- 1Department of Physical Geography, Institute of Geography, University of Göttingen, Göttingen, Germany
- 2Faculty of Geosciences, University of Münster, Münster, Germany
- 3Faculty of Agriculture, Tadulako University, Palu, Indonesia
- 4Department of Agroecosystems, University of Göttingen, Göttingen, Germany
Potential effects of land-use/land-cover (LULC) transformation from lowland rainforest into oil-palm plantations on silicon (Si) pools in tropical soils remain poorly understood, although appropriate levels of plant-available Si in soils may contribute to maintain high crop yields and increase the vitality and drought resistance of oil palms. Therefore, the aim of our study was to identify possible effects of such LULC change on soil Si pools. For this purpose, we compared soil Si pools under lowland rainforest and ca. 20 year-old oil-palm plantations in Jambi Province, Indonesia. The investigated soils were Acrisols and Stagnosols, in which we quantified six different soil Si pools following a sequential extraction procedure to evaluate, whether 20 years of oil-palm cultivation has led to a depletion of these soil Si pools. The considered pools included mobile Si, adsorbed Si, Si bound in soil organic matter (SOM), Si included in pedogenic oxides and hydroxides, and Si in amorphous silica of biogenic and pedogenic origin. Finally, we also determined total Si. All oil-palm plantations established on sloping terrain and Acrisols only showed decreased Si stocks of mobile Si, adsorbed Si and SOM-bound Si; those established in floodplains and Stagnosols had decreased stocks of SOM-bound Si and biogenic-amorphous silica. Lower Si stocks were mostly attributed to a missing “stable” phytolith pool in the subsoil and less organic matter in topsoils under oil-palm plantations. When comparing well-drained and riparian areas, flooding seemed to increase phytolith dissolution. We conclude that 20 years of oil-palm cultivation has not yet led to a significant depletion of soil Si pools. As topsoils comprise the highest concentrations of SOM-bound Si and Si in amorphous silica of biogenic origin but are susceptible to erosion and surface runoff under managed oil-palm plantations, it would be advisable to instate specific management practices that maintain organic-rich and well-aired topsoils on oil-palm plantations.
1 Introduction
Jambi Province in Sumatra, Indonesia, has a long history of crop cultivation, including, e.g., rubber, oil palm, sugar cane, coffee, and tea (FAO, 2020). Oil-palm (Eleais guineensis) cultivation increased noticeably in the 1980s after a governmental transmigration policy had been implemented (McCarthy and Cramb, 2009; Gatto et al., 2015; Tsujino et al., 2016). The economic value of palm oil became increasingly recognized as oil-palm cultivation required less labor and cash yields per hectare exceeded those of rubber (Euler et al., 2016). Since then, lowland rainforest has been progressively converted to rubber and oil-palm plantations in Jambi Province (Clough et al., 2016; Drescher et al., 2016; Dislich et al., 2017), leading to reduced biodiversity (Kotowska et al., 2015; Nazarreta et al., 2020) and ecosystem services (Dislich et al., 2017). With respect to soil functioning, this transformation resulted among others in decreased nutrient stocks and increased nutrient leaching (Guillaume et al., 2015; Allen et al., 2016; Kurniawan et al., 2018). Decreasing soil functioning may be mitigated by optimizing oil-palm management, e.g., adapting fertilizer applications, reducing herbicide application, and managing understory vegetation (Darras et al., 2019; Luke et al., 2019; Woittiez et al., 2019; Zemp et al., 2019; Grass et al., 2020).
Such improved oil-palm management practices could also include monitoring levels of plant-available silicon (Si) in soils, as Si is known to increase stress tolerance and crop yield (Epstein, 1994; Najihah et al., 2015; Schaller et al., 2018; Sirisuntornlak et al., 2020). In addition, Si can mitigate toxic effects of various elements in plants (Epstein, 1999). This effect of Si is particularly relevant in the tropics, where crops are often grown on highly weathered, acidic soils, because toxic ions (e.g., Al, Cd, Mn) become increasingly soluble in the soil solution below around pH 4 (Epstein, 1999). However, until present, little is known about the status of soil Si pools under oil-palm plantations and how it is affected by land-use/land-cover (LULC) change.
Various practices affect ecosystem Si cycling during LULC change and may involve Si losses from the system. For instance, LULC change through deforestation (logging and/or fire) may enhance the amounts of Si released from soil (Conley et al., 2008; Struyf et al., 2010; von der Lühe et al., 2020), potentially resulting in temporarily increased Si leaching. High Si concentrations measured in topsoils and water seem to originate from the dissolution of plant-derived amorphous silica (Conley et al., 2008), although other Si fractions in soils may also release Si into soil solution (Sauer et al., 2006; Georgiadis et al., 2013). Struyf et al. (2010) and Clymans et al. (2011) detected noticeably lower Si concentrations and fluxes after centuries of soil cultivation (250–500 years) in watersheds of temperate ecosystems. Conley et al. (2008) already recognized a disruption in the Si cycle within 20–40 years after deforestation. Munevar and Romero (2015) suggested that oil-palm cultivation could lead to similar disruptions as described by Conley et al. (2008).
Plants take up Si as monosilicic acid (H4SiO4) from soil solution (Liang et al., 2015), whereby several crops such as rice, wheat, sugarcane, maize, and oil palm are referred to as Si accumulators (Ma and Takahashi, 2002; Matichenkov and Calvert, 2002; Liang et al., 2015; Munevar and Romero, 2015). Transpiration causes Si to precipitate in the biomass (Epstein, 1994; Carey and Fulweiler, 2016), partially in the form of cell-shaped amorphous silica bodies called phytoliths, which accumulate with time (Epstein, 1994). In an undisturbed environment, Si returns to soil through litterfall, whereby phytoliths accumulate in the topsoil (Lucas et al., 1993; Alexandre et al., 1997; Schaller et al., 2018). In oil-palm plantations, natural litterfall is disturbed by cutting off and stacking palm fronds in every second oil-palm row, a management practice referred to as frond-pile stacking (Dislich et al., 2017). Thus, the majority of biomass-bound Si returns to soil under frond piles, where phytoliths are released upon litter decomposition (von der Lühe et al., 2022; Greenshields et al., 2023). Additional disturbances of Si cycling may be caused by fruit harvest and topsoil erosion, which can both lead to Si export from the system (Guntzer et al., 2012; Vandevenne et al., 2012; Hughes et al., 2020; Puppe et al., 2021; Greenshields et al., 2022). Fruit bunches are collected immediately after harvesting to be further processed in a mill (Dislich et al., 2017). A lack in understory vegetation, which is often intentionally achieved by herbicide application, permits erosion of phytolith-enriched topsoil (Guillaume et al., 2015). In this way, an important source to replenish plant-available Si in soil solution in highly weathered tropical soils may be lost (Lucas et al., 1993; Derry et al., 2005; Cornelis et al., 2011; de Tombeur et al., 2020).
Based on the above-mentioned potential disturbances of Si cycling under oil-palm cultivation, our study addresses the question, how the transformation of lowland rainforest into oil-palm plantations affects Si pools in soils of two regionally very common Reference Soil Groups, Acrisols and Stagnosols (IUSS Working Group WRB, 2022). We hypothesized that soil Si pools are decreased under oil-palm plantations compared to lowland rainforest for two reasons.
1) Oil palms are considered Si-accumulating plants (Munevar and Romero, 2015) that take up substantial amounts of Si from soil solution. Thus, Si losses are to be expected through fruit-bunch harvest and management practices that return litter to soil only in certain areas of the plantation.
2) Oil-palm plantations that are kept free of understory vegetation are susceptible to topsoil erosion. As topsoils contain the highest amounts of phytoliths and SOM-bound Si, thus providing major sources of plant-available Si in soils (Conley et al., 2008), considerable Si losses are expected through topsoil erosion.
We further hypothesized that the Stagnosols in our study area, commonly found in riparian areas and lower landscape positions, are less prone to net Si depletion as they may receive dissolved Si through groundwater and slope water from higher landscape positions.
To test the above hypotheses, we quantified various soil Si pools using a sequential extraction method (Georgiadis et al., 2013). We replaced the originally included extraction of Si from amorphous silica by a modified alkaline extraction technique (Barão et al., 2014; Unzué-Belmonte et al., 2017).
2 Materials and methods
2.1 Study area and sampling scheme
2.1.1 Study area
The study area is in the Harapan region of Jambi Province, Sumatra, Indonesia (1° 55′ 0″ S, 103° 15′ 0″ E; 50 m ± 5 m NN). Geologically, the Harapan region is located within the South Sumatra basin, which is comprised of pre-Paleogene metamorphic and igneous bedrock that is covered by lacustrine and fluvial Neogene and Quaternary sediments (de Coster, 2006). The Harapan region has a humid-tropical climate (mean annual temperature −27°C; mean annual precipitation −2,230 mm) with a rainy season from December to March and a dry period from July to August (Drescher et al., 2016). The region is dominated by loamy Acrisols on hilltops and slopes (well-drained areas) and loamy to clayey Stagnosols in riparian areas (Table 1 and Supplementary Tables SA1, A2). The natural vegetation is tropical lowland rainforest (Laumonier and Seameo-Biotrop, 1997), which, however, has largely disappeared and is almost exclusively found within the Harapan Rainforest - an ecosystem restauration concession in the South of the region (Harrison and Swinfield, 2015). Oil-palm plantations (smallholder-, private company-, and state-owned plantations of Indonesia), rubber monocultures and rubber agroforestry systems constitute much of the rest of the region (Dislich et al., 2017).
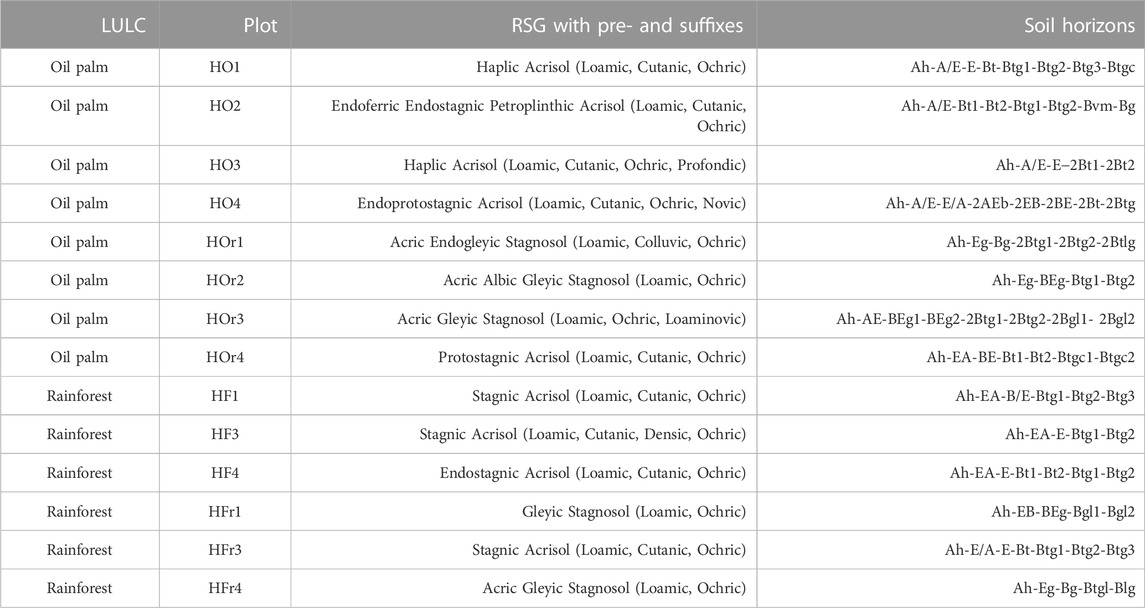
TABLE 1. Classification of oil palm and rainforest plots (PT REKI) in the Harapan landscape of Jambi Province in Sumatra, Indonesia.
2.1.2 Sampling scheme
Our study was conducted on smallholder oil-palm plantations, which typically comprise 2 ha and still account for −40% of oil-palm plantations in the province (Dislich et al., 2017). Oil palms were planted in a triangular planting scheme between 1998 and 2008. Old palm fronds are cut off and stacked in every second row, called “interrow.” The remaining “empty” interrows are used as paths for oil-palm pruning, herbicide application, and fruit-bunch harvesting (Darras et al., 2019; Greenshields et al., 2023). Herbicides (e.g., glyphosate) are commonly sprayed every 6 months to clear understory vegetation in the interrows and NPK fertilizers (300 kg–550 kg ha-1 yr-1,e.g., Phonska, Mahkota) are applied within the palm circle (Allen et al., 2015), i.e., the immediate circulate area (−2 m radius) surrounding the palm stem (Munevar and Romero, 2015).
Fourteen plots (50 × 50 m) established by the Collaborative Research Centre 990 EFForTs (Environmental and socioeconomic functions of tropical lowland transformation systems) were selected for soil sampling. Eight plots were located in smallholder oil-palm plantations, whereby four were in well-drained areas (HO1 - 4) and four in riparian areas (HOr1 - 4). Another six plots were located in lowland rainforest, whereby again three were in well-drained areas (HF1, 3, 4) and three in riparian areas (HFr1, 3, 4). Soil profiles (1 m depth) were established either in oil-palm rows (between two palm trees) or in interrows (between two palm rows) but not in those interrows, where oil-palm fronds were deposited. The soils were classified according to WRB (IUSS Working Group WRB, 2022). Soil samples were taken of each horizon down to a depth of 1 m, whereby horizons exceeding 20–25 cm were subdivided into two sampling depths (top, bottom). Bulk-density samples (n = 4 per horizon) were taken in 100 cm2 steel cylinders. The samples were air-dried (40°C, −24 h), sieved (≤2 mm) and stored at room temperature until further analysis.
2.2 Methods
2.2.1 Basic soil analyses and general procedure used to determine six soil Si fractions
Basic analyses for general soil characterization included the following: bulk density, potential cation exchange capacity and base saturation (using 1 M NH4Cl solution for cation release and analysing the released Ca2+, Mg2+, K+, Na+, Al3+, Fe2+, and Mn2+ by ICP-OES), soil pH (in water and in CaCl2 solution, measured at a soil:solution ratio of 1:5), and particle size distribution (Atterberg method, after dissolution of iron oxides by sodium dithionite), as well as total C and N contents, measured by CHN analyzer (Leco TrueSpec), whereby total C contents corresponded to SOC contents as no inorganic C was present (Supplementary Table SB1).
We followed the sequential extraction procedure developed by Georgiadis et al. (2013) to extract different soil Si fractions. All extractions were conducted in two lab replicates. After each extraction step, the extract for analysis was obtained by centrifuging (5–15 min, 3,000 rpm) and filtering the supernatant through ash-free paper filters (1–2 µm). Between two subsequent extraction steps, soil samples were rinsed twice with deionized water (18.2 MΩ cm-1) to remove any residues of the previous extractant and dried overnight at 45°C.
Mobile Si (SiM) and adsorbed Si (SiAd) were analyzed by the molybdenum blue method (Grasshoff et al., 2009) using an UV-VIS spectrophotometer (Lamda 40, Perkin Elmer, Ridgau, Germany) at 810 nm. SOM-bound Si (SiOrg) and Si occluded in pedogenic Fe-Al oxides and hydroxides (SiOcc) were measured with an inductively coupled plasma atomic emission spectrometer (ICP-AES, iCap 7,000, Thermo Fisher Scientific GmbH, Dreieich, Germany).
2.2.1.1 Mobile Si: SiM
SiM is the Si fraction that is extractable by calcium chloride (CaCl2) solution and is usually present in terrestrial environments as monomeric silicic acid (H4SiO4). The soil samples were mixed with 5 mL of 0.01 M CaCl2 and left for 24 h, shaking them for 1 min h-1 on an overhead shaker.
2.2.1.2 Adsorbed Si: SiAd
SiAd is the Si fraction that is extractable by acetic acid (Georgiadis et al., 2013). This extraction aims at determining the amount of silicic acid adsorbed to mineral surfaces (Sauer et al., 2006). The second extraction step was carried out in an analogous manner to the first step but using 10 mL of 0.01 M acetic acid to extract SiAd.
2.2.1.3 Si bound in soil organic matter: SiOrg
SiOrg refers to Si that is released when soil organic matter (SOM) is oxidized by hydrogen peroxide treatment (Georgiadis et al., 2013). SiOrg was obtained by treating the samples with 20 mL H2O2 (17.5%) and letting the samples react at room temperature until the reaction subsided, typically within half an hour. Then, an additional 10 mL H2O2 (35%) was added. The samples were placed into a shaking hot water bath at 85°C and left until the reaction ceased (up to 48 h).
2.2.1.4 Si occluded in Fe-Al oxides and hydroxides: SiOcc
SiOcc refers to Si that is released when Fe-Al oxides and hydroxides are dissolved with ammonium-acetate-oxalic acid and UV-light exposure (Georgiadis et al., 2013). 50 mL of a solution containing 0.2 M ammonium-acetate and 0.14 M oxalic acid were added and samples were placed on an orbital shaker for 8 h, shaking for 1 min h-1. After 8 h, the soil samples were exposed to UV-light while they were left on the orbital shaker for another 16 h, shaking for 1 min h-1.
We used an alkaline extraction that was modified from Barão et al. (2014) and Unzué-Belmonte et al. (2017) to extract Si from amorphous silica of biogenic and pedogenic origin. In detail, 0.4 L of 0.2 M NaOH solution was poured into a metal beaker, placed into a hot water bath, and heated to 75°C. Once heated, the same soil sample that had already gone through the previous steps of the sequential Si extraction was added to the alkaline solution. The extraction was run for 45 min, while a stirrer continuously homogenized the solution. During the extraction, subsamples were taken with a fraction collector at 36 times, namely, every 45 s during the first 15 min of the extraction, every 90 s during the second 15 min, and every 180 s during the last 15 min. These subsamples were analyzed for Si and Al concentrations photometrically, using the molybdenum blue method and the eriochrome cyanine R method (Shull and Guthan, 1967). In the calibrations, R2 = ≥ 0.99 was accepted for Si, and R2 ≥ 0.98 was accepted for Al. The method was validated by adding known quantities of oil-palm phytoliths (extracted according to Parr et al., 2001) to soil samples. A precision of 98% was reached.
2.2.1.5 Si in amorphous silica of biogenic and pedogenic origin: SiBa and SiPa
Si in amorphous silica can be of either biogenic (SiBa) or pedogenic (SiPa) origin and is considered the most readily mobilizable Si fraction in soils. SiBa mainly consists of Si from phytoliths alongside diatoms and other protozoic Si compounds (Sommer et al., 2006; 2013; Haynes, 2017). SiPa predominantly consists of siliceous Si coatings or void infillings (Sauer et al., 2015); SiPa can also be occluded within pedogenic Fe-Al oxides and hydroxides (Schaller et al., 2021). Si released from amorphous silica over time during an alkaline extraction can be quantified by solving a first-order mathematical model (Eq. 1) that accounts for the non-linear (first part of the equation) and the linear (second part of the equation) Si release from the residual soil sample material (Unzué-Belmonte et al., 2017).
AlkExSii is the non-linearly released Si from amorphous silica, Sit and Alt are the calculated concentrations of Si and Al at a given time, Si/Ali is the fraction’s Si/Al ratio, which is used to determine its origin, k is the fraction’s specific Si-release rate constant, t defines the beginning of Si release, b is the non-linear Si intercept and Si:Almin the linear Si intercept. Detailed information on the calculation and computation using an open-access python script (https://github.com/nschenkels/AlkExSi, (Unzué-Belmonte et al., 2017) is given in Unzué-Belmonte et al. (2017). This method requires the analysis of both Si and Al concentrations to calculate AlkExSi (Eq. 1) and to determine Si/Al ratios. SiBa commonly has Si/Al ratios of ≥5 (Koning et al., 2002; Barão et al., 2014; Unzué-Belmonte et al., 2017), whereas SiPa has Si/Al ratios of 1−4 (Koning et al., 2002). We attributed all amorphous fractions that displayed a dissolution curve typical for phytoliths to SiBa, even though some of these fractions had Si/Al ratios between 4 and 5.
2.2.2 Determination of total Si
We also determined total Si to evaluate, which proportion of total Si was present in those fractions that may serve as sources of readily plant-available Si. Total Si was determined on separately milled (tungsten carbide cups, 10 min, 2000 rpm) soil samples by alkaline fusion using lithium borate (DIN ISO 14869-2, 2003) and analysis by use of inductively coupled plasma atomic emission spectroscopy (ICP-AES).
2.2.3 Calculation of Si stocks per hectare down to 1 m soil depth
After converting the obtained results to Si concentrations in dry soil samples (105°C, 24 h), Si stocks were first calculated horizon-wise for every Si pool according to Eq. 2.
[Si] is the Si concentration in mg g-1 dry soil, BD the bulk density in g cm-3, h the soil horizon thickness in cm and 10 is the conversion factor from mg cm-2 to Mg ha-1. In this way, we calculated stocks of the six Si fractions per hectare for each soil horizon. We then summed up the Si stocks of all horizons of a soil profile down to 1 m depth to be able to compare potentially mobilizable Si stocks (Mg ha-1 to 1 m depth) in soils under oil-palm plantations and lowland rainforest. Riparian areas are often drained before establishing an oil-palm plantation because oil palms cannot be cultivated on water-logged soils (Corley and Tinker, 2016). As we observed fine roots down to 1 m soil depth both in Acrisols and Stagnosols, indicating that the oil-palm can exploit nutrients over this soil depth, we calculated soil Si stocks down to 1 m depth for both WRB Reference Soil Groups.
The above calculation of Si stocks per given soil volume (Mg ha-1 to 1 m depth) was done because the focus of this work was on potential management effects on Si fractions instead of Si related to mineral weathering (for the latter, it would have been more appropriate to relate Si amounts to soil weight units). In this case, Si fractions in a given soil volume were more relevant for two reasons: 1. Plant roots can exploit nutrients from a certain soil volume, not soil weight. 2. Fertilizers and phytoliths released from decaying litter are spread over a certain soil surface. Nutrients and Si released from dissolving phytoliths are then leached into a certain soil volume, irrespective of the weight of soil in that volume (i.e., bulk density of the soil). The horizon-wise Si concentrations in mg g-1 soil are provided in Table B2 and horizon-wise Si contents in Mg dm-3 are provided in Supplementary Table SB3.
2.2.4 Statistics
Soil profiles were grouped according to WRB Reference Soil Group and LULC type into 1) Acrisols under oil-palm plantations (n = 5), 2) Acrisols under lowland rainforest (n = 4), 3) Stagnosols under oil-palm plantations (n = 3) and 4) Stagnosols under lowland rainforest (n = 2). The level of significance was set at p ≤ 0.05 for the difference to be considered significant. In addition, those differences that were almost significant at a level of p = 0.06 are mentioned, whereby the deviant level of p = 0.06 is clearly indicated. Data was tested for normal distribution (Shapiro-Wilk test) and for homogeneity of variances (Levene test). Due to an unbalanced sampling design, skewness and kurtosis in several Si stocks and a limited number of samples, non-parametric tests were used for statistical analysis. The Kruskal–Wallis test was used to detect significant differences between total Si stocks (Table 2). We used the open-source software R version 3.6.2 and R CRAN packages ggplot2 (Wickham, 2016), dplyr (Wickham et al., 2023), car (Fox and Weisberg, 2019), psych (Revelle, 2022), pastecs (Grosjean and Ibanez, 2018) and pgirmess (Giraudoux, 2022) to perform these statistical analyses.
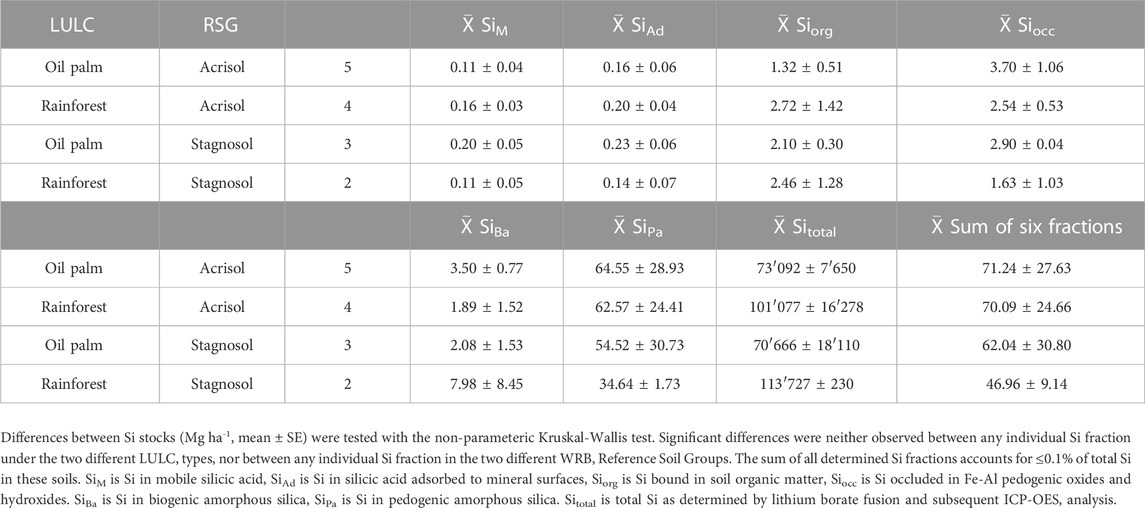
TABLE 2. Stocks [Mg ha-1, 1 m soil profile] of six Si fractions and total Si in Acrisols and Stagnosols under oil-palm plantations and lowland rainforest.
3 Results
3.1 Soil classification and soil characteristics
All soils in the generally well-drained higher areas were classified as Acrisols (IUSS Working Group WRB, 2022), both under oil-palm plantations and under lowland rainforest (Table 1 and Supplementary Tables SA1, A2). However, even in these higher areas, all soil profiles under lowland rainforest and half of the soil profiles under oil-palm plantations had stagnic properties at ≥ 50 cm soil depth (IUSS Working Group WRB, 2022), indicating that these Acrisols are periodically affected by perched water in their subsoil horizons (Supplementary Tables SA1, A2). Acrisols under both LULC types generally showed the following horizon sequence: Ah—A/E—(EA)—E—EB—Bt—Btg. Soils in riparian areas under both LULC types were mostly classified as Stagnosols. Only the soils on plots HOr4 and HFr3 were Acrisols (Supplementary Table SA1), although the two plots were in riparian areas. This was because the CRC plot area designated for destructive research activities such as digging soil pits was located in a well-drained, higher landscape position within the plot. The Acrisol in HFr3 showed stagnic properties starting at a depth of 53 cm. The typical horizon sequence of the Stagnosols was Ah—Eg—Bg—(BEg)—Btg—(Btgl)—(Bgl), both under oil-palm plantations and lowland rainforest. Hydromorphic features of the soil profiles HOr1—3 and HFr4 included both iron oxide mottles inside soil peds, indicating temporarily perched water, as well as iron oxide precipitates around macropores and on ped surfaces, pointing to groundwater influence (Btgl and Bgl horizons). Soil-chemical and soil-physical characteristics of the soil profiles are shown in the Supplementary Table SB1.
3.2 Silicon stocks per hectare in soils under oil-palm plantations and rainforest
The sum of all extractable Si fractions down to 1 m soil depth amounted to 47.0–71.3 Mg ha-1, corresponding to ≤0.1% of the total Si stocks in the soils (Table 1). In all soils, SiPa represented the largest Si stock (34.6–64.5 Mg ha-1), followed by SiBa (1.9–8.0 Mg ha-1), SiOcc (1.6–3.7 Mg ha-1), SiOrg (1.3–2.7 Mg ha-1) and finally SiAd and SiM (both together ≤0.3 Mg ha-1) (Table 2).
Acrisols under oil-palm plantations tended to have lower stocks of SiAd, SiM, SiOrg, and SiBa, compared to those under rainforest, however these observed differences were not significant (p ≤ 0.05). At a level of p = 0.006, the stocks of SiOrg under oil-palm plantations differed most compared to those under rainforest (1.3 Mg ha-1 versus 2.7 Mg ha-1).
In the Stagnosols, only the stocks of SiOrg and SiBa tended to be lower under oil-palm plantations compared to lowland rainforest, whereas the stocks of all other Si fractions tended to be higher under oil-palm plantations. None of the Si stocks differed significantly (p ≤ 0.05) between the two LULC types.
Under oil-palm cultivation, the stocks of SiM were twice as high (difference only at p = 0.06) in Stagnosols compared to Acrisols, whereas the stocks of SiAd and SiOrg were only slightly higher in Stagnosols than in Acrisols (0.23 Mg ha-1 versus 0.16 Mg ha-1 for SiAd and 2.1 Mg ha-1 versus 1.3 Mg ha-1 for SiOrg). Stocks of all other Si fractions were in a similar range in Stagnosols and Acrisols under oil-palm plantations. Under lowland rainforest, stocks of SiMa tended to be higher in Acrisols than in Stagnosols (63 Mg ha-1 versus 35 Mg ha-1), while stocks of SiBa tended to be higher in Stagnosols compared to Acrisols (8.0 Mg ha-1 versus 1.9 Mg ha-1). However, none of the Si stocks differed significantly between Stagnosols and Acrisols under rainforest.
3.3 Silicon concentrations in soils under oil-palm plantations and rainforest
3.3.1 Silicon concentrations with soil depth in the Acrisols under the two land-use/land-cover types
SiM and SiAd concentrations in the Acrisols ranged from −5 μg g-1–15 μg g-1 under both LULC types (Figures 1A,B,G,H). Under lowland rainforest, both SiM and SiAd concentrations showed a regular increase in their concentrations with soil depth (Figures 1G,H), whereas the depth distribution of SiM and SiAd was much more irregular under oil-palm cultivation (Figures 1A,B).
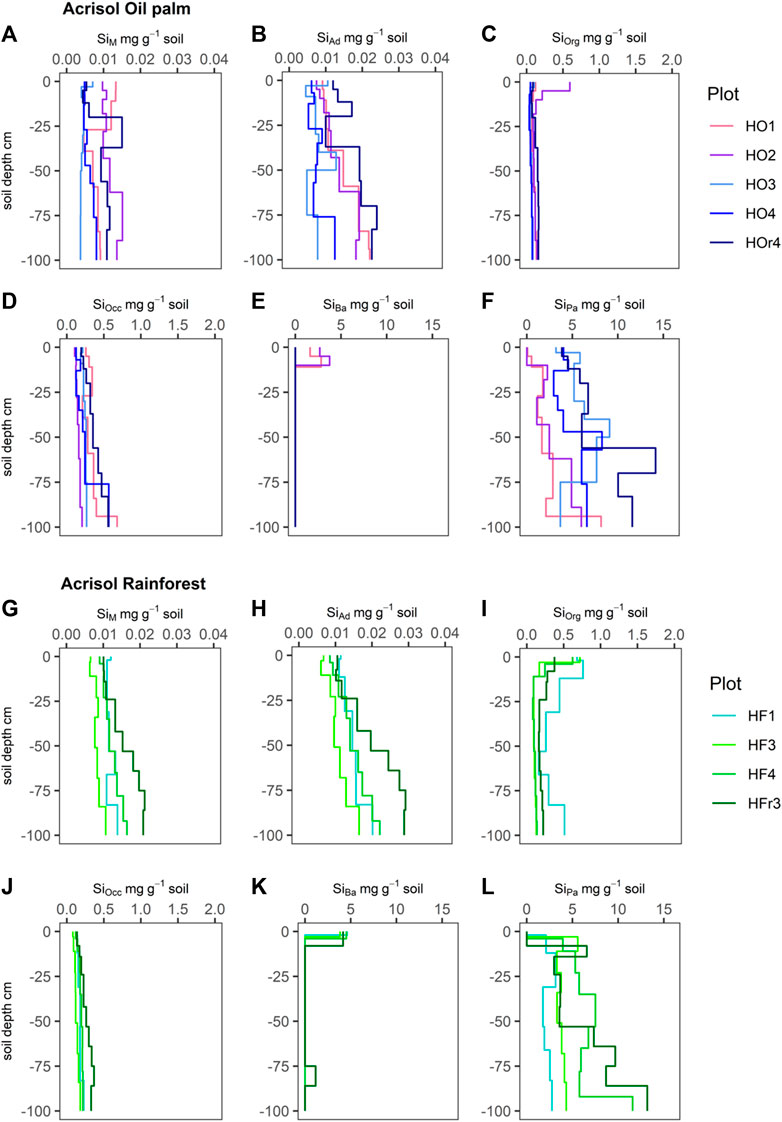
FIGURE 1. Silicon (Si) concentrations (mg g-1 soil) of six Si fractions, including SiM = mobile Si (A and G), SiAd = adsorbed Si (B and H), SiOrg = Si bound in soil organic matter (C and I), SiOcc = Si occluded in pedogenic oxides and hydroxides (D and J), SiBa = Si in biogenic amorphous silica (E and K), SiPa = Si in pedogenic amorphous silica (F and L) in Acrisols under oil-palm plantations (plots: HO1-4, HOr4) and rainforest (plots: HF1, HF3, HF4, HFr3).
SiOrg concentrations in the Acrisols under lowland rainforest were highest (∼0.4–0.6 mg g-1) in their topsoils, i.e., in their Ah and AE horizons (Figure 1I). In contrast, most Acrisols under oil-palm cultivation did not exhibit such SiOrg maximum in their topsoils; instead, their SiOrg concentrations remained around ∼0.1–0.2 mg g-1 throughout the soil profile, except for the Acrisol in plot HO2 that showed a similar SiOrg maximum (∼0.6 mg g-1) in its Ah horizon (Figure 1C).
SiOcc concentrations in the Acrisols showed a steady increase with soil depth under both LULC types, which was somewhat weaker expressed under rainforest compared to oil-palm plantations (Figures 1D,J). In Acrisols under oil-palm plantations, SiOcc concentrations increased to ≥0.4 mg g-1 in the second half meter soil depth in three out of five plots (HO1, HO4, HOr4), while under lowland rainforest, SiOcc concentrations remained below 0.4 mg g-1 in all four plots.
SiBa concentrations in all four Acrisol profiles under rainforest showed maxima in their topsoils (∼3.8–4.6 mg g-1). In contrast, under oil-palm plantations, only the two Acrisols in plots HO1 and HO2 showed comparable SiBa maxima in their topsoils (1.6–3.8 mg g-1), whereas SiBa was not detectable in the topsoils of the Acrisols in plots HO3, HO4 and HOr4. Apart from their topsoils, most Acrisols had no SiBa in any other soil horizons, except for the one on plot HFr3, which showed SiBa concentrations of ∼1.1 mg g-1 also in its Btg2 horizon, at 75–86 cm depth.
SiPa stocks in the Acrisols ranged in the same order of magnitude (0–13 mg g-1) under both LULC types and partially tended to increase with soil depth (Figures 1F,L). All four Acrisols under lowland rainforest had no SiPa in their topsoils, while only one out of five Acrisols under oil-palm plantations had no SiPa in their topsoils.
3.3.2 Silicon concentrations with soil depth in the Stagnosols under the two land-use/land-cover types
SiM and SiAd concentrations in the Stagnosols under rainforest showed a slight to clear increase with depth, from ∼6 μg g-1 in the topsoils to ∼5–16 μg g-1 in the subsoils (Figures 2G,H). SiM and SiAd concentrations in the Stagnosols under oil-palm plantations showed much more irregular depth patterns, ranging between ∼5–24 μg g-1 in the topsoils and ∼10–31 μg g-1 in the subsoils (Figures 2A,B).
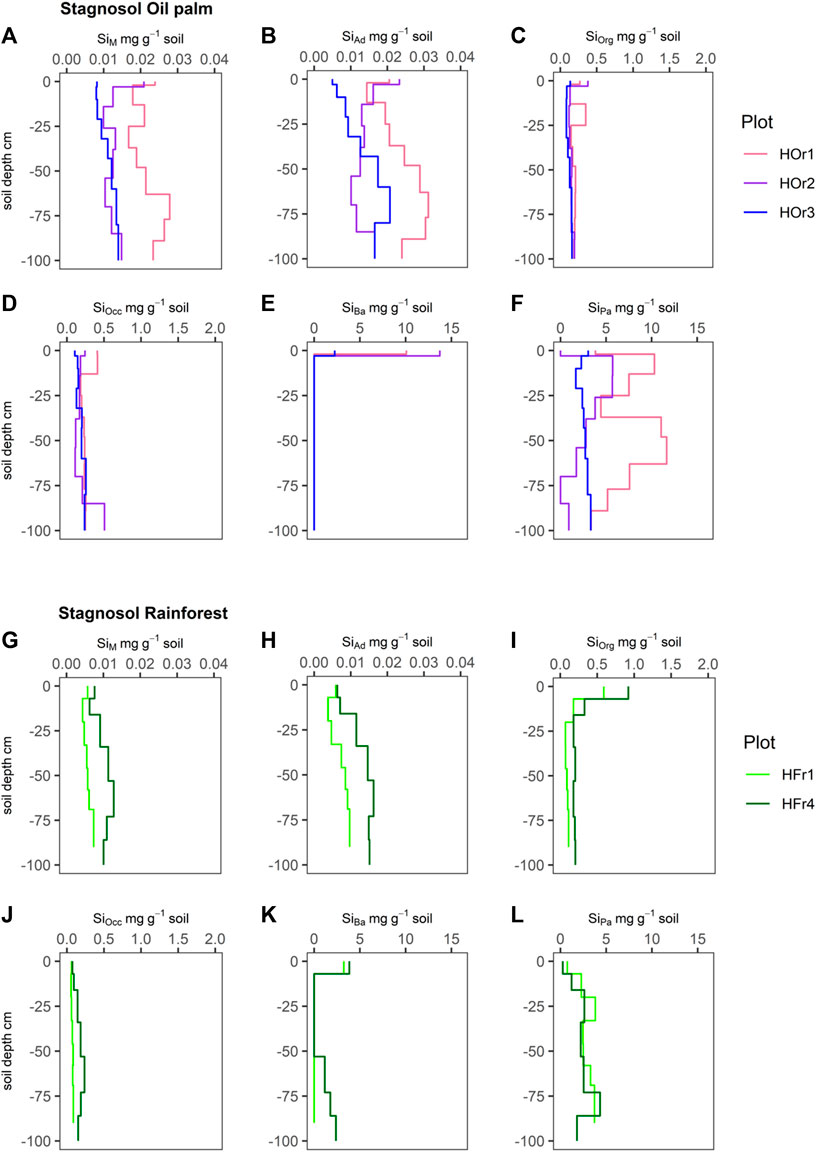
FIGURE 2. Silicon concentrations (mg g-1 soil) of six Si fractions, including SiM = mobile Si (A and G), SiAd = adsorbed Si (B and H), SiOrg = Si bound in soil organic matter (C and I), SiOcc = Si occluded in pedogenic oxides and hydroxides (D and J), SiBa = Si in biogenic amorphous silica (E and K), SiPa = Si in pedogenic amorphous silica (F and L) in Stagnosols under oil-palm plantations (HOr1-3) and rainforest (HFr1, HFr4).
SiOrg concentrations in the Stagnosols under lowland rainforest exhibited clear maxima (∼0.6–0.9 mg g-1) in the topsoils and ranged about ∼0.1–0.2 mg g-1 in the subsoils (Figure 2I). SiOrg concentrations in the Stagnosols under oil-palm plantations showed less clear maxima in the topsoils (∼0.1–0.4 mg g-1) and mostly low values (∼0.1–0.2 mg g-1) in the subsoils, whereby plot HOr1 exhibited a second SiOrg maximum (∼0.4 mg g-1) between 13–25 cm soil depth (Bg horizon) (Figure 2C).
SiOcc concentrations in the Stagnosols under lowland rainforest were low (∼0.06–0.24 mg g-1) throughout the soil profile (Figure 2J). SiOcc concentrations in the Stagnosols under oil-palm plantations were similarly low. Yet, they showed a somewhat more irregular depth distribution, whereby the Stagnosol in plot HOr1 exhibited a maximum in the topsoil (0.4 mg g-1), and the one in plot HOr2 showed a maximum (0.5 mg g-1) at 80–100 cm soil depth, in its Btg2 horizon (Figure 2D).
SiBa concentrations in the Stagnosols under lowland rainforest showed clear maxima (3–4 mg g-1) in the topsoils. The Stagnosol in plot HFr4 showed again increasing SiBa concentrations up to 2.4 mg g-1 from 53 cm downwards (Figure 2K). SiBa concentrations in the Stagnosols under oil-palm plantations showed a very pronounced maximum in the topsoils (up to 13 mg g-1), while SiBa was not at all detectable in any other soil horizons (Figure 2E).
SiPa concentrations in the Stagnosols under lowland rainforest were very low (0–0.25 mg g-1) in the topsoils and varied between 1 mg g-1 and 4 mg g-1 in the subsoils (Figure 2L). SiPa concentrations in the Stagnosols under oil-palm plantations were in a range of 0.1–10 mg g-1 in the topsoils and showed great variability in the subsoils, varying between 2 mg g-1 and 12 mg g-1 (Figure 2F).
4 Discussion
4.1 Has 20 years of oil-palm cultivation caused soil Si depletion?
The soil Si pools participating in the biogeochemical Si cycling within the oil-palm plantations and rainforests that were investigated in this study altogether made up less than 1% of the total Si in soils, which is similar to the proportion in a temperate forest ecosystem (Cornu et al., 2022). All oil-palm plantations established on Acrisols in sloping terrain (Table 1) only showed a non-significant trend of decreased Si stocks of the soil Si fractions SiM, SiAd SiOrg and SiBa (Table 2; Figures 1E,K), but no decrease of the larger pools SiOcc and SiPa. Only at p = 0.06, the decrease in SiOrg stocks under oil-palm plantations differed. The studied oil-palm plantations established on Stagnosols in floodplains (Table 1) tended to have insignificantly decreased stocks of SiOrg and SiBa (Table 2; Figures 2C,E). As no statistically significant differences were observed, our hypothesis that rainforest transformation into oil-palm plantations diminishes soil Si pools in Acrisols and Stagnosols was not verified.
4.1.1 Acrisols
4.1.1.1 Soil SiM, SiAd, and SiOcc stocks per hectare and depth distribution
The slightly lower SiM stocks in Acrisols under oil-palm plantations compared to rainforests were not significant. If they exist, they probably result from low SiM concentrations in the subsoil horizons of the Acrisols under oil-palm plantations (Figures 1A,G). Both rainforest trees (Lucas et al., 1993; Schaller et al., 2018) and oil palms (Munevar and Romero, 2015; Greenshields et al., 2023b) can take up high amounts of Si from soil solution. Yet they differ in their rooting system (vander Linden and Delvaux, 2019). Roots of rainforest trees either immediately exploit forest litter and the uppermost topsoil for nutrients, or tap into strongly weathered, deep subsoil horizons (up to 7 m soil depth) (Lucas et al., 1993; vander Linden and Delvaux, 2019). In contrast, the rooting system of most crops only reaches ∼2 m soil depth and is less dense than the rooting system in a rainforest biome (Corley and Tinker, 2016; vander Linden and Delvaux, 2019). White et al. (2012) assumed that SiM concentrations are similar across a soil profile, if Si uptake rates of a plant are in equilibrium with Si leaching and Si discharge in pore water. Low SiM concentrations throughout the entire soil profile in Acrisols under oil-palm plantations could therefore reflect such equilibrium state (i.e., similar Si uptake and leaching rates), whereas rainforest trees predominantly exploit nutrients in upper horizons, leading to less Si uptake from the lower soil horizons. In addition, water percolation through soils and, hence, leaching of SiM under oil-palm plantations should exceed that under rainforest, being characterized by a dense, a multilayered tree canopy.
Lower SiAd stocks in Acrisols under oil-palm plantations can be attributed to the equally low SiM stocks in Acrisols under oil-palm plantations (Table 2), since SiM and SiAd are in continuous interaction, forming a dynamic equilibrium. Consequently, all Acrisols showed a very similar depth distribution in their SiM and SiAd concentrations across the 1 m deep soil profiles (Figures 1A,B,G,H). Similar to the SiM concentrations, SiAd concentrations were lower and much more variable in Acrisols under oil-palm plantations compared to lowland rainforest. Munevar and Romero (2015) made similar observations and showed that in three strongly weathered soil profiles, concentrations of plant-available Si increased with soil depth and were in a range of 73–90 mg Si kg-1, which is in the same order of magnitude as our SiAd concentrations (30–197 mg Si kg-1). Georgiadis et al. (2017) found concentrations of SiM and SiAd to be strongly influenced by the presence or absence of pedogenic Fe and Al oxides and hydroxides. To a lesser extent, clay minerals may also serve as adsorption sites for SiAd (Georgiadis et al., 2014; 2017). As most Acrisols under oil-palm plantations (Table 1, plots HO2, HO4) and all Acrisols under lowland rainforest (Table 1, plots HF1, HF3 and HF4) showed stagnic properties in their subsoil horizons, the pedogenic oxides and hydroxides in these horizons offer abundant adsorption surfaces for Si in the studied Acrisols (Georgiadis et al., 2017). The relevance of this effect is supported by the observation that SiAd concentrations (Figures 1B,H) show a clearer increase with soil depth than SiM concentrations (Figures 1A,G). In the rainforest, an increase of SiM and SiAd concentrations over soil depth might be linked to an increase in clay content (e.g., HFr3, HF4, Supplementary Table SB1). This observation might also be a reason for higher SiM stocks in rainforest Acrisols compared to rainforest Stagnosols with less clay content difference with soil depth. With a higher clay content, more sorption sites might be available for SiM and SiAd leading to the observed depth distribution.
Earlier studies have shown that a further labile Si pool exists alongside phytoliths, which is activated during litter degradation (Watteau and Villemin, 2001; Schaller and Struyf, 2013). In our study, this additional labile pool is referred to as SiOrg, i.e., SOM-bound Si. Lower SiOrg stocks under oil-palm plantations compared to lowland rainforest may be attributed to plantation management practices that control the presence or absence of ground vegetation in oil-palm plantations. SiOrg showed diminished SiOrg concentrations in four out of five topsoil horizons of Acrisols under oil-palm plantations. In contrast, SiOrg concentrations were high in all topsoil horizons of Acrisols under lowland rainforest (Figures 1C,I). This implies that Acrisol topsoils under rainforest have relevant amounts of soil organic matter, while many Acrisol topsoils under oil-palm plantations contain little or no soil organic matter. These observations correspond well to findings from vander Linden and Delvaux (2019), von der Lühe et al. (2022) and Greenshields et al., 2023. Vander Linden and Delvaux (2019) identified soil Si pools associated to topsoils (mainly phytogenic Si) as important soil Si pools in rainforest biomes. In oil-palm plantations, on the other hand, von der Lühe et al. (2022); Greenshields et al., 2023 found litter return and decomposition to be largely restricted to frond piles areas, which may make up as little as 15% of a plantation (Tarigan et al., 2020). This is a crucial finding, because the decomposition of palm fronds provide most plant-available Si to soils under oil-palm plantations. Litter return on oil-palm plantations is additionally restricted because interrows are commonly kept free of vegetation, which prevents litter production there and promotes topsoil erosion as well as Si leaching from soils (Oliveira et al., 2013; Guillaume et al., 2015; Greenshields et al., 2023).
Increased SiOrg concentrations at greater soil depth in one case under lowland rainforest (Figure 1I, plot HF1) might be related to biological activity or tree uprooting in the past. Soils under tropical rainforest may genuinely be more heterogeneous than soils under plantation systems due to higher biological activity, e.g., by termites (Donovan et al., 2001). The Acrisol in plot HF1 showed a termite borrow at greater soil depth (Supplement, Table A1).
4.1.1.2 Soil SiOcc, SiBa, and SiPa stocks per hectare and depth distribution
High SiOcc stocks in Acrisols may be explained by the abundance of pedogenic oxides and hydroxides (Georgiadis et al., 2017), since almost all studied Acrisols had stagnic properties at some depth, and two Acrisols under oil-palm plantations (HO1 and HOr4) even contained a layer of Fe concretions at 75 and 90 cm soil depth, respectively, coinciding with higher SiOcc concentrations (Figure 1D) compared to the other Acrisols (HO2, HO3, HF1, 3–4) without such layer.
Biogenic amorphous silica pools can be depleted after some decades (Barão et al., 2014; Vandevenne et al., 2015; Unzué-Belmonte et al., 2017) or centuries (Struyf et al., 2010; Clymans et al., 2011) of cultivation. In oil-palm plantations, management practices that result in the presence or absence of cover crops seem to play a crucial role. In our study, the only two Acrisols in oil-palm plantations that had high SiBa concentrations in their topsoil horizons (HO1-2) were covered by grassy understory vegetation, securing phytolith production and return to the topsoils through the grass litter (Figure 1C). In contrast, no SiBa was detected in any of the three oil-palm plots that were situated in cleared oil-palm interrows (HO3-4, HOr4) (Figure 1C, Supplementary Table SA1). Oil-palm plantations contain up to 25% of other plant species, including grasses and sedges (Rembold et al., 2017), which can effectively return phytoliths to soils (Blecker et al., 2006; White et al., 2012; von der Lühe et al., 2022; Greenshields et al., 2023). In addition, protection of the soil surface by cover crops can prevent topsoil erosion. As phytoliths preferentially accumulate in topsoils (Conley et al., 2008), concentrations of SiBa in cleared oil-palm interrows (HO3, HO4, and HOr4) could also have been diminished due to erosion. Guillaume et al. (2015) pointed out that topsoil erosion led to a loss of soil organic carbon (SOC) stocks in oil-palm monocultures (Alexandre et al., 1997; Schaller et al., 2018; Li et al., 2020), where clearing understory vegetation had been part of management practices. Moreover, an assessment of annual soil erosion (by erosion traps in the same 20-year-old oil-palm plantations on sloping terrain), combined with analysis of Si contents of the eroded soil material, pointed to considerable annual Si losses through soil erosion, if the plantations were kept free of ground vegetation (Greenshields et al., 2023b). Soil erosion stopped completely, where ground vegetation was allowed to grow. Thus, maintaining understory vegetation could not only benefit biodiversity (Luke et al., 2019), but also sustain SOC, stocks of nutrients (Guillaume et al., 2015) and biogenic Si.
Genuinely higher SiBa concentrations in the Acrisols under rainforest (Figures 1E,K) can be explained by the presence of phytoliths in the topsoils of all rainforest plots and in the subsoil of the Acrisol in plot HFr3 (Figure 1K). The latter might be explained by a “stable” phytolith pool that may occur at greater soil depth (Lucas et al., 1993; Alexandre et al., 1997; Li et al., 2020), if phytolith solubility has been decreased. Li et al. (2020) reported that phytoliths can be preserved in microaggregates. Alexandre et al. (1997) found preserved (“inactive”) phytoliths in the subsoil of a Ferrasol under a tropical rainforest and explained their presence by vertical translocation. Thereby, the preserved phytoliths made up ≤ 10% of the estimated total phytolith pool. Lucas et al. (1993) and Fujii et al. (2018) suggested that microbial activity and ectomycorrhiza fungi can increase the dissolution of secondary silicates (clays) and phytoliths especially in the uppermost meter of a soil (Figures 1G,K). It is thus possible that a “stable” phytolith pool formed in the subsoil of the Acrisol in plot HFr3 under rainforest, e.g., through tree uprooting, which was then preserved because of the naturally low microbial activity at that depth.
Fairly similar SiPa stocks in Acrisols under oil-palm plantations and lowland rainforest were also reflected in a similar distribution of SiPa concentrations with depth under both LULC systems (Figures 1F,L). Apparently, this fraction is more strongly controlled by other factors than land use. An increase of SiPa concentrations with depth may result from Si release during mineral weathering and dissolution of phytoliths in the topsoils and re-precipitation at some depth, e.g., as siliceous coatings on clay mineral and Fe-oxide surfaces (Lucas et al., 1993; White et al., 2012; Cornelis et al., 2014; Fujii et al., 2018). White et al. (2012) reported that shallow grass roots mainly took up Si from soil solution that had a biogenic Si source, whereas deeper roots predominantly extracted Si from soil solution that originally had a pedogenic Si source. This observation suggests that the SiPa pool, which in our study tended to increase with soil depth, may be also an important soil Si pool, especially for deeper-rooting trees that can exploit this deep pool, and can incorporate it in the Si cycling of the system.
4.1.2 Stagnosols
4.1.2.1 Soil SiOrg and SiBa stocks per hectare and depth distribution
The Stagnosols in the riparian areas showed very similar SiOrg and SiBa stocks per hectare and SiOrg and SiBa depth trends compared to the Acrisols of the higher areas. Both SiOrg and SiBa are linked to the presence and decomposition of litter, which explains that both fractions showed clear maxima in the topsoils. Lower SiOrg stocks per hectare in Stagnosols under oil-palm plantations compared to Stagnosols under rainforest were directly linked with lower SiOrg concentrations in their topsoil horizons (Figures 2C,I). Ah horizons of Stagnosols under oil-palm plantations were not only thinner than under lowland rainforest, but also received less litter. In oil-palm plantations, additional litter is provided by the decay of understory vegetation in oil-palm rows and interrows, if management practices allow this vegetation cover (Albert et al., 2006; Rembold et al., 2017; von der Lühe et al., 2020; Greenshields et al., 2023).
Lower SiBa stocks in Stagnosols under oil-palm plantations compared to lowland rainforest likely result from the shallowness of the (partially eroded) topsoils under oil-palm plantations that contain very high SiBa concentrations, as well as from the absence of any relevant SiBa concentrations in the subsoils of the Stagnosols under oil-palm plantations (Figures 2E,K). Like Acrisols, Stagnosols under lowland rainforest may have an additional “stable” phytolith pool in the subsoil (Alexandre et al., 1997). High SiBa concentrations in the topsoils of Stagnosols under both LULC systems, with maximum SiBa concentrations in the topsoils of Stagnosols under oil-palm plantations, point towards a continuous provision and accumulation of phytoliths in the topsoils (Albert et al., 2006; Greenshields et al., 2023). The high SiBa concentrations in the shallow topsoils of the Stagnosols under oil-palm plantations can be explained by the fact that palm trees are Si accumulators and may thus produce more phytoliths than lowland rainforest trees (Albert et al., 2015). Besides palm trees (e.g., Phoenix reclinata, Hyphaene petersiana, E. guineensis Jacq.), grasses and sedges also produce abundant phytoliths that persist well in soil (Albert et al., 2006), which again points to the importance of a grassy vegetation cover in between the oil-palm rows.
4.1.2.2 Soil SiM, SiAd, SiOcc, and SiPa stocks per hectare and depth distribution
The similarity of the SiM, SiAd, SiOcc and SiPa stocks per hectare suggests that LULC change has barely affected these Si fractions in Stagnosols. The variability in the depth distributions of SiM, SiAd, SiOcc and SiPa among the Stagnosols under oil-palm plantations was greater than the difference in the depth trends of these fractions between Stagnosols under the two LULC systems. This observation suggests that other factors than LULC (e.g., the depth distributions of pedogenic oxides and clay minerals) are more important in controlling these fractions than LULC. The only influence of land use on the depth patterns of the Si fraction was detected for SiPa. The absence of this fraction in the topsoils of all Stagnosols under rainforest (Figure 2L) suggests that in undisturbed soils, the SiPa pool exclusively occurs in the subsoils. SiPa was also absent in the topsoil of the Stagnosol in the oil-palm plot HOr2, but it was present in the topsoils of the Stagnosols in the oil-palm plots HOr1 and HOr3 (Figure 2F), pointing to some anthropogenic mixing of topsoil and subsoil horizons, probably in the course of establishing the oil-palm plantation.
4.1.2.3 Are oil-palm plantations in riparian areas less prone to soil Si depletion?
The only Si fractions that showed insignificantly larger stocks in the Stagnosols of the riparian areas compared to the Acrisols of the higher landscape positions (both under oil-palm plantations), were the SiM and SiAd fractions. These increased SiM and SiAd stocks can be attributed to higher SiM and SiAd concentrations across the whole soil profile (Figures 1A–C; Figures 2A–C). They may be due to SiM influx to the riparian areas with the water that is regularly flooding these areas, and due to higher Si dissolution rates in riparian areas compared to well-drained areas. This latter explanation is supported by earlier studies, where alternating redox conditions in soils explained higher phytolith dissolution rates in riparian areas (Georgiadis et al., 2017; vander Linden and Delvaux, 2019; Greenshields et al., 2023).
5 Conclusion
We conclude that 20 years of oil-palm cultivation has not yet caused soil Si depletion, although the oil palm is a Si accumulating plant, and consequently considerable amounts of Si are exported through fruitbunch harvest each year. Thus, the assumed depletion effect that we investigated was not proven in this study. All oil-palm plantations established on Acrisols in sloping terrain only showed insignificantly decreased Si stocks of mobile Si, adsorbed Si and SOM-bound Si; those established on Stagnosols in riparian areas had insignificantly decreased stocks of SOM-bound Si and biogenic amorphous silica. All soil Si pools together comprised less than 1% of the total Si in the soils. Lower Si stocks of biogenic amorphous Si and SOM-bound Si were mostly attributed to 1) a missing “stable” phytolith pool, 2) very shallow, partially eroded Ah horizons, and 3) less organic matter in soils under oil-palm plantations, implying that topsoils are particularly vulnerable. When comparing well-drained and riparian areas, flooding seemed to increase SiM and SiAd stocks, possibly directly and/or through enhanced phytolith dissolution. As our results lack statistically significant differences, both our hypotheses were not verified for up to 20-year-old oil-palm plantations. However, this does not exclude the risk of Si depletion on a longer term. Amongst others, this long-term risk could arise from topsoil erosion or fruit-bunch harvest. As topsoils comprise the highest concentrations of SOM-bound Si and Si in amorphous silica of biogenic origin under managed oil-palm plantations, it would be advisable to keep a cover crop on the smallholder oil-palm plantations of our study area.
Data availability statement
The raw data supporting the conclusion of this article will be made available by the authors, without undue reservation.
Author contributions
BvL, DS, HH, and AT developed the idea and concept for this project. BG conducted fieldwork and lab work with input from all co-authors. NH contributed complementary soil data. BG wrote the first draft of the manuscript. All authors listed have made a substantial, direct, and intellectual contribution to the work and approved it for publication.
Funding
We thank the German Research Foundation for funding this work (DFG Project No. 391702217). The project was associated to the Collaborative Research Center 990 “Environmental and Socioeconomic Functions of Tropical Lowland Rainforest Transformation Systems” (DFG Project No. 192626868).
Acknowledgments
We thank the Ministry of Forestry, Technology and Higher Education (RISTEKDIKTI), the Indonesian Institute of Sciences (LIPI) and the Ministry of Forestry (PHKA) for granting the research permit 110/SIP/FRP/E5/Dit.KI/IV/2018 to conduct the soil sampling for this project. We thank our Indonesian counterparts, the CRC-990 office members, our fieldwork assistants and the laboratory staff of the Institute of Geography, Dr. Jürgen Grotheer, Petra Voigt and Anja Södje. Without the support of all these colleagues, this work would not have been possible. We thank Thomas Guillaume for providing complementary soil data of oil-palm plantation sites.
Conflict of interest
The authors declare that the research was conducted in the absence of any commercial or financial relationships that could be construed as a potential conflict of interest.
Publisher’s note
All claims expressed in this article are solely those of the authors and do not necessarily represent those of their affiliated organizations, or those of the publisher, the editors and the reviewers. Any product that may be evaluated in this article, or claim that may be made by its manufacturer, is not guaranteed or endorsed by the publisher.
Supplementary material
The Supplementary Material for this article can be found online at: https://www.frontiersin.org/articles/10.3389/fenvs.2023.1189502/full#supplementary-material
References
Albert, R. M., Bamford, M. K., and Cabanes, D. (2006). Taphonomy of phytoliths and macroplants in different soils from Olduvai Gorge (Tanzania) and the application to Plio-Pleistocene palaeoanthropological samples. Quatern. Int. 148, 78–94. doi:10.1016/j.quaint.2005.11.026
Albert, R. M., Bamford, M. K., and Esteban, I. (2015). Reconstruction of ancient palm vegetation landscapes using a phytolith approach. Quatern. Int. 369, 51–66. doi:10.1016/j.quaint.2014.06.067
Alexandre, A., Meunier, J.-D., Colin, F., and Koud, J.-M. (1997). Plant impact on the biogeochemical cycle of silicon and related weathering processes. Geochim. Cosmochim. Acta 61, 677–682. doi:10.1016/S0016-7037(97)00001-X
Allen, K., Corre, M. D., Kurniawan, S., Utami, S. R., and Veldkamp, E. (2016). Spatial variability surpasses land-use change effects on soil biochemical properties of converted lowland landscapes in Sumatra, Indonesia. Geoderma 284, 42–50. doi:10.1016/j.geoderma.2016.08.010
Allen, K., Corre, M. D., Tjoa, A., and Veldkamp, E. (2015). Soil nitrogen-cycling responses to conversion of lowland forests to oil palm and rubber plantations in Sumatra, Indonesia. PLoS ONE 10, e0133325. doi:10.1371/journal.pone.0133325
Barão, L., Clymans, W., Vandevenne, F., Meire, P., Conley, D. J., and Struyf, E. (2014). Pedogenic and biogenic alkaline-extracted silicon distributions along a temperate land-use gradient. Eur. J. Soil Sci. 65, 693–705. doi:10.1111/ejss.12161
Blecker, S. W., McCulley, R. L., Chadwick, O. A., and Kelly, E. F. (2006). Biologic cycling of silica across a grassland bioclimosequence. Glob. Biogeochem. Cycles 20, GB3023. doi:10.1029/2006GB002690
Carey, J. C., and Fulweiler, R. W. (2016). Human appropriation of biogenic silicon – The increasing role of agriculture. Funct. Ecol. 30, 1331–1339. doi:10.1111/1365-2435.12544
Clough, Y., Krishna, V. v., Corre, M. D., Darras, K., Denmead, L. H., Meijide, A., et al. (2016). Land-use choices follow profitability at the expense of ecological functions in Indonesian smallholder landscapes. Nat. Commun. 7, 13137–13212. doi:10.1038/ncomms13137
Clymans, W., Struyf, E., Govers, G., Vandevenne, F., and Conley, D. J. (2011). Anthropogenic impact on amorphous silica pools in temperate soils. Biogeosciences 8, 2281–2293. doi:10.5194/bg-8-2281-2011
Conley, D. J., Likens, G. E., Buso, D. C., Saccone, L., Bailey, S. W., and Johnson, C. E. (2008). Deforestation causes increased dissolved silicate losses in the Hubbard Brook Experimental Forest. Glob. Chang. Biol. 14, 2548–2554. doi:10.1111/j.1365-2486.2008.01667.x
Corley, R. H. V., and Tinker, P. B. H. (2016): The oil palm (world agricultural series). Chichester, UK: Wiley Blackwell fifth edition, 647. doi:10.1017/cbo9781316530122.010
Cornelis, J., Delvaux, B., Georg, R. B., Lucas, Y., Ranger, J., and Opfergelt, S. (2011). Tracing the origin of dissolved silicon transferred from various soil-plant systems towards rivers: A review. Biogeosciences 8, 89–112. doi:10.5194/bg-8-89-2011
Cornelis, J. T., Weis, D., Lavkulich, L., Vermeire, M. L., Delvaux, B., and Barling, J. (2014). Silicon isotopes record dissolution and re-precipitation of pedogenic clay minerals in a podzolic soil chronosequence. Geoderma 235–236, 19–29. doi:10.1016/j.geoderma.2014.06.023
Cornu, S., Meunier, J. D., Ratie, C., Ouedraogo, F., Lucas, Y., Merdy, P., et al. (2022). Allophanes, a significant soil pool of silicon for plants. Geoderma 412, 115722. doi:10.1016/j.geoderma.2022.115722
Darras, K. F. A., Corre, M. D., Formaglio, G., Tjoa, A., Potapov, A., Brambach, F., et al. (2019). Reducing fertilizer and avoiding herbicides in oil palm plantations—ecological and economic valuations. Front. For. Glob. Change 2, 1–15. doi:10.3389/ffgc.2019.00065
de Coster, G. L. (2006) The Geology of the central and south Sumatra basins, in: IPA, 2006 - 3rd annual convention proceedings, Indonesia: Indonesian Petroleum Association.
de Tombeur, F., Turner, B. L., Laliberté, E., Lambers, H., Mahy, G., Faucon, M. P., et al. (2020). Plants sustain the terrestrial silicon cycle during ecosystem retrogression. Science 369, 1245–1248. doi:10.1126/science.abc0393
Derry, L. A., Kurtz, A. C., Ziegler, K., and Chadwick, O. A. (2005). Biological control of terrestrial silica cycling and export fluxes to watersheds. Nature 433, 728–731. doi:10.1038/nature03299
DIN ISO 14869-2 (2003). Soil quality — dissolution for the determination of total element content — Part 2: Dissolution by alkaline fusion. Chichester, UK: Wiley Blackwell.
Dislich, C., Keyel, A. C., Salecker, J., Kisel, Y., Meyer, K. M., Auliya, M., et al. (2017). A review of the ecosystem functions in oil palm plantations, using forests as a reference system. Biol. Rev. 92, 1539–1569. doi:10.1111/brv.12295
Donovan, S. E., Eggleton, P., Dubbin, W. E., Batchelder, M., and Dibog, L. (2001). The effect of a soil-feeding termite, cubitermes fungifaber (isoptera: Termitidae) on soil properties: Termites may be an important source of soil microhabitat heterogeneity in tropical forests. Pedobiologia 45, 1–11. doi:10.1078/0031-4056-00063
Drescher, J., Rembold, K., Allen, K., Beckscha, P., Buchori, D., Clough, Y., et al. (2016). Ecological and socio-economic functions across tropical land use systems after rainforest conversion. Philos. Trans. Roy. Soc. B 371, 20150275. doi:10.1098/rstb.2015.0275
Epstein, E. (1999). Silicon. Annu. Rev. Plant Physiol. Plant Mol. Biol. 50, 641–664. doi:10.1146/annurev.arplant.50.1.641
Epstein, E. (1994). The anomaly of silicon in plant biology. Proc. Natl. Acad. Sci. 91, 11–17. doi:10.1073/pnas.91.1.11
Euler, M., Schwarze, S., Siregar, H., and Qaim, M. (2016). Oil palm expansion among smallholder farmers in Sumatra, Indonesia. J. Agric. Econ. 67, 658–676. doi:10.1111/1477-9552.12163
FAO (2020). Oilseeds, oils & meals. Monthly price and policy update. Food Agric. Organ. United Nations. No. 136, 2–12.
Fox, J., and Weisberg, S. (2019) An {R} companion to applied regression. Available at: https://socialsciences.mcmaster.ca/jfox/Books/Companion/[Accessed February 10 2023]
Fujii, K., Shibata, M., Kitajima, K., Ichie, T., Kitayama, K., and Turner, B. L. (2018). Plant–soil interactions maintain biodiversity and functions of tropical forest ecosystems. Ecol. Res. 33, 149–160. doi:10.1007/s11284-017-1511-y
Gatto, M., Wollni, M., and Qaim, M. (2015). Oil palm boom and land-use dynamics in Indonesia: The role of policies and socioeconomic factors. Land use policy 46, 292–303. doi:10.1016/j.landusepol.2015.03.001
Georgiadis, A., Rinklebe, J., Straubinger, M., and Rennert, T. (2017). Silicon fractionation in mollic fluvisols along the central elbe river, Germany. Catena 153, 100–105. doi:10.1016/j.catena.2017.01.027
Georgiadis, A., Sauer, D., Herrmann, L., Breuer, J., Zarei, M., and Stahr, K. (2013). Development of a method for sequential Si extraction from soils. Geoderma 209, 251–261. –210. doi:10.1016/j.geoderma.2013.06.023
Georgiadis, A., Sauer, D., Herrmann, L., Breuer, J., Zarei, M., and Stahr, K. (2014). Testing a new method for sequential silicon extraction on soils of a temperate-humid climate. Soil Res. 52, 645–657. doi:10.1071/SR14016
Giraudoux, P. (2022) pgirmess: Spatial analysis and data mining for field ecologists, Available at: https://CRAN.R-project.org/package=pgirmess [Accessed February 10, 2023]
Grass, I., Kubitza, C., Krishna, V. v., Corre, M. D., Mußhoff, O., Pütz, P., et al. (2020). Trade-offs between multifunctionality and profit in tropical smallholder landscapes. Nat. Commun. 11, 1186–1213. doi:10.1038/s41467-020-15013-5
Grasshoff, K., Kremling, K., and Ehrhardt, M. (2009). Methods of sea water analysis. Weinheim: Wiley VCH.
Greenshields, B., von der Lühe, B., Hughes, H. J., Stiegler, C., Tarigan, S., Tjoa, A., et al. (2022a). Oil-palm management alters the spatial distribution of amorphous silica and mobile silicon in topsoils. SOIL 9, 169–188. doi:10.5194/soil-9-169-2023
Greenshields, B., von der Lühe, B., Schwarz, F., Hughes, H. J., Tjoa, A., Kotowska, M., et al. (2023b). Estimating oil-palm Si storage, Si return to soils and Si losses through harvest in smallholder oil-palm plantations of Sumatra, Indonesia. Biogeosciences 20, 1259–1276. doi:10.5194/bg-20-1259-2023
Grosjean, P., and Ibanez, F. (2018) pastecs: Package for analysis of space-time ecological series, Available at: https://CRAN.R-project.org/package=pastecs. [Accessed February 5, 2023]
Guillaume, T., Damris, M., and Kuzyakov, Y. (2015). Losses of soil carbon by converting tropical forest to plantations: Erosion and decomposition estimated by δ13C. Glob. Chang. Biol. 21, 3548–3560. doi:10.1111/gcb.12907
Guntzer, F., Keller, C., Poulton, P. R., McGrath, S. P., and Meunier, J. D. (2012). Long-term removal of wheat straw decreases soil amorphous silica at Broadbalk, Rothamsted. Plant Soil 352, 173–184. doi:10.1007/s11104-011-0987-4
Harrison, R. D., and Swinfield, T. (2015). Restoration of logged humid tropical forests: An experimental programme at harapan rainforest, Indonesia. Trop. Conserv. Sci. 8, 4–16. doi:10.1177/194008291500800103
Haynes, R. J. (2017). The nature of biogenic Si and its potential role in Si supply in agricultural soils. Agric. Ecosyst. Environ. 245, 100–111. doi:10.1016/j.agee.2017.04.021
Hughes, H. J., Hung, D. T., and Sauer, D. (2020). Silicon recycling through rice residue management does not prevent silicon depletion in paddy rice cultivation. Nutr. Cycl. Agroecosyst. 118, 75–89. doi:10.1007/s10705-020-10084-8
IUSS Working Group WRB (2022). World Reference Base for Soil Resources. International soil classification system for naming soils and creating legends for soil maps. 4 edition. Vienna, Austria: International Union of Soil Sciences.
Koning, E., Epping, E., and Van Raaphorst, W. (2002). Determining biogenic silica in marine samples by tracking silicate and aluminium concentrations in alkaline leaching solutions. Aquat. Geochem. 8, 37–67. doi:10.1023/A:1020318610178
Kotowska, M. M., Leuschner, C., Triadiati, T., Meriem, S., and Hertel, D. (2015). Quantifying above- and belowground biomass carbon loss with forest conversion in tropical lowlands of Sumatra (Indonesia). Glob. Chang. Biol. 21, 3620–3634. doi:10.1111/gcb.12979
Kurniawan, S., Corre, M. D., Matson, A. L., Schulte-Bisping, H., Utami, S. R., Van Straaten, O., et al. (2018). Conversion of tropical forests to smallholder rubber and oil palm plantations impacts nutrient leaching losses and nutrient retention efficiency in highly weathered soils. Biogeosciences 15, 5131–5154. doi:10.5194/bg-15-5131-2018
Laumonier, Y., and Seameo-Biotrop, (1997). The vegetation and physiography of Sumatra. Dordrecht: Springer.
Li, Z., de Tombeur, F., vander Linden, C., Cornelis, J. T., and Delvaux, B. (2020). Soil microaggregates store phytoliths in a sandy loam. Geoderma 360, 114037. doi:10.1016/j.geoderma.2019.114037
Liang, Y., Nikolic, M., Bélanger, R., Gong, H., and Song, A. (2015). Silicon in agriculture. Dordrecht: Springer.
Lucas, Y., Luizão, F. J., Chauvel, A., Rouiller, J., and Nahon, D. (1993). The relation between biological activity of the rain forest and mineral composition of soils. Science 260, 521–523. doi:10.1126/science.260.5107.521
Luke, S. H., Purnomo, D., Advento, A. D., Aryawan, A. A. K., Naim, M., Pikstein, R. N., et al. (2019). Effects of understory vegetation management on plant communities in oil palm plantations in Sumatra, Indonesia. Front. For. Glob. Change 2, 1–13. doi:10.3389/ffgc.2019.00033
Ma, J. F., and Takahashi, E. (2002). “Silicon-accumulating plants in the plant kingdom,” in Soil, fertilizer, and plant silicon research in Japan Editors J. F. Ma, and E. Takahashi E (Amsterdam: Elsevier), 63–71.
Matichenkov, V. v., and Calvert, D. v. (2002). Silicon as a beneficial element for sugarcane. J. Am. Soc. Sugarcane Technol. 22, 21–29.
McCarthy, J. F., and Cramb, R. A. (2009). Policy narratives, landholder engagement, and oil palm expansion on the Malaysian and Indonesian frontiers. Geogr. J. 175, 112–123. doi:10.1111/j.1475-4959.2009.00322.x
Munevar, F., and Romero, A. (2015). Soil and plant silicon status in oil palm crops in Colombia. Exp. Agric. 51, 382–392. doi:10.1017/S0014479714000374
Najihah, N. I., Hanafi, M. M., Idris, A. S., and Hakim, M. A. (2015). Silicon treatment in oil palms confers resistance to basal stem rot disease caused by Ganoderma boninense. Crop Prot. 67, 151–159. doi:10.1016/j.cropro.2014.10.004
Nazarreta, R., Hartke, T. R., Hidayat, P., Scheu, S., Buchori, D., and Drescher, J. (2020). Rainforest conversion to smallholder plantations of rubber or oil palm leads to species loss and community shifts in canopy ants (Hymenoptera: Formicidae). Myrmecol. News 30, 175–186. doi:10.25849/myrmecol.news_030:175
Oliveira, P. T. S., Wendland, E., and Nearing, M. A. (2013). Rainfall erosivity in Brazil: A review. Catena 100, 139–147. doi:10.1016/j.catena.2012.08.006
Parr, J. F., Lentfer, C. J., and William, E. B. (2001). A comparative analysis of wet and dry ashing techniques for the extraction of phytoliths from plant material. J. Archaeol. Sci. 28, 875–886. doi:10.1006/jasc.2000.0623
Puppe, D., Kaczorek, D., Schaller, J., Barkusky, D., and Sommer, M. (2021). Crop straw recycling prevents anthropogenic desilication of agricultural soil–plant systems in the temperate zone – results from a long-term field experiment in NE Germany. Geoderma 403, 115187–115213. doi:10.1016/j.geoderma.2021.115187
Rembold, K., Mangopo, H., Tjitrosoedirdjo, S. S., and Kreft, H. (2017). Plant diversity, forest dependency, and alien plant invasions in tropical agricultural landscapes. Biol. Conserv. 213, 234–242. doi:10.1016/j.biocon.2017.07.020
Revelle, W. (2022) psych: Procedures for personality and psychological research. Available at: https://CRAN.R-project.org/package=psych. [Accessed February 10, 2023]
Sauer, D., Saccone, L., Conley, D. J., Herrmann, L., and Sommer, M. (2006). Review of methodologies for extracting plant-available and amorphous Si from soils and aquatic sediments. Biogeochemistry 80, 89–108. doi:10.1007/s10533-005-5879-3
Sauer, D., Stein, C., Glatzel, S., Kühn, J., Zarei, M., and Stahr, K. (2015). Duricrusts in soils of the Alentejo (southern Portugal) - types, distribution, Genesis and time of their formation. J. Soil Sedim 15 (6), 1437–1453. doi:10.1007/s11368-015-1066-x
Schaller, J., Puppe, D., Kaczorek, D., Ellerbrock, R., and Sommer, M. (2021). Silicon cycling in soils revisited. Plants 10, 1–33. doi:10.3390/plants10020295
Schaller, J., and Struyf, E. (2013). Silicon controls microbial decay and nutrient release of grass litter during aquatic decomposition. Hydrobiologia 709, 201–212. doi:10.1007/s10750-013-1449-1
Schaller, J., Turner, B. L., Weissflog, A., Pino, D., Bielnicka, A. W., and Engelbrecht, B. M. J. (2018). Silicon in tropical forests: Large variation across soils and leaves suggests ecological significance. Biogeochemistry 140, 161–174. doi:10.1007/s10533-018-0483-5
Shull, K. E., and Guthan, G. R. (1967). Rapid modified eriochrome cyanine R method for determination of aluminum in water. J. Am. Water Works Assoc. 59, 1456–1468. doi:10.1002/j.1551-8833.1967.tb03476.x
Sirisuntornlak, N., Ullah, H., Sonjaroon, W., Anusontpornperm, S., Arirob, W., and Datta, A. (2020). Interactive effects of silicon and soil pH on growth, yield and nutrient uptake of maize. Silicon 13, 289. doi:10.1007/s12633-020-00427-z
Sommer, M., Jochheim, H., Höhn, A., Breuer, J., Zagorski, Z., Busse, J., et al. (2013). Si cycling in a forest biogeosystem – The importance of transient state biogenic Si pools. Biogeosciences 10, 4991–5007. doi:10.5194/bg-10-4991-2013
Sommer, M., Kaczorek, D., Kuzyakov, Y., and Breuer, J. (2006). Silicon pools and fluxes in soils and landscapes - a review. J. Plant Nutr. Soil Sci. 169, 310–329. doi:10.1002/jpln.200521981
Struyf, E., Smis, A., Van Damme, S., Garnier, J., Govers, G., Van Wesemael, B., et al. (2010). Historical land use change has lowered terrestrial silica mobilization. Nat. Commun. 1, 129. doi:10.1038/ncomms1128
Tarigan, S., Stiegler, C., Wiegand, K., Knohl, A., and Murtilaksono, K. (2020). Relative contribution of evapotranspiration and soil compaction to the fluctuation of catchment discharge: Case study from a plantation landscape. Hydrological Sci. J. 65, 1239–1248. doi:10.1080/02626667.2020.1739287
Tsujino, R., Yumoto, T., Kitamura, S., Djamaluddin, I., and Darnaedi, D. (2016). History of forest loss and degradation in Indonesia. Land use policy 57, 335–347. doi:10.1016/j.landusepol.2016.05.034
Unzué-Belmonte, D., Ameijeiras-Mariño, Y., Opfergelt, S., Cornelis, J. T., Barão, L., Minella, J., et al. (2017). Land use change affects biogenic silica pool distribution in a subtropical soil toposequence. Solid earth. 8, 737–750. doi:10.5194/se-8-737-2017
vander Linden, C., and Delvaux, B. (2019). The weathering stage of tropical soils affects the soil-plant cycle of silicon, but depending on land use. Geoderma 351, 209–220. doi:10.1016/j.geoderma.2019.05.033
Vandevenne, F., Barão, L., Ronchi, B., Govers, G., Meire, P., Kelly, E. F., et al. (2015). Silicon pools in human impacted soils of temperate zones. Glob. Biogeochem. Cycles 29, 1439–1450. doi:10.1002/2014GB005049
Vandevenne, F., Struyf, E., Clymans, W., and Meire, P. (2012). Agricultural silica harvest: Have humans created a new loop in the global silica cycle? Front. Ecol. Environ. 10, 243–248. doi:10.1890/110046
von der Lühe, B., Bezler, K., Hughes, H. J., Greenshields, B., Tjoa, A., and Sauer, D. (2022) Ultrastructural study of the biogeochemical cycle of silicon in the soil and litter of a temperate forest: Ultrastructural study of the silicon cycle. Silicon 13, doi:10.1007/s12633-022-02066-y
von der Lühe, B., Pauli, L., Greenshields, B., Hughes, H. J., Tjoa, A., and Sauer, D. (2020). Transformation of lowland rainforest into oil-palm plantations and use of fire alter topsoil and litter silicon pools and fluxes. Silicon 13, 4345–4353. doi:10.1007/s12633-020-00680-2
Watteau, F., and Villemin, G. (2001). Ultrastructural study of the biogeochemical cycle of silicon in the soil and litter of a temperate forest. Eur. J. Soil Sci. 52, 385–396. doi:10.1046/j.1365-2389.2001.00391.x
White, A. F., Vivit, D. v., Schulz, M. S., Bullen, T. D., Evett, R. R., and Aagarwal, J. (2012). Biogenic and pedogenic controls on Si distributions and cycling in grasslands of the Santa Cruz soil chronosequence, California. Geochim. Cosmochim. Acta 94, 72–94. doi:10.1016/j.gca.2012.06.009
Wickham, H., François, R., Henry, L., Müller, K., and Vaughan, D. (2023) Dplyr: A grammer of data manipulation, Available at: https://CRAN.R-project.org/package=dplyr [Accessed February 10, 2023]
Woittiez, L. S., Turhina, S. R. I., Deccy, D., Slingerland, M., Van Noordwijk, M., and Giller, K. E. N. E. (2019). Fertiliser application practices and nutrient deficiencies in smallholder oil palm plantations in Indonesia. Exp. Agric. 55, 543–559. doi:10.1017/S0014479718000182
Keywords: oil-palm plantation, rainforest, land-use/land-cover (LULC), silicon pools, silicon extraction, tropical soil
Citation: Greenshields B, von der Lühe B, Hughes H, Tjoa A, Hennings N and Sauer D (2023) Effects of turning rainforest into oil-palm plantations on silicon pools in soils within the first 20 years after the transformation. Front. Environ. Sci. 11:1189502. doi: 10.3389/fenvs.2023.1189502
Received: 19 March 2023; Accepted: 10 May 2023;
Published: 25 May 2023.
Edited by:
Jonas Schoelynck, University of Antwerp, BelgiumCopyright © 2023 Greenshields, von der Lühe, Hughes, Tjoa, Hennings and Sauer. This is an open-access article distributed under the terms of the Creative Commons Attribution License (CC BY). The use, distribution or reproduction in other forums is permitted, provided the original author(s) and the copyright owner(s) are credited and that the original publication in this journal is cited, in accordance with accepted academic practice. No use, distribution or reproduction is permitted which does not comply with these terms.
*Correspondence: Barbara von der Lühe, QmFyYmFyYS52b24tZGVyLWx1ZWhlQHVuaS1tdWVuc3Rlci5kZQ==