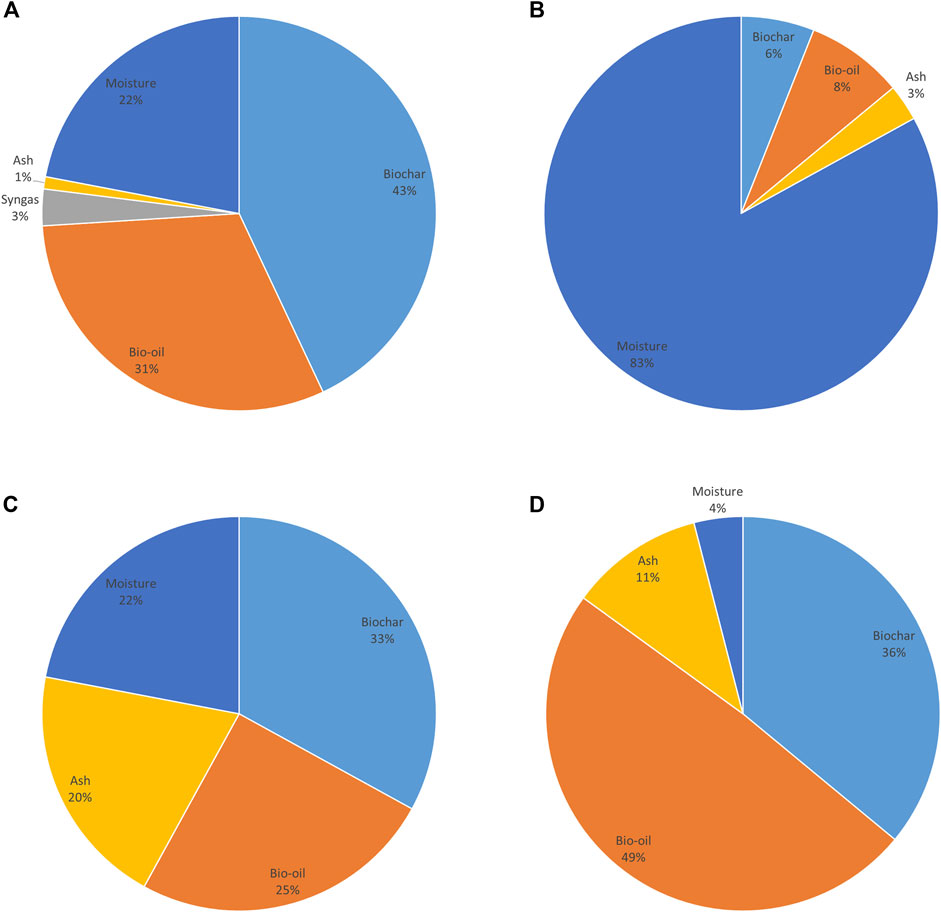
FIGURE 4. Product distribution: (A) date pits, (B) sewage sludge, (C) camel manure, and (D) coffee waste.

TABLE 7. GWP, cost, water footprint, and energy footprint for different biomass feedstocks, where the functional unit is per 1 kg of biochar.
4.2 Pathway utilization analysis
When evaluating the changes to energy and GWP with the increased reduction in AC adsorbent requirements, it is found to be inversely proportional equivalent for all types of biomass. As the amount of AC reduced and the amount of BC increased, the energy required and carbon emissions released associated with the WWT pathway decreased, as shown in Figure 5 and detailed in Table 8. Despite the fact that utilizing biochar from camel manure, date pits, and coffee waste for WWT is more cost effective, sewage sludge-based biochar adsorbents are found to be more expensive relative to AC adsorbents. Notably, the utilization of biochar adsorbent obtained from sewage sludge is a more sustainable option to treat wastewater than AC adsorbent from a water-saving perspective.
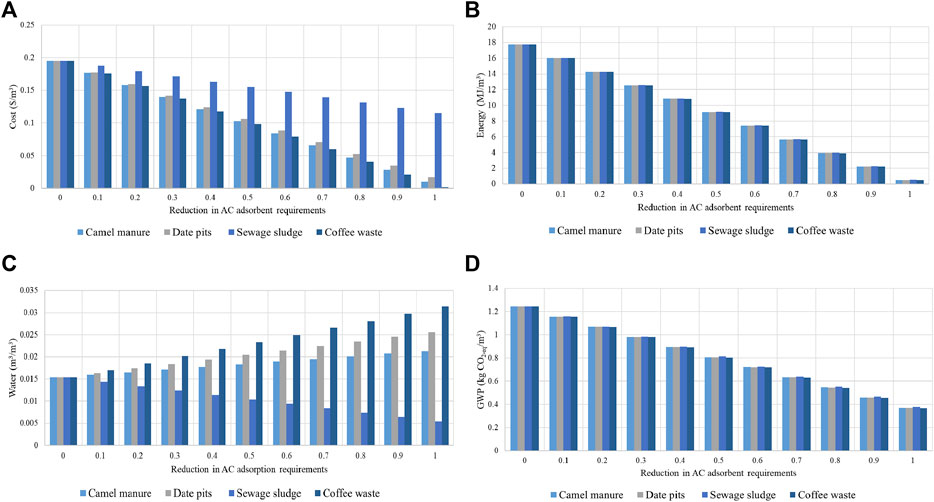
FIGURE 5. Biochar utilization analysis for the wastewater treatment pathway: (A) cost, (B) energy, (C) water, and (D) GWP.
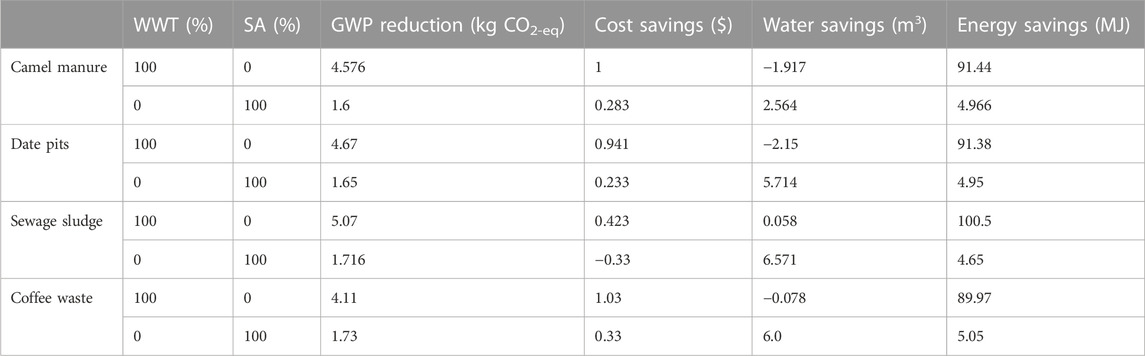
TABLE 8. GWP, cost, water, and energy savings when utilizing biochar from each biomass for each pathway, where FU is per 1 kg of biochar.
The results, as shown in Figure 6 and detailed in Table 8, for the soil amendment pathway conclude that substituting NPK fertilizer with biochar-based fertilizer is a more sustainable option for the four different types of biomass. This comes as a result of reducing energy and water consumption, carbon emissions, and costs. Utilizing NPK fertilizer can only be considered a sustainable choice when comparing it to using sewage sludge-based biochar for soil from a cost perspective.
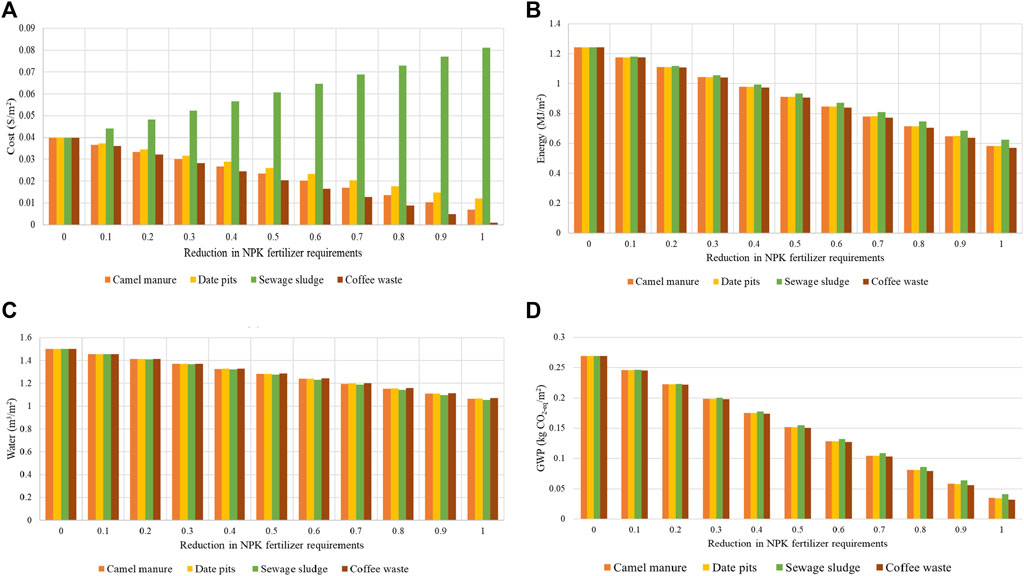
FIGURE 6. Biochar utilization analysis for the soil amendment pathway: (A) cost, (B) energy, (C) water, and (D) GWP.
4.3 Optimization
As it is a multi-objective optimization, it was impossible to compute a single solution as there are contradicting objectives that cannot be ideally and collectively fulfilled in a single solution. Thus, the genetic algorithm tool performs a trade-off between the four different objectives, and therefore, 18 and 70 solutions are obtained in single- and multi-end use stages, respectively.
4.3.1 Single-end use
For the WWT pathway, optimal solutions are mainly considered due to the high energy savings achieved by utilizing BC adsorbents and the increased reduction in GHG emissions, as shown in Figure 7.
The use of a BC adsorbent was not a good option from a water-saving point of view. The biomass mix varied for each solution, favoring the sewage sludge-based BC adsorbents and a lower composition of other biomass. One of the solutions was considered an optimal mix of 3% camel manure, 8% date pits, 77% sewage sludge, and 12% coffee waste. On the other hand, another solution considered 7% camel manure, 0% date pits, 39% sewage sludge, and 54% coffee waste as an optimal alternative mix.
For the SA pathway, the results, as displayed in Figure 8, illustrate that all 18 solutions agreed on an optimal mix of biomass, where mostly coffee waste is utilized and no sewage sludge is to be used. This mix consists of approximately 95% coffee waste, 4% date pits, 1% camel manure, and 0% sewage sludge. When the model selects optimal solutions based on multiple objectives, it initially investigates the inputs that satisfy each objective separately and tries to perform a trade-off between the different objectives. For the WWT pathway, most of the solutions are leaned toward sewage sludge-based adsorbent, as it contributes to the lowest water footprint due to its high moisture content. This is why it has been suggested to be part of the blend to satisfy the objective of minimizing the water footprint. On the other hand, the optimal biomass blend suggested for the SA pathway consisted of high proportions of coffee waste as it contributes to the lowest cost, energy, and carbon footprint. Another reason could be its high carbon content, which accounts for 79.3% of its content when being compared to other biomass.
4.3.2 Multi-end use
When considering the two different end-uses of BC, more optimal solutions are collectively obtained. Approximately 70 solutions are collected for each scenario, as illustrated in Figure 9, Figure 10, Figure 11, Figure 12, Figure 13, Figure 14, excluding scenario 3, which was concerned with achieving the maximum energy and emission savings resulted in one single optimal solution. Some of the solutions obtained were as follows:
(a) Scenario 1 (four objectives: maximize water, energy, cost, and emission savings)
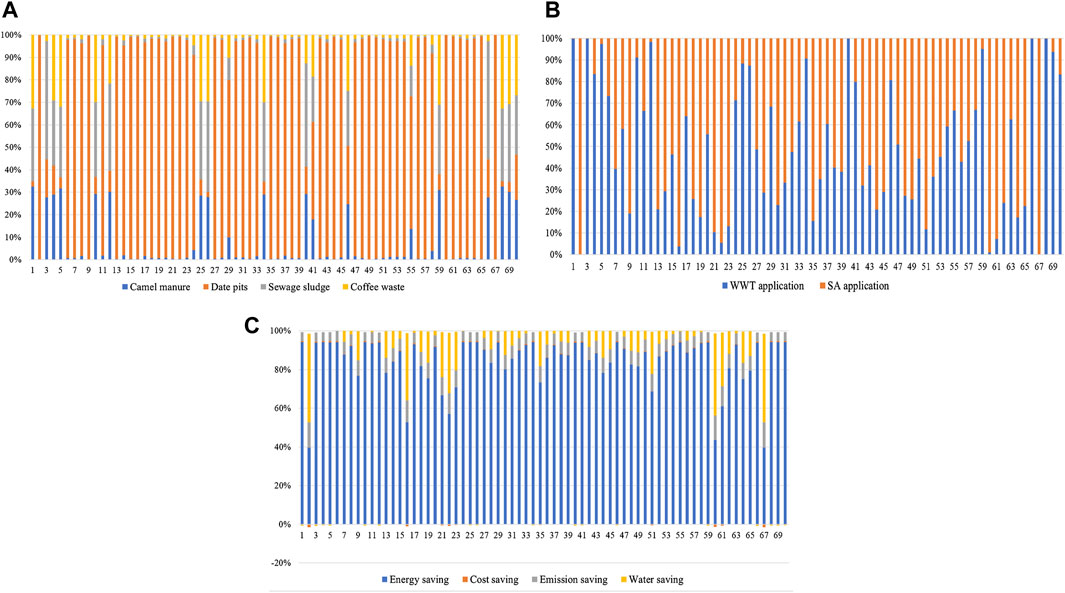
FIGURE 9. Scenario 1—maximum energy, water, emission, and cost savings for (A) optimal biomass blend, (B) optimal biochar end-use mix, and (C) optimal solutions of the scenario. (C) Scenario 2 (two objectives: maximize energy and cost savings).
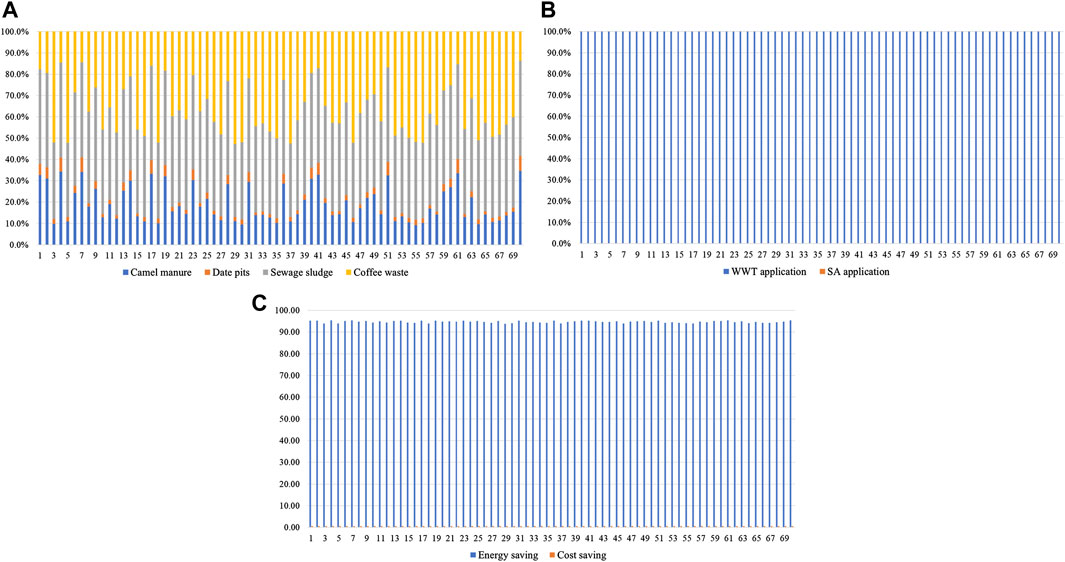
FIGURE 10. Scenario 2—maximum energy and cost savings for (A) optimal biomass blend, (B) optimal biochar end-use mix, and (C) optimal solutions of the scenario.
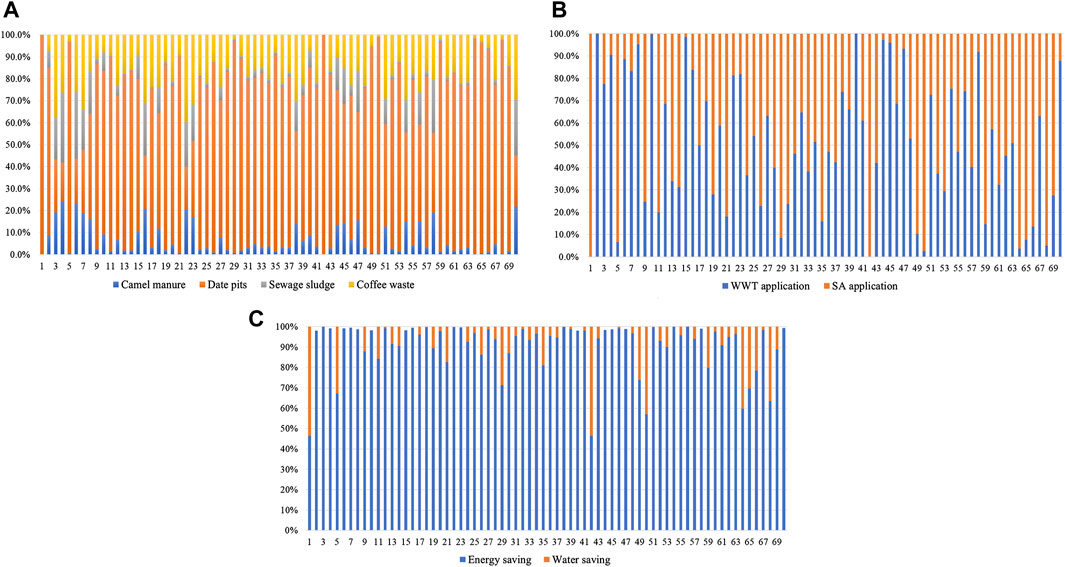
FIGURE 11. Scenario 4—maximum energy and water savings for (A) optimal biomass blend, (B) optimal biochar end-use mix, and (C) optimal solutions of the scenario.
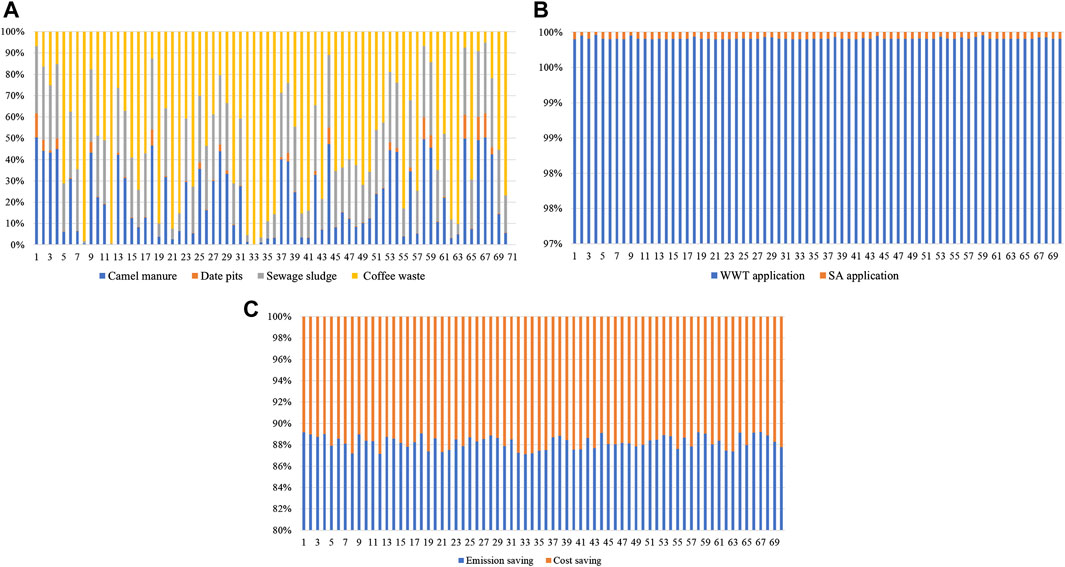
FIGURE 12. Scenario 5—maximum emission and cost savings for (A) optimal biomass blend, (B) optimal biochar end-use mix, and (C) optimal solutions of the scenario.
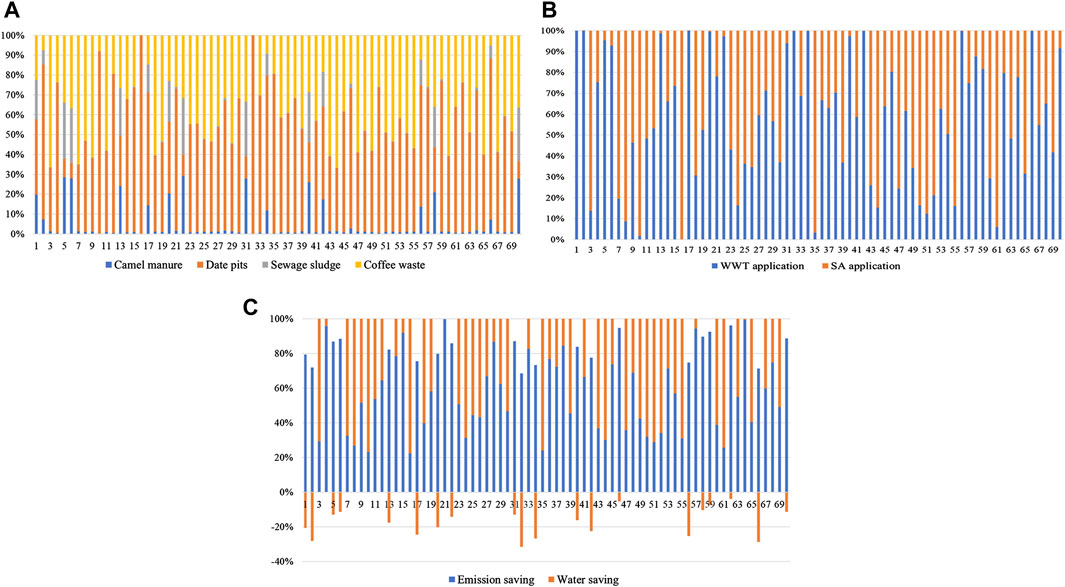
FIGURE 13. Scenario 6—maximum emission and water saving for (A) optimal biomass blend, (B) optimal biochar end-use mix, and (C) optimal solutions of the scenario.
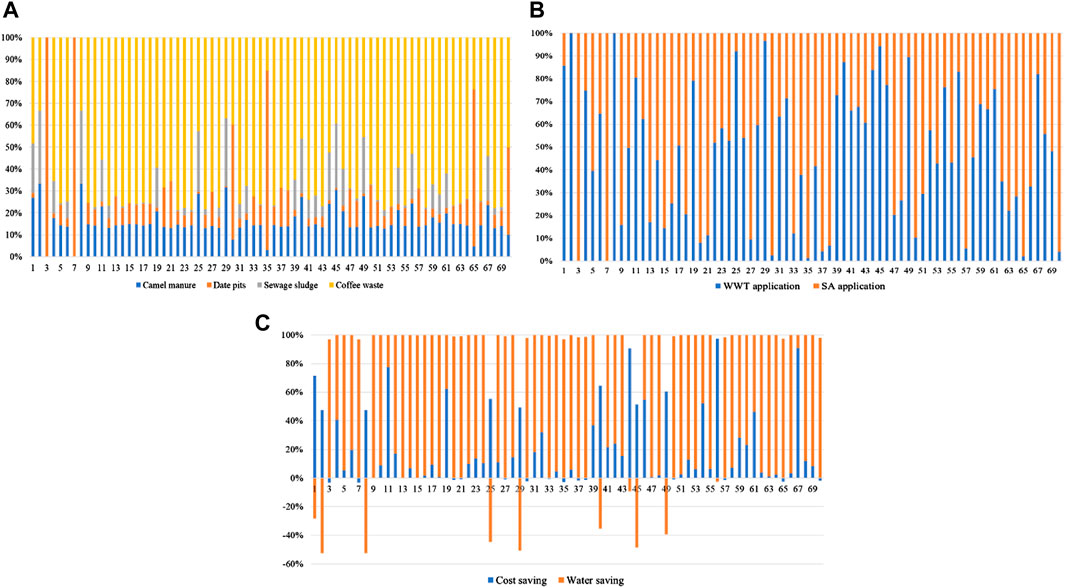
FIGURE 14. Scenario 7—maximum cost and water savings for (A) optimal biomass blend, (B) optimal biochar end-use mix, and (C) optimal solutions of the scenario.
The end-use share varied randomly; however, with this variation, there are specific ratios for each biomass required in the blend. Most of the solutions, as shown in Figure 9, that lean toward the soil application pathway have very high proportions of date pits in the mix within the range 97%–99% with relatively smaller proportions of the other biomass of approximately 0.1%–1%. On the other hand, the other solutions that lean toward the WWT pathway suggested that the optimum biomass mix should consist of relatively equal proportions of camel manure, sewage sludge, and coffee waste of approximately 29%–33% and smaller amounts of date pits of approximately 2%–5%. One of the proposed solution involved using a biomass blend consisting of 0.1% camel manure, 99.5% date pits, 0.1% sewage sludge, and 0.3% coffee waste to produce biochar that will be utilized with 19% serving as an adsorbent for WWT and the remaining 81% as a fertilizer for soil. Another optimal solution suggested using a biomass blend composed of 31.7% camel manure, 4.9% date pits, 31.6% sewage sludge, and 31.9% coffee waste to prepare biochar that will be used, with 97.5% serving for WWT application and 2.6% for soil application.
(b) Scenario 2 (two objectives: maximize energy and cost savings)
When only considering maximum energy and cost savings, biochar should be exclusively used as an adsorbent for wastewater treatment applications, as shown in Figure 10. Most of the optimal solutions agreed on utilizing sewage sludge in the highest ratio, approximately 45%, followed by coffee waste, camel manure, and date pits, respectively. One of the solutions that proposed 100% utilization toward WWT suggested the proportions as follows: 19.6% camel manure, 2.2% date pits, 43.4% sewage sludge, and 34.9% coffee waste.
(c) Scenario 3 (two objectives: maximize energy and emission savings)
To obtain the highest energy and emission savings, the most optimal biomass blend consists of 6.1% camel manure, 13.8% date pits, 66% sewage sludge, and 14.2% coffee waste. This blend should be utilized as approximately 100% for WWT application and only 0.1% as a fertilizer for soil.
(d) Scenario 4 (two objectives: maximize energy and water savings)
No specified end-use is favored in this scenario. However, when considering 50% utilization of biochar in each pathway, the highest proportion to be utilized is 72.5% of date pits, followed by 23.7% of coffee waste, 3.15% of camel manure, and the least, which is 0.7% of sewage sludge, as shown in Figure 11.
(e) Scenario 5 (two objectives: maximize emissions and cost savings)
Achieving the highest emission and cost savings requires the utilization of 100% biochar in WWT applications, as shown in Figure 12, where the biomass blend mix varies between camel manure, sewage sludge, and coffee waste as the constituents of the highest proportion, although it was agreed that the least amount to be used is of date pits. One of the solutions suggested the utilization of approximately 29.5% each of camel manure and sewage sludge, 41% of coffee waste, and 0.5% date pits.
(f) Scenario 6 (two objectives: maximize emission and water savings)
For the different proportions of the end-use mix, the changes in the proportion of biomass varied continuously and randomly, as shown in Figure 13. One of the solutions proposed utilizing 0.9% camel manure, 54.2% date pits, 0.2% sewage sludge, and 44.8% coffee waste when applying biochar, with 43.15% for WWT and 57% for SA.
(g) Scenario 7 (two objectives: maximize cost and water savings)
Considering the highest cost and water savings, the highest proportion of biomass mix is suggested to be coffee waste as it guarantees achieving the objectives. As Figure 14 illustrates, one of the optimal solutions proposed using a biomass blend of 13.2% camel manure, 4.3% date pits, 5.9% sewage sludge, and 76.7% coffee waste to produce biochar that will be utilized, with 62.3% serving as an adsorbent for WWT and 37.8% as a fertilizer for soil.
5 Conclusion
The deployment of biomass as an alternative energy resource has been widely used as a modern solution for providing green energy and reducing dependence on non-renewable resources. Pyrolysis is one of the processes concerned with the conversion of biomass into energy value-added products, including biochar. BC has been utilized in many applications within the context of the EWF nexus; it can provide a source of energy replacing fossil fuels, can be used as fertilizer for soil applications, and can also be used to treat wastewater and enhance water retention.
This study has provided insights into the end-use sustainable solutions in the use of biochar from an energy, water, and food perspective. It also investigated optimized methods for end-use utilization options, considering the most optimum biomass mix for wastewater treatment and soil amendment applications, using a structured approach consisting of a literature review, model simulation, and multi-objective optimization using the genetic algorithm tool in MATLAB. The results of this study are acquired in two different stages, where 18 solutions are collected for the single-end use stage, considering the four objectives: maximum emission reduction and maximum energy, water, and cost savings. When considering the WWT pathway alone, the biomass mix leaned toward favoring the sewage sludge-based biochar adsorbents, with a lower composition of other biomass. However, if 100% of biochar was planned to be used as fertilizer for soil, the optimal mix proposed should consist of approximately 95% coffee waste, 4% date pits, 1% camel manure, and 0% sewage sludge. For the second stage for multi-end use, around 70 optimal solutions are obtained for each scenario except for scenario three, which resulted in only one solution. In the first scenario, the four objectives were achieved: maximum emission reduction and maximum energy, water, and cost savings. The optimal solutions that favored utilizing BC for soil application require a higher ratio of date pits to the other biomass of an approximate proportion of 98%, while the solutions that leaned toward wastewater treatment application favor a relatively equal amount of camel manure, sewage sludge, and coffee waste (29%–33%) but a smaller amount of date pits of approximately 2%–5%.
This study is novel as it provides sustainable solutions (environment and economic perspectives) for decision makers and researchers to utilize biochar depending on the objectives they desire to achieve as a function of the type of biomass. Furthermore, there are few limitations to the study to be considered for future work.
i. This model is biomass specific, so for new biomass, resource-intensive analysis and optimization have to be conducted again. Therefore, a machine learning approach may be employed in future studies to be able to predict the model response whenever a new biomass is introduced without the need for intensive analysis.
ii. The process is studied under fixed operating conditions for all biomass blends, which may not correspond to the optimal conditions in each scenario. Therefore, it is crucial to understand the correlation between biomass composition, blending ratios, and the optimal operating conditions to maximize biochar production.
iii. This model is only concerned with the utilization of biochar produced from the pyrolysis process; future studies can use this model to investigate the sustainable utilization options of the other pyrolysis products, including bio-oil and biogas.
Data availability statement
The original contributions presented in the study are included in the article/Supplementary Materials; further inquiries can be directed to the corresponding author.
Author contributions
FO: conceptualization, methodology, software, validation, formal analysis, investigation, resources, data curation, writing–original draft, and visualization. MA: validation, formal analysis, investigation, resources, data curation, writing–original draft, and visualization. GM: conceptualization, methodology, project administration, supervision, conceptualization, methodology, and writing–review and editing. TA-A: project administration, supervision, conceptualization, methodology, and writing–review and editing. All authors contributed to the article and approved the submitted version.
Acknowledgments
The authors acknowledge the support of the Qatar National Research Fund (QNRF) by proposal number NPRP11S-0107-180216 and Open Access funding provided by the Qatar National Library. The contents herein are solely the responsibility of the authors [s].
Conflict of interest
The authors declare that the research was conducted in the absence of any commercial or financial relationships that could be construed as a potential conflict of interest.
Publisher’s note
All claims expressed in this article are solely those of the authors and do not necessarily represent those of their affiliated organizations, or those of the publisher, the editors, and the reviewers. Any product that may be evaluated in this article, or claim that may be made by its manufacturer, is not guaranteed or endorsed by the publisher.
Abbreviations
EWF, energy–water–food; BC, biochar; WWT, wastewater treatment; SA, soil amendment; FAO, Food and Agriculture Organization; SDG, sustainable development goal; GHG, greenhouse gase; CE, circular economy; CF, chemical fertilizer; LCA, life cycle assessment; GWP, global warming potential; BECCS, bio-energy carbon capture and storage; CO2, carbon dioxide; EU, European Union; FU, functional unit; NPK, nitrogen–phosphorus–potassium; AC, activated carbon; Cd, cadmium; Pb, lead; Zn, zinc; GCC, Gulf Cooperation Council; CEPCI, Chemical Engineering Plant Cost Index.
References
Ahn, Y., Cho, D. W., Ahmad, W., Jo, J., Jurng, J., Kurade, M. B., et al. (2021). Efficient removal of formaldehyde using metal-biochar derived from acid mine drainage sludge and spent coffee waste. J. Environ. Manag. 298, 113468. doi:10.1016/J.JENVMAN.2021.113468
Al-Ansari, T., AlNouss, A., Al-Thani, N., Parthasarathy, P., ElKhalifa, S., Mckay, G., et al. (2020). Computer Aided Chemical Engineering, 48. doi:10.1016/B978-0-12-823377-1.50273-1Optimising multi biomass feedstock utilisation considering a multi technology approach
Al-Ansari, T., Korre, A., Nie, Z., and Shah, N. (2015). Development of a life cycle assessment tool for the assessment of food production systems within the energy, water and food nexus. Sustain. Prod. Consum. 2, 52–66. doi:10.1016/J.SPC.2015.07.005
Al-Ansari, T., Korre, A., Nie, Z., and Shah, N. (2017). Integration of greenhouse gas control technologies within the energy, water and food nexus to enhance the environmental performance of food production systems. J. Clean. Prod. 162, 1592–1606. doi:10.1016/J.JCLEPRO.2017.06.097
Al-Enazi, A., Okonkwo, E. C., Bicer, Y., and Al-Ansari, T. (2021). Energy Reports, 7. doi:10.1016/j.egyr.2021.03.036A review of cleaner alternative fuels for maritime transportation
Al-Rumaihi, A., Alherbawi, M., Mckay, G., Mackey, H., Parthasarathy, P., and Al-Ansari, T. (2023). Assessing plastic and biomass-based biochar’s potential for carbon sequestration: An energy-water-environment approach. Front. Sustain. 4. doi:10.3389/frsus.2023.1200094
Al-Rumaihi, A., Alherbawi, M., Parthasarathy, P., Mackey, H. R., McKay, G., and Al-Ansari, T. (2022). A techno-economic assessment of biochar production from date pits in the MENA region. Comput. Aided Chem. Eng. 51, 805–810. doi:10.1016/B978-0-323-95879-0.50135-1
Al-Rumaihi, A., Parthasarathy, P., Fernandez, A., Al-Ansari, T., Mackey, H. R., Rodriguez, R., et al. (2021). Thermal degradation characteristics and kinetic study of camel manure pyrolysis. J. Environ. Chem. Eng. 9 (5), 106071. doi:10.1016/J.JECE.2021.106071
Al-Rumaihi, A., Shahbaz, M., Mckay, G., Mackey, H., and Al-Ansari, T. (2022). A review of pyrolysis technologies and feedstock: A blending approach for plastic and biomass towards optimum biochar yield. Renew. Sustain. Energy Rev. 167, 112715. doi:10.1016/J.RSER.2022.112715
Al-Thani, N. A., and Al-Ansari, T. (2021). Comparing the convergence and divergence within industrial ecology, circular economy, and the energy-water-food nexus based on resource management objectives. Sustain. Prod. Consum. 27, 1743–1761. doi:10.1016/J.SPC.2021.04.008
Al-Thani, N. A., Govindan, R., and Al-Ansari, T. (2020). Maximising nutritional benefits within the energy, water and food nexus. J. Clean. Prod. 266, 121877. doi:10.1016/j.jclepro.2020.121877
Alherbawi, M., AlNouss, A., McKay, G., and Al-Ansari, T. (2021). Optimum sustainable utilisation of the whole fruit of Jatropha curcas: An energy, water and food nexus approach. Renew. Sustain. Energy Rev. 137, 110605. doi:10.1016/J.RSER.2020.110605
Alherbawi, M., AlNouss, A., Mckay, G., and Al-Ansari, T. (2020). Optimum utilization of jatropha seedcake considering the energy, water and food nexus. Comput. Aided Chem. Eng. 48, 229–234. doi:10.1016/B978-0-12-823377-1.50039-2
Allan, T., Keulertz, M., and Woertz, E. (2015). The water–food–energy nexus: An introduction to nexus concepts and some conceptual and operational problems. Int. J. Water Resour. Dev. 31 (3), 301–311. doi:10.1080/07900627.2015.1029118
Almanassra, I. W., Mckay, G., Kochkodan, V., Ali Atieh, M., and Al-Ansari, T. (2021). A state of the art review on phosphate removal from water by biochars. Chem. Eng. J. 409, 128211. doi:10.1016/J.CEJ.2020.128211
AlNouss, A., Namany, S., McKay, G., and Al-Ansari, T. (2019). Applying a sustainability metric in energy, water and food nexus applications; A biomass utilization case study to improve investment decisions. Comput. Aided Chem. Eng. 46, 205–210. doi:10.1016/B978-0-12-818634-3.50035-7
AlNouss, A., Shahbaz, M., Mckay, G., and Al-Ansari, T. (2022). Bio-methanol production from palm wastes steam gasification with application of CaO for CO2 capture: Techno-economic-environmental analysis. J. Clean. Prod. 341, 130849. doi:10.1016/J.JCLEPRO.2022.130849
Amin, F. R., Huang, Y., He, Y., Zhang, R., Liu, G., and Chen, C. (2016). Biochar applications and modern techniques for characterization. Clean. Technol. Environ. Policy 18 (5), 1457–1473. doi:10.1007/s10098-016-1218-8
Anderson, A., Anbarasu, A., Pasupuleti, R. R., Manigandan, S., Praveenkumar, T. R., and Aravind Kumar, J. (2022). Treatment of heavy metals containing wastewater using biodegradable adsorbents: A review of mechanism and future trends. Chemosphere 295, 133724. doi:10.1016/j.chemosphere.2022.133724
Atabani, A. E., Ali, I., Naqvi, S. R., Badruddin, I. A., Aslam, M., Mahmoud, E., et al. (2022). A state-of-the-art review on spent coffee ground (SCG) pyrolysis for future biorefinery. Chemosphere 286, 131730. doi:10.1016/J.CHEMOSPHERE.2021.131730
Bai, S. H., Omidvar, N., Gallart, M., Kämper, W., Tahmasbian, I., Farrar, M. B., et al. (2022). Combined effects of biochar and fertilizer applications on yield: A review and meta-analysis. Sci. Total Environ. 808, 152073. doi:10.1016/J.SCITOTENV.2021.152073
Bazilian, M., Rogner, H., Howells, M., Hermann, S., Arent, D., Gielen, D., et al. (2011). Considering the energy, water and food nexus: Towards an integrated modelling approach. Energy Policy 39 (12), 7896–7906. doi:10.1016/J.ENPOL.2011.09.039
Bieber, N., Ker, J. H., Wang, X., Triantafyllidis, C., van Dam, K. H., Koppelaar, R. H. E. M., et al. (2018). Sustainable planning of the energy-water-food nexus using decision making tools. Energy Policy 113, 584–607. doi:10.1016/j.enpol.2017.11.037
Bilal, H., Govindan, R., and Al-Ansari, T. (2021). Investigation of groundwater depletion in the state of Qatar and its implication to energy water and food nexus. WaterSwitzerl. 13 (18), 2464. doi:10.3390/w13182464
Bonn 2011 Conference (2012). The water, energy and food security nexus – solutions for a green economy. Policy Recommendations.
Brandoni, C., and Bo njakovic, B. (2018). Energy, food and water nexus in the European Union: Towards a circular economy. Proc. Institution Civ. Eng. Energy 171 (3), 140–144. doi:10.1680/jener.18.00005
Brentrup, F., and Palliere, C. (2008). GHG emissions and energy efficiency in European nitrogen fertiliser production and use. Proc. - Int. Fertil. Soc. 2 (12).
Campi, M., Dueñas, M., and Fagiolo, G. (2021). Specialization in food production affects global food security and food systems sustainability. World Dev. 141, 105411. doi:10.1016/J.WORLDDEV.2021.105411
Campos-Vega, R., Loarca-Piña, G., Vergara-Castañeda, H. A., and Dave Oomah, B. (2015). Spent coffee grounds: A review on current research and future prospects. Trends Food Sci. Technol. 45 (1), 24–36. doi:10.1016/j.tifs.2015.04.012
Cansino-Loeza, B., Tovar-Facio, J., and Ponce-Ortega, J. M. (2021). Stochastic optimization of the water-energy-food nexus in disadvantaged rural communities to achieve the sustainable development goals. Sustain. Prod. Consum. 28, 1249–1261. doi:10.1016/J.SPC.2021.08.005
Cheng, F., Luo, H., and Colosi, L. M. (2020). Slow pyrolysis as a platform for negative emissions technology: An integration of machine learning models, life cycle assessment, and economic analysis. Energy Convers. Manag. 223, 113258. doi:10.1016/j.enconman.2020.113258
Chung, J., Sharma, N., Kim, M., and Yun, K. (2022). Activated carbon derived from sucrose and melamine as low-cost adsorbent with fast adsorption rate for removal of methylene blue in wastewaters. J. Water Process Eng. 47, 102763. doi:10.1016/j.jwpe.2022.102763
Crini, G., Lichtfouse, E., Wilson, L. D., and Morin-Crini, N. (2019). Conventional and non-conventional adsorbents for wastewater treatment. Environ. Chem. Lett. 17 (1), 195–213. doi:10.1007/s10311-018-0786-8
De Laurentiis, V., Hunt, D. V. L., and Rogers, C. D. F. (2016). Overcoming food security challenges within an energy/water/food nexus (EWFN) approach. Sustain. Switz. 8 (1), 95. doi:10.3390/su8010095
Del Borghi, A., Moreschi, L., and Gallo, M. (2020). Circular economy approach to reduce water–energy–food nexus. Curr. Opin. Environ. Sci. Health 13, 23–28. doi:10.1016/J.COESH.2019.10.002
Deng, W., Feng, Y., Fu, J., Guo, H., Guo, Y., Han, B., et al. (2022). Catalytic conversion of lignocellulosic biomass into chemicals and fuels. Green Energy and Environment. doi:10.1016/J.GEE.2022.07.003
D’Odorico, P., Davis, K. F., Rosa, L., Carr, J. A., Chiarelli, D., Dell’Angelo, J., et al. (2018). The global food-energy-water nexus. Rev. Geophys. 56 (3), 456–531. doi:10.1029/2017RG000591
Elkhalifa, S., Al-Ansari, T., Mackey, H. R., and McKay, G. (2019). Food waste to biochars through pyrolysis: A review. Resour. Conservation Recycl. 144, 310–320. doi:10.1016/J.RESCONREC.2019.01.024
Endo, A., Tsurita, I., Burnett, K., and Orencio, P. M. (2017). A review of the current state of research on the water, energy, and food nexus. J. Hydrology Regional Stud. 11, 20–30. doi:10.1016/J.EJRH.2015.11.010
Estran, R., Souchaud, A., and Abitbol, D. (2022). Using a genetic algorithm to optimize an expert credit rating model. Expert Syst. Appl. 203, 117506. doi:10.1016/J.ESWA.2022.117506
Falconer, R. A. (2021). Water security: Why we need global solutions. Engineering 16, 13–15. doi:10.1016/J.ENG.2021.10.009
Faostat, (2022). Food and agriculture organization of the united Nations - statistics division. FAOSTAT. http://www.fao.org/faostat/en/#data/QC (Accessed March 08, 2022).
Ferronato, N., Rada, E. C., Gorritty Portillo, M. A., Cioca, L. I., Ragazzi, M., and Torretta, V. (2019). Introduction of the circular economy within developing regions: A comparative analysis of advantages and opportunities for waste valorization. J. Environ. Manag. 230, 366–378. doi:10.1016/J.JENVMAN.2018.09.095
Fetanat, A., Tayebi, M., and Mofid, H. (2021). Water-energy-food security nexus based selection of energy recovery from wastewater treatment technologies: An extended decision making framework under intuitionistic fuzzy environment. Sustain. Energy Technol. Assessments 43, 100937. doi:10.1016/j.seta.2020.100937
Finkbeiner, M., Inaba, A., Tan, R. B. H., Christiansen, K., and Klüppel, H. J. (2006). The new international standards for life cycle assessment: ISO 14040 and ISO 14044. Int. J. Life Cycle Assess. 11 (2), 80–85. doi:10.1065/lca2006.02.002
Fouladi, J., AlNouss, A., and Al-Ansari, T. (2021). Sustainable energy-water-food nexus integration and optimisation in eco-industrial parks. Comput. Chem. Eng. 146, 107229. doi:10.1016/J.COMPCHEMENG.2021.107229
Gellings, C., and Parmenter, K. (2004). Encyclopedia of life support systems (EOLSS).Energy efficiency in fertilizer production and use
Ghiat, I., and Al-Ansari, T. (2021). A review of carbon capture and utilisation as a CO2 abatement opportunity within the EWF nexus. J. CO2 Util. 45, 101432. doi:10.1016/J.JCOU.2020.101432
Ghiat, I., Mahmood, F., Govindan, R., and Al-Ansari, T. (2021). CO2 utilisation in agricultural greenhouses: A novel ‘plant to plant’ approach driven by bioenergy with carbon capture systems within the energy, water and food nexus. Energy Convers. Manag. 228, 113668. doi:10.1016/J.ENCONMAN.2020.113668
Govindan, R., and Al-Ansari, T. (2019). Computational decision framework for enhancing resilience of the energy, water and food nexus in risky environments. Renew. Sustain. Energy Rev. 112, 653–668. doi:10.1016/J.RSER.2019.06.015
Guo, X. X., Zhao, D., Zhuang, M. H., Wang, C., and Zhang, F. S. (2021). Fertilizer and pesticide reduction in cherry tomato production to achieve multiple environmental benefits in Guangxi, China, 793. Science of the Total Environment. doi:10.1016/j.scitotenv.2021.148527
Gupta, M., Savla, N., Pandit, C., Pandit, S., Gupta, P. K., Pant, M., et al. (2022). Use of biomass-derived biochar in wastewater treatment and power production: A promising solution for a sustainable environment. Sci. Total Environ. 825, 153892. doi:10.1016/J.SCITOTENV.2022.153892
Haas, P. M., and Ivanovskis, N. (2022). Prospects for implementing the SDGs. Curr. Opin. Environ. Sustain. 56, 101176. doi:10.1016/J.COSUST.2022.101176
Haitsma Mulier, M. C. G., Van de Ven, F. H. M., and Kirshen, P. (2022). Circularity in the urban water-energy-nutrients-food nexus. Energy Nexus 100081, 100081. doi:10.1016/J.NEXUS.2022.100081
Haji, M., Govindan, R., and Al-Ansari, T. (2022). A computational modelling approach based on the ‘Energy - water - Food nexus node’ to support decision-making for sustainable and resilient food security. Comput. Chem. Eng. 163, 107846. doi:10.1016/J.COMPCHEMENG.2022.107846
Hao, L., Wang, P., Yu, J., and Ruan, H. (2022). An integrative analytical framework of water-energy-food security for sustainable development at the country scale: A case study of five central asian countries. J. Hydrology 607, 127530. doi:10.1016/J.JHYDROL.2022.127530
Hasler, K., Bröring, S., Omta, S. W. F., and Olfs, H. W. (2015). Life cycle assessment (LCA) of different fertilizer product types. Eur. J. Agron. 69, 41–51. doi:10.1016/J.EJA.2015.06.001
Herrmann, B., and Rundshagen, V. (2020). Paradigm shift to implement SDG 2 (end hunger): A humanistic management lens on the education of future leaders. Int. J. Manag. Educ. 18 (1), 100368. doi:10.1016/J.IJME.2020.100368
Hu, Q., Jung, J., Chen, D., Leong, K., Song, S., Li, F., et al. (2021). Biochar industry to circular economy. Sci. Total Environ. 757, 143820. doi:10.1016/J.SCITOTENV.2020.143820
Huang, C., Mohamed, B. A., and Li, L. Y. (2022). Comparative life-cycle assessment of pyrolysis processes for producing bio-oil, biochar, and activated carbon from sewage sludge. Resour. Conservation Recycl. 181, 106273. doi:10.1016/J.RESCONREC.2022.106273
Huang, X., Yu, F., Peng, Q., and Huang, Y. (2018). Superb adsorption capacity of biochar derived from leather shavings for Congo red. RSC Adv. 8 (52), 29781–29788. doi:10.1039/c8ra06370b
Humbird, D., Davis, R., Tao, L., Kinchin, C., Hsu, D., Aden, A., et al. (2011). Process design and economics for biochemical conversion of lignocellulosic biomass to ethanol. May: national renewable energy laboratory. National Renewable Energy Laboratory-NREL.
Irannezhad, M., Ahmadi, B., Liu, J., Chen, D., and Matthews, J. H. (2022). Global water security: A shining star in the dark sky of achieving the sustainable development goals. Sustain. Horizons 1, 100005. doi:10.1016/J.HORIZ.2021.100005
Jeswani, H. K., Gujba, H., Brown, N. W., Roberts, E. P. L., and Azapagic, A. (2015). Removal of organic compounds from water: Life cycle environmental impacts and economic costs of the Arvia process compared to granulated activated carbon. J. Clean. Prod. 89, 203–213. doi:10.1016/j.jclepro.2014.11.017
Ji, L., Zheng, Z., Wu, T., Xie, Y., Liu, Z., Huang, G., et al. (2020). Synergetic optimization management of crop-biomass coproduction with food-energy-water nexus under uncertainties. J. Clean. Prod. 258, 120645. doi:10.1016/j.jclepro.2020.120645
Jiang, Z., Zheng, H., and Xing, B. (2021). Environmental life cycle assessment of wheat production using chemical fertilizer, manure compost, and biochar-amended manure compost strategies. Sci. Total Environ. 760, 143342. doi:10.1016/J.SCITOTENV.2020.143342
Jindo, K., Sánchez-Monedero, M. A., Mastrolonardo, G., Audette, Y., Higashikawa, F. S., Silva, C. A., et al. (2020). Role of biochar in promoting circular economy in the agriculture sector. Part 2: A review of the biochar roles in growing media, composting and as soil amendment. Chem. Biol. Technol. Agric. 7 (1), 16. doi:10.1186/s40538-020-00179-3
Jjagwe, J., Olupot, P. W., Menya, E., and Kalibbala, H. M. (2021). Synthesis and application of granular activated carbon from biomass waste materials for water treatment: A review. J. Bioresour. Bioprod. 6 (4), 292–322. doi:10.1016/j.jobab.2021.03.003
Jones, S., Meyer, P., Snowden-Swan, L., Padmaperuma, A., Tan, E., Dutta, A., et al. (2013). Process design and economics for the conversion of lignocellulosic biomass to hydrocarbon fuels: Fast pyrolysis and hydrotreating bio-oil pathway. Golden, CO (United States): National Renewable Energy Lab.
Jurgilevich, A., Birge, T., Kentala-Lehtonen, J., Korhonen-Kurki, K., Pietikäinen, J., Saikku, L., et al. (2016). Transition towards circular economy in the food system. Sustain. Switz. 8 (1), 69. doi:10.3390/su8010069
Kamil, M., Ramadan, K. M., Olabi, A. G., Shanableh, A., Ghenai, C., Al Naqbi, A. K., et al. (2019). Comprehensive evaluation of the life cycle of liquid and solid fuels derived from recycled coffee waste. Resour. Conservation Recycl. 150, 104446. doi:10.1016/J.RESCONREC.2019.104446
Kanyilmaz, A., Tichell, P. R. N., and Loiacono, D. (2022). A genetic algorithm tool for conceptual structural design with cost and embodied carbon optimization. Eng. Appl. Artif. Intell. 112, 104711. doi:10.1016/j.engappai.2022.104711
Ke, L., Wu, Q., Zhou, N., Xiong, J., Yang, Q., Zhang, L., et al. (2022). Lignocellulosic biomass pyrolysis for aromatic hydrocarbons production: Pre and in-process enhancement methods. Renew. Sustain. Energy Rev. 165, 112607. doi:10.1016/J.RSER.2022.112607
Khajuria, A., Atienza, V. A., Chavanich, S., Henning, W., Islam, I., Kral, U., et al. (2022). Accelerating circular economy solutions to achieve the 2030 agenda for sustainable development goals. Circ. Econ. 1 (1), 100001. doi:10.1016/J.CEC.2022.100001
Klufallah, M. M. A., Nuruddin, M. F., Khamidi, M. F., and Jamaludin, N. (2014). Assessment of carbon emission reduction for buildings projects in Malaysia-A comparative analysis. E3S Web Conf. 3, 01016. doi:10.1051/e3sconf/20140301016
Kua, H. W., Mašek, O., and Gupta, S. (2020). Biochar-based circular economy. Biochar, 7-1–7-9. doi:10.1088/978-0-7503-2660-5ch7
Kumar, M., Gikas, P., Kuroda, K., and Vithanage, M. (2022). Tackling water security: A global need of cross-cutting approaches. J. Environ. Manag. 306, 114447. doi:10.1016/J.JENVMAN.2022.114447
Laso, J., Margallo, M., García-Herrero, I., Fullana, P., Bala, A., Gazulla, C., et al. (2018). Combined application of Life Cycle Assessment and linear programming to evaluate food waste-to-food strategies: Seeking for answers in the nexus approach. Waste Manag. 80, 186–197. doi:10.1016/J.WASMAN.2018.09.009
Li, H., Li, M., Fu, Q., Cao, K., Liu, D., and Li, T. (2022). Optimization of biochar systems in the water-food-energy-carbon nexus for sustainable circular agriculture. J. Clean. Prod. 355, 131791. doi:10.1016/J.JCLEPRO.2022.131791
Li, K., Niu, X., Zhang, D., Guo, H., Zhu, X., Yin, H., et al. (2022). Renewable biochar derived from mixed sewage sludge and pine sawdust for carbon dioxide capture. Environ. Pollut. 306, 119399. doi:10.1016/J.ENVPOL.2022.119399
Li, M., Li, H., Fu, Q., Liu, D., Yu, L., and Li, T. (2021). Approach for optimizing the water-land-food-energy nexus in agroforestry systems under climate change. Agric. Syst. 192, 103201. doi:10.1016/J.AGSY.2021.103201
Li, M., Zhao, L., Zhang, C., Liu, Y., and Fu, Q. (2022). Optimization of agricultural resources in water-energy-food nexus in complex environment: A perspective on multienergy coordination. Energy Convers. Manag. 258, 115537. doi:10.1016/J.ENCONMAN.2022.115537
Li, S., and Skelly, S. (2023). Physicochemical properties and applications of biochars derived from municipal solid waste: A review. Environ. Adv. 13, 100395. doi:10.1016/J.ENVADV.2023.100395
Li, S., Wu, J., Wang, X., and Ma, L. (2020). Economic and environmental sustainability of maize-wheat rotation production when substituting mineral fertilizers with manure in the North China Plain. J. Clean. Prod. 271, 122683. doi:10.1016/j.jclepro.2020.122683
Liberati, D., Ahmed, S. W., Samad, N., Mugnaioni, R., Shaukat, S., Muddasir, M., et al. (2023). Biochar amendment for reducing the environmental impacts of reclaimed polluted sediments. J. Environ. Manag. 344, 118623. doi:10.1016/J.JENVMAN.2023.118623
Liu, Q., Kong, G., Zhang, G., Cao, T., Wang, K., Zhang, X., et al. (2023). Recent advances in hydrothermal liquefaction of manure wastes into value-added products. Energy Convers. Manag. 292, 117392. doi:10.1016/J.ENCONMAN.2023.117392
Lu, Y., Gu, K., Shen, Z., Tang, C. S., Shi, B., and Zhou, Q. (2023). Biochar implications for the engineering properties of soils: A review. Sci. Total Environ. 888, 164185. doi:10.1016/J.SCITOTENV.2023.164185
Majumder, S., Neogi, S., Dutta, T., Powel, M. A., and Banik, P. (2019). The impact of biochar on soil carbon sequestration: Meta-analytical approach to evaluating environmental and economic advantages. J. Environ. Manag. 250, 109466. doi:10.1016/j.jenvman.2019.109466
Malik, P. K. (2004). Dye removal from wastewater using activated carbon developed from sawdust: Adsorption equilibrium and kinetics. J. Hazard. Mater. 113 (1–3), 81–88. doi:10.1016/j.jhazmat.2004.05.022
Mannan, M., Al-Ansari, T., Mackey, H. R., and Al-Ghamdi, S. G. (2018). Quantifying the energy, water and food nexus: A review of the latest developments based on life-cycle assessment. J. Clean. Prod. 193, 300–314. doi:10.1016/J.JCLEPRO.2018.05.050
Mariyam, S., Shahbaz, M., Al-Ansari, T., Mackey, H. R., and McKay, G. (2022). A critical review on co-gasification and co-pyrolysis for gas production. Renew. Sustain. Energy Rev. 161, 112349. doi:10.1016/J.RSER.2022.112349
Marttunen, M., Mustajoki, J., Sojamo, S., Ahopelto, L., and Keskinen, M. (2019). A framework for assessing water security and the water-energy-food nexus-the case of Finland. Sustain. Switz. 11 (10), 2900. doi:10.3390/su11102900
Marufuzzaman, M., Ekşioğlu, S. D., and Hernandez, R. (2015). Truck versus pipeline transportation cost analysis of wastewater sludge. Transp. Res. Part A Policy Pract. 74, 14–30. doi:10.1016/j.tra.2015.02.001
Mercure, J. F., Paim, M. A., Bocquillon, P., Lindner, S., Salas, P., Martinelli, P., et al. (2019). Renewable and Sustainable Energy Reviews, 105. doi:10.1016/j.rser.2019.01.045System complexity and policy integration challenges: The Brazilian Energy- Water-Food Nexus
Mikos-Szymańska, M., Schab, S., Rusek, P., Borowik, K., Bogusz, P., and Wyzińska, M. (2019). Preliminary study of a method for obtaining Brown coal and biochar based granular compound fertilizer. Waste Biomass Valorization 10 (12), 3673–3685. doi:10.1007/s12649-019-00655-4
Mirzaei, A., Saghafian, B., Mirchi, A., and Madani, K. (2019). The groundwater-energy-food nexus in Iran’s agricultural sector: Implications for water security. WaterSwitzerl. 11 (9), 1835. doi:10.3390/w11091835
Mukherjee, A., and Lal, R. (2013). Biochar impacts on soil physical properties and greenhouse gas emissions. Agronomy 3 (2), 313–339. doi:10.3390/agronomy3020313
Mylavarapu, R., Nair, V., and Morgan, K. (2013). An introduction to biochars and their uses in agriculture. EDIS 2013 (8). doi:10.32473/edis-ss585-2013
Naher, U. A., Ahmed, M. N., Imran, M., Sarkar, U., Biswas, J. C., and Panhwar, Q. A. (2018). “Fertilizer management strategies for sustainable rice production,” in Organic farming: Global perspectives and methods. doi:10.1016/B978-0-12-813272-2.00009-4
Namany, S., and Al-Ansari, T. (2021). “Energy, water, food nexus decision-making for sustainable food security,” in The water–energy–food nexus (Springer), 191–216.
Namany, S., Al-Ansari, T., and Govindan, R. (2019). Optimisation of the energy, water, and food nexus for food security scenarios. Comput. Chem. Eng. 129, 106513. doi:10.1016/j.compchemeng.2019.106513
Namany, S., Govindan, R., Martino, M., Pistikopoulos, E. N., Linke, P., Avraamidou, S., et al. (2021). An energy-water-food nexus-based decision-making framework to guide national priorities in Qatar. Sustain. Cities Soc. 75, 103342. doi:10.1016/j.scs.2021.103342
National Environment Agency (2019). NEA | waste statistics and overall recycling. NEA Official Website.
Nepal, S., Neupane, N., Belbase, D., Pandey, V. P., and Mukherji, A. (2021). Achieving water security in Nepal through unravelling the water-energy-agriculture nexus. Int. J. Water Resour. Dev. 37 (1), 67–93. doi:10.1080/07900627.2019.1694867
Neves, D., Thunman, H., Matos, A., Tarelho, L., and Gómez-Barea, A. (2011). Characterization and prediction of biomass pyrolysis products. Prog. Energy Combust. Sci. 37 (5), 611–630. doi:10.1016/j.pecs.2011.01.001
Núñez-López, J. M., Rubio-Castro, E., and Ponce-Ortega, J. M. (2022). Optimizing resilience at water-energy-food nexus. Comput. Chem. Eng. 160, 107710. doi:10.1016/J.COMPCHEMENG.2022.107710
Ogbolumani, O. A., and Nwulu, N. I. (2022). A food-energy-water nexus meta-model for food and energy security. Sustain. Prod. Consum. 30, 438–453. doi:10.1016/j.spc.2021.12.019
Ouedrhiri, A., Ait Himi, M., Youbi, B., Lghazi, Y., Bahar, J., El Haimer, C., et al. (2022). Biochar material derived from natural waste with superior dye adsorption performance. Mater. Today Proc. 66, 259–267. doi:10.1016/J.MATPR.2022.04.928
Owen, A., Scott, K., and Barrett, J. (2018). Identifying critical supply chains and final products: An input-output approach to exploring the energy-water-food nexus. Appl. Energy 210, 632–642. doi:10.1016/J.APENERGY.2017.09.069
Paiho, S., Mäki, E., Wessberg, N., Paavola, M., Tuominen, P., Antikainen, M., et al. (2020). Towards circular cities—conceptualizing core aspects. Sustain. Cities Soc. 59, 102143. doi:10.1016/J.SCS.2020.102143
Parsa, A., Van De Wiel, M. J., and Schmutz, U. (2021). Intersection, interrelation or interdependence? The relationship between circular economy and nexus approach. J. Clean. Prod. 313, 127794. doi:10.1016/J.JCLEPRO.2021.127794
Parthasarathy, P., Al-Ansari, T., Mackey, H. R., Sheeba Narayanan, K., and McKay, G. (2022). A review on prominent animal and municipal wastes as potential feedstocks for solar pyrolysis for biochar production. Fuel 316, 123378. doi:10.1016/J.FUEL.2022.123378
Parthasarathy, P., Alherbawi, M., Pradhan, S., McKay, G., and Al-Ansari, T. (2023). Pyrolysis of livestock manures: Optimal operating conditions and feedstock blending ratios. Comput. Aided Chem. Eng. 52, 2297–2302. doi:10.1016/B978-0-443-15274-0.50366-8
Parthasarathy, P., Fernandez, A., Al-Ansari, T., Mackey, H. R., Rodriguez, R., and McKay, G. (2021). Thermal degradation characteristics and gasification kinetics of camel manure using thermogravimetric analysis. J. Environ. Manag. 287, 112345. doi:10.1016/J.JENVMAN.2021.112345
Parthasarathy, P., Sajjad, S., Saleem, J., Alherbawi, M., and McKay, G. (2022). A review of the removal of dyestuffs from effluents onto biochar. Separations 9 (6), 139. doi:10.3390/separations9060139
Partzsch, L., Hartung, K., Lümmen, J., and Zickgraf, C. (2021). Water in your coffee? Accelerating SDG 6 through voluntary certification programs. J. Clean. Prod. 324, 129252. doi:10.1016/J.JCLEPRO.2021.129252
Patra, J. M., Panda, S. S., and Dhal, N. K. (2017). Biochar as a low-cost adsorbent for heavy metal removal: A review. Int. J. Res. Biosci. 6 (1), 1–7.
Poudel, D., and Gopinath, M. (2021). Exploring the disparity in global food security indicators. Glob. Food Secur. 29, 100549. doi:10.1016/J.GFS.2021.100549
Poudel, J., Ohm, T. I., Lee, S. H., and Oh, S. C. (2015). A study on torrefaction of sewage sludge to enhance solid fuel qualities. Waste Manag. 40, 112–118. doi:10.1016/J.WASMAN.2015.03.012
Pradhan, S., Abdelaal, A. H., Mroue, K., Al-Ansari, T., Mackey, H. R., and McKay, G. (2020). Biochar from vegetable wastes: Agro-environmental characterization. Biochar 2 (4), 439–453. doi:10.1007/s42773-020-00069-9
Rasul, G., and Sharma, B. (2016). The nexus approach to water–energy–food security: An option for adaptation to climate change. Clim. Policy 16 (6), 682–702. doi:10.1080/14693062.2015.1029865
Requejo-Castro, D., Giné-Garriga, R., and Pérez-Foguet, A. (2020). Data-driven Bayesian network modelling to explore the relationships between SDG 6 and the 2030 Agenda. Sci. Total Environ. 710, 136014. doi:10.1016/J.SCITOTENV.2019.136014
Rodias, E., Aivazidou, E., Achillas, C., Aidonis, D., and Bochtis, D. (2021). Water-energy-nutrients synergies in the agrifood sector: A circular economy framework. Energies 14 (1), 159. doi:10.3390/en14010159
Rombel, A., Krasucka, P., and Oleszczuk, P. (2022). Sustainable biochar-based soil fertilizers and amendments as a new trend in biochar research. Sci. Total Environ. 816, 151588. doi:10.1016/j.scitotenv.2021.151588
Rosales-Asensio, E., de la Puente-Gil, Á., García-Moya, F. J., Blanes-Peiró, J., and de Simón-Martín, M. (2020). Decision-making tools for sustainable planning and conceptual framework for the energy–water–food nexus. Energy Rep. 6, 4–15. doi:10.1016/J.EGYR.2020.08.020
Sachs, I., and Silk, D. (1990). Food and energy: Strategies for sustainable development, 1. United Nations University Press.
Samoraj, M., Mironiuk, M., Witek-Krowiak, A., Izydorczyk, G., Skrzypczak, D., Mikula, K., et al. (2022). Biochar in environmental friendly fertilizers - prospects of development products and technologies. Chemosphere 296, 133975. doi:10.1016/j.chemosphere.2022.133975
Schmidt, J. I., Johnson, B., Huntington, H. P., and Whitney, E. (2022). A framework for assessing food-energy-water security: A few case studies from rural Alaska. Sci. Total Environ. 821, 153355. doi:10.1016/J.SCITOTENV.2022.153355
Seow, Y. X., Tan, Y. H., Mubarak, N. M., Kansedo, J., Khalid, M., Ibrahim, M. L., et al. (2022). A review on biochar production from different biomass wastes by recent carbonization technologies and its sustainable applications. J. Environ. Chem. Eng. 10 (1), 107017. doi:10.1016/J.JECE.2021.107017
Sgroi, M., Vagliasindi, F. G. A., and Roccaro, P. (2018). Feasibility, sustainability and circular economy concepts in water reuse. Curr. Opin. Environ. Sci. Health 2, 20–25. doi:10.1016/J.COESH.2018.01.004
Shahbaz, M., AlNouss, A., Ghiat, I., Mckay, G., Mackey, H., Elkhalifa, S., et al. (2021). A comprehensive review of biomass based thermochemical conversion technologies integrated with CO2 capture and utilisation within BECCS networks. Resour. Conservation Recycl. 173, 105734. doi:10.1016/J.RESCONREC.2021.105734
Singh, A. (2012). An overview of the optimization modelling applications. J. Hydrology 466–467, 167–182. doi:10.1016/J.JHYDROL.2012.08.004
Slorach, P. C., Jeswani, H. K., Cuéllar-Franca, R., and Azapagic, A. (2020). Environmental sustainability in the food-energy-water-health nexus: A new methodology and an application to food waste in a circular economy. Waste Manag. 113, 359–368. doi:10.1016/j.wasman.2020.06.012
Smith, P. (2016). Soil carbon sequestration and biochar as negative emission technologies. Glob. Change Biol. 22 (3), 1315–1324. doi:10.1111/gcb.13178
Smol, M., Adam, C., and Preisner, M. (2020). Circular economy model framework in the European water and wastewater sector. J. Material Cycles Waste Manag. 22 (3), 682–697. doi:10.1007/s10163-019-00960-z
Stabnikova, O., Ding, H. B., Tay, J. H., and Wang, J. Y. (2005). Biotechnology for aerobic conversion of food waste into organic fertilizer. Waste Manag. Res. 23 (1), 39–47. doi:10.1177/0734242X05049768
Stahel, W. R. (2013). The business angle of a circular economy – higher competitiveness, higher resource security and material efficiency. A New Dyn. - Eff. Bus. a Circular Econ. 1.
Stringer, L. C., Mirzabaev, A., Benjaminsen, T. A., Harris, R. M. B., Jafari, M., Lissner, T. K., et al. (2021). Climate change impacts on water security in global dryland. One Earth 4 (6), 851–864. doi:10.1016/J.ONEEAR.2021.05.010
Sukhwani, V., Shaw, R., Mitra, B. K., and Yan, W. (2019). Optimizing Food-Energy-Water (FEW) nexus to foster collective resilience in urban-rural systems. Prog. Disaster Sci. 1, 100005. doi:10.1016/J.PDISAS.2019.100005
Summit, W. F. (1996). Rome declaration on world food security and world food summit plan of action Rome declaration on world food security. Rome: World Food Summit, 1–2.
Sun, Y., Wang, T., Sun, X., Bai, L., Han, C., and Zhang, P. (2021). The potential of biochar and lignin-based adsorbents for wastewater treatment: Comparison, mechanism, and application—a review. Industrial Crops Prod. 166, 113473. doi:10.1016/j.indcrop.2021.113473
Tan, A. H. P., and Yap, E. H. (2019). Energy security within Malaysia’s water-energy-food nexus—A systems approach. Systems 7 (1), 14. doi:10.3390/systems7010014
Thompson, K. A., Shimabuku, K. K., Kearns, J. P., Knappe, D. R. U., Summers, R. S., and Cook, S. M. (2016). Environmental comparison of biochar and activated carbon for tertiary wastewater treatment. Environ. Sci. Technol. 50 (20), 11253–11262. doi:10.1021/acs.est.6b03239
Tiseo, I. (2021). Global waste generation - statistics and facts. Environment, development and sustainability.
Uwaoma, R. C., Strydom, C. A., Bunt, J. R., Matjie, R. H., Okolo, G. N., and Marx, S. (2021). Co-gasification reactivity and kinetics of municipality and coffee waste residue hydrochar and South African density separated coal blends. Bioresour. Technol. Rep. 16, 100877. doi:10.1016/J.BITEB.2021.100877
Vieira da Silva Filho, S., Peyerl, D., and Moutinho dos Santos, E. (2022). Early insights on the fracking impacts to the water-energy nexus in Brazil: Is there a risk of water scarcity in the shale gas prospective areas? J. Clean. Prod. 336, 130390. doi:10.1016/J.JCLEPRO.2022.130390
Walling, E., and Vaneeckhaute, C. (2020). Greenhouse gas emissions from inorganic and organic fertilizer production and use: A review of emission factors and their variability. J. Environ. Manag. 276, 111211. doi:10.1016/j.jenvman.2020.111211
Wang, J., and Wang, S. (2019). Preparation, modification and environmental application of biochar: A review. J. Clean. Prod. 227, 1002–1022. doi:10.1016/J.JCLEPRO.2019.04.282
Water reuse (2012). “Potential for expanding the nation’s water supply through reuse of municipal wastewater,” in Water reuse: Potential for expanding the nation’s water supply through reuse of municipal wastewater. doi:10.17226/13303
Weber, K., and Quicker, P. (2018). Properties of biochar. Fuel 217, 240–261. doi:10.1016/J.FUEL.2017.12.054
Wennersten, R., and Qie, S. (2018). “United Nations sustainable development goals for 2030 and resource use,” in World sustainability series. doi:10.1007/978-3-319-63007-6_19
WHO/UNICEF, I.-S. S. 6 P. R (2021). “WHO/UNICEF joint monitoring Program for water supply, sanitation and hygiene (JMP) – progress on household drinking water, sanitation and hygiene 2000 – 2020,” in Imi-Sdg6 sd 6 progress reports.
Wong, K. V. (2015). Energy–water–food nexus and recommendations for security. J. Energy Resour. Technol. 137 (3). doi:10.1115/1.4028773
Wright, M. M., Daugaard, D. E., Satrio, J. A., and Brown, R. C. (2010). Techno-economic analysis of biomass fast pyrolysis to transportation fuels. Fuel 89 (1), S2–S10. doi:10.1016/j.fuel.2010.07.029
Wu, H., MacDonald, G. K., Galloway, J. N., Zhang, L., Gao, L., Yang, L., et al. (2021). The influence of crop and chemical fertilizer combinations on greenhouse gas emissions: A partial life-cycle assessment of fertilizer production and use in China. Resour. Conservation Recycl. 168, 105303. doi:10.1016/J.RESCONREC.2020.105303
Xiang, W., Zhang, X., Chen, J., Zou, W., He, F., Hu, X., et al. (2020). Biochar technology in wastewater treatment: A critical review. Chemosphere 252, 126539. doi:10.1016/j.chemosphere.2020.126539
Yang, H., Liu, X., Liu, Y., Cui, J., and Xiao, Y. (2023). Revolutionizing biochar synthesis for enhanced heavy metal adsorption: Harnessing machine learning and Bayesian optimization. J. Environ. Chem. Eng. 11 (5), 110593. doi:10.1016/J.JECE.2023.110593
Yuxi, Z., Jingke, H., Changlin, X., and Zhangmiao, L. (2021). Unfolding the synergy and interaction of water-land-food nexus for sustainable resource management: A supernetwork analysis. Sci. Total Environ. 784, 147085. doi:10.1016/J.SCITOTENV.2021.147085
Zheng, Z., Ji, L., Xie, Y., Huang, G., and Pan, J. (2022). Synergic management of crop planting structure and biomass utilization pathways under a food-energy-water nexus perspective. J. Clean. Prod. 335, 130314. doi:10.1016/J.JCLEPRO.2021.130314
Ziane, H., Hamza, N., and Meddad-Hamza, A. (2021). Arbuscular mycorrhizal fungi and fertilization rates optimize tomato (Solanum lycopersicum L.) growth and yield in a Mediterranean agroecosystem. J. Saudi Soc. Agric. Sci. 20 (7), 454–458. doi:10.1016/j.jssas.2021.05.009
Keywords: biochar, energy, water, and food nexus, optimization, circular economy, biomass, sustainability
Citation: Obar F, Alherbawi M, Mckay G and Al-Ansari T (2023) Optimizing the utilization of biochar from waste: an energy–water–food nexus assessment approach considering water treatment and soil application scenarios. Front. Environ. Sci. 11:1238810. doi: 10.3389/fenvs.2023.1238810
Received: 14 June 2023; Accepted: 04 September 2023;
Published: 28 September 2023.
Edited by:
Xiaofei Tan, Hunan University, ChinaReviewed by:
Shaon Kumar Das, The ICAR Research Complex for North Eastern Hill Region (ICAR RC NEH), IndiaZhengang Liu, Chinese Academy of Sciences (CAS), China
Copyright © 2023 Obar, Alherbawi, Mckay and Al-Ansari. This is an open-access article distributed under the terms of the Creative Commons Attribution License (CC BY). The use, distribution or reproduction in other forums is permitted, provided the original author(s) and the copyright owner(s) are credited and that the original publication in this journal is cited, in accordance with accepted academic practice. No use, distribution or reproduction is permitted which does not comply with these terms.
*Correspondence: Tareq Al-Ansari, dGFsYW5zYXJpQGhia3UuZWR1LnFh