- 1Center for Marine Environmental Studies (CMES), Ehime University, Matsuyama, Ehime, Japan
- 2Graduate School of Science and Engineering, Ehime University, Matsuyama, Ehime, Japan
- 3Faculty of Engineering, Tohoku University, Sendai, Japan
- 4Disaster Prevention Research Institute, Kyoto University, Uji, Kyoto, Japan
Microbial communities in the hyporheic zone are important for self-purification as the riverbed is metabolically active and responsible for the retention, storage, and mineralisation of organic matter transported in the surface water. Studies exploring the response of the hyporheic microbial community to flow regime alterations remain scarce. Here, we characterised the microbial community structure over downwelling, upwelling and intermediate hyporheic zone areas within and among gravel bars at high and low discharge levels to determine flow-induced changes in a dam-regulated river using 16S rRNA gene sequencing. At low discharge, microbial composition varied across the different areas of gravel bars, reflecting heterogeneity in local abiotic conditions. However, high discharge lead to microbial community homogenisation across the three areas of the gravel bars. Microbial communities across adjacent gravel bars remained dissimilar regardless of discharge levels, likely reflecting differences in bar morphology. Our results can help better understand how anthropogenic changes in hydrologic conditions can affect microbial diversity and function in the hyporheic zone. This knowledge will help us to understand better how changes in hydrological conditions affect ecosystem functions and services in rivers affected by human impacts.
Introduction
Rivers are dynamic environments and examining how flooding affects community structure has long been an important research topic in disturbance ecology (Graham et al., 2021). However, previous studies focused only on surface water organisms such as microbiota, fishes, and macroinvertebrates (Dole-Olivier et al., 1997; Calderon et al., 2017; Miyake et al., 2021). Limited studies were done on organisms in the hyporheic zone. In riverine ecosystems, the hyporheic zone in gravel bars is essential in providing habitat and refuge for microbial communities (Boulton and Stanley, 1995; Boulton et al., 2010; Boano et al., 2014; Harjung et al., 2019) and in maintaining healthy waterways (Lewandowski et al., 2019). This hyporheic flow carries different materials with it and other microbial communities, removing water pollutants and natural solutes.
In the hyporheic zone, environmental conditions can vary widely between areas of downwelling zone (surface water movement toward the groundwater) at the bar head to the upwelling zone (groundwater movement toward the surface water) at the bar tail of the gravel bar water flows into the hyporheic zone (Hendricks, 1993). Increased discharge in a river, i.e., dam-regulated discharge, can increase hydraulic pressure on the riverbed, potentially increasing the downwelling flow from the surface water to the hyporheic zone, or the flow velocity of the normally slow-flowing hyporheic water. Such changes in the hydrologic conditions could result in hyporheic zone community disturbance during flooding. If a heterogeneous community structure is formed along the hyporheic zone path of gravel bar under low discharge conditions, a disturbance occurring under high river surface water discharge, such as a flood, could homogenise microbial communities. To test this hypothesis, the composition and community structure of microbial communities should be compared within and across gravel bars, during periods of contrasting streamflow levels. Limited studies focused on the effects of low regulation on microbial diversity in the hyporheic zone. One study focused on the altered flow regime as disturbance ecology (Bruno, et al., 2023). However, this study did not focus on differences in microbial community structure across different areas of hyporheic gravel bars.
Previous studies used molecular approaches to investigate how the hyporheic flow path influences microbial communities in hyporheic zones in gravel bars (Doering et al., 2021). For example, one study demonstrated microbial communities were spatially diverse along a 100-m hyporheic flow path with large environmental heterogeneity such as chemicals and nutrients using polymerase chain reaction-denaturing gel electrophoresis (PCR-DGGE) (Lowell et al., 2009). One study how vertical flow direction (i.e., upwelling/downwelling) affect the microbial community and identified different microbial community compositions between the upwelling/downwelling zones using pyrosequencing (Kim and Lee, 2019). Our study focused on microbial community diversity in response to altered flow disturbance in different areas of hyporheic zone in gravel bars using 16S rRNA gene sequencing.
Understanding the functional traits of each species could contribute to deepening our knowledge of the biological processes and ecological mechanisms that shape community structure variation along environmental gradients. Recent studies on freshwater microbial communities also estimated functional traits based on taxonomic annotation using databases (e.g., SILVA rRNA, Greengenes, National Center for Biotechnology, and Ribosomal Database Project) and 16S rRNA gene sequencing (Galand et al., 2018; Fasching et al., 2020; Serrana et al., 2022). Several studies have demonstrated the validity and utility of this approach, although the estimated functional traits were putative as 16S rRNA gene sequencing could not identify functional genes in the genome, and database limitations allowed annotating only a proportion of the taxa. However, applied cases are still scarce and further verifications would be required.
Our study examined the effects of flow regulation as a disturbance for the hyporheic microorganisms. In particular, we aimed to 1) profile the microbial diversity and community structure among hyporheic zones at three hyporheic, i.e., downwelling, upwelling, and intermediate, areas of gravel bars, 2) identify the microbial community structure-influencing environmental factors among the hyporheic zones areas, 3) compare the divergence levels of the microbial communities among the hyporheic areas under low and high discharge conditions of the river surface water, and 4) identify the putative metabolic functions of the microbial communities on these sites under different discharge levels. This study can provide valuable insights into the role of spatial environmental heterogeneity in the biological processes and ecological mechanisms that govern the local adaptation of microbial communities at taxonomic and functional levels in gravel bar hyporheic zones.
Materials and methods
Study site and water sample collection
The Tenryu River is located in central Honshu, Japan, with a length and basin area of 213 km and 5,090 km2, respectively. The river originates from Lake Suwa in Nagano Prefecture and is discharged into the Pacific Ocean (Figure 1). The Funagira Dam (34°53′26′N, 137°48′54′) is the last major dam to be completed on the Tenryu River supplying water to the nearby Funagira Hydroelectric Power Station. The dam releases different water discharge levels throughout the year, thereby altering the water flow discharge of the river.
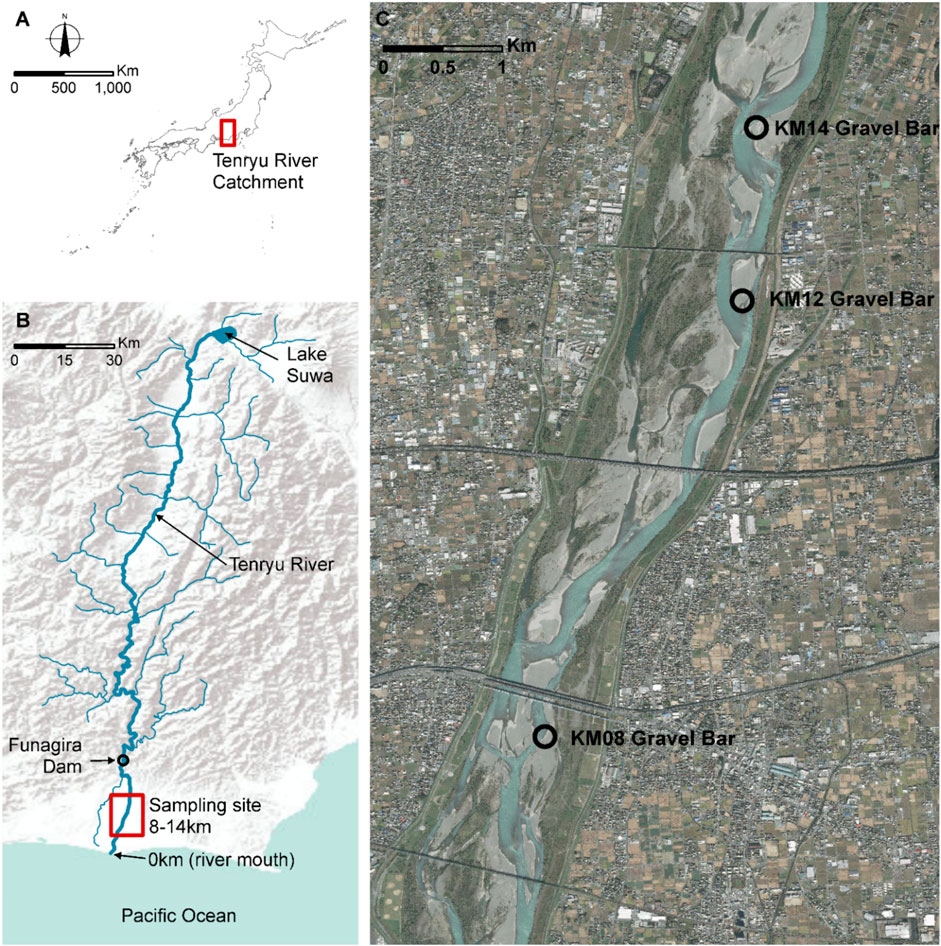
FIGURE 1. Detailed map of the sampling sites (A) The top panel shows the contour of the map of Japan indicating the Tenryu River. (B) The figure shows the location of Lake Suwa where the river originates and Funagira Dam over Esri elevation model. (C) Google map aerial photo of the three gravel bars (KM08, KM12 and KM14) from the river mouth.
Visual inspection of sampling sites was done before the actual field survey. Field surveys were conducted between November 13–17, 2019. The mean dam discharge was high (250 m3/s) and low (150 m3/s) between November 13–15 and 16–17, 2019, respectively. During the field survey, flow fluctuations reduced the water level to 80 cm, thereby changing gravel bar morphology. Three gravel bars located 8, 12, and 14 km (KM08, KM12 and KM14, respectively) from the river mouth were selected in this study. These three large gravel bars are distributed adjacent to each other within 6 km of the middle reach of the Tenryu River, with relatively similar environmental conditions. We collected and measured water samples and environmental parameters at three sampling areas among each gravel bar site. The downwelling area can be defined as the area at the upper side of gravel bar where surface water enters the hyporheic zone, the upwelling area can be defined as the area at the lower side of gravel bar where hyporheic water upwells. The intermediate area is located on the between the down area and the up area.
However, no sample was collected from the intermediate area of gravel bar KM14 as no hyporheic water was collected 20 cm from the ground. In total, we assessed 16 sampling areas, n = 8 both at low and high discharges, in this study and collected water samples in triplicates for each sampling area (a total of 48 water samples).
A 1,220-mm-long solid steel cone piezometer (AXEL, Japan) was used to collect water samples from a depth of 20 cm from the ground surface at each hyporheic area. Hyporheic water samples of 250–500 mL was filtered using a 50-mL sterile syringe (Terumo) and 0.22-μm Sterivex™ filters (Merck Millipore, Merck KgaA, Darmstadt, 346 Germany) on-site. A negative field control sample was produced by filtering 1 L of ddH2O through a Sterivex™ filter, similar to the field samples. The filtered samples were preserved in 99% molecular-grade ethanol, brought to the laboratory, and stored at −20°C until processing for molecular analysis.
Water quality parameters, i.e., pH, electrical conductivity (EC), water temperature and turbidity, were recorded in situ. pH, EC, and water temperature were measured with a multi-parameter water quality checker (HORIBA, D-54, Japan). Turbidity was measured with a portable turbidity metre (TB-31, DKK-TOA, Japan). Suspended solids (SS) were collected by filtering 0.5 litres of hyporheic river water, passing it through a 1-mm sieve onto a GF/F filter (pore size: 0.7 μm), drying (105°C, 4°h) and weighing on an electronic balance. Anions (F-, Cl-, NO3-N, SO42-) were measured using ion chromatography (Metrohm, Basic IC plus 883, Swiss). Inorganic elements (Mg, Al, and Na) were measured using an inductively coupled plasma mass spectrometer (Agilent Technologies, Agilent8800 ICP-QQQ, Japan).
DNA extraction, library preparation and amplicon sequencing
Total genomic DNA was extracted from each filter using the Qiagen PowerSoil DNA Isolation Kit (Qiagen, Hilden, Germany) following the manufacturer’s protocol. DNA quality and concentration were determined using a NanoDrop spectrophotometer (Thermo Scientific Nanodrop 2,000), and the QuantiFluor dsDNA System (Promega, Madison, United States). The amplicon library was prepared through a one-step PCR protocol using modified primer sequences targeting the V4-V5 hypervariable region of the 16S SSU rRNA gene from the Earth Microbiome Protocol (Caporaso, et al., 2012). The forward sequence is 515F AATGATACGGCGACCACCGAGATCTACACGCT and the reverse primer sequence was 926R CAAGCAGAAGACGGCATACGAGATAGTCAGCCAGGGCCGYCAATTYMTTTRAGTTT. The PCR was performed using a T100 Thermal Cycler (Bio-Rad Laboratories, United States) and Phusion high-fidelity DNA polymerase (New England, Biolabs) for the PCR amplification. The 15-μL PCR reaction mixture consisted of 3 μL of 5X Phusion GC Buffer, 0.5 μL each of both the forward and reverse primers (10 μM), 0.6 μL dNTPs (2.5 mM), 0.45 μL DMSO, 0.3 μL Phusion Polymerase (1U), and 1 μL of template DNA at a concentration of 5 ng/μL. The PCR cycling conditions were as follows: initial denaturation at 98°C for 3 min, 25 cycles of denaturation, annealing, and extension at 98°C for 15 s, 67°C for 30 s, and 72°C for 30 s, respectively, followed by a final extension cycle at 72°C for 5 min. The amplicon size was 420 bp. Two negative controls, i.e., field control sample filtered from sterile water and nuclease-free water were included to monitor the potential contamination from DNA extraction to PCR amplification. A total of 50 amplicon libraries (i.e. 2 negative controls, and triplicates of 16 areas) were constructed. The PCR products were verified on a 2% agarose gel using gel electrophoresis. Next, we quantified the concentration of each PCR product using the KAPA Illumina Library qPCR Quantification kit (Kapa Biosystems, Wilmington, MA, United States). Equimolar concentrations of each amplicon library were then pooled. The pooled samples were purified and size-selected using solid-phase reversible immobilisation beads (Beckman Coulter, Inc. CA). We used the Agilent Bioanalyzer 2100 system to assess the quality of the pooled library by the obtention of a single clear band of 420 bp. Sequencing was conducted on an Illumina Miseq (Illumina, Inc. San Diego, CA, United States) using a v3 Miseq sequencing kit (300 × 2, MS-102-3003) with 30% PhiX (Illumina, Inc. San Diego, CA, United States) and a starting concentration of 4 nM denatured to a final concentration of 8pm.
Read processing and taxonomic assignment
Raw paired-end reads were verified for quality using FastQC v0.11.8 (Andrews, 2010). The raw reads were demultiplexed using the QIIME v2018.11 package (Boylen et al., 2019). Demultiplexed sequence data were then quality screened, processed and denoised using the DADA2 pipeline v1.16 package (Callahan et al., 2016). The reads were quality-filtered and truncated into 100-bp fragments. The chimeric sequences and singletons were also removed. Based on the read error files, the reverse reads displayed poor read quality. Therefore, only the forward reads were processed for downstream analyses. Amplicon sequence variants (ASVs) were inferred from the sequence data using the DADA2 pipeline v1.16 package. Taxonomic ASV identification was performed against the SILVA SSU database v132 using the SILVA ACT (www.arb-silva.de/aligner) (Pruesse et al., 2012). Subsequent analyses were performed at the ASV-level.
Statistical analyses and visualisation
All statistical analyses and visualisations were performed using the R software v4.0.1 (R Core Team, 2018). Read number normalisations were performed in each sample using median sequencing depth before the analyses as an alternative to total counts because it is less influenced by most highly abundant taxa, while not disregarding rare taxa. Species diversity at each area (alpha diversity) was determined using Chao1 richness (Chao, 1984), Shannon diversity (Shannon, 1949), and Simpson diversity (Simpson, 1949) via the plot_richness command in the phyloseq package (McMurdie and Holmes, 2015). The mean alpha diversity values for each replicate in the hyporheic areas were computed. Analysis of variance (ANOVA) was conducted via phyloseq to test whether the mean alpha diversities were significantly different among the three or two areas per gravel bars. Differences in microbial composition among areas of the gravel bars were computed using Bray–Curtis dissimilarity (Bray and Curtis, 1957) in vegan package, and visualised by non-metric multidimensional scaling (NMDS) using the plot_ordination function in the phyloseq package (Dixon, 2003). Permutational multivariate analysis of variance (PERMANOVA) was measured using the phyloseq package to test potential significant differences between areas per gravel bars (Anderson et al., 2008). Distance-based redundancy analysis (dbRDA) was performed to determine the environmental factors influencing the microbial community structure among the hyporheic areas and visualised via the cca function of the vegan package. We identified the putative metabolic functions using the functional annotations for the prokaryotic (FAPROTAX) v1.2.3 (Louca et al., 2016; Louca and Doepeli, 2017) database. The mantel test (Legendre and Legendre, 2012) was conducted via the mantel.correlog function with 9.999 permutations to test for significant correlations between beta diversity based on taxonomic structure among the areas and that based on the putative metabolic function structure.
Results
Microbial diversity and community composition
We generated a total of 15,346,131 raw reads from the 50 amplicon libraries. The reads one and six of the two negative controls were removed for subsequent analyses. After quality filtering, chimeric sequence removal and denoising, we retained a total of 5,439,168 reads (Supplementary Table S1). Out of the inferred 19,592 ASVs, only 5,453 ASVs exhibited genus level, and from the latter, 260 ASVs species-level assignments. At the genus level, Flavobacterium (440,130 reads, 21% of the total number of reads assigned to genera) was the most abundant among the three different areas followed by Methylobacter (115,342 reads, 6%), Sediminibacterium (92,722 reads, 5%), and Rhodoferax (88,531 reads, 4%) (Supplementary Figure S1C). Proteobacteria was the most abundant phylum (1,321,906 reads, 33% of total numbers of reads assigned to phyla), followed by Bacteriodiota (1,311,523 reads, 32%), Verrucomicrobiota (269,793 reads, 7%), Acidobacteria (217,836 reads, 6%), and Actinobacteria (60,563 reads, 2%) (Supplementary Figure S1D).
In each gravel bar, the NMDS ordination (Figure 2A) showed clustering of the three hyporheic areas within the gravel bars at low discharge while such clustering could not be observed at high discharge using ASV-level (Figure 2C). PERMANOVA (Table 1) showed significant dissimilarity in the community structure among the three areas at low discharge (p = 0.004) but no significant difference at high discharge (p = 0.326). The NMDS results (Figures 2B, D) of the three gravel bars showed clustered patterns under both high and low discharge conditions. PERMANOVA revealed significant community divergence among the three gravel bars at low (p = 0.005) and high (p = 0.001) discharge (Table 2).
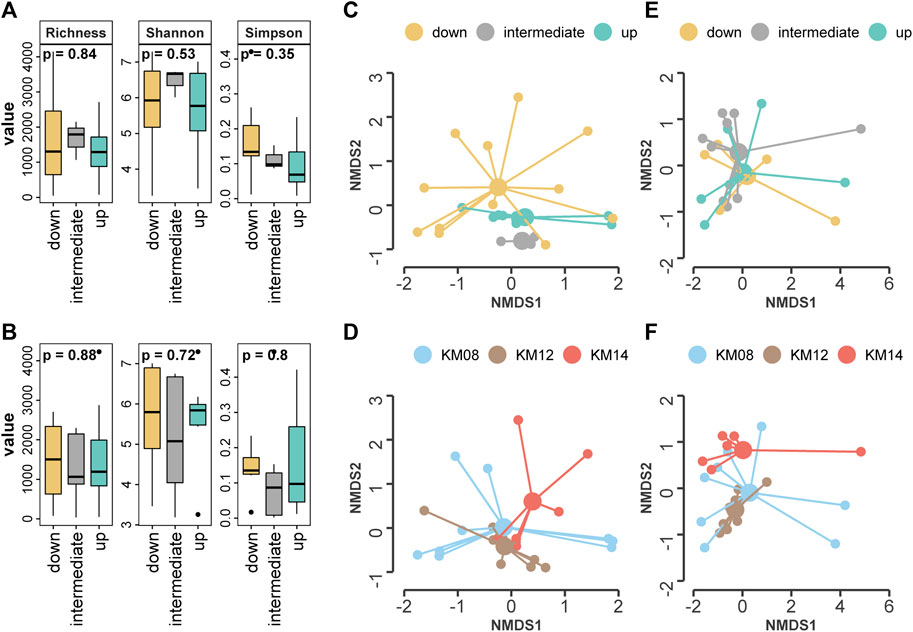
FIGURE 2. Non-multidimensional scaling (NMDS) showing the microbial community structure in (A) low discharge by hyporheic area (B) low discharge by gravel bar (C) high discharge by hyporheic area (D) high discharge by gravel bar. Box plot showing the alpha diversity per hyporheic area (i.e., downwelling, upwelling and intermediate) (E) Alpha diversity in low discharge (F) Alpha diversity in high discharge.
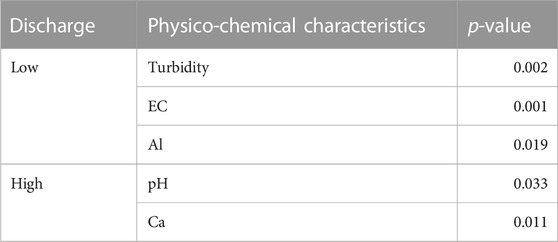
TABLE 1. Distance-based redundancy (dbRDA) analysis shows the environmental factors affecting the microbial community structure for each gravel bar in two discharges levels.
The alpha diversity chao1 richness, Shannon, and Simpson indices ranged between 35 and 4023, 3.18–7.33, and 0.002–0.471, respectively, among the 16 areas based on the ASV-level (Supplementary Table S2). We observed no significant difference between the alpha metrics between the areas within gravel bars under both low and high discharge conditions (Figures 2E, F).
The dbRDA results (Figures 3A, B) showed that turbidity (p = 0.002), EC (p = 0.001), and Al (p = 0.019) significantly influenced the microbial community structure as environmental factors at low discharge, while pH (p = 0.033) and Ca (p = 0.011) were the environmental factors influencing microbial communities at high discharge (Table 3).
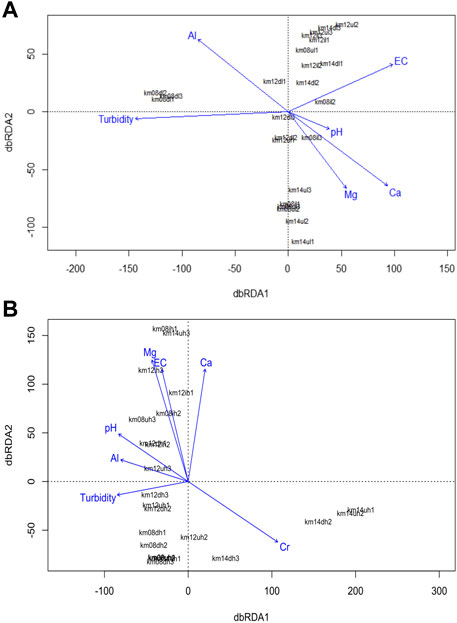
FIGURE 3. The dbRDA plot showing the significant environmental factors influencing microbial community: (A) dbRDA in low discharge (B) dbRDA in high discharge.
Putative metabolic function of the hyporheic microbial communities
A total of 2,962 ASVs (15.12%) out of 5,453 ASVs were assigned to at least one putative metabolic function. We identified 67 out of 92 putative metabolic functions in the FAPROTAX database. Chemoheterotrophy and aerobic chemoheterotrophy obtained annotated putative metabolic function throughout the 16 areas (Supplementary Figure S2). Methanotrophy and methylotrophy were described as dominant in the downwelling areas while methanogenesis was dominant in the upwelling areas both at low and high discharge. Aerobic ammonia oxidation was the most abundant putative metabolic function identified at the intermediate area. We also described a significant positive correlation between beta diversity based on community structure and putative metabolic function structure (Figure 4).
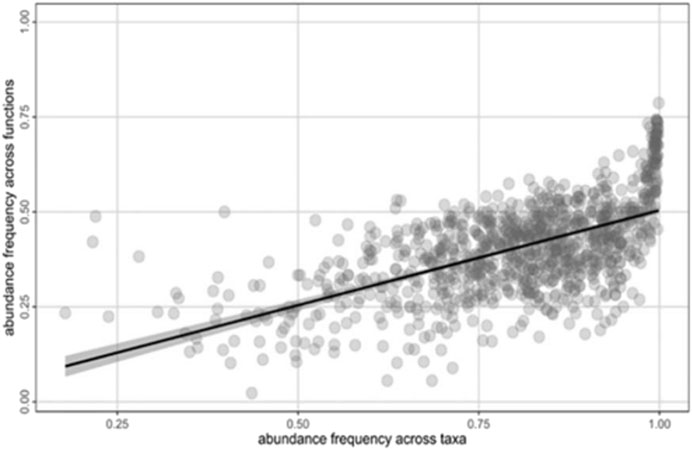
FIGURE 4. Mantel test analysis of abundance frequency based on taxa β-diversity versus abundance frequency based on putative metabolic functions using the Bray-Curtis index.
Discussion
This study aimed to contrast the microbial community structure and putative metabolic function among the hyporheic, i.e., downwelling, upwelling, and intermediate areas within and among gravel bars between two (i.e., low and high) discharge levels of a dam-regulated river.
Community divergence among the three sampling areas
Our results revealed significant microbial community divergence among the three areas within the gravel bars under low discharge conditions. This could be attributed to local adaptation reflecting environmental heterogeneity among hyporheic areas within the gravel bar at low discharge. Moreover, we observed significant community divergence between 2-km distance gravel bars that remained even under high discharge conditions. However, we could not observe any significant divergence within the gravel bar areas under high discharge condition. The observed differences between the gravel bars could be attributed to their bar geomorphology and sediment characteristics (Ock et al., 2015). Since microorganisms are adapted to the local environment of each gravel bar and the geographic distance between the bars was relevant, inter-bar migration that could homogenise the microbial community structure among the bars was not evident regardless of the discharge level.
Our results also suggest that the microorganisms cannot disperse as far as 2 km even under high discharge but can only move strongly within gravel bar. The contrasting results of community divergence observed between two spatial areas, i.e., within and between gravel bar areas, provided valuable insights into the role of environmental heterogeneity in microbial community structure in the hyporheic zone at different spatial areas. Our observation is in accordance with Yang et al. (2021) observation that microbial species could still be detected within a 1-km distance downstream of the sampling site.
This significant finding underlines the similarity of microbial community structure among the three hyporheic areas observed under high discharge conditions. These results support our hypothesis that an increased river surface water discharge would also represent mean hydrologic changes in hyporheic zones. The disturbance among the hyporheic zone microbial communities might rise during the high discharge condition introducing homogenisation. This could be explained by the potential mechanism of mixing hyporheic water between the three areas. However, determining the driving process was not possible since the surface water was not examined. Understanding how communities respond to disturbances such as a flood is essential to identify biological and ecological processes that determine their assembly and predict future effects on diversity and function (Marmonier et al., 2012). To the best of our knowledge, this is the first study to investigate how increased river water discharge influences heterogeneous microbial communities among the hyporheic flow path in gravel bars.
Environmental factors shaped microbial community divergence
We demonstrated that turbidity, EC, Al, pH, and Ca were the environmental factors that significantly shaped microbial community divergence. Certain microbial community groups cannot withstand when metal concentrations increased in the environment. Moreover, metal concentrations play an essential role in influencing microbial communities (Zeglin, 2015). For example, Feris et al. (2003) described a significant relationship between streambed metal concentrations in hyporheic zone and microbial community structure in six different rivers. Turbidity is essential in water microbial communities as it influences the processes within the primary producers, such as phytoplankton and photosynthetic bacteria. These primary producers rely on light energy and oxygen production for growth and variable water chemical properties, that in turn, influence bacterial nutrient use in the river ecosystem (Wagner et al., 2015).
Our results showed that several water qualities, e.g., Turbidity, EC, pH, Al and Ca, were shown to be associated with microbial communities. The difference in water discharge is assumed to cause changes in environmental conditions in which water quality and microbial communities respond. However, the environmental variables associated with the microbial community may be temporal, and associated environmental variables maybe interchangeable depending on the flow magnitude and duration.
Putative functions involve in biogeochemical processes
Nitrification, denitrification and methanogensesis were involved in biogeochemical processes in the hyporheic zone. However, this study did not directly measure biogeochemical processes, so it should be noted that these identified functions were potential or putative ecological functions based on the 16S rRNA gene sequencing. We identified a dominant putative metabolic function at each hyporheic area (Supplementary Figure S2). Methanogenesis was abundant at the upwelling, while methanotrophy and methlylotrophy at the downwelling areas in both discharge levels. This study supported the with the findings of Jones et al. (2015) that methanogenesis accounted for all the respiration in anoxic sediments and 0.3%–0.6% of the total respiration. These findings implied that hyporheic zone is essential in the carbon cycle such as methylotrophy, methanotrophy and methanogenesis in gravel bars. Moreover, the hyporheic zone through methanogenesis appears to be an important pathway for organic cycling and is a potential source of labile organic carbon on the surface of stream water. Putative metabolic functions, chemoheterotrophy and aerobic chemoheterotrophy, were the most dominant throughout all the 16 areas, suggesting the importance of metabolically active microorganisms in hyporheic zone. Mainly the abundant bacteria (e.g., Proteobacteria, Acidobacteria, and Verrucomicrobiota) contributed to chemoheterotrophy and aerobic chemoheterotrophy as putative metabolic functions. Our study provided profiles of ecological functions across the different areas of gravel bars. Overall, our study also implies that hyporheic zone is suitable for thriving heterotrophic microorganisms.
Furthermore, we identified a positive correlation between differences in taxonomic composition and differences in potential metabolic functions (Figure 4). This finding implies that the taxonomic structure divergence observed between areas or gravel bars reflects different putative metabolic functions that emerged among the heterogeneous environments. This result is in good agreement with previous studies on stream microbial communities (Galand et al., 2018; Fasching et al., 2020; Serrana et al., 2022). However, it should be also noted that the use of 16S rRNA gene sequences to predict metabolic function is constrained by the limited information content of the amplicon and limited databases. Hence, the putative metabolic function would require further validation using metagenomics and metatranscriptomics analyses that could provide comprehensive information on functional genes.
We observed no significant difference in alpha diversity among any of the three areas in the three gravel bars under either low or high discharge conditions. This result coincided with those of Nelson, et al. (2019) and Sackett, et al. (2019), showing no significantly different variation level in microbial communities among the areas in the gravel bar. We consider that each area can harbor similar alpha diversity levels with species turnover among the areas. Potential mechanisms for the similar alpha diversity among the areas, such as the environmental capacity for the number of species that each area can hold or limit of the number of detectable species per area by metabarcoding, should be explored in future studies. Although the alpha diversity did not varied across gravel bars with low and high flow conditions, microbial community structure might have differences within gravel bar areas, given the environmental heterogeneity of hyporheic zones in the gravel bar.
Conclusion
In conclusion, spatial environmental heterogeneity among the hyporheic zone at low discharge resulted in microbial community structure divergence at three hyporheic (i.e., downwelling, upwelling, and intermediate) areas within the gravel bars. Our finding highlights how high discharge influences microbial community convergence, as explained by the homogenisation in hyporheic zones. Putative metabolic functions were identified in the three hyporheic areas, correlating with the taxonomic community structure, and were determined to understand the process of microbial ecology dynamics in hyporheic zones. Furthermore, we identified environmental factors such as turbidity, EC, and Al as drivers of divergence between hyporheic areas within gravel bars. To further understand the biological mechanisms of microbial communities in the hyporheic zone, we believe that future studies should focus on metagenomics and metatranscriptomics to understand better the functional genes and activity of microbial communities in the hyporheic zone in gravel bars. Moreover, further studies are recommended on a longer time scale to validate the influence of flow discharge on hyporheic microbial communities.
Data availability statement
The original contributions presented in the study are publicly available. This data can be found here: https://www.ncbi.nlm.nih.gov/search/all/?term=PRJNA855985, PRJNA855985
Author contributions
AD-L: Conceptualisation, Methodology, Investigation, Data curation, Formal analysis, Visualisation, Writing–original draft. JS: Investigation, Formal analysis, Writing–review and editing. ST: Investigation, Data curation, Methodology, Writing–review and editing. YT: Investigation, Data curation, Writing–review and editing, Funding acquisition. KW: Conceptualisation, Writing–review and editing, Resources, Supervision, Project administration, Funding acquisition. All authors contributed to the article and approved the submitted version.
Funding
This work was supported by the Japan Society for the Promotion of Science (JSPS) Grant-in-Aid for Scientific Research (22H00571 and 22H01627).
Acknowledgments
We acknowledge the substantial field work assistance of Dr. Maribet Gamboa, Somar Israel Fernando, and the Kyoto University Disaster Prevention Institute. We would also like to thank Dr. Naohito Tokunaga of Ehime University, Division of Analytical Biomedicine Advance Research Support Center (ADRES) and Dr. Paul Johnston of Leibniz-Institute of Freshwater Ecology and Inland Fisheries (IGB) for their assistance in the sequencing and data processing, and Micanaldo Ernesto Francisco for making a detailed map of the sampling sites. We also thank Jane Watanabe, Dr. Ming–Chih Chiu and Prof. Dr. Christoph Stein-Thoeringer for constructive comments on the manuscript.
Conflict of interest
The authors declare that the research was conducted in the absence of any commercial or financial relationships that could be construed as a potential conflict of interest.
Publisher’s note
All claims expressed in this article are solely those of the authors and do not necessarily represent those of their affiliated organizations, or those of the publisher, the editors and the reviewers. Any product that may be evaluated in this article, or claim that may be made by its manufacturer, is not guaranteed or endorsed by the publisher.
Supplementary material
The Supplementary Material for this article can be found online at: https://www.frontiersin.org/articles/10.3389/fenvs.2023.1205561/full#supplementary-material
Supplementary Figure S1 | Microbial taxonomy and diversity for each hyporheic area (A) Top 10 most abundant genera. (B) Top 10 most abundant phyla. (C) Top five most abundant genera (D) Top 5 most abundant phyla.
Supplementary Figure S2 | Heatmap showing the abundant putative metabolic function for each hyporheic area i.e., downwelling, upwelling and intermediate.
References
Anderson, M., Gorley, R., and Clarke, R. (2008). Permanova+ for primer: guide to software and statistical methods. Plymouth: PRIMER-E limited.
Andrews, S. (2010). FastQC: a quality control tool for high throughput sequence data. Available at: http://www.bioinformatics.babraham.ac.uk/projects/fastqc.
Boano, F., Harvey, J., Marion, A., Packman, A., Revelli, R., Ridolfi, L., et al. (2014). Hyporheic flow and transport processes: mechanisms, models, and biogeochemical implications. Rev. Geophys. 52 (52), 603–679. doi:10.1002/2012RG000417
Boulton, A., Datry, T., Kasahara, T., Mutz, M., and Standfor, J. (2010). Ecology and management of the hyporheic zone: stream-groundwater interactions of running waters and their floodplains. J. North Am. Benthol. Soc. 29, 26–40. doi:10.1899/08-017.1
Boulton, A., and Stanley, E. (1995). Hyporheic processes during flooding and drying in a Sonoran Desert stream. II. Faunal dynamics. Arch. Hydrobiol. 134, 27–52. doi:10.1127/archiv-hydrobiol/134/1995/27
Boylen, E., Rideout, J., Dillon, M., Bokulich, N. A., Abnet, C., Al-Ghalith, G., et al. (2019). QIIME2: reproducible, interactive, scalable, and extensible microbiome data science. Nat. Biotechnol. 6, e27295v2. doi:10.1038/s41587-019-0209-9
Bray, J. R., and Curtis, J. T. (1957). An ordination of the upland forest communities of southern Wisconsin. Ecol. Monogr. 27 (4), 325–349. doi:10.2307/1942268
Bruno, M. C., Vallefuoco, F., Casari, A., Larse, S., Dallaflor, V., and Zolezzi, G. (2023). “Moving waters to mitigate hydropeaking: a case study from the Italian Alps,” in Special issue: innovationsation in hydropeaking research, 570–587.
Calderon, M., Baldigo, B., Smith, A., and Endreny, T. (2017). Effects of extreme floods on macroinvertebrate assemblages in tributaries to the Mohawk. New York, USA: River Research and Application, 1060–1070. doi:10.1002/rra.3158
Callahan, B., McMurdie, P., Rosen, M., Han, A., Johnson, A., and Holmes, S. P. (2016). DADA2: high-resolution sample inference from Illumina amplicon data. Nat. Methods 13 (7), 581–583. doi:10.1038/nmeth.3869
Caporaso, J., Lauber, C. L., Walters, W. B.-L., Huntley, J., Fierer, N., Gormley, N., et al. (2012). Ultra-high-throughput microbial community analysis on the Illumina Hiseq and Miseq platforms. ISME J. 6 (8), 1621–1624. doi:10.1038/ismej.2012.8
Chao, A. (1984). Non-parametric estimation of the classess in a population. Scandivanian J. Statistics 11 (4), 265–270. doi:10.2307/4615964
Dixon, P. (2003). VEGAN, a package of R functions for community ecology. J. Veg. Sci. 14, 927–930. doi:10.1111/j.1654-1103.2003.tb02228.x
Doering, M., Freimann, R., Antenen, N., Roschi, A., Robinson, C. T., Fabio Rezzonico, F., et al. (2021). Microbial communities in floodplain ecosystems in relation to altered flow regimes and experimental flooding. Sci. Total Environ. 788, 147497, 147497. ISSN 0048 9697. doi:10.1016/j.scitotenv.2021.147497
Dole-Olivier, M. J., Marmonier, P., and Beffy, J.-L. (1997). Response of invertebrates to lotic disturbance: is the hyporheic zone a patchy refugium? Freshw. Biol. 37, 257–276. doi:10.1046/j.1365-2427.1997.00140.x
Fasching, C., Akotoye, C., Bizic, M., Fonvielle, J., Ionescu, D., Mathavarajah, S., et al. (2020). Linking stream microbial community functional genes to dissolved organic matter and inorganic nutrients. Limnol. Oceanogr. 65, S71–S87. doi:10.1002/lno.11356
Feris, K., Ramsey, P., Frazar, C., Moore, J., Gannon, J., and Holben, W. (2003). Structure and seasonal dynamics of hyporheic zone microbial communities in free-stone rivers of the estern United States. Microb. Ecol. 46, 200–215. doi:10.1007/BF03036883
Galand, P., Pereira, O., Hochart, C. A., Auguet, J. C., and Debroas, D. (2018). A strong link between marine microbial community composition and function challenges the idea of functional redundancy. ISME 12, 2470–2478. doi:10.1038/s41396-018-0158-1
Graham, E., Averil, C., Knelma, J., Krause, S., Peralta, A., Peralta, A. L., et al. (2021). Toward a generalizable framework of disturbance ecology through crowdsourced science. Front. Ecol. Evol. 9, 1–14. doi:10.3389/fevo.2021.588940
Harjung, A., Sabater, F., Perujo, N., and Butturini, A. (2019). Responses of microbial activity in hyporheic pore water to biogeochemical changes in a drying headwater stream. Freshw. Biol. 64, 735–749. doi:10.1111/fwb.13258
Hendricks, S. (1993). Microbial ecology of the hyporheic zone: a perspective integrating hydrology and biology. J. North Am. Benthol. Soc. 12 (1), 70–78. doi:10.2307/1467687
Jones, I., Growns, I., Arnold, A., McCall, S. B., and Bowes, M. (2015). The effects of increased flow and fine sediment on hyporheic invertebrates and nutrients in stream mesocosms. Freshw. Biol. 60, 813–826. doi:10.111/fwb.12536
Kim, H., and Lee, K. (2019). Effect of vertical flow exchange on microbial community distributions in hyporheic zones. Episodes 42 (1), 1doi–16. doi:10.18814/epiiugs/2019/019001
Legendre, P., and Legendre, F. J. L. (2012). Numerical ecology. Radarweg, radarweg.ElsevierISBN, 0444538690, 9780444538697.
Lewandowski, J., Arnon, S., Banks, E., Batelann, O., Betterle, A., Broecker, T., et al. (2019). Is the hyporheic zone relevant beyond the scientific community? Water 11, 2230. doi:10.3390/w11112230
Louca, L., and Doepeli, M. (2017). Taxonomic variability and functional stability in microbial communities infected by phages. Environ. Microbiol. 19 (10), 3863–3878. doi:10.1111/1462-2920.13743
Louca, S., Parfrey, L., and Doebeli, M. (2016). Decoupling function and taxonomy in the global ocean microbiome. Science 353, 1272–1277. doi:10.1126/science.aaf4507
Lowell, J., Gordon, N. E., Holben, W. G., Stanford, J. A., and Gannon, J. E. (2009). Habitat heterogeneity and associated microbial community structure in a small-scale floodplain hyporheic flow path. Microb. Ecol. 58 (3), 611–620. doi:10.1007/s00248-009-9525-9
Marmonier, P., Archambaud, G., Belaidi, N., Bougon, N., Cornut, J., Datry, T., et al. (2012). The role of organisms in hyporheic processes: gaps in current knowledge, needs for future research and applications. Int. Journaof Limnol. 48, 253–266. doi:10.1051/limn/2012009
McMurdie, P., and Holmes, S. (2015). Shiny-phyloseq:Web application for interactive microbiome analysis with provenance tracking. Bioinformatics(Oxford, Engl. 31 (2), 282–283. doi:10.1093/bioinformatics/btu616
Miyake, Y., Makino, H., and Fukusaki, K. (2021). Assessing invertebrate response to an extreme flood at a regional scale utilizing past survey data. Limnology 22 (2), 16–177. doi:10.1007/s10201-021-00651-5
Naegeli, M., and Uehlinger, U. (1997). Contribution of the hyporheic zone to ecosystem metabolism in a prealpine gravel-bed river. J. N. Am. Benthol. Soc. 16 (4), 794–804. doi:10.2307/1468172
Nelson, A. R., Sawyer, H., Gabor, R. S., Saup, C. M., Bryant, S. R., Harris, K. D., et al. (2019). Heterogenity in hyporheic flow, pore water, chemistry, and microbial community composition in alpine streambed. J. Geophys. Res. 124 (11), 1–14. doi:10.1029/2019JG005226
Ock, G., Gaeuman, D., McSloy, J., and Kondolf, G. (2015). Ecological functions of restored gravel bars, the Trinity River, California. Ecolgical Eng. 83, 49–60. doi:10.1016/j.ecoleng.2015.06.005
Pruesse, E., Peplies, J., and Glöckner, F. (2012). SINA: accurate high-throughput multiple sequence alignment of ribosomal RNA genes. Bioinforma. Oxf. Engl. 28 (14), 1823–1829. doi:10.1093/bioinformatics/bts252
R Core Team (2018). R: language and environment for statistical computing. Vienna Austria: R Foundation for Statistical Computing. Retrieved from Available at: https://www.R-project.org/.
Sackett, J., Shope, C., Bruckner, J., Wallace, J., Cooper, C., and Moser, D. (2019). Microbial community structure and metabolic potential of the hyporheic zone of a large mid-stream channel bar. Geomiccrobiology J. 36, 765–776. doi:10.1080/01490451.2019.1621964
Serrana, J., Li, B., Sumi, T., Takemon, Y., and Watanabe, K. (2022). Profiling the microbial community structure and functional diversity of a dam-regulated river undergoing gravel bar restoration. Freshw. Biol. 66, 2170–2184. doi:10.1111/fwb.13824
Shannon, C. E. (1949). The mathematical theory of communication. Bell Syst. Tech. J. 27 (3). doi:10.1002/j.1538-7305.1948.tb01338.x
Wagner, K., Basemer, K., Burns, N. R., Battin, T., and Bengstsson, M. M. (2015). Light availability affects stream biofilm bacterial community composition and function, but not diversity. Environ. Microbiol. 17, 5036–5047. doi:10.1111/1462-2920.12913
Yang, H., Du, H., Qi, H., Yu, L., Hou, X., Zhang, H., et al. (2021). Effectiveness assessment of using riverine water eDNA to simultaneously monitor the riverine and riparian biodiversity information. Sci. Rep. 11, 24241. doi:10.1038/s41598-021-03733-7
Keywords: hyporheic zone, microbial community structure, 16S rRNA gene sequencing, river discharge, putative metabolic function
Citation: Doloiras-Laraño AD, Serrana JM, Takahashi S, Takemon Y and Watanabe K (2023) Short-term influences of flow alteration on microbial community structure and putative metabolic functions in gravel bar hyporheic zones. Front. Environ. Sci. 11:1205561. doi: 10.3389/fenvs.2023.1205561
Received: 04 May 2023; Accepted: 15 November 2023;
Published: 19 December 2023.
Edited by:
Emily B. Graham, Pacific Northwest National Laboratory (DOE), United StatesReviewed by:
Stefano Larsen, Fondazione Edmund Mach, ItalyShuyin Li, Yangtze River Basin Ecological Environment Monitoring and Scientific Research Center, China
Copyright © 2023 Doloiras-Laraño, Serrana, Takahashi, Takemon and Watanabe. This is an open-access article distributed under the terms of the Creative Commons Attribution License (CC BY). The use, distribution or reproduction in other forums is permitted, provided the original author(s) and the copyright owner(s) are credited and that the original publication in this journal is cited, in accordance with accepted academic practice. No use, distribution or reproduction is permitted which does not comply with these terms.
*Correspondence: Kozo Watanabe, watanabe.kozo.mj@ehime-u.ac.jp
†Present Address: Joeselle M. Serrana, Department of Environmental Science and the Stockholm University, Center for Circular and Sustainable Systems, Stockholm University, Stockholm, Sweden
Arnelyn D. Doloiras-Laraño, Clinic for Internal Medicine I, University Hospital Tuebingen, Tuebingen, Germany