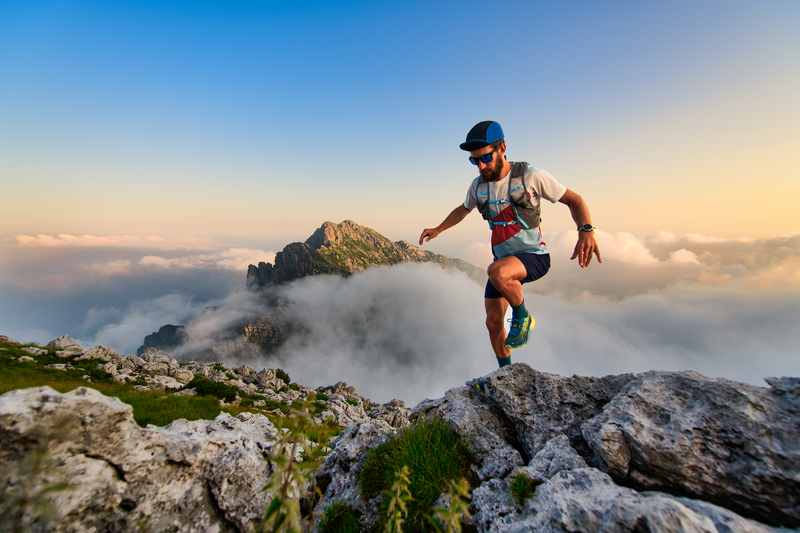
94% of researchers rate our articles as excellent or good
Learn more about the work of our research integrity team to safeguard the quality of each article we publish.
Find out more
ORIGINAL RESEARCH article
Front. Environ. Sci. , 29 June 2023
Sec. Toxicology, Pollution and the Environment
Volume 11 - 2023 | https://doi.org/10.3389/fenvs.2023.1221573
This article is part of the Research Topic Recent Research Advances on Heavy Metals, Microplastics, Persistent Organic Pollutants, and Solid Waste in Aquatic and Terrestrial Ecosystems View all 11 articles
Environmental pollution caused by heavy metals has long been considered a relevant threat to ecosystem survival and human health. The use of safer substitutes for the most toxic heavy metals in many industrial applications is discussed as a potential way to face this issue. In this regard, Bi has been proposed for replacing Pb in several production processes. However, few literature records reported on the effects of Bi on living organisms, particularly on plants. In this study, garden cress (Lepidium sativum L.) plants were exposed to different concentrations of Bi nitrate added to soil in growth chambers for 21 days. Results evidenced the toxic effect of Bi on shoot growth, regardless of the Bi nitrate concentration in the soil, paralleled by a similar reduction in the chlorophyll and carotenoid content, a decrease in the nitrogen balance index values, and an impairment of the photosynthetic machinery evaluated by chlorophyll fluorescence image analysis. The presence of Bi in the soil was shown to affect element accumulation in roots and translocation to shoots, with micronutrient content particularly reduced in the leaves of Bi-treated plants. A dose-dependent plant accumulation of Bi to metal concentration in the soil was observed, even if very low metal bioconcentration ability was highlighted. The reduced Bi translocation from roots to shoots in plants exposed to increasing Bi concentrations in the soil is discussed as a possible defense mechanism likely associated with the observed increase of anthocyan and flavonol contents and the activation of photoprotection mechanisms preventing higher damages to the photosynthetic apparatus.
The increasing occurrence of heavy metals (HMs) in the environmental matrices, resulting from industrial processes and mining activities, and their recognized toxicity on biota are necessitating the adoption of effective solutions for mitigating their impact on the ecosystem and human health. In this context, replacing toxic HMs in industrial processes with safer ones is considered promising. To enhance the environmental sustainability of many industrial applications involving lead (Pb), the utilization of bismuth (Bi) as a Pb substitute has attracted increasing interest (Rohr, 2002; Esquivel-Gaon et al., 2015). In this regard, Wang et al. (2019) reported the use of Bi as a nontoxic replacement for Pb in brass plumbing fixtures, fishing sinkers, free machining steels, and solders, as well as a metallurgical additive in the foundry. Bismuth, a minor metal with a natural abundance 10-fold less than that of antimony (Sb), is considered a “green metal” given its use for treating many health issues since ancient times (Udalova et al., 2008; Wang et al., 2019). The increasing use of Bi among medicinal applications is highly predictable as Bi has been recognized as a theranostic agent (Badrigilan et al., 2020). As the use of Bi-containing products is increasing, a rapid increase in the concentrations of this HM in the environment has to be expected. In this regard, with respect to Bi concentrations in natural soil, ranging from 0.13 to 40 μg g−1 D.W. (Das et al., 2006; Fahey et al., 2008), extremely high values were reported by Elekes and Busuioc (2010) for a forest soil close to a highway (Bi concentrations ranged from 930 to 1,891 mg kg−1) and by Wei et al. (2011) near an antimony mine (Bi concentrations up to 1,672 mg kg−1). Soil enrichment in Bi is also predictable as its release in agriculture through phosphate fertilization is suspected. Concerning the aquatic compartment, Bi increase can be the result of the leaching from metal-contaminated soils, as for other metals (Fu et al., 2010), or the release from wastewater (Amneklev et al., 2015; Amneklev et al., 2016). Also, the presence of Bi in the alpine ice was recently reported by Legrand et al. (2023). Finally, Bi can be found in the environment at high relative concentrations due to the use of fireworks (Massimi et al., 2021).
Despite the growing interest in the use of Bi, information on the effects of Bi on biological organisms is not exhaustive. In fact, scarce literature is actually present on the toxicity of Bi on biota, as reported by Badrigilan et al. (2020) in human and animal cells and by Murata et al. (2006) and Omouri et al. (2018) in microbes and earthworms, respectively. Consistently, only few papers recently focused on the effects of Bi on plant species. Seed germination and root elongation were negatively affected by treating perennial ryegrass seeds with Bi nitrate and citrate (Omouri et al., 2019), while the exposure of tomato plants to increasing concentrations of Bi nitrate resulted in enhanced toxicity effects, such as the reduction of shoot and root biomass (Nagata and Kimoto, 2020). Furthermore, the germination and root growth of radish seeds were reduced in soil with 30 and 300 mg kg−1 of Bi nitrate (Sudina et al., 2021).
Physiological processes underpinning photosynthetic machinery in plants are very sensitive to metals (Khan et al., 2019). Among them, pigment metabolism and the photochemical efficiency of photosystem II have been long claimed (Aggarwal et al., 2012; Pietrini et al., 2015). In particular, chlorophyll content is recognized as an endpoint to assess ecotoxicity in plants, also in official protocols (ISO/DIS, 20079, 2004; OECD n. 221, 2006a). Carotenoids and anthocyans and flavonols (flavonoids) have also been reported as involved in the plant response to metal stress (Chandra and Kang, 2016; Baskar et al., 2018; Shomali et al., 2022). In this context, it is worth mentioning that pigment content can be effectively evaluated in a non-destructive manner by leaf transmittance and spectral reflectance (Goulas et al., 2004; Croft and Chen, 2017; Pietrini et al., 2023). As a proxy of the photosynthetic efficiency, chlorophyll fluorescence parameters are commonly utilized for detecting toxic effects caused in leaves by stressors (Moustakas et al., 2021), including metals (Pietrini et al., 2015; 2017; 2020). In this regard, the suitability of different chlorophyll fluorescence parameters to highlight the damaging effect of a stressor is debated in the literature. In fact, the photochemical efficiency of photosystem II (Fv/Fm), measured in dark-adapted leaves, is the most used parameter in plant stress studies, but it is often reported as less sensitive and responsive than others, as, e.g., the effective quantum yield of PSII photochemistry (ΦPSII) (Pietrini et al., 2015; Malinská et al., 2020).
Recently, Passatore et al. (2022) observed root growth inhibition, genotoxic effects, and dose-dependent Bi accumulation in Lepidium sativum L (garden cress) plantlets after treating the seeds with different Bi nitrate concentrations. In order to get an insight into the toxicity effects and accumulation ability in plants, plants of Lepidium sativum were grown in potted soil supplied with three different levels of Bi nitrate, chosen among those previously used (Passatore et al., 2022). Lepidium sativum was utilized, as in the previous work, being a model plant for ecotoxicological assay (APAT-RTI CTN_TES 1/2004, 2004; OECD n. 208, 2006b). The effects at biometric and physiological levels, specifically focusing on growth, element uptake and translocation, and photosynthetic performances, were investigated along with the Bi accumulation in roots and shoots. The results were discussed, highlighting the main physiological traits involved both in Bi toxicity in plants and in the defense response likely counteracting the toxic action of the metal.
Garden cress (L. sativum) seeds (Ingegnoli seed company, Italy) were sowed in plastic pots (2 L volume, 20 seeds/pot, full seed germination) filled with soil (select black peat substrate, pH 6, EC 0.3 dS/m, apparent density 100 kg/m3, total porosity 85%, Klasmann-Deilmann, Germany) and placed in a growth chamber (temperature 25°C ± 1°C, 16 L/8 D photoperiod, 70% ± 5% humidity, 350 ± 50 µEm2s−1 PPFD). The soil was added or not with Bi nitrate pentahydrate (Sigma-Aldrich, code 248592, 98% purity) solution to reach four different Bi nitrate concentrations: 0 mg kg−1, control; 30 mg kg−1; 121 mg kg−1; and 485 mg kg−1. Particular care was taken to assure that all the soil volume was homogeneously mixed with the Bi solution. Four pots per thesis were randomly distributed in the growth chamber. Soil humidity was maintained at 70% water holding capacity. At the end of the cultivation period (21 days), after physiological measurements (see Section 2.2), the plants were harvested, gently washed with distilled water, separated into roots and shoots, oven-dried, and weighed for dry weight (DW) evaluation and further processing for elemental analysis (see Section 2.3), as described by Passatore et al. (2022).
The estimation of leaf nitrogen and pigment contents was obtained using the leaf-clip portable fluorescence sensor Dualex Scientific+™ (Force-A, France). According to the following equations, it provides four indices: the chlorophyll index (Eq. 1) for leaf chlorophyll concentration in chlorophyll units (Cerovic et al., 2012); the flavonol index (Eq. 2) for epidermal flavonol concentration in absorbance units (Abs. unit); the anthocyanin index (Eq. 3) for epidermal anthocyanin concentration in absorbance units (Abs. unit), and the Nitrogen Balance Index (NBI) index (Eq. 4), as the ratio of chlorophyll to flavonol (Cartelat et al., 2005), which refers to leaf nitrogen content (Cerovic et al., 2012; Cerovic et al., 2015),
where T850 and T710 are the leaf transmittance at 850 nm and 710 nm, respectively; FRF is the far-red fluorescence emission (>710 nm) excited by red (R, 650 nm), UV (UV, 375 nm), or green (G, 505 nm) light.
The measurements were taken from at least two representative fully developed leaves per pot. Two Dualex readings were taken from the widest portion of the leaf lamina, avoiding major veins. The two Dualex readings were averaged to represent the leaf value.
Leaf reflectance spectra were acquired in the spectral range of 350–1025 nm using an ASD FieldSpec-3 spectroradiometer (Analytical Spectral Devices Inc., Colorado, United States), as reported by Testone et al. (2019), except for respecting a fixed distance (10 cm) to the plant layer to assure a field of view (FOV) of approximately 4 cm (using a 25° bare fiber-optic). Five spectra were then determined to provide a mean spectral value. Three spectral reflectance indices, namely, pigment-specific simple ratio a (PSSRa), pigment-specific simple ratio b (PSSRb), and pigment-specific simple ratio c (PSSRc) (Blackburn et al., 1998), respectively, associated with the chlorophyll a, chlorophyll b, and carotenoid content, were derived from the collected data and calculated according to the following equations, where R is the reflectance value measured in each band expressed in nm, indicated by the subscript number:
Chlorophyll fluorescence measurements were performed to assess the efficiency of the plant photosynthetic apparatus. In particular, the maximal quantum yield of PSII photochemistry (Fv/Fm, as the ratio between variable fluorescence and maximal fluorescence), the effective quantum yield of PSII photochemistry (ΦPSII), the quantum yield of regulated non-photochemical energy loss (ΦNPQ), the quantum yield of non-regulated non-photochemical energy loss (ΦNO), the photochemical quenching (qP), the non-photochemical quenching (NPQ), and the electron transport rate (ETR) were measured on fully developed leaves using a MAXI-Imaging-PAM (Walz, Germany). The parameters were measured in 30 min dark-adapted leaves (Fv/Fm) and in leaves adapted to 310 μmol m−2 s−1 PPFD for at least 5 min to reach a steady-state condition (ΦPSII, ΦNPQ, ΦNO, NPQ, qP, and ETR) and calculated as reported by Di Baccio et al. (2017) and Kramer et al. (2004).
After collection, shoots and roots were oven-dried at 70°C for 48 h and weighed; 0.02–0.1 g DW of each sample was subjected to microwave-assisted acid digestion for 30 min at 180°C using a HNO3/H2O2 mixture (2:1, v/v). The digested solution was then diluted with deionized water and filtered before elemental analysis.
The concentration of 40 chemical elements, of which only the concentration of 12 macro- and trace elements is reported and discussed, was analyzed in replicate with an inductively coupled plasma mass spectrometer (ICP-MS; model 820-MS; Bruker, Germany). Element concentrations were divided by the DW of each sample to obtain mg kg-1 concentrations. Standard deviations of the replicates were all below 10%. Further details on sample preparation, elemental analysis, and instrumental conditions are reported in the work of Passatore et al. (2022) and Astolfi et al. (2020), respectively. As reported by Zacchini et al. (2009), the bioconcentration factor (BCF) was calculated as the ratio between the metal concentration in the plant organ (mg Kg-1) and the metal concentration in the soil (mg Kg-1); the translocation factor (Tf) was calculated as the ratio between the metal concentration in plant shoots (mg Kg-1) and the metal concentration in plant roots (mg Kg−1).
The trial was arranged following the methodologies of the OECD guidelines for ecotoxicological tests with L. sativum (OECD n. 208, 2006b). Data reported in tables and figures refer to at least four replicates for the variant. One-way ANOVA was used to process normally distributed data to evaluate the effects of Bi nitrate concentrations on the different parameters using the SPSS (IL, United States) software tool. The statistical significance of the mean data was assessed by Tukey’s test (p ≤ 0.05), unless otherwise stated.
Shoot biomass produced within 21 days of cultivation in soil supplied with four different Bi nitrate concentrations was evaluated to investigate the effect of Bi on the growth of L. sativum plants. The results shown in Figure 1 highlighted that regardless of the Bi concentration, Bi exposure caused a significant growth reduction, ranging approximately from 20% to 26% to control. This finding is in accordance with a previous work on the germination of L. sativum seeds, where root growth inhibition was observed (Passatore et al., 2022). To our knowledge, no medium-term experiments with plants grown in Bi-treated soil are present in the literature. In a short trial (7 days) with artificially Bi-spiked soil, Omouri et al. (2019) observed a significant root growth inhibition in ryegrass plants, but did not find any shoot biomass reduction. A toxic effect on shoot growth was instead reported by Nagata and Kimoto (2020) after the treatment of tomato seeds with Bi nitrate in agar. The toxicity of Bi could be related to DNA damage, as recently reported by Passatore et al. (2022) on L. sativum seedlings, affecting both gene expression and primary and secondary metabolisms.
FIGURE 1. Shoot biomass (g plant-1 dw) in garden cress (Lepidium sativum L.) plants grown in pots filled with soil supplied with bismuth nitrate (0 mg kg−1, control; 30 mg kg−1; 121 mg kg−1; and 485 mg kg−1) for 21 days in controlled conditions in a growth chamber. In each bar, mean data (n = 4, ± S.E.) are shown. Different letters correspond to statistically different values (Tukey’s test, p ≤ 0.05).
To shed light on the effects of Bi at the physiological level, the estimation of both the pigment contents and the nitrogen balance index (NBI, da Silva et al., 2021) was performed using the Dualex sensor (see Section 2.2.1). In Table 1, a reduction in the total chlorophyll content in leaves of plants grown in Bi-added soil is shown. The NBI evidenced a similar trend, being statistically lower in Bi-treated plants compared to control plants, as expected due to the well-studied relationship between chlorophyll and leaf nitrogen content (Sage et al., 1987; Evans, 1989). The reduction of the total chlorophyll content in Bi-exposed plants paralleled the reduction of shoot biomass, indicating this trait as a valuable physiological endpoint for assessing Bi toxicity, as reported for other metals (Pietrini et al., 2015; Chandra and Kang, 2016) and organic pollutants (Kummerová et al., 2006; Pietrini et al., 2022). The suitability of chlorophyll content as a proxy for ecotoxicity assessment in plants has already been proven (ISO/DIS, 20079, 2004; OECD n. 221, 2006a).
TABLE 1. Chlorophyll index (μg cm−2), anthocyanin index (Abs. unit), flavonol index (Abs. unit), and nitrogen balance index (rel. un.) in leaves of garden cress (Lepidium sativum L.) plants grown in pots filled with soil supplied with bismuth nitrate (0 mg kg−1, control; 30 mg kg−1; 121 mg kg−1; and 485 mg kg−1) for 21 days in controlled conditions in a growth chamber (mean data± SE; n = 4). In each column, mean values with different letters are significantly different (Tukey’s test, p ≤ 0.05).
As a protective response to the toxic action of Bi, an overall increase of anthocyan and flavonol leaf levels in Bi-treated plants to control was observed (Table 1). As reported in the literature, in addition to the long-reported functions as screeners of damaging short-wave solar radiation, flavonoids have been suggested as playing key functions as antioxidants in stressed plants by inhibiting the generation and reducing reactive oxygen species (ROS) once formed (Agati et al., 2012; 2013). In this context, a role for anthocyans (Ai et al., 2018) and flavonoids (Ferdinando et al., 2012) as defense compounds against the oxidative attack caused by HMs has been reported. In this study, the increase in flavonoid content in response to Bi treatment could be due to a stimulation of the ROS production by the metal, as observed by Huang et al. (2022) in the microalga Chlamydomonas reinhardtii exposed to nanoscale bismuth oxyiodide (nano-BiOI) at a concentration higher than 25 mg L−1.
Therefore, to further characterize the effects of Bi on pigment content, leaf reflectance spectra of garden cress plants were investigated by analyzing some spectral indices (see Section 2.2.2). As reported by Peñuelas and Filella (1998), leaf surface and internal structure characteristics, in addition to the concentration and distribution of biochemical molecules, are mainly responsible for the leaf reflectance properties. For this reason, leaf reflectance spectra represent a valuable tool to monitor the physiological status of plants under different stress conditions (Croft and Chen, 2017). The values of three spectral reflectance indices (PSSRa, PSSRb, and PSSRc), respectively, associated with chlorophyll a, chlorophyll b, and carotenoid content, are reported in Figure 2. The reduction in chlorophyll content in Bi-treated plants, previously highlighted in Table 1, was also confirmed by the analysis of PSSRa and PSSRb that decreased similarly, regardless of the Bi concentration, with respect to the control. A similar trend was observed for the PSSRc index (Figure 2). As extensively reported (Sun et al., 2022), carotenoids are not only photosynthetic pigments but also important antioxidants. In Bi-treated plants, the reduction in carotenoid content can be ascribed to a damaging effect exerted by the presence of the metal, likely hampering the plants to eliminate oxygen free radicals in chloroplasts and, thereby, protect chlorophylls from photooxidative damage (Guidi et al., 2017).
FIGURE 2. Values of spectral indices, PSSRa- pigment-specific simple ratio for Chla, PSSRb- pigment-specific simple ratio for Chlb, and PSSRc- pigment-specific simple ratio for carotenoids, in leaves of garden cress (Lepidium sativum L.) plants grown in pots filled with peat substrate supplied with bismuth nitrate (0 mg kg−1, control; 30 mg kg−1; 121 mg kg−1; and 485 mg kg−1) for 21 days in controlled conditions in a growth chamber (mean data± SE; n = 4). Different lower-case letters indicate a significant difference in PSSRa among different bismuth nitrate concentrations, different capital letters indicate a significant difference in PSSRb among different bismuth nitrate concentrations, and different Greek letters indicate a significant difference in PSSRc among different bismuth nitrate concentrations (Tukey’s test, p ≤ 0.05).
To get an insight into the possible mechanisms by which Bi could have affected the photosynthetic machinery in garden cress plants, the analysis of chlorophyll fluorescence parameters and their associated images was utilized. Fluorescence image analysis has been commonly used to evaluate the effects of several HMs on plants, providing information on the photosynthetic apparatus performances (Pietrini et al., 2015; 2017; 2020).
In Figure 3, a representative image of chlorophyll fluorescence parameters (Figure 3A, Fv/Fm, ΦPSII, ΦNPQ, and ΦNO) and chlorophyll fluorescence data (Figures 3B, C) in garden cress leaves are presented. Overall, chlorophyll fluorescence images (Figure 3A) showed that, in dark-adapted leaves, Fv/Fm revealed an almost homogeneous pattern of distribution, both in control and Bi-treated plants. Contrarily, in light-adapted leaves, ΦPSII, ΦNPQ, and ΦNO showed an appreciable heterogeneous pattern of light utilization and photosynthetic activity, especially in plants exposed to 121 and 485 mg kg−1 of Bi nitrate. Regarding the photochemical efficiency of photosystem II (PSII), evaluated by Fv/Fm in dark-adapted leaves, results showed a slight decreasing trend as the Bi concentration increased (Figure 3B). Compared with control (0), the Fv/Fm ratio at 30, 121, and 485 mg kg−1 of Bi nitrate decreased by approximately 1, 4, and 6%, respectively. However, it should be stated that all the Fv/Fm values were in the range of 0.75–0.83, considered optimal values for many plant species (Maxwell and Johnson, 2000). In fact, lower values of this parameter indicate that a proportion of the PSII reaction center is damaged or inactivated, commonly observed under stress (Baker and Rosenqvist, 2004). In this regard, Kearns and Turner (2016) reported that the Fv/Fm ratio was not affected in the macroalgae U. lactuca exposed for 48 h to 50 μg L−1 of Bi. However, it is worth stating that the Fv/Fm ratio is considered less sensitive and responsive to different stressors in comparison to the effective quantum yield of PSII photochemistry (ΦPSII) (Pietrini et al., 2015; Malinská et al., 2020). Therefore, to deepen the knowledge on the effects of Bi on plant photochemistry, the evaluation of the balance between the light capture and the photochemical energy use in plants was performed by measuring the effective quantum yield of PSII photochemistry (ΦPSII), the quantum yield of regulated non-photochemical energy loss (ΦNPQ), and the quantum yield of non-regulated non-photochemical energy loss (ΦNO) (Figures 3B, C). The analysis of the ΦPSII response, measured in light-adapted leaves, confirmed the higher sensitivity of this parameter with respect to Fv/Fm. In fact, compared with control (0), the ΦPSII values in plants exposed to 30, 121, and 485 mg kg−1 of Bi nitrate decreased by approximately 7%, 17%, and 26%, respectively (Figure 3B). Regarding the quantum yield of non-photochemical energy loss, with respect to control, the regulated process (ΦNPQ) increased by 10%, 25%, and 29% in 30, 121, and 485 mg kg−1 of Bi-treated plants, respectively, while the non-regulated process (ΦNO) decreased only at the highest Bi nitrate concentrations by 18% and 11%, respectively. Overall, the reported data highlight a quick effective photoprotection mechanism to Bi exposure. In fact, the decrease in the ΦPSII values can result from a photoprotective increase in thermal energy dissipation (ΦNPQ) induced by an excess of absorbed light (Demmig-Adams and Adams, 1992). A non-effective dissipation of excess excitation energy can result in photo-oxidative stress, possibly leading to the production of reactive oxygen species (ROS) (Smirnoff, 1993). Therefore, the increased dissipation by downregulation of the PSII photochemistry, following Bi treatments, seems to overcompensate for the decrease of ΦPSII in such a way that ΦNO decreased. Non-regulated, non-photochemical quenching (ΦNO) consists of chlorophyll fluorescence internal conversions and intersystem crossing, leading to the formation of singlet oxygen (1O2) (Apel and Hirt, 2004; Klughammer and Schreiber, 2008). The decline or similarity to control of ΦNO values in Bi-treated plants could suggest that ΦNPQ was active in protecting plants from ROS, likely by reducing the 1O2 production, thus limiting the oxidative attack to the photosynthetic apparatus (Moustaka et al., 2018).
FIGURE 3. Images of chlorophyll fluorescence parameters (A) and their associated values (B, C) in leaves of garden cress (Lepidium sativum L.) plants grown in pots filled with soil supplied with bismuth nitrate (0 mg kg−1, control; 30 mg kg−1; 121 mg kg−1; and 485 mg kg−1) for 21 days in controlled conditions in a growth chamber. Maximum quantum yield of PSII photochemistry (Fv/Fm), the quantum efficiency of PSII photochemistry (ΦPSII), and the quantum yield of regulated (ΦNPQ) and non-regulated (ΦNO) energy dissipation in PSII are measured with an Imaging-PAM M-series system. The false color code depicted at the bottom of each image ranges from 0.000 (black) to 1.000 (pink). Vertical bars represent means (n = 4) ± S.E. One-way ANOVA was applied, and different letters indicate significant differences (Tukey’s test, p ≤ 0.05).
The reduction of the electron transport rate (ETR) (Figure 4A), a proxy for photosynthesis, paralleled the response of ΦPSII in Bi-exposed plants, likely being caused by the processes affecting excess light energy dissipation (NPQ) (Kalefetoğlu Macar and Ekmekci, 2009), which, in the form of harmless heat, protect plants from ROS (Hideg et al., 2008; Roach et al., 2020). The overall data assessing the status of the photosynthetic machinery evidenced that the greatest effects in Bi-exposed plants were observed at 121 and 485 mg kg−1 of Bi nitrate, concomitantly with the highest decrease of ΦPSII and ETR (Figures 3B, 4A). However, the highest increase in NPQ (Figure 4B) resulted in maintaining a high fraction of open PSII reaction centers (qP), as suggested by the nonstatistical differences between Bi-treated plants and control plants (Figure 4B). According to the literature (Lambrev et al., 2012), this feature is considered a response of maximal photoprotection. Scarce information is reported about the effect of Bi on the plant photosynthetic process. Consistent with the reported results, Prabhavati et al. (2017) highlighted the inhibitory effect on the photosynthesis of Macrotyloma uniflorum (Lam.) plants exposed to different Bi concentrations (from 50 to 400 μg g−1).
FIGURE 4. Changes in the electron transport rate through PSII (ETR) (A) and photochemical (qP) and non-photochemical quenching (NPQ) (B) in leaves of garden cress (Lepidium sativum L.) plants grown in pots filled with soil supplied with bismuth nitrate (0 mg Kg−1, control; 30 mg Kg−1; 121 mg Kg−1; 485 mg Kg−1) for 21 days in controlled conditions in growth chamber. Vertical bars represent means (n = 4) ±S.E. One-way ANOVA was applied and different letters indicate significant difference (Tukey test, p ≤ 0.05).
To evaluate the effects of Bi presence in the soil on the absorption and translocation of nutrients and trace elements, an ionomic analysis was performed by ICP-MS on the roots and shoots of Lepidium plants (Figure 5, 6). The study of the ionome has been long described as a useful tool to simultaneously assess the elemental composition and its change in response to physiological stimuli in plants (Salt et al., 2008). Ionomic analysis was performed by evaluating the concentration of 40 chemical elements in Bi-treated plants (data not shown). The dataset evaluation allowed highlighting the modification of the concentration of 12 chemical elements (9 macro/micronutrients plus non-essential elements such as Na, Co, and Li) in the roots and shoots of Bi-treated Lepidium plants. Regarding roots (Figure 5), results put in evidence how the concentrations of the main macronutrients analyzed (e.g., K, P, Ca, Mg) were not substantially modified after Bi treatment, while the uptake of most micronutrients was negatively affected by all the Bi concentrations tested (e.g., Ni, Cu) or by the highest Bi concentration in the soil only (e.g., Zn, Mn). Contrary to the work of Nishimura and Nagata (2021), Fe concentration in roots was not altered by the presence of Bi in the substrate, likely due to the differences in both the experimental approaches and the plant species used. Concerning not essential elements, while Na concentration was not modified, the uptake of Li and Co was altered in Bi-exposed plants, with a particular reduction at the highest Bi concentration.
FIGURE 5. Ionomic profile (ion concentration, g kg−1 dw) in roots of garden cress (Lepidium sativum L.) plants grown in pots filled with soil supplied with bismuth nitrate (0 mg kg−1, control; 30 mg kg−1; 121 mg kg−1; and 485 mg kg−1) for 21 days in controlled conditions in a growth chamber. In each bar, mean data (n = 4, ± S.E.) are shown. Different letters correspond to statistically different values (Tukey’s test, p ≤ 0.05, ns = not significant).
FIGURE 6. Ionomic profile (ion concentration, g kg−1 dw) in shoots of garden cress (Lepidium sativum L.) plants grown in pots filled with soil supplied with bismuth nitrate (0 mg kg−1, control; 30 mg kg−1; 121 mg kg−1; and 485 mg kg−1) for 21 days in controlled conditions in a growth chamber. In each bar, mean data (n = 4, ± S.E.) are shown. Different letters correspond to statistically different values (Tukey’s test, p ≤ 0.05, ns = not significant).
A data overview on macro-, micro-, and non-essential nutrients in shoots of Bi-treated plants (Figure 6) highlighted a reduction in the concentrations of all the elements, except for Na. In particular, macronutrients P and Ca revealed a near dose-dependent relationship between content in shoots and Bi level in soil, while K and Mg concentrations were negatively affected only in plants grown in soil with 485 mg kg−1 of Bi nitrate. A reduction of the micronutrient (Cu, Fe, Zn, Ni, and Mn) concentrations in the shoots of Bi-exposed plants was also observed, even with different extents among elements. Finally, non-essential element concentration in shoots was drastically reduced at the highest Bi level in the soil, except for Na, which, as observed in roots, was not affected by Bi treatment.
The analysis of other 28 elements in Lepidium plants exposed to different Bi concentrations was also performed, and results for roots (Supplementary Table S1) and shoots (Supplementary Table S2) were reported. Interestingly, a decreasing trend of element concentration in roots as a consequence of Bi treatment was observed for Al, As, and Cd (Supplementary Table S1). At the shoot level (Supplementary Table S2), this trend was evidenced for As and Cd. Notably, Al is the third most abundant element on Earth’s crust, while As and Cd are among the most toxic elements occurring in metal-contaminated sites. Therefore, further investigations will be aimed to shed light on the interference of Bi with the accumulation of these elements in plants.
Taken all together, data on ionomic analysis revealed that Bi presence in the soil had a higher impact on the uptake and translocation of micronutrients rather than macronutrients, where a relevant effect could be observed only for the lower translocation of K, P, Ca, and Mg from roots to shoots in plants exposed to the highest Bi concentration. In this regard, it is worth mentioning the work by Nagata (2015), which highlighted how the exposure to Bi of Arabidopsis thaliana seedlings notably affected the expression levels of genes involved in the absorption of several ions, including Fe, Cu, and Zn. Interestingly, Nagata also put in evidence that the Bi treatment might disturb iron homeostasis in seedlings, resulting in a significant increase of iron content in plant roots. Therefore, even if the experimental conditions of Nagata’s work were quite different from those utilized in the present experimental trial (plant species, substrate, and Bi concentrations), the alteration of the expression profile of genes involved in the element transport caused by Bi exposure could have been involved in the modification of the uptake and translocation of some elements, especially micronutrients, in Bi-treated plants (Figure 5, 6).
The exposure of Lepidium plants to Bi could have negatively affected the growth of plants by interfering with different physiological processes. In fact, the reduction of the macronutrient content in shoots could be associated with the biomass decrease in Bi-treated plants, as reported in Figure 1, considering the primary role of macronutrients for plant growth and development (Tripathi et al., 2014). In this context, the Mg content reduction observed in shoots of Bi-treated plants could have affected the functionality of the photosynthetic process by altering the pigment biosynthetic pathway (Table 1). Moreover, the decrease in micronutrient concentration, especially occurring in the shoots of Bi-treated plants, could have affected the physiological status of plants by altering the enzymatic activity, given the role of these elements (i.e., Cu, Fe, Zn, Ni, and Mn) as cofactors in many fundamental enzymatic reactions, especially those linked to the oxidative status of plants (Pandey, 2018). In addition, a role for the micronutrient content reduction in the impairment of the photosynthetic machinery can be suggested, given their participation in many electron chain-related reactions, with a particular role of Mn in the oxygen-evolving complex (Raymond and Blankenship, 2008). In the literature, to our knowledge, a role for Bi in interfering with plant nutrient homeostasis was put in evidence by Nagata (2015) and Nishimura and Nagata (2021) for iron. Therefore, in this work, a first report on the possible effect of Bi in altering the nutrient uptake and translocation in plants was shown. In this context, a similar feature about the shoot micronutrient content decrease after metal exposure was reported by Metanat et al. (2019) in plants treated with Pb, a metal belonging to the same chemical group (post-transition metals) of Bi.
In Figure 7, the results of ICP-MS analyses revealed that in both roots and shoots of Bi-exposed plants, a dose-dependent accumulation of Bi to metal concentration in the soil was observed. Bi concentration in roots of Bi-treated plants (Figure 7A) was more than 10-fold higher than in shoots (Figure 7B). A detectable level of Bi was also observed in control plants, likely due to metal uptake from impurities of the peat substrate (data not shown). The low but significant Bi concentrations found in the shoots (Figure 7B) demonstrated that, at least in part, Bi entered the root cells, reaching the vascular tissues to be transported to the aerial parts, possibly through the transpiration flow. As underlined by Babula et al. (2010), the mechanisms of Bi transport in plants are still unknown, and to date, no studies dealing with it have been reported in the literature. Consistently, few papers discussed the accumulation of Bi in plants. An increasing concentration of Bi in garden cress young seedlings to metal concentration in the solution was shown by Passatore et al. (2022). Similarly, Nagata and Kimoto (2020) showed that Bi accumulation occurred in tomato seedlings, probably disturbing iron homeostasis by altering the primary Fe2+ uptake transporter in the roots, as reported for A. thaliana (Nishimura and Nagata, 2021). Low Bi detection in plants grown in soil enriched by Bi pellets was instead found by Fahey et al. (2008). Even if the Bi uptake processes in plants are not fully clarified, it is known that a suite of soil characteristics can affect the uptake of Bi by plants, such as pH, organic matter, cation exchange capacity, and soil texture (Fahey et al., 2008).
FIGURE 7. Bismuth concentration (g Kg−1 dw) in garden cress (Lepidium sativum L.) plants (A = Bi concentration in shoot; B = Bi concentration in roots) grown in pots filled with soil supplied with bismuth nitrate (0 mg Kg−1, control; 30 mg Kg−1; 121 mg Kg−1; 485 mg Kg−1) for 21 days in controlled conditions in growth chamber. In each bar, mean data (n = 4, ±S.E.) are shown. Different letters correspond to statistical different values (Tukey test, p ≤ 0.05).
The bioconcentration factor (BCF) and the translocation factor (Tf) are commonly used indices to evaluate the ability of plant species to accumulate metals in plants and transfer them in the aerial part (Zacchini et al., 2009). In Table 2, BCF values for both shoots and roots highlighted the low capability of garden cress plants to accumulate Bi from the soil, as evidenced in other plant species (Fahey et al., 2008). As markedly depending on both metal concentration and agronomical properties of soil, BCF comparison among different studies is not easy. Anyway, BCF values in above-ground organs for some non-metal-hyperaccumulating plants grown in metal-enriched soils have been reported to be higher than 1 (Wang et al., 2002), even if decreasing with the increase in the metal concentration in soil, as also observed in our experiment. Also, at the root level, a 2 to 10 fold higher BCF compared to that reported in Table 2 has been highlighted for Cd, Cr, Cu, Ni, Pb, and Zn in barley plants grown in soil amended with sludge containing different HM concentrations (Soriano-Disla et al., 2014). Interestingly, the authors reported the root to shoot metal transfer, fully comparable to the Tf shown in Table 2, ranging from 0.14 to 0.010 for the different metals. Therefore, such data evidenced that the Tf of Bi found in our experiments is very similar to that reported for Cr, Ni, and Pb, commonly described as low-mobile elements in plants. Remarkably, in Table 2, Tf values highlighted that the translocation of Bi from roots to shoots is progressively lower with the increase in Bi accumulation in plants. This mechanism could be ascribed to a protective response possibly limiting the harmful action exerted by Bi on the physiological processes occurring in leaves, as observed by Seregin and Kozhevnikova (2006) for other metals and described as a defense response against metal toxicity.
TABLE 2. Bioconcentration factor (shoots and roots) and translocation factor in garden cress (Lepidium sativum L.) plants grown in pots filled with soil supplied with bismuth nitrate (0 mg kg−1, control; 30 mg kg−1; 121 mg kg−1; and 485 mg kg−1) for 21 days in controlled conditions in a growth chamber (mean data; n = 4).
The overall results of this study put in evidence that plants grown in Bi-enriched soil underwent a toxicity status, as revealed by the growth reduction, the lower chlorophyll and carotenoid contents and nitrogen level (NBI), and the decrease in the effective quantum yield of PSII photochemistry (ΦPSII). The reduction in nutrient accumulation in shoots in Bi-treated plants could be associated with these toxic effects, likely affecting the balance within primary metabolism between growth and defense processes. Protective mechanisms were also highlighted, including the overall increase in anthocyan and flavonol levels in leaves, photoprotection mechanisms, and, finally, the restriction of Bi transport from roots to leaves in order to preserve the fundamental physiological processes occurring in leaves. Interestingly, chlorophyll content, NBI, and ΦPSII emerged as valuable proxies of Bi toxicity in plants as their values paralleled the extension of the damaging effects at the growth level. Overall, the results of this study showed that the extent of damage in L. sativum is not related to the Bi concentrations both in soil and plant tissues, providing evidence of the lower toxicity of Bi compared to other metals. Finally, even if some information about the adverse effects of Bi in plants is given in this study, the overall mechanism of Bi phytotoxicity is far from being clarified and requires further studies (Pietrini et al., 2009).
The raw data supporting the conclusion of this article will be made available by the authors, without undue reservation.
FP: conceptualization, methodology, validation, formal analysis, investigation, data curation, and writing—review and editing; LP: investigation, resources, data curation, and visualization; SC: investigation and resources; LM: methodology, validation, formal analysis, and writing—review and editing; MA: methodology, validation, formal analysis, and writing—review and editing; CG: investigation; and MZ: conceptualization, validation, investigation, writing—original draft, writing—review and editing, supervision, and project administration. All authors contributed to the article and approved the submitted version.
This project was funded under the National Recovery and Resilience Plan (NRRP), Mission 4 Component 2 Investment 1.4—Call for tender No. 3138 of 16 December 2021, rectified by Decree n.3175 of 18 December 2021 of the Italian Ministry of University and Research funded by the European Union—NextGenerationEU; Award Number: Project code CN_00000033, Concession Decree No. 1034 of 17 June 2022 adopted by the Italian Ministry of University and Research, CUP B83C22002930006, Project title “National Biodiversity Future Center—NBFC.”
The authors declare that the research was conducted in the absence of any commercial or financial relationships that could be construed as a potential conflict of interest.
All claims expressed in this article are solely those of the authors and do not necessarily represent those of their affiliated organizations, or those of the publisher, the editors, and the reviewers. Any product that may be evaluated in this article, or claim that may be made by its manufacturer, is not guaranteed or endorsed by the publisher.
The Supplementary Material for this article can be found online at: https://www.frontiersin.org/articles/10.3389/fenvs.2023.1221573/full#supplementary-material
Agati, G., Azzarello, E., Pollastri, S., and Tattini, M. (2012). Flavonoids as antioxidants in plants: Location and functional significance. Plant Sci. 196, 67–76. doi:10.1016/j.plantsci.2012.07.014
Agati, G., Brunetti, C., Di Ferdinando, M., Ferrini, F., Pollastri, S., and Tattini, M. (2013). Functional roles of flavonoids in photoprotection: New evidence, lessons from the past. Plant Physiology Biochem. 72, 35–45. doi:10.1016/j.plaphy.2013.03.014
Aggarwal, A., Sharma, I., Tripathi, B. N., Munjal, A. K., Baunthiyal, M., and Sharma, V. (2012). Metal toxicity and photosynthesis Photosynthesis: Overviews on recent progress and future perspectives. New Delhi: I K International Publishing House Pvt, 229–236.
Ai, T. N., Naing, A. H., Yun, B. W., Lim, S. H., and Kim, C. K. (2018). Overexpression of RsMYB1 enhances anthocyanin accumulation and heavy metal stress tolerance in transgenic petunia. Front. Plant Sci. 1388, 1388. doi:10.3389/fpls.2018.01388
Amneklev, J., Augustsson, A., Sörme, L., and Bergbäck, B. (2016). Bismuth and silver in cosmetic products: A source of environmental and resource concern? J. Industrial Ecol. 20 (1), 99–106. doi:10.1111/jiec.12251
Amneklev, J., Sörme, L., Augustsson, A., and Bergbäck, B. (2015). The increase in bismuth consumption as reflected in sewage sludge. Water, Air, & Soil Pollut. 226, 92–11. doi:10.1007/s11270-015-2374-x
APAT-RTI CTN_TES 1/2004 (2004). “Proposal of a technical guidance on analysis methods for soil and contaminated sites,” in Use of Ecotoxicological and Biological Indicators (Proposta di Guida Tecnica su Metodi di Analisi per il Suolo e i Siti Contaminati. Utilizzo di Indicatori Biologici ed ecotossicologici) APAT, Agenzia per la Protezione dell’Ambiente e per i servizi Tecnici (Rome, Italy: Isprambiente), 160.
Apel, K., and Hirt, H. (2004). Reactive oxygen species: Metabolism, oxidative stress, and signal transduction. Annu. Rev. Plant Biol. 55, 373–399. doi:10.1146/annurev.arplant.55.031903.141701
Astolfi, M. L., Marconi, E., Protano, C., and Canepari, S. (2020). Comparative elemental analysis of dairy milk and plant-based milk alternatives. Food control. 116, 107327. doi:10.1016/j.foodcont.2020.107327
Babula, P., Adam, V., Opatrilova, R., Zehnalek, J., Havel, L., and Kizek, R. (2010). “Uncommon heavy metals, metalloids and their plant toxicity: A review,” in Organic farming, pest control and remediation of soil pollutants: Organic farming, pest control and remediation of soil pollutants (Berlin, Germany: Springer), 275–317.
Badrigilan, S., Heydarpanahi, F., Choupani, J., Jaymand, M., Samadian, H., Hoseini-Ghahfarokhi, M., et al. (2020). A review on the biodistribution, pharmacokinetics and toxicity of bismuth-based nanomaterials. Int. J. Nanomedicine 15, 7079–7096. doi:10.2147/ijn.s250001
Baker, N. R., and Rosenqvist, E. (2004). Applications of chlorophyll fluorescence can improve crop production strategies: An examination of future possibilities. J. Exp. Bot. 55, 1607–1621. doi:10.1093/jxb/erh196
Baskar, V., Venkatesh, R., and Ramalingam, S. (2018). Flavonoids (antioxidants systems) in higher plants and their response to stresses. Antioxidants Antioxid. Enzym. High. plants, 253–268. doi:10.1007/978-3-319-75088-0_12
Blackburn, G. A. (1998). Quantifying chlorophylls and carotenoids at leaf and canopy scales: An evaluation of some hyperspectral approaches. Remote Sens. Environ. 66 (3), 273–285. doi:10.1016/s0034-4257(98)00059-5
Cartelat, A., Cerovic, Z. G., Goulas, Y., Meyer, S., Lelarge, C., Prioul, J. L., et al. (2005). Optically assessed contents of leaf polyphenolics and chlorophyll as indicators of nitrogen deficiency in wheat (Triticum aestivum L). Field Crops Res. 91, 35–49. doi:10.1016/j.fcr.2004.05.002
Cerovic, Z. G., Ben Ghozlen, N., Milhade, C., Obert, M., Debuisson, S., and Le Moigne, M. (2015). Non-destructive diagnostic test for nitrogen nutrition of grapevine (Vitis vinifera L) based on Dualex leaf-clip measurements in the field. J. Agric. Food Chem. 63, 3669–3680. doi:10.1021/acs.jafc.5b00304
Cerovic, Z. G., Masdoumier, G., Ben Ghozlen, N., and Latouche, G. (2012). A new optical leaf-clip meter for simultaneous non-destructive assessment of leaf chlorophyll and epidermal flavonoids. Physiol. Plant. 146, 251–260. doi:10.1111/j.1399-3054.2012.01639.x
Chandra, R., and Kang, H. (2016). Mixed heavy metal stress on photosynthesis, transpiration rate, and chlorophyll content in poplar hybrids. For. Sci. Technol. 12 (2), 55–61. doi:10.1080/21580103.2015.1044024
Croft, H., Chen, J. M., Rasiah, R., Levett-Jones, T., and Schneider, J. (2017). Thinking in pharmacy practice: A study of community pharmacists' clinical reasoning in medication supply using the think-aloud method. Reference module earth Syst. Environ. Sci. 6, 1–22. doi:10.3390/pharmacy6010001
da Silva, J. M., Fontes, P. C. R., Milagres, C. D. C., and Garcia Junior, E. (2021). Application of proximal optical sensors to assess nitrogen status and yield of bell pepper grown in slab. J. Soil Sci. Plant Nutr. 21 (1), 229–237. doi:10.1007/s42729-020-00355-2
Das, A. K., Chakraborty, R., Cervera, M. L., and de la Guardia, M. (2006). Analytical techniques for the determination of bismuth in solid environmental samples. TrAC Trends Anal. Chem. 25 (6), 599–608. doi:10.1016/j.trac.2006.01.006
Demmig-Adams, B., and Adams, W. W. (1992). Photoprotection and other responses of plants to high light stress. Annu. Rev. Plant Physiol. Plant Mol. Biol. 43, 599–626. doi:10.1146/annurev.pp.43.060192.003123
Di Baccio, D., Pietrini, F., Bertolotto, P., Pérez, S., Barcelò, D., Zacchini, M., et al. (2017). Response of Lemna gibba L. To high and environmentally relevant concentrations of ibuprofen: Removal, metabolism and morpho-physiological traits for biomonitoring of emerging contaminants. Sci. Tot. Environ. 584, 363–373. doi:10.1016/j.scitotenv.2016.12.191
Elekes, C. C., and Busuioc, G. (2010). The mycoremediation of metals polluted soils using wild growing species of mushrooms. Latest Trends Eng. Educ. 1 (1), 36–39.
Esquivel-Gaon, M., Anguissola, S., Garry, D., Gallegos-Melgar, A. D. C., Saldana, J. M., Dawson, K. A., et al. (2015). Bismuth-based nanoparticles as the environmentally friendly replacement for lead-based piezoelectrics. Rsc Adv. 5 (35), 27295–27304. doi:10.1039/c5ra02151k
Evans, J. R. (1989). Photosynthesis and nitrogen relationships in leaves of C3 plants. Oecologia 78 (1), 9–19. doi:10.1007/bf00377192
Fahey, N. S., Karagatzides, J. D., Jayasinghe, R., and Tsuji, L. J. (2008). Wetland soil and vegetation bismuth content following experimental deposition of bismuth pellets. J. Environ. Monit. 10 (8), 951–954. doi:10.1039/b801535j
Ferdinando, M. D., Brunetti, C., Fini, A., and Tattini, M. (2012). Flavonoids as antioxidants in plants under abiotic stresses. Abiotic stress responses plants, 159–179. doi:10.1007/978-1-4614-0634-1_9
Fu, Z., Wu, F., Amarasiriwardena, D., Mo, C., Liu, B., Zhu, J., et al. (2010). Antimony, arsenic and mercury in the aquatic environment and fish in a large antimony mining area in Hunan, China. Sci. Total Environ. 408 (16), 3403–3410. doi:10.1016/j.scitotenv.2010.04.031
Goulas, Y., Cerovic, Z. G., Cartelat, A., and Moya, I. (2004). Dualex: A new instrument for field measurements of epidermal ultraviolet absorbance by chlorophyll fluorescence. Appl. Opt. 43, 4488–4496. doi:10.1364/ao.43.004488
Guidi, L., Tattini, M., and Landi, M. (2017). How does chloroplast protect chlorophyll against excessive light. Chlorophyll 21, 21–36. doi:10.5772/67887
Hideg, E., Kós, P. B., and Schreiber, U. (2008). Imaging of NPQ and ROS formation in tobacco leaves: Heat inactivation of the water-water cycle prevents down-regulation of PSII. Plant Cell Physiol. 49 (12), 1879–1886. doi:10.1093/pcp/pcn170
Huang, Y., Lai, J., Huang, Y., Luo, X., Yang, X., Liu, Z., et al. (2022). Response mechanism of Chlamydomonas reinhardtii to nanoscale bismuth oxyiodide (nano-BiOI): Integrating analysis of mineral nutrient metabolism and metabolomics. J. Environ. Sci. 121, 13–24. doi:10.1016/j.jes.2021.09.008
ISO/DIS 20079 (2004). Water quality –determination of the toxic effect of water constituents and waste water to duckweed (Lemna minor)–Duckweed growth inhibition test. Geneva, Switzerland: ISO TC 147/SC 5/WG 5.
Kalefetoğlu Macar, T., and Ekmekci, Y. (2009). Alterations in photochemical and physiological activities of chickpea (Cicer arietinum L) cultivars under drought stress. J. Agron. Crop Sci. 195 (5), 335–346. doi:10.1111/j.1439-037x.2009.00374.x
Kearns, J., and Turner, A. (2016). An evaluation of the toxicity and bioaccumulation of bismuth in the coastal environment using three species of macroalga. Environ. Pollut. 208, 435–441. doi:10.1016/j.envpol.2015.10.011
Khan, M., Nawaz, N., Ali, I., Azam, M., Rizwan, M., Ahmad, P., et al. (2019). Regulation of photosynthesis under metal stress. Photosynth. Prod. Environ. Stress, 95–105. doi:10.1002/9781119501800.ch6
Klughammer, C., and Schreiber, U. (2008). Complementary PS II quantum yields calculated from simple fluorescence parameters measured by PAM fluorometry and the Saturation Pulse method. Pam. Appl. Notes 1, 27–35.
Kramer, D. M., Johnson, G., Kiirats, O., and Edwards, G. E. (2004). New fluorescence parameters for the determination of QA redox state and excitation energy fluxes. Photosyn. Res. 79, 209–218. doi:10.1023/b:pres.0000015391.99477.0d
Kummerová, M., Krulová, J., Zezulka, Š., and Tříska, J. (2006). Evaluation of fluoranthene phytotoxicity in pea plants by Hill reaction and chlorophyll fluorescence. Chemosphere 65 (3), 489–496. doi:10.1016/j.chemosphere.2006.01.052
Lambrev, P. H., Miloslavina, Y., Jahns, P., and Holzwarth, A. R. (2012). On the relationship between non-photochemical quenching and photoprotection of photosystem II. Biochim. Biophys. Acta 1817, 760–769. doi:10.1016/j.bbabio.2012.02.002
Legrand, M., McConnell, J. R., Bergametti, G., Preunkert, S., Chellman, N., Desboeufs, K., et al. (2023). Alpine-ice record of bismuth pollution implies a major role of military use during World War II. Sci. Rep. 13 (1), 1166. doi:10.1038/s41598-023-28319-3
Malinská, H., Pidlisnyuk, V., Nebeská, D., Erol, A., Medžová, A., and Trögl, J. (2020). Physiological response of Miscanthus x giganteus to plant growth regulators in nutritionally poor soil. Plants 9, 194. doi:10.3390/plants9020194
Massimi, L., Wesseling, J., van Ratingen, S., Javed, I., Frezzini, M. A., Astolfi, M. L., et al. (2021). Identification and spatial mapping of tracers of PM10 emission sources using a high spatial resolution distributed network in an urban setting. Atmos. Res. 262, 105771. doi:10.1016/j.atmosres.2021.105771
Maxwell, K., and Johnson, G. N. (2000). Chlorophyll fluorescence—a practical guide. J. Exp. Bot. 51, 659–668. doi:10.1093/jexbot/51.345.659
Metanat, K., Ghasemi-Fasaei, R., Ronaghi, A., and Yasrebi, J. (2019). Lead phytostabilization and cationic micronutrient uptake by maize as influenced by Pb levels and application of low molecular weight organic acids. Commun. Soil Sci. Plant Analysis 50 (15), 1887–1896. doi:10.1080/00103624.2019.1648493
Moustaka, J., Ouzounidou, G., Sperdouli, I., and Moustakas, M. (2018). Photosystem II is more sensitive than photosystem I to Al3+ induced phytotoxicity. Mater. (Basel) 11, 1772. doi:10.3390/ma11091772
Moustakas, M., Calatayud, Á., and Guidi, L. (2021). Editorial: Chlorophyll fluorescence imaging analysis in biotic and abiotic stress. Front. Plant Sci. 12, 658500. doi:10.3389/fpls.2021.658500
Murata, T. (2006). Effects of bismuth contamination on the growth and activity of soil microorganisms using thiols as model compounds. J. Environ. Sci. Health, Part A 41 (2), 161–172. doi:10.1080/10934520500349276
Nagata, T. (2015). Growth inhibition and IRT1 induction of Arabidopsis thaliana in response to bismuth. J. Plant Biol. 58, 311–317. doi:10.1007/s12374-015-0256-9
Nagata, T., and Kimoto, S. (2020). Growth inhibition and root damage of bismuth in Solanum lycopersicum. SCIREA J. Biol. 54, 72–86.
Nishimura, M., and Nagata, T. (2021). Phytotoxicity and iron homeostasis disturbance of bismuth on IRT1 promoter knockout Arabidopsis thaliana. Plant 9 (3), 48. doi:10.11648/j.plant.20210903.12
OECD (2006b). Test No. 208: Terrestrial plant test: Seedling emergence and seedling growth test. OECD Guidel. Test. Chem. Section 2.
Omouri, Z., Hawari, J., Fournier, M., and Robidoux, P. Y. (2018). Bioavailability and chronic toxicity of bismuth citrate to earthworm Eisenia andrei exposed to natural sandy soil. Ecotoxicol. Environ. Saf. 147, 1–8. doi:10.1016/j.ecoenv.2017.08.018
Omouri, Z., Jalal, H., Michel, F., and Pierre, Y. R. (2019). phytotoxicity of bismuth nitrate and bismuth citrate on germination and growth of lolium perenne exposed on filter paper and on artificially contaminated soil. J. Soil Sci. Plant Health 3 (1).
Pandey, S. N. (2018). Biomolecular functions of micronutrients toward abiotic stress tolerance in plants. Plant Nutrients Abiotic Stress Toler., 153–170. doi:10.1007/978-981-10-9044-8_6
Passatore, L., Pietrini, F., Carloni, S., Massimi, L., Giusto, C., Zacchini, M., et al. (2022). Morpho-physiological and molecular responses of Lepidium sativum L. seeds induced by bismuth exposure. Sci. Total Environ. 831, 154896. doi:10.1016/j.scitotenv.2022.154896
Peñuelas, J., and Filella, I. (1998). Visible and near-infrared reflectance techniques for diagnosing plant physiological status. Trends Plant Sci. 3 (4), 151–156. doi:10.1016/s1360-1385(98)01213-8
Pietrini, F., Di Baccio, D., Iori, V., Veliksar, S., Lemanova, N., Juškaitė, L., et al. (2017). Investigation on metal tolerance and phytoremoval activity in the poplar hybrid clone “Monviso” under Cu-spiked water: Potential use for wastewater treatment. Sci. Total Environ. 592, 412–418. doi:10.1016/j.scitotenv.2017.03.101
Pietrini, F., Iannilli, V., Passatore, L., Carloni, S., Sciacca, G., Cerasa, M., et al. (2022). Ecotoxicological and genotoxic effects of dimethyl phthalate (DMP) on Lemna minor L. and Spirodela polyrhiza (L) Schleid. plants under a short-term laboratory assay. Sci. Total Environ. 806, 150972. doi:10.1016/j.scitotenv.2021.150972
Pietrini, F., Iori, V., Cheremisina, A., Shevyakova, N. I., Radyukina, N., Kuznetsov, V. V., et al. (2015). Evaluation of nickel tolerance in Amaranthus paniculatus L. plants by measuring photosynthesis, oxidative status, antioxidative response and metal-binding molecule content. Environ. Sci. Pollut. Res. 22, 482–494. doi:10.1007/s11356-014-3349-y
Pietrini, F., Iori, V., Pietrosanti, L., Zacchini, M., and Massacci, A. (2020). Evaluation of multiple responses associated with arsenic tolerance and accumulation in Pteris vittata L. Plants exposed to high as concentrations under hydroponics. Water 12 (11), 3127. doi:10.3390/w12113127
Pietrini, F., Passatore, L., Carloni, S., and Zacchini, M. (2023). “Non-standard physiological endpoints to evaluate the toxicity of emerging contaminants in aquatic plants: A case study on the exposure of Lemna minor L. and Spirodela polyrhiza (L.) schleid. To dimethyl phthalate (dmp),” in Emerging contaminants and plants: Interactions, adaptations and remediation technologies (Cham: Springer International Publishing), 87–108.
Pietrini, F., Zacchini, M., Iori, V., Pietrosanti, L., Bianconi, D., and Massacci, A. (2009). Screening of poplar clones for cadmium phytoremediation using photosynthesis, biomass and cadmium content analyses. Int. J. Phytoremediation 12 (1), 105–120. doi:10.1080/15226510902767163
Prabhavati, E., Vishnuvardhan, Z., and Mallaiah, K. V. (2017). Photosynthetic ability of Horse gram [Macrotyloma uniflorum (Lam) Verdc] plants in response to metal stress. Russ. Agric. Sci. 43, 144–148. doi:10.3103/s1068367417020148
Raymond, J., and Blankenship, R. E. (2008). The origin of the oxygen-evolving complex. Coord. Chem. Rev. 252 (3-4), 377–383. doi:10.1016/j.ccr.2007.08.026
Roach, T., Na, C. S., Stöggl, W., and Krieger-Liszkay, A. (2020). The non-photochemical quenching protein LHCSR3 prevents oxygen-dependent photoinhibition in Chlamydomonas reinhardtii. J. Exp. Bot. 71 (9), 2650–2660. doi:10.1093/jxb/eraa022
Rohr, O. (2002). Bismuth–the new ecologically green metal for modern lubricating engineering. Industrial Lubr. Tribol. 54 (4), 153–164. doi:10.1108/00368790210431709
Sage, R. F., Pearcy, R. W., and Seemann, J. R. (1987). The nitrogen use efficiency of C3 and C4 plants III. Leaf nitrogen effects on the activity of carboxylating enzymes in Chenopodium album (L) and Amaranthus retroflexus (L). Plant Physiol. 85 (2), 355–359. doi:10.1104/pp.85.2.355
Salt, D. E., Baxter, I., and Lahner, B. (2008). Ionomics and the study of the plant ionome. Annu. Rev. Plant Biol. 59, 709–733. doi:10.1146/annurev.arplant.59.032607.092942
Seregin, I. V., and Kozhevnikova, A. D. (2006). Physiological role of nickel and its toxic effects on higher plants. Russ. J. Plant Physiology 53, 257–277. doi:10.1134/s1021443706020178
Shomali, A., Arif, S., Sarraf, N., Zahra, M., Yadav, N. V., Aliniaeifard, S., et al. (2022). Diverse physiological roles of flavonoids in plant environmental stress responses and tolerance. Plants 11, 3158. doi:10.3390/plants11223158
Smirnoff, N. (1993). The role of active oxygen in the response of plants to water deficit and desiccation. New Phytol. 125, 27–58. doi:10.1111/j.1469-8137.1993.tb03863.x
Soriano-Disla, J. M., Gómez, I., Navarro-Pedreño, J., and Jordán, M. M. (2014). The transfer of heavy metals to barley plants from soils amended with sewage sludge with different heavy metal burdens. J. Soils Sediments 14 (4), 687–696. doi:10.1007/s11368-013-0773-4
Sudina, L., Kolesnikov, S., Minnikova, T., Kazeev, K., Sushkova, S., and Minkina, T. (2021). Assessment of ecotoxicity of the bismuth by biological indicators of soil condition. Eurasian J. Soil Sci. 10 (3), 236–242. doi:10.18393/ejss.926759
Sun, T., Rao, S., Zhou, X., and Li, L. (2022). Plant carotenoids: Recent advances and future perspectives. Mol. Hortic. 2 (1), 3. doi:10.1186/s43897-022-00023-2
Testone, G., Baldoni, E., Iannelli, M. A., Nicolodi, C., Di Giacomo, E., Pietrini, F., et al. (2019). Transcription factor networks in leaves of Cichorium endivia: New insights into the relationship between photosynthesis and leaf development. Plants 8 (12), 531. doi:10.3390/plants8120531
Tripathi, D. K., Singh, V. P., Chauhan, D. K., Prasad, S. M., and Dubey, N. K. (2014). Role of macronutrients in plant growth and acclimation: Recent advances and future prospective. Improv. Crops Era Clim. Changes 2, 197–216. doi:10.1007/978-1-4614-8824-8_8
Udalova, T. A., Logutenko, O. A., Timakova, E. V., Afonina, L. I., Naydenko, E. S., and Yukhin, Y. M. (2008). “Bismuth compounds in medicine,” in Third international Forum on strategic technologies (New York: IEEE), 137–140.
Wang, Q. R., Liu, X. M., Cui, Y. S., Dong, Y. T., and Christie, P. (2002). Responses of legume and non-legume crop species to heavy metals in soils with multiple metal contamination. J. Environ. Sci. Health, Part A 37 (4), 611–621. doi:10.1081/ese-120003241
Wang, R., Li, H., Sun, H., Dong, X. Y., Anenberg, E., Jiang, P. F., et al. (2019). A negative regulator of synaptic development: MDGA and its links to neurodevelopmental disorders. Encycl. Environ. Health 15, 415–421. doi:10.1007/s12519-019-00253-3
Wei, C., Deng, Q., Wu, F., Fu, Z., and Xu, L. (2011). Arsenic, antimony, and bismuth uptake and accumulation by plants in an old antimony mine, China. Biol. trace Elem. Res. 144, 1150–1158. doi:10.1007/s12011-011-9017-x
Keywords: environmental pollution, heavy metals, metal toxicity, photosynthesis, pigments, trace elements
Citation: Pietrini F, Passatore L, Carloni S, Massimi L, Astolfi ML, Giusto C and Zacchini M (2023) Bismuth exposure affects morpho-physiological performances and the ionomic profile in garden cress (Lepidium sativum L.) plants. Front. Environ. Sci. 11:1221573. doi: 10.3389/fenvs.2023.1221573
Received: 12 May 2023; Accepted: 19 June 2023;
Published: 29 June 2023.
Edited by:
Baile Xu, Free University of Berlin, GermanyCopyright © 2023 Pietrini, Passatore, Carloni, Massimi, Astolfi, Giusto and Zacchini. This is an open-access article distributed under the terms of the Creative Commons Attribution License (CC BY). The use, distribution or reproduction in other forums is permitted, provided the original author(s) and the copyright owner(s) are credited and that the original publication in this journal is cited, in accordance with accepted academic practice. No use, distribution or reproduction is permitted which does not comply with these terms.
*Correspondence: Massimo Zacchini, bWFzc2ltby56YWNjaGluaUBjbnIuaXQ=
Disclaimer: All claims expressed in this article are solely those of the authors and do not necessarily represent those of their affiliated organizations, or those of the publisher, the editors and the reviewers. Any product that may be evaluated in this article or claim that may be made by its manufacturer is not guaranteed or endorsed by the publisher.
Research integrity at Frontiers
Learn more about the work of our research integrity team to safeguard the quality of each article we publish.