- 1Qilu University of Technology (Shandong Academy of Sciences), Shandong Analysis and Test Center, Jinan, Shandong, China
- 2Faculty of Environmental Science and Engineering, Qilu University of Technology (Shandong Academy of Sciences), Jinan, Shandong, China
- 3Liaocheng People’s Hospital, Liaocheng, Shandong, China
- 4School of Food and Biological Engineering, Jiangsu University, Zhenjiang, China
- 5Botany and Microbiology Department, Faculty of Science, Tanta University, Tanta, Egypt
Soil salinization is a serious ecological problem. Bacteria and cyanobacteria both have great potential for saline-alkali soil improvement. However, the effect of co-applying bacteria and cyanobacteria on soil improvement and crop growth promotion in saline-alkali soil remains unclear. In this study, the effects of Paenibacillus sabinae (potassium-solubilizing bacteria) and Leptolyngbya sp. RBD05 (cyanobacteria), produced in brewery wastewater, on soil properties, wheat growth, and wheat stress tolerance were studied by applying them to saline-alkali soil alone or in combination. The study indicated that P. sabinae and Leptolyngbya sp. RBD05 have important roles in increasing wheat growth, N:P ratio, K:Na ratio, proline content, and superoxide dismutase activity, as well as in slowing the decline of soil nutrient content caused by wheat absorption. Compared to the control group, the co-application had the best effect on soil available K content, wheat dry weight, and wheat root length (increased by 26%, 85%, and 70%, respectively); and it was more conducive to promoting the wheat K:Na ratio (increased by 41%), which would better improve the wheat’s saline-alkali stress tolerance. This study provided a new and clean strategy to improve saline-alkali soil quality and promote crop growth by the bacteria and cyanobacteria produced from wastewater treatment.
1 Introduction
In recent years, the synergistic treatment of wastewater by bacteria and microalgae (including cyanobacteria) has attracted more and more attention due to its high efficiency (Aditya et al., 2022). But how to make full use of the bacteria and microalgae biomass produced in the wastewater treatment process still needs further development. Generally, the biomass of bacteria and microalgae is usually sent to waste treatment plants as solid waste, which results in significant disposal costs and resource waste. A large amount of wastewater is produced during beer brewing. It is estimated that about 10 L of wastewater were produced for every 1 L of beer (Simate et al., 2011). Unlike industrial wastewater, brewery wastewater contains almost no toxic and harmful substances and contains a lot of nutrients, proteins, minerals, and trace elements (Ashraf et al., 2021). Therefore, the bacteria and microalgae biomass produced in the process of brewery wastewater treatment has great application potential.
There are many saline-alkali fields around the world (Kumar and Sharma, 2020). Soil properties and crop growth are seriously affected by soil salinization (Aycan et al., 2021). It is critical to develop, utilize, and improve saline-alkali land on a global scale to meet the growing food demand. Great achievements have been made by physical and chemical means in the process of saline-alkali soil improvement. However, defects such as a large amount of work and high costs have also been noted (Tejada et al., 2006). The use of microorganisms to improve soil properties, improve the fertilizer utilization rate, and promote the crop growth (Ramadoss et al., 2013), has attracted increasing attention (Renuka et al., 2016). The bacteria and microalgae biomass produced in the process of wastewater treatment might be a good source for saline-alkali soil improvement (Ferreira et al., 2019).
Potassium-solubilizing bacteria (KSB), growing well in wastewater (Wang et al., 2022), can transform the soil insoluble potassium (K) into a soluble state that can be absorbed by plants (Etesami et al., 2017). It is promising to make full use of the potassium-dissolving property of KSB to release mineral K into soil for plant use. In addition, some studies indicated that KSB could secrete plant growth-promoting hormones that promote growth and stress tolerance of plants as well (Sanyal et al., 2020). Ashfaq et al. (2020) reported that the rice plants growth under a saline-alkali environment was improved, the utilization rate of K was increased, and the harmful effect of salt on rice was reduced significantly by the application of Acinetobacter pittii. The expression of antioxidase-related genes was enhanced, and the superoxide dismutase (SOD) activity was significantly increased by the application of Bacillus mojavensis, which then enhanced corn salt tolerance (Feng et al., 2019). The growth of apple seedlings and the absorption of K by apple seedlings were promoted by the phytohormones produced by Paenibacillus mucilaginosus (Chen et al., 2020).
Microalgae, especially cyanobacteria, are photosynthetic autotrophic microorganisms that can survive under harsh conditions and have an effective role in wastewater treatment and soil remediation (Singh et al., 2016). In particular, nitrogen fixation by cyanobacteria has been widely used. Karthikeyan et al. (2007) reported that Calothrix ghosei promoted wheat growth by producing somatotropin and fixing atmospheric nitrogen. Microalgae have also received more attention for their role in the remediation of saline-alkali soil. For example, the absorption of salt by Anabaena sp. in soil could reduce the impact of salt on plants (Mahanty et al., 2017). The saline-alkali soil fertility could be improved by some nitrogen-fixing microalgae (Li et al., 2019).
Owing to chemical substances exchange, cell signal transduction, and gene transfer between bacteria and microalgae (Aditya et al., 2022), the application of bacteria-microalgae symbiosis for environmental remediation is often better than the application of a single microorganism, particularly in wastewater treatment (Kohler et al., 2007; Zhang et al., 2011). Previous studies have shown that the bacteria and microalgae symbiosis system has been widely used to treat nutrient-rich wastewater, and the microalgae and bacteria system has greater advantages in the nutrient removal of wastewater (Kim et al., 2014). In addition, bacteria could contribute to the flocculation of microalgae, effectively and better harvest microalgae bacterial biomass, and reduce the harvest cost (Lee et al., 2013). The symbiosis of bacteria and microalgae also showed higher efficiency when using biological measures to deal with environmental pollution. Tang et al. (2010) found that the symbiosis of bacteria and algae significantly promoted the degradation ability of oil pollutant-degrading bacteria, especially the ability of biodegradable polycyclic aromatic hydrocarbons (PAHs). The study by Subashchandrabose et al. (2013) also proved the effective use of bacteria and microalgae symbiosis in the degradation of methyl parathion, furan, and other agricultural chemicals. In addition, bacterial communities and microalgae detoxify each other, making bacteria and microalgae have stronger tolerances to higher concentrations of heavy metal content, and therefore the bacteria and microalgae symbiosis has a higher removal rate in the treatment of heavy metal pollution (Muñoz and Guieysse, 2006). Recently, the role of co-application of bacteria and microalgae in soil quality improvement has received increasing attention. For example, the growth and yield of chickpea were significantly improved by the use of Anabaena–Rhizobium biofilm (Bidyarani et al., 2016). The use of nitrogen fertilizer could be reduced by the co-use of rhizosphere growth-promoting bacteria and cyanobacteria in normal soil (Rana et al., 2012). The co-application of Brevundimonas sp. and Anabaena sp. had a positive impact in terms of increasing rice growth and grain yield, as well as improving paddy soil health (Prasanna et al., 2012). However, up to date, the effects of the co-application of bacteria and microalgae on saline-alkali soil improvement and crop growth are still not clear.
In this study, the bacterium (Paenibacillus sabinae) and cyanobacterium (Leptolyngbya sp. RBD05) produced in the process of brewery wastewater treatment were applied to saline-alkali soil alone or jointly. The goal was to investigate their effects on the chemical properties and nutrient composition of saline-alkali soil, as well as on the growth status and stress resistance of wheat in a saline-alkali environment. This study was expected to provide a clean, sustainable, and green method for the improvement of saline-alkali soil and the further utilization of bacteria and cyanobacteria produced in wastewater treatment.
2 Materials and methods
2.1 The bacteria strain and cyanobacteria strain
The P. sabinae (potassium-solubilizing bacterium) and Leptolyngbya sp. RBD05 (cyanobacterium) were isolated by our laboratory from a wheat field in the Yellow River Delta, China, characterized by coastal saline-alkali soil. In the experiment of treating brewery wastewater (unpublished), it was found that both P. sabinae and Leptolyngbya sp. RBD05 showed strong salt tolerance (up to 2% salinity level). In addition, P. sabinae showed the ability to solve K from K-feldspar, and Leptolyngbya sp. RBD05 showed the ability to fix atmospheric N2. Therefore, it was appropriate to apply these two microorganisms to the saline-alkali soil. A pH meter (PHS-3C, Shanghai Yidian Scientific Instrument Co., LTD) was used to directly measure the pH of brewery wastewater. After the brewery wastewater was filtered by 0.45 μm filter membrane, the DOC (dissolved organic matter) in the brewery wastewater sample was analyzed by a total organic carbon analyzer, and the NO3-N was determined by high-performance liquid chromatography. According to the national standard of the People’s Republic of China, the salinity of brewery wastewater was determined by the gravimetric method (HJ/T 51-1999); the COD (chemical oxygen demand) in water was determined by the standard potassium dichromate method (GB 11914-1989); the NH4-N was determined by Nath reagent spectrophotometry (HJ 535-2009); the PO43--P was determined by ammonium molybdate spectrophotometry (GB/T 9727-2007); the total nitrogen (TN) was determined by ultraviolet spectrophotometry with potassium persulfate digestion (HJ 636-2012); the total phosphorus (TP) was determined by ammonium molybdate spectrophotometry (GB 11893-89); and the heavy metal content was determined by atomic absorption spectrometry (GB/T 9735-2008). The main characteristics of brewery wastewater are shown in Table 1. P. sabinae and Leptolyngbya sp. RBD05 were inoculated into sterilized brewery wastewater for culture, respectively. The cultural conditions were as follows: The diurnal-night cycle was 12/12 h, the temperature was 28°C, and the light intensity was 2000 lux. Culture of bacterial solution for 3 days and cyanobacterial solution for 7 days. The brewery wastewater containing P. sabinae and Leptolyngbya sp. RBD05 was harvested at the log phase by centrifuging at 10,000 rpm for 5 min, the supernatant was removed, and the bacterium and cyanobacterium were then resuspended in sterile deionized water. This process was repeated 3 times to obtain a live inoculum. Part of the live inoculum was sterilized at 121°C for 30 min to obtain the inactivated inoculum.
2.2 Design and analysis of the pot experiments
The wheat pot experiment was divided into the following groups: the P. sabinae group (JK), the Leptolyngbya sp. RBD05 group (CL), the P. sabinae + Leptolyngbya sp. RBD05 group (JKCL), and the control group (CK). Fifteen pots were set up in each group. Potting soil comes from the same source as the soil used for screening bacteria and microalgae. The soil was directly used without treatment. The basic soil properties were determined in the same way as the experimental soil samples collected below. The wheat seed source is Datang Seed Industry Co., Ltd., China; the seed number is 20190045. The evenly sized and plump wheat seeds were soaked in 10% alcohol for 3 min, washed with sterile water five times, repeated once, and then set aside for use. The planting depth is 2 cm, and 20 seeds are planted in each pot. The materials used in the experiments and the culture conditions are shown in Table 2. The inoculum volume at the log phase of each pot was as follows: CK (50 mL inactivated P. sabinae inoculum and 50 mL inactivated Leptolyngbya sp. RBD05 inoculum), JK (50 mL P. sabinae inoculum and 50 mL inactivated Leptolyngbya sp. RBD05 inoculum), CL (50 mL Leptolyngbya sp. RBD05 inoculum and 50 mL inactivated P. sabinae inoculum), JKCL (50 mL P. sabinae inoculum and Leptolyngbya sp. RBD05 inoculum). Inactivated inoculants are added to eliminate errors caused by nutrients introduced into the inoculant solution. The potted plants were placed in a completely random arrangement in a growth chamber (the growth conditions are illustrated in Table2); the pot positions were changed periodically. No fertilizer was added throughout the culture cycle. All pots were watered every 3 days, until they reached field capacity. Field water holding capacity is about 60%. The water content of soil was determined by drying method.
Wheat and soil were collected from five randomly selected pots from each set of 15 parallel samples in each group at 30, 60, and 90 days. The growth status, nitrogen (N), phosphorus (P), potassium (K), sodium (Na), proline content, malondialdehyde (MDA) content, and superoxide dismutase (SOD) activity of wheat were determined. The pH, electrical conductivity (EC), available nitrogen (A-N), available phosphorus (A-P), available potassium (A-K), and available sodium (A-Na) in soil were determined. The determination methods are shown in Table 3.
2.3 Statistical analysis
The result is the mean ± standard error of the five parallel values. One-way analysis of variance (ANOVA) was used to analyze the difference in the same index among different treatments. The same letter (a, b, c, ab, etc.) in the same time group indicated no significant difference using Duncan’s test at p < 0.05. The correlation among indicators of the same treatment was analyzed by the Pearson correlation coefficient. Data analysis and mapping were performed with IBM SPSS Statistics 26 and Origin 2022 software.
3 Results and discussion
3.1 Effects of Paenibacillus sabinae and Leptolyngbya sp. RBD05 on soil chemical properties
3.1.1 Effects on soil A-K and A-Na
Slow-release K compounds could be dissolved by KSB; thereby, the content of soil A-K would increase (Xiao et al., 2017). At 30 days, there was no difference in soil A-K content between the JK and CK groups (p > 0.05, Figure 1A). The soil A-K content of the CL and JKCL groups was lower than that of the CK group by 6% and 14% (p < 0.05, Figure 1A). It may be that both P. sabinae and Leptolyngbya sp. RBD05 prolifically increased, which resulted in the enhanced ability of the JK and JKCL groups to release soil A-K at 60 days. The soil A-K content of the JK and JKCL groups was 157.96 and 169.24 mg kg−1, respectively, which was higher than that of the CK group by 16% and 26% (Figure 1A). These results indicated that the soil A-K content was increased by both the application of P. sabinae and the co-application of P. sabinae and Leptolyngbya sp. RBD05, and the co-application of the two microorganisms had a better effect on the soil A-K content than the single application of P. sabinae. Liu et al. (2012) showed that Paenibacillus sp. had a great K-solubilizing effect, which supported the results of this study. Rossi et al. (2017) reported that some microalgae had a good effect on improving soil nutrients, but most studies focused on their N fixation ability, and no studies have shown that Leptolyngbya can increase the A-K content in soil. Our study found that Leptolyngbya sp. RBD05 also had a certain improvement effect on the A-K content in soil as discussed below. The K content of wheat in the treatment groups was not lower than that in the control group (Figure 3A), while the dry weight of the treated wheat groups was heavier (Figure 2D). Therefore, the K absorption of wheat growth from the soil in the treatment groups was higher than that in the control group. The difference of soil A-K content between the four groups was not significant at 90 days (p > 0.05, Figure 1A). These showed that, at 90 days, P. sabinae and Leptolyngbya sp. RBD05 actually increased the soil A-K content. A comprehensive analysis of all the results on days 30, 60, and 90 showed that the co-application of P. sabinae and Leptolyngbya sp. RBD05 showed a better K dissolution effect than the application of P. sabinae or Leptolyngbya sp. RBD05 alone. Previous studies have also shown that the application of multiple microorganisms has better effects in improving soil A-K content than one microorganism, which supports the results in this study (Muthuraja and Muthukumar, 2022).
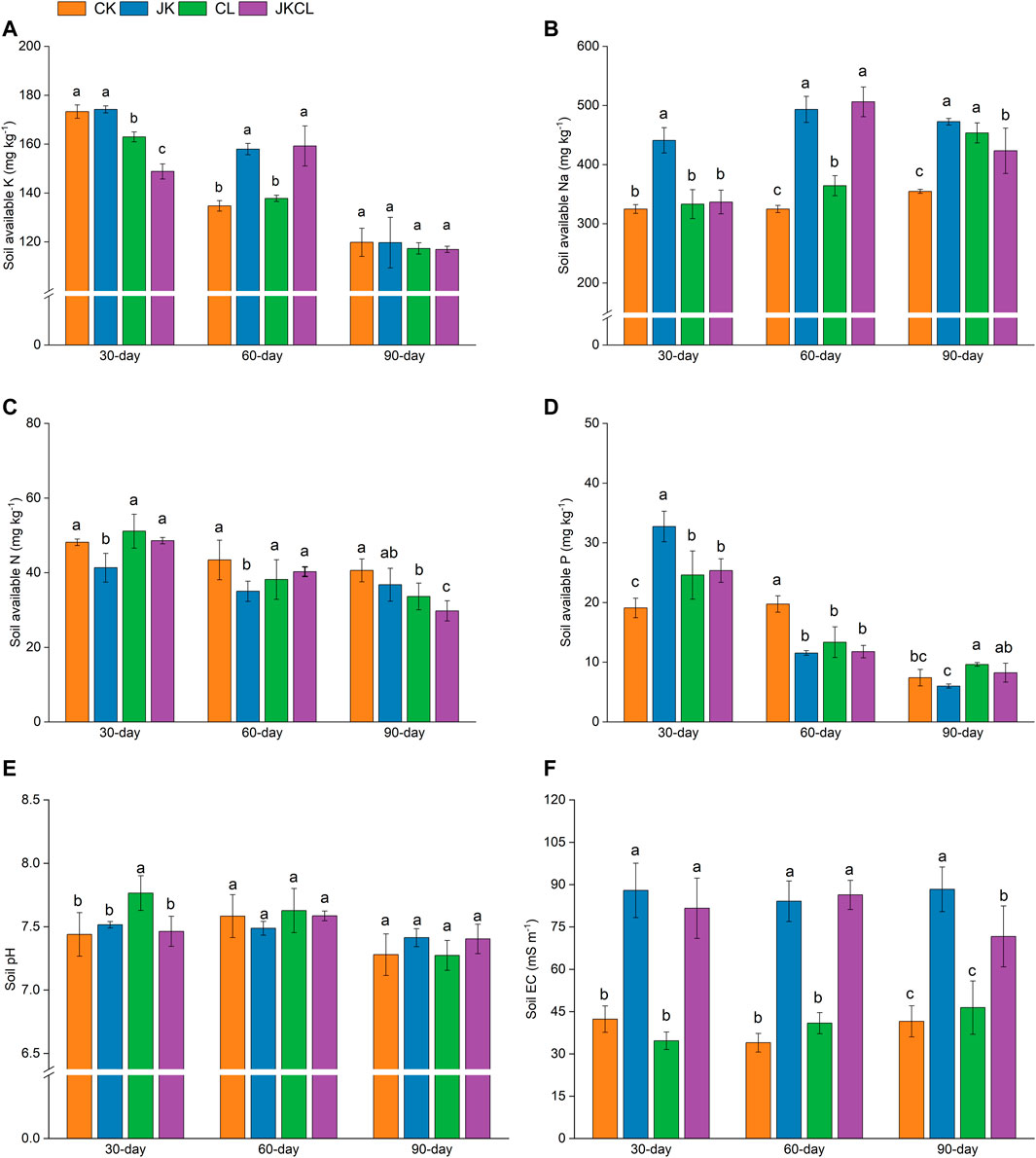
FIGURE 1. Effects of Paenibacillus sabinae and Leptolyngbya sp. RBD05 on soil available K (A), available Na (B), available N (C), available P (D), soil pH (E), and electrical conductivity (F) at different times. CK = the control group, JK = the Paenibacillus sabinae group, CL = the Leptolyngbya sp. RBD05 group, JKCL = the Paenibacillus sabinae + Leptolyngbya sp. RBD05 group.
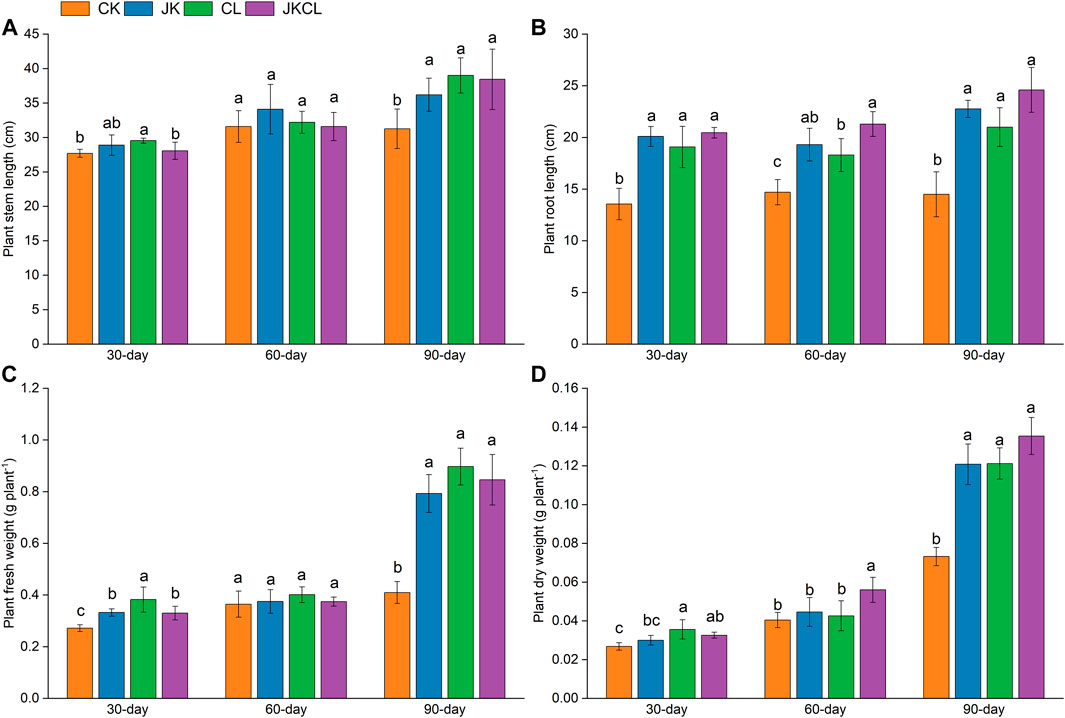
FIGURE 2. Effects of Paenibacillus sabinae and Leptolyngbya sp. RBD05 on wheat stem length (A), root length (B), fresh weight (C), and dry weight (D) at different times. CK = the control group, JK = the Paenibacillus sabinae group, CL = the Leptolyngbya sp. RBD05 group, JKCL = the Paenibacillus sabinae + Leptolyngbya sp. RBD05 group.
Excessive Na adversely affects plant development and osmotic pressure balance in saline-alkali soil (Rengasamy, 2010). This study found that the soil A-Na content increased with the application of P. sabinae. At 30, 60, and 90 days, soil A-Na content in group JK was 440.90, 493.22, and 472.51 mg kg−1, respectively, significantly increased by 36%, 52%, and 33% compared with group CK (p < 0.05, Figure 1B), which aggravated the stress of Na on plant growth. At 30 and 90 days, the soil A-Na content of the JKCL group was lower than that of the JK group by 24% and 15%, respectively, at 60 days, there was no significant difference in soil A-Na content between the JK group and the JKCL group (p < 0.05, Figure 1B). Studies have shown that cyanobacteria can reduce the A-Na content in soil by fixing Na in their cells or producing exopolysaccharides to adsorb Na (Prasanna et al., 2008). Thus, the co-application of P. sabinae and Leptolyngbya sp. RBD05 made up for the defect of applying P. sabinae alone. At 90 days, the A-Na content in the JKCL group soil was lower than that in the CL and JK groups (p < 0.05, Figure 1B). The above results indicated that the co-application of P. sabinae and Leptolyngbya sp. RBD05 was more conducive to reducing salt stress than a single application. The reason was that when P. sabinae and Leptolyngbya sp. RBD05 were collaboratively applied, the P. sabinae promoted the growth of Leptolyngbya sp. RBD05, and made it showing a better ability to fix Na than itself (Li et al., 2019).
3.1.2 Effects on soil A-N and A-P
Nitrogen-fixing microalgae such as Anabaena doliolum HH-209, Cylindrospermum sphaerica, and Calothrix elenkinii and their combinations with other bacteria, all showed good nitrogen-fixing effects in soil (Renuka et al., 2018). Similar to the K consumption by wheat, the N and P consumption by wheat in the soil was also high. However, the A-N content of the CL and JKCL groups did not decrease significantly at 30 and 60 days (p > 0.05, Figure 1C), indicating that the application of Leptolyngbya sp. RBD05 increased the A-N content in the soil at 30 and 60 days. The growth of wheat accelerated the consumption of soil A-N content. Therefore, at 90 days, the A-N content in soil of the CL and JKCL groups were lower than that of the CK group by 17% and 27%, respectively (p < 0.05, Figure 1C). At 30, 60, and 90 days, the A-N content in soil of the JK group was lower than that of the CK group by 14%, 19%, and 16%, respectively (p < 0.05, Figure 1C). This might be because the wheat in the JK group grew better and consumed more soil A-N than that in the CK group, which was boosted by P. sabinae. Phosphorus (P) soluble microorganisms can contribute to P release in several ways. Studies have found that some KSB also have a P-dissolving ability (Abou-el-Seoud and Abdel-Megeed, 2012). Our study found that while promoting the release of K in the soil, P. sabinae also showed the ability to increase the content of A-P in the soil. At 30 days, the application of P. sabinae alone was more beneficial in increasing the A-P content in the soil than the other treatment groups, which was 71% higher than that of the CK group (p < 0.05, Figure 1D). Although the application of P. sabinae and Leptolyngbya sp. RBD05 increased the content of A-P in the soil to a certain extent, the amount required for wheat growth was also high. Therefore, soil A-P content in the three treatment groups decreased at 60 days, and the difference value between the four groups was small at 90 days (Figure 1D). The above results indicated that the co-application of P. sabinae and Leptolyngbya sp. RBD05 or the application of P. sabinae alone could improve the soil A-P content in the early stage (30 days), and the application of P. sabinae alone increased the soil A-P content most obviously, but both could not maintain a high level of soil phosphorus content in the middle and later stage (60 and 90 days).
3.1.3 Effects on soil pH and EC
There was little change in soil pH in each group (p > 0.05, Figure 1E). This indicated that neither P. sabinae nor Leptolyngbya sp. RBD05 could significantly affect soil pH.
Soil EC value is an important indicator for monitoring soil health. When the EC value is too high, the soil structure and soil respiration will be adversely affected (Yan and Marschner, 2013). Soil EC will increase to a certain extent if the soil nutrient content increases (Maltas et al., 2022). According to Section 3.1.1 and Section 3.1.2, the application of P. sabinae and Leptolyngbya sp. RBD05 increased the soil nutrient element content, which increased the EC value in the soil. At 30, 60, and 90 days, the soil EC value of the JK group was higher than that of the CK group by 108%, 148%, and 113%, respectively. The soil EC value of the JKCL group was higher than that of the CK group by 93%, 148%, and 73%, respectively. The soil EC values of the CL and CK group showed little difference (Figure 1F). Although the soil EC in the JKCL group was still higher than that in the CK group at 90 days, it was 23% lower than that at 30 and 60 days, respectively (p < 0.05, Figure 1F). However, at 90 days, the EC values of the JK and CL group were higher than those at 30 and 60 days. These results indicated that the EC was reduced by the co-application of P. sabinae and Leptolyngbya sp. RBD05 over time.
3.2 Effects of Paenibacillus sabinae and Leptolyngbya sp. RBD05 on the growth and stress resistance of wheat
3.2.1 Effects on wheat growth
The analysis in Section 3.1 showed that, compared with the CK group, the application of P. sabinae and Leptolyngbya sp. RBD05 could significantly improve the soil environment, which was more conducive to wheat growth. There was little difference in the stem length of wheat in all groups at 30 and 60 days. However, at 90 days, the stem length of wheat in the three treatment groups showed obvious advantages (Figure 2A), indicating that the application of P. sabinae and Leptolyngbya sp. RBD05 promoted the growth of wheat at the later stage. Majeed et al. (2015) reported that plant development could be improved by the addition of beneficial microorganisms. P. sabinae and Leptolyngbya sp. RBD05 might secrete plant hormones such as kinetin and auxin to promote wheat growth (Ahmeda et al., 2014; Chen et al., 2020).
The belowground parts of plants are more sensitive to salt than the aboveground parts (Munns and Tester, 2008), and the root development of wheat is obviously affected by salinity (Robin et al., 2016). Compared with the stem length, the promoting effect of each treatment group on the root length of wheat was more significant. At 30, 60 and 90 days, the root length of wheat in each treatment group was higher than that in the CK group. The root growth of the JKCL group was the most obvious, and the root length of JKCL group was the longest at 90 days, which was 70% higher than that of CK group (Figure 2B). This suggested that the co-application of P. sabinae and Leptolyngbya sp. RBD05 best promoted the root development of wheat, which helped wheat resist saline-alkali stress (Robin et al., 2016). AminiHajiabadi et al. (2021) found that Bacillus sp. could promote the wheat root development, which further confirmed the results of this study.
Cyanobacteria usually play an important role in preventing soil erosion and water evaporation, which helps plants to absorb water (Acea et al., 2003). At 30 and 60 days, there was little difference in fresh weight among all groups. At 90 days, the wheat fresh weight in the three treatment groups were all higher than that in the CK group, and the fresh weight in the CL group was the highest, which was 119% higher than that in the CK group (Figure 2C), indicating that the application of Leptolyngbya sp. RBD05 alone was more beneficial to wheat water retention. At 30 days, all groups had little difference in dry weight. At 60 days, the dry weight of wheat in the JKCL group was 39% higher than that in the CK group, and there was no significant difference in the dry weight of wheat in other groups except the JKCL group. At 90 days, the dry weight of wheat in the three treatment groups was all higher than that in the CK group. The co-application of P. sabinae and Leptolyngbya sp. RBD05 had the greatest promoting effect on the dry matter accumulation of wheat, which made the wheat dry weight in the JKCL group the highest, 85% higher than that in the CK group (Figure 2D). In the early stage, the nutrient element demand of wheat was lower, but with the passage of time, the nutrient element demand of wheat increased, and the side effects of stress on wheat were also accumulating. Under the promotion of bacteria and cyanobacteria, the wheat growth status of the treatment group and the control group gradually opened a gap; therefore, at 90 days, the fresh weight and dry weight of wheat between the treatment group and the control group were significantly different. Among them, the co-application of P. sabinae and Leptolyngbya sp. RBD05 showed the best effect on promoting wheat dry matter accumulation. The reason might be that the bioactivity of bacteria and cyanobacteria could be significantly improved by their cooperation, which was more conducive to promoting plant growth (Prasanna et al., 2012).
3.2.3 Effects on wheat K, Na, and K:Na ratio
K can maintain the homeostasis of osmotic pressure in plants, improve enzyme activity, and reduce damage to plant cells (Assaha et al., 2017). KSB promotes plant growth by dissolving slow-release potassium compounds in soil and secreting auxin while simultaneously promoting plant K absorption (Lin et al., 2002). As shown in Figure 3A, at 30 days, there was little difference between the JK and CK groups in wheat K content. At 60 and 90 days, the wheat K content in the JK group was higher than that in the CK group by 15% and 20%, respectively. The results indicated that although the application of P. sabinae alone had no significant effect on increasing the wheat K content at the early stage (30 days), its effect on increasing the wheat K content was significantly enhanced as time extended. The reason might be that the proliferation of P. sabinae increased wheat’s ability to absorb K from the soil. At 30 and 60 days, the wheat K content in the CL group was significantly higher than in the CK group by 21% and 12%, respectively. At 90 days, there was little difference between the CL and CK groups in wheat K content. These results indicated that with time passing, the promotion effect of Leptolyngbya sp. RBD05 alone on wheat K content had a downward trend. The reason might be that Leptolyngbya sp. RBD05 is mainly grown on the surface of the soil, and soil salt accumulates on soil surface by transpiration (Tejada et al., 2006), worsening the growth environment of Leptolyngbya sp. RBD05 and reducing its activity, which in turn reduced its promoting effect on the K content in wheat. Rana et al. (2012) reported that the co-application of bacteria and cyanobacteria could fully enhance and play their respective advantages. This study found that at 30, 60, and 90 days, the wheat K content in the JKCL group was higher than in the CK group by 27%, 17%, and 18%, respectively. These results indicated that the K content of wheat could be significantly increased at 30, 60, and 90 days by the combination of P. sabinae and Leptolyngbya sp. RBD05 would effectively maintain the balance of osmotic pressure in wheat (Etesami et al., 2017). Rana et al. (2012) found that the K absorption by wheat was significantly increased by the co-use of rhizosphere growth-promoting bacteria and cyanobacteria, which supported the above results of this study. K deficiency would lead to slow plant growth (Gupta et al., 2015). Therefore, wheat growth in the CK group was worse than in all treatment groups (Figure 2).
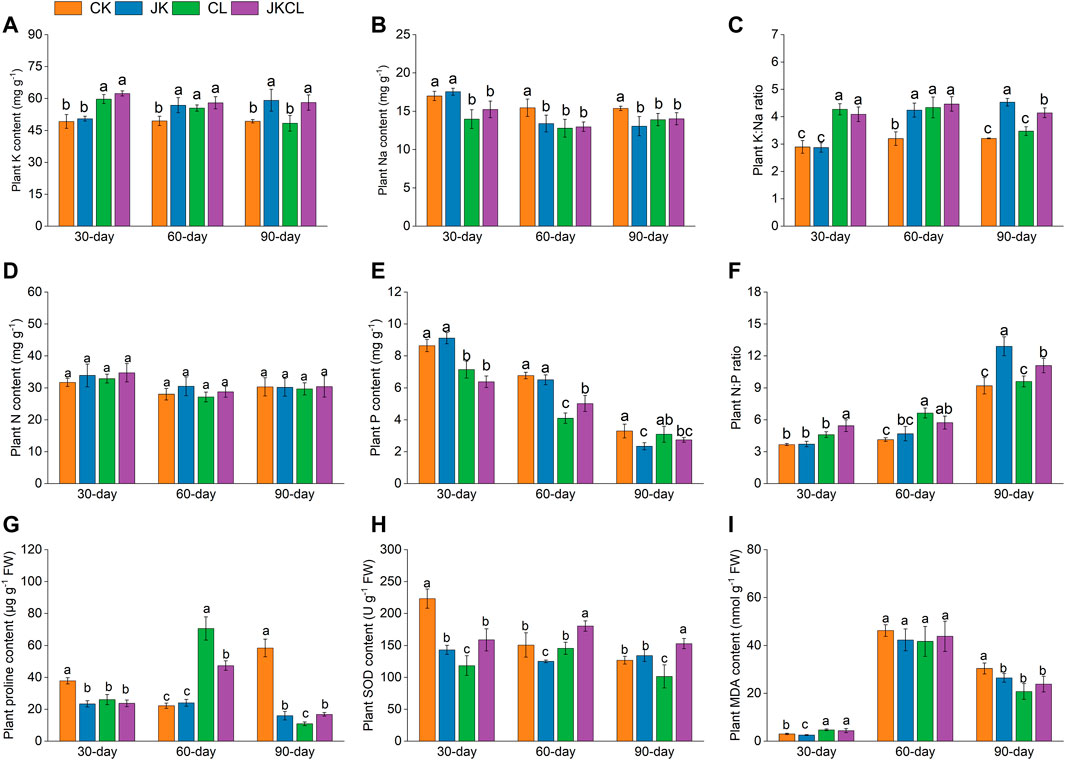
FIGURE 3. Effects of Paenibacillus sabinae and Leptolyngbya sp. RBD05 on wheat K (A), Na (B), (K) Na ratio (C), nitrogen (D), phosphorus (E), N:P ratio (F), wheat proline (G), SOD (H), and MDA (I) at different times. CK = the control group, JK = the Paenibacillus sabinae group, CL = the Leptolyngbya sp. RBD05 group, JKCL = the Paenibacillus sabinae + Leptolyngbya sp. RBD05 group. FW = fresh weight.
Excessive Na in plants could destroy the osmotic pressure balance, leading to physiological drought, and even damaging plant cells (Fang et al., 2021). Grieve and Poss (2000) discovered an inverse relationship between Na and K in plants, and that the ability of plants to resist salt stress is partly due to the continuous uptake of K by roots, which in turn limits the uptake of Na. The study found that inoculation of exopolysaccharide-producing bacteria under salt stress could limit the absorption of Na by wheat seedlings (Ashraf et al., 2004). Our study revealed that, except there was no significant difference in wheat Na content between the JK and CK groups at 30 days, the wheat Na content in all treatment groups at 30, 60 and 90 days was all lower than that in the CK group, decreasing by 13%–18% (Figure 3B). The results indicated that the Na content of wheat was reduced and the application of P. sabinae and Leptolyngbya sp. RBD05 alleviated salt stress. The difference was that Leptolyngbya sp. RBD05 had more obvious effect at 30 days and 60 days. The reason might be that the soil A-Na of the CL group can be adsorbed by exopolysaccharides secreted by cyanobacteria, thus reducing the absorption of Na by plants (Li et al., 2019).
When the regulation of osmotic pressure and the stability of metabolic function in plants are subjected to a high salt stress level, the plant K:Na ratio will change. A higher plant K:Na ratio can generally ensure that the various physiological functions of plant cells are in a good state, maintaining osmotic balance in plants and protecting enzyme activities (Falhof et al., 2016). Previous studies have reported that both cyanobacteria or KSB could promote the K content of plants and thus increase the K:Na ratio in plants (Rana et al., 2012; Li et al., 2019). In this study, the wheat K:Na ratio in the CL and JK groups was not higher than that in the group CK at 30, 60, and 90 days, but the wheat K:Na ratio in the JKCL group was all higher than that in the CK group by 29%–41% (Figure 3C). Maintaining high K:Na ratio levels could help plants tolerate salt stress (Maathuis and Amtmann, 1999). The results of wheat K:Na ratio further showed that the co-application of P. sabinae and Leptolyngbya sp. RBD05 was more effective in increasing the wheat K:Na ratio, maintaining the balance of osmotic pressure in wheat, and alleviating salt stress.
Correlation analysis was conducted to analyze the mutual effects among K, Na content and K:Na ratio in wheat. As shown in Table 4, the correlation between the K content and Na content of wheat and the K:Na ratio among the four groups had significant changes. There was a significant positive correlation between the K content and K:Na ratio of wheat in the CK and CL groups (p < 0.01). These results indicated that the wheat K:Na ratio in group CK and CL was mainly affected by the wheat K content. The wheat K:Na ratio in the JK group was significantly positively correlated with the wheat K content (p < 0.05) and negatively correlated with the wheat Na content (p < 0.01), implying that the wheat K:Na ratio in the JK group was affected by the increase of wheat K content and the decrease of wheat Na content. There was no significant correlation between the K and Na contents of wheat and the K:Na ratio in the JKCL group (p < 0.05). In addition to regulating the contents of K and Na, plants can also enhance their osmotic potential and regulate the balance of osmotic pressure by accumulating small molecules such as soluble sugars (Kerepesi and Galiba, 2000). The above analysis indicated that the wheat in JKCL group may affect the wheat K: Na through this mechanism.
3.2.3 Effects on wheat N, P, and N:P ratio
N and P are the key factors affecting the growth of plants, and their effects on plant growth are interdependent. An appropriate N:P ratio is more conducive to plant growth and the N:P ratio can be used to indicate whether plant growth is affected or limited by N or P (Güsewell, 2004). Studies have shown that when the N: P ratio in a plant is lower than 14, the plant growth will be limited by N, and when the N: P ratio is higher than 16, the plant growth is limited by P (Güsewell, 2004; Cernusak et al., 2010). In this study, the highest N:P ratio of wheat was 12.91 in the JK group at 90 days (Figure 3F), which was lower than 14, indicating that the N content in saline-alkali soil was limited and the wheat growth was restricted by N.
At 30, 60, and 90 days, there was no difference in the N content of wheat among the four groups (p > 0.05, Figure 3D). The differences in the wheat P content among the groups were obvious, and the overall trend of decline was observed with an increase in time. In addition, the wheat P contents in all treatment groups were mostly lower than in the CK group (Figure 3E). This implied that the wheat N:P ratio regulation was mainly due to the decrease in wheat P content under the effects of P. sabinae and Leptolyngbya sp. RBD05. Pandey et al. (2022) pointed out that plant-related microorganisms could regulate the biosynthesis of plant secondary metabolite, thus promoting the ability of plants to cope with environmental stress. The wheat N:P ratio in all groups in this study showed an overall increasing trend and the application of P. sabinae and Leptolyngbya sp. RBD05 made the wheat N:P ratio in all treatment groups higher than that in the CK group. At 90 days, the wheat N:P ratio in the JK group was the highest, which was higher than in the CK group by 40% (Figure 3F). These results indicated that the application of P. sabinae and Leptolyngbya sp. RBD05 both had significant effect on the wheat N: P ratio, and an increase in N: P ratio would then reduce the adverse effect of low N content on wheat growth in the poor saline soil environment (Güsewell, 2004).
The relationship between N and P is always considered to be allometric. When environmental conditions change, plants will store excess nutrients, which may lead to a reduced correlation between changes in nitrogen and phosphorus content in plants (He et al., 2008). As shown in Table 4, the wheat P content in the CK, JK, and CL groups was significantly negatively correlated with the wheat N: P ratio (p < 0.01, Table 4), and there was no significant correlation between the wheat N, P content, and wheat N:P ratio in the JKCL group. These results showed that, except in the JKCL group, the wheat N:P ratio in other groups was mainly affected by the change of wheat P content. The effect of the wheat N or P content on wheat N:P ratio was weakened under the joint action of P. sabinae and Leptolyngbya sp. RBD05.
3.2.4 Effects on wheat proline, SOD, and MDA
Proline is an important amino acid that plays an important role in plant response to abiotic stress. Overproduction of proline in plant cells helps to maintain cell homeostasis, water absorption, osmotic regulation, and REDOX balance to restore cell structure and reduce oxidative damage. In order to cope with saline-alkali stress, plants usually accumulate small organic compounds such as proline to stabilize their osmotic potential (Ahanger et al., 2019; Ghosh et al., 2022). The stressed environment leads to an increase in the content of reactive oxygen species (ROS) content in plants, which can damage the structure of plant cells. SOD activity will increase rapidly to remove ROS, protect the healthy development of plant cells, and reduce the damage to plant cells caused by stressed conditions (Baltruschat et al., 2008; Liu et al., 2018). In addition, when plant cells undergo lipid peroxidation, MDA is usually produced, which is a cytotoxic product and is usually used as an indicator of the degree of plant stress (Liu et al., 2017).
This study found that each treatment group reduced the saline-alkali stress on wheat by promoting K absorption and reducing Na absorption at 30 days (Figure 3), which made the response of wheat in each treatment group to stress weaker than that in the CK group. Therefore, at 30 days, the proline content and SOD activity of the treatment groups were all lower than that of the CK group (Figures 3G, H). At the same time, the MDA content of wheat in all groups was also low, which indicated that the stress had little effect on wheat at this time.
At 60 days, the MDA content in each group increased sharply (Figure 3I), indicating that the stress had intensified (Liu et al., 2017). The proline content in the CL and JKCL groups was significantly higher than that in the other two groups, which were 70.62 and 47.38 μg g−1 FW (fresh weight), and was 218% and 113% higher than that in the CK group (p < 0.05, Figure 3G). Hemavathi et al. (2011) reported that the activity of antioxidant enzymes in plants could be increased to a certain extent under the influence of increasing K content. As shown in Figure 3A, the wheat K content in the JKCL group was significantly higher than that in the CK group. A higher wheat K content can improve SOD activity. As a result, the activity of wheat SOD in the JKCL group was higher than that in other groups (Figure 3G). These results indicated that the application of Leptolyngbya sp. RBD05 alone or the co-application of P. sabinae and Leptolyngbya sp. RBD05 had a greater ability to promote proline synthesis and increase the SOD activity in the face of saline-alkali stress.
At 90 days, the MDA content of wheat in all groups decreased, and the difference among treatment groups was not significantly and lower than that observed in the CK group (Figure 3I). However, the proline content of the CK group was significantly higher than that of the other groups (Figure 3G). The accumulation of proline plays a good role in stabilizing the osmotic potential of plants and is also an indicator of the degree of salt stress (Singh et al., 2000). This indicated that the wheat in the CK group was still under high stress at this time, and the increase of proline content mainly regulated the wheat osmotic balance. But the other treatment groups regulated the wheat osmotic balance by increasing the K content and reducing the Na content, as shown in Section 3.2.2.
4 Conclusion
The study showed that the potassium-solubilizing bacterium P. sabinae and the cyanobacterium Leptolyngbya sp. RBD05 produced in brewery wastewater had the ability to enhance the nutrient level of saline-alkali soil, and promote the stress tolerance and growth of wheat. Among all the treatment groups, the co-application of P. sabinae and Leptolyngbya sp. RBD05 was more conducive to the increase of the K content in saline-alkali soil, the development of wheat roots, and the improvement of K:Na ratio, proline content and SOD activity of wheat. The application of Leptolyngbya sp. RBD05 alone had a more prominent effects on water retention of the wheat plant. The application of P. sabinae alone increased soil available P and wheat N:P ratio more obviously. The results of this study provided new ideas for the use of microorganisms to improve crop growth in saline-alkali land and the further utilization of bacteria and cyanobacteria produced in wastewater treatment.
Data availability statement
The original contributions presented in the study are included in the article/supplementary material, further inquiries can be directed to the corresponding author.
Author contributions
HuD: Conceptualization, Formal analysis, Writing—original draft. WL: Data curation, Funding acquisition, Supervision, Writing—review and editing. LZ: Data curation, Methodology. BH and SH: Data curation, Investigation. ME-S and ME: Data curation, Writing—review and editing. HaD and TX: Investigation. XL: Data curation. All authors listed have made a substantial, direct, and intellectual contribution to the work and approved it for publication. All authors contributed to the article and approved the submitted version.
Funding
This work was supported in part by Natural Science Foundation of Shandong Province (ZR2022MC204); Innovation Ability Improvement Project of Small and Medium-sized High-tech Company in Shandong Province (2022TSGC2199); Major Science and Technology Innovation Projects in Shandong Province (2019JZZY010723). Innovation Ability Improvement Project of Small and Medium-sized High-tech Company in Zaozhuang City (2023TSGC15).
Conflict of interest
The authors declare that the research was conducted in the absence of any commercial or financial relationships that could be construed as a potential conflict of interest.
Publisher’s note
All claims expressed in this article are solely those of the authors and do not necessarily represent those of their affiliated organizations, or those of the publisher, the editors and the reviewers. Any product that may be evaluated in this article, or claim that may be made by its manufacturer, is not guaranteed or endorsed by the publisher.
References
Abou-el-Seoud, I. I., and Abdel-Megeed, A. (2012). Impact of rock materials and biofertilizations on P and K availability for maize (Zea Maize) under calcareous soil conditions. Saudi J. Biol. Sci. 19, 55–63. doi:10.1016/j.sjbs.2011.09.001
Acea, M. J., Prieto-Fernández, A., and Diz-Cid, N. (2003). Cyanobacterial inoculation of heated soils: Effect on microorganisms of C and N cycles and on chemical composition in soil surface. Soil Biol. Biochem. 35, 513–524. doi:10.1016/S0038-0717(03)00005-1
Aditya, L., Mahlia, T. M. I., Nguyen, L. N., Vu, H. P., and Nghiem, L. D. (2022). Microalgae-bacteria consortium for wastewater treatment and biomass production. Sci. Total Environ. 838, 155871. doi:10.1016/j.scitotenv.2022.155871
Ahanger, M. A., Aziz, U., Alsahli, A. A., Alyemeni, M. N., and Ahmad, P. (2019). Influence of exogenous salicylic acid and nitric oxide on growth, photosynthesis, and ascorbate-glutathione cycle in salt stressed Vigna angularis. Biomolecules 10, 42. doi:10.3390/biom10010042
Ahmeda, M., Stal, L. J., and Hasnain, S. (2014). The morphology and bioactivity of the rice field cyanobacterium Leptolyngbya. Rev. Biol. Trop. 62, 1251–1260. doi:10.15517/rbt.v62i3.8657
AminiHajiabadi, A., MoslehArani, A., Ghasemi, S., Rad, M. H., Etesami, H., ShabaziManshadi, S., et al. (2021). Mining the rhizosphere of halophytic rangeland plants for halotolerant bacteria to improve growth and yield of salinity-stressed wheat. Plant Physiol. Bioch 163, 139–153. doi:10.1016/j.plaphy.2021.03.059
Ashfaq, M., Hassan, H. M., Ghazali, A. H. A., and Ahmad, M. (2020). Halotolerant potassium solubilizing plant growth promoting rhizobacteria may improve potassium availability under saline conditions. Environ. Monit. Assess. 192, 697. doi:10.1007/s10661-020-08655-x
Ashraf, A., Ramamurthy, R., and Rene, E. R. (2021). Wastewater treatment and resource recovery technologies in the brewery industry: Current trends and emerging practices. Sustain Energy Techn 47, 101432. doi:10.1016/j.seta.2021.101432
Ashraf, M., Hasnain, S., Berge, O., and Mahmood, T. (2004). Inoculating wheat seedlings with exopolysaccharide-producing bacteria restricts sodium uptake and stimulates plant growth under salt stress. Biol. Fertil. Soils 40, 157–162. doi:10.1007/s00374-004-0766-y
Assaha, D. V. M., Ueda, A., Saneoka, H., Al-Yahyai, R., and Yaish, M. W. (2017). The role of Na+ and K+ transporters in salt stress adaptation in glycophytes. Front. Physiol. 8, 509. doi:10.3389/fphys.2017.00509
Aycan, M., Baslam, M., Asiloglu, R., Mitsui, T., and Yildiz, M. (2021). Development of new high-salt tolerant bread wheat (Triticum aestivum L) genotypes and insight into the tolerance mechanisms. Plant Physiol. Bioch 166, 314–327. doi:10.1016/j.plaphy.2021.05.041
Baltruschat, H., Fodor, J., Harrach, B. D., Niemczyk, E., Barna, B., Gullner, G., et al. (2008). Salt tolerance of barley induced by the root endophyte Piriformospora indica is associated with a strong increase in antioxidants. New Phytol. 180, 501–510. doi:10.1111/j.1469-8137.2008.02583.x
Bates, L. S., Waldren, R. P., and Teare, I. D. (1973). Rapid determination of free proline for water-stress studies. Plant Soil 39, 205–207. doi:10.1007/BF00018060
Bidyarani, N., Prasanna, R., Babu, S., Hossain, F., and Saxena, A. K. (2016). Enhancement of plant growth and yields in Chickpea (Cicer arietinum L) through novel cyanobacterial and biofilmed inoculants. Microbiol. Res. 188–189, 97–105. doi:10.1016/j.micres.2016.04.005
Cernusak, L. A., Winter, K., and Turner, B. L. (2010). Leaf nitrogen to phosphorus ratios of tropical trees: Experimental assessment of physiological and environmental controls. New Phytol. 185, 770–779. doi:10.1111/j.1469-8137.2009.03106.x
Chen, Y. H., Yang, X. Z., Li, Z., An, X. H., Ma, R. P., Li, Y. Q., et al. (2020). Efficiency of potassium-solubilizing Paenibacillus mucilaginosus for the growth of apple seedling. J. Integr. Agr. 19, 2458–2469. doi:10.1016/S2095-3119(20)63303-2
Etesami, H., Emami, S., and Alikhani, H. A. (2017). Potassium solubilizing bacteria (KSB): Mechanisms, promotion of plant growth, and future prospects - a review. J. Soil Sci. Plant Nut 17, 897–911. doi:10.4067/S0718-95162017000400005
Faisal Alharby, H. (2021). Using some growth stimuli, a comparative study of salt tolerance in two tomatoes cultivars and a related wild line with special reference to superoxide dismutases and related micronutrients. Saudi J. Biol. Sci. 28, 6133–6144. doi:10.1016/j.sjbs.2021.06.062
Falhof, J., Pedersen, J. T., Fuglsang, A. T., and Palmgren, M. (2016). Plasma Membrane H+ -ATPase regulation in the center of plant physiology. Mol. Plant 9, 323–337. doi:10.1016/j.molp.2015.11.002
Fang, S., Hou, X., and Liang, X. (2021). Response mechanisms of plants under saline-alkali stress. Front. Plant Sci. 12, 667458. doi:10.3389/fpls.2021.667458
Feng, K., Cai, Z., Ding, T., Yan, H., Liu, X., and Zhang, Z. (2019). Effects of potassium-solubulizing and photosynthetic bacteria on tolerance to salt stress in maize. J. Appl. Microbiol. 126, 1530–1540. doi:10.1111/jam.14220
Ferreira, A., Ribeiro, B., Ferreira, A. F., Tavares, M. L. A., Vladic, J., Vidović, S., et al. (2019). Scenedesmus obliquus microalga-based biorefinery – from brewery effluent to bioactive compounds, biofuels and biofertilizers – aiming at a circular bioeconomy. Biofuel, Bioprod. Bior 13, 1169–1186. doi:10.1002/bbb.2032
Ghosh, U. K., Islam, M. N., Siddiqui, M. N., Cao, X., and Khan, M. A. R. (2022). Proline, a multifaceted signalling molecule in plant responses to abiotic stress: Understanding the physiological mechanisms. Plant Biol. 24 (2), 227–239. doi:10.1111/plb.13363
Grieve, C. M., and Poss, J. A. (2000). Wheat response to interactive effects of boron and salinity. J. Plant Nutr. 23, 1217–1226. doi:10.1080/01904160009382095
Gupta, G., Parihar, S. S., Ahirwar, N. K., Snehi, S. K., and Singh, V. (2015). Plant growth promoting rhizobacteria (PGPR): Current and future prospects for development of sustainable agriculture. J. Microb. Biochem. Technol. 7, 96–102.
Güsewell, S. (2004). N:P ratios in terrestrial plants: Variation and functional significance. New Phytol. 164, 243–266. doi:10.1111/j.1469-8137.2004.01192.x
He, J.-S., Wang, L., Flynn, D. F. B., Wang, X., Ma, W., and Fang, J. (2008). Leaf nitrogen: Phosphorus stoichiometry across Chinese grassland biomes. Oecologia 155, 301–310. doi:10.1007/s00442-007-0912-y
Hemavathi, U., C. P., Akula, N., Kim, H. S., Jeon, J. H., Ho, O. M., Chun, S. C., et al. (2011). Biochemical analysis of enhanced tolerance in transgenic potato plants overexpressing d-galacturonic acid reductase gene in response to various abiotic stresses. Mol. Breed. 28, 105–115. doi:10.1007/s11032-010-9465-6
Karthikeyan, N., Prasanna, R., Nain, L., and Kaushik, B. D. (2007). Evaluating the potential of plant growth promoting cyanobacteria as inoculants for wheat. Eur. J. Soil Biol. 43, 23–30. doi:10.1016/j.ejsobi.2006.11.001
Kerepesi, I., and Galiba, G. (2000). Osmotic and salt stress-induced alteration in soluble carbohydrate content in wheat seedlings. Crop Sci. 40, 482–487. doi:10.2135/cropsci2000.402482x
Kim, B.-H., Kang, Z., Ramanan, R., Choi, J.-E., Cho, D.-H., Oh, H.-M., et al. (2014). Nutrient removal and biofuel production in high rate algal pond using real municipal wastewater. J. Microbiol. Biotechnol. 24, 1123–1132. doi:10.4014/jmb.1312.12057
Kohler, J., Caravaca, F., Carrasco, L., and Roldán, A. (2007). Interactions between a plant growth-promoting rhizobacterium, an AM fungus and a phosphate-solubilising fungus in the rhizosphere of Lactuca sativa. Appl. Soil Ecol. 35, 480–487. doi:10.1016/j.apsoil.2006.10.006
Kumar, P., and Sharma, P. K. (2020). Soil salinity and food security in India. Front. Sustain Food Syst. 4. doi:10.3389/fsufs.2020.533781
Lee, J., Cho, D.-H., Ramanan, R., Kim, B.-H., Oh, H.-M., and Kim, H.-S. (2013). Microalgae-associated bacteria play a key role in the flocculation of Chlorella vulgaris. Bioresour. Technol. 131, 195–201. doi:10.1016/j.biortech.2012.11.130
Li, H., Zhao, Q., and Huang, H. (2019). Current states and challenges of salt-affected soil remediation by cyanobacteria. Sci. Total Environ. 669, 258–272. doi:10.1016/j.scitotenv.2019.03.104
Lin, Q. M., Rao, Z. H., Sun, Y. X., Yao, J., and Xing, L. J. (2002). Identification and practical application of silicate-dissolving bacteria. Agric. Sci. China 1, 81–85.
Liu, D. F., Lian, B., and Dong, H. L. (2012). Isolation of Paenibacillus sp and assessment of its potential for enhancing mineral weathering. Geomicrobiol. J. 29, 413–421. doi:10.1080/01490451.2011.576602
Liu, D., Liu, H., Wang, S., Chen, J., and Xia, Y. (2018). The toxicity of ionic liquid 1-decylpyridinium bromide to the algae Scenedesmus obliquus: Growth inhibition, phototoxicity, and oxidative stress. Sci. Total Environ. 622–623, 1572–1580. doi:10.1016/j.scitotenv.2017.10.021
Liu, H., Xia, Y., Cai, W., Zhang, Y., Zhang, X., and Du, S. (2017). Enantioselective oxidative stress and oxidative damage caused by Rac- and S-metolachlor to Scenedesmus obliquus. Chemosphere 173, 22–30. doi:10.1016/j.chemosphere.2017.01.028
Lu, R. (1999). Agricultural chemistry analysis of soil. Beijing: China Agricultural Science and Technology Press.
Maathuis, F. J. M., and Amtmann, A. (1999). K+ nutrition and Na+ toxicity: The basis of cellular K+/Na+ ratios. Ann. Bot. 84, 123–133. doi:10.1006/anbo.1999.0912
Mahanty, T., Bhattacharjee, S., Goswami, M., Bhattacharyya, P., Das, B., Ghosh, A., et al. (2017). Biofertilizers: A potential approach for sustainable agriculture development. Environ. Sci. Pollut. R. 24, 3315–3335. doi:10.1007/s11356-016-8104-0
Majeed, A., Abbasi, M. K., Hameed, S., Imran, A., and Rahim, N. (2015). Isolation and characterization of plant growth-promoting rhizobacteria from wheat rhizosphere and their effect on plant growth promotion. Front. Microbiol. 6, 198. doi:10.3389/fmicb.2015.00198
Maltas, A. S., Tavali, I. E., Uz, I., and Kaplan, M. (2022). Monitoring the effects of pH and EC regulated drip fertigation on microbial dynamics of calcareous soil in tomato (Solanum lycopersicum L) cultivation under greenhouse conditions in a Mediterranean climate. Sci. Hortic. 306, 111448. doi:10.1016/j.scienta.2022.111448
Munns, R., and Tester, M. (2008). Mechanisms of salinity tolerance. Annu. Rev. Plant Biol. 59, 651–681. doi:10.1146/annurev.arplant.59.032607.092911
Muñoz, R., and Guieysse, B. (2006). Algal–bacterial processes for the treatment of hazardous contaminants: A review. Water Res. 40, 2799–2815. doi:10.1016/j.watres.2006.06.011
Muthuraja, R., and Muthukumar, T. (2022). Co-inoculation of halotolerant potassium solubilizing Bacillus licheniformis and Aspergillus violaceofuscus improves tomato growth and potassium uptake in different soil types under salinity. Chemosphere 294, 133718. doi:10.1016/j.chemosphere.2022.133718
Pandey, S. S., Jain, R., Bhardwaj, P., Thakur, A., Kumari, M., Bhushan, S., et al. (2022). Plant probiotics – endophytes pivotal to plant health. Microbiol. Res. 263, 127148. doi:10.1016/j.micres.2022.127148
Prasanna, R., Jaiswal, P., and Kaushik, B. D. (2008). Cyanobacteria as potential options for environmental sustainability — Promises and challenges. Indian J. Microbiol. 48, 89–94. doi:10.1007/s12088-008-0009-2
Prasanna, R., Joshi, M., Rana, A., Shivay, Y. S., and Nain, L. (2012). Influence of co-inoculation of bacteria-cyanobacteria on crop yield and C–N sequestration in soil under rice crop. World J. Microbiol. Bio Technol. 28, 1223–1235. doi:10.1007/s11274-011-0926-9
Ramadoss, D., Lakkineni, V. K., Bose, P., Ali, S., and Annapurna, K. (2013). Mitigation of salt stress in wheat seedlings by halotolerant bacteria isolated from saline habitats. Springerplus 2, 6. doi:10.1186/2193-1801-2-6
Rana, A., Joshi, M., Prasanna, R., Shivay, Y. S., and Nain, L. (2012). Biofortification of wheat through inoculation of plant growth promoting rhizobacteria and cyanobacteria. Eur. J. Soil Biol. 50, 118–126. doi:10.1016/j.ejsobi.2012.01.005
Rengasamy, P. (2010). Soil processes affecting crop production in salt-affected soils. Funct. Plant Biol. 37, 613–620. doi:10.1071/FP09249
Renuka, N., Guldhe, A., Prasanna, R., Singh, P., and Bux, F. (2018). Microalgae as multi-functional options in modern agriculture: Current trends, prospects and challenges. Biotechnol. Adv. 36, 1255–1273. doi:10.1016/j.biotechadv.2018.04.004
Renuka, N., Prasanna, R., Sood, A., Ahluwalia, A. S., Bansal, R., Babu, S., et al. (2016). Exploring the efficacy of wastewater-grown microalgal biomass as a biofertilizer for wheat. Environ. Sci. Pollut. Res. 23, 6608–6620. doi:10.1007/s11356-015-5884-6
Robin, A. H. K., Matthew, C., Uddin, M. J., and Bayazid, K. N. (2016). Salinity-induced reduction in root surface area and changes in major root and shoot traits at the phytomer level in wheat. J. Exp. Bot. 67, 3719–3729. doi:10.1093/jxb/erw064
Rossi, F., Li, H., Liu, Y., and de Philippis, R. (2017). Cyanobacterial inoculation (cyanobacterisation): Perspectives for the development of a standardized multifunctional technology for soil fertilization and desertification reversal. Earth Sci. Rev. 171, 28–43. doi:10.1016/j.earscirev.2017.05.006
Sanyal, S. K., Rajasheker, G., Kishor, P. B. K., Kumar, S. A., Kumari, P. H., Saritha, K. V., et al. (2020). “Role of protein phosphatases in signaling, potassium transport, and abiotic stress responses,” in Protein phosphatases and stress management in plants: Functional genomic perspective. Editor G. K. Pandey (Cham: Springer International Publishing), 203–232. doi:10.1007/978-3-030-48733-1_11
Simate, G. S., Cluett, J., Iyuke, S. E., Musapatika, E. T., Ndlovu, S., Walubita, L. F., et al. (2011). The treatment of brewery wastewater for reuse: State of the art. Desalination 273, 235–247. doi:10.1016/j.desal.2011.02.035
Singh, J. S., Kumar, A., Rai, A. N., and Singh, D. P. (2016). Cyanobacteria: A precious bio-resource in agriculture, ecosystem, and environmental sustainability. Front. Microbiol. 7, 529. doi:10.3389/fmicb.2016.00529
Singh, S. K., Sharma, H. C., Goswami, A. M., Datta, S. P., and Singh, S. P. (2000). In vitro growth and leaf composition of grapevine cultivars as affected by sodium chloride. Biol. Plant 43, 283–286. doi:10.1023/A:1002720714781
Subashchandrabose, S. R., Ramakrishnan, B., Megharaj, M., Venkateswarlu, K., and Naidu, R. (2013). Mixotrophic cyanobacteria and microalgae as distinctive biological agents for organic pollutant degradation. Environ. Int. 51, 59–72. doi:10.1016/j.envint.2012.10.007
Tang, X., He, L. Y., Tao, X. Q., Dang, Z., Guo, C. L., Lu, G. N., et al. (2010). Construction of an artificial microalgal-bacterial consortium that efficiently degrades crude oil. J. Hazard Mater 181, 1158–1162. doi:10.1016/j.jhazmat.2010.05.033
Tejada, M., Garcia, C., Gonzalez, J. L., and Hernandez, M. T. (2006). Use of organic amendment as a strategy for saline soil remediation: Influence on the physical, chemical and biological properties of soil. Soil Biol. Biochem. 38, 1413–1421. doi:10.1016/j.soilbio.2005.10.017
Wang, Y. S., Tian, S. P., Xu, Y., Qin, G. Z., and Yao, H. (2004). Changes in the activities of pro- and anti-oxidant enzymes in peach fruit inoculated with Cryptococcus laurentii or Penicillium expansum at 0 or 20 °C. Postharvest Biol. Tec. 34, 21–28. doi:10.1016/j.postharvbio.2004.04.003
Wang, Y., Yan, X., Su, M., Li, J., Man, T., Wang, S., et al. (2022). Isolation of potassium solubilizing bacteria in soil and preparation of liquid bacteria fertilizer from food wastewater. Biochem. Eng. J. 181, 108378. doi:10.1016/j.bej.2022.108378
Xiao, Y., Wang, X., Chen, W., and Huang, Q. (2017). Isolation and identification of three potassium-solubilizing bacteria from rape rhizospheric soil and their effects on ryegrass. Geomicrobiol. J. 34, 873–880. doi:10.1080/01490451.2017.1286416
Yan, N., and Marschner, P. (2013). Response of soil respiration and microbial biomass to changing EC in saline soils. Soil Biol. Biochem. 65, 322–328. doi:10.1016/j.soilbio.2013.06.008
Zhang, H., Wu, X., Li, G., and Qin, P. (2011). Interactions between arbuscular mycorrhizal fungi and phosphate-solubilizing fungus (Mortierella sp) and their effects on Kostelelzkya virginica growth and enzyme activities of rhizosphere and bulk soils at different salinities. Biol. Fert. Soils 47, 543. doi:10.1007/s00374-011-0563-3
Keywords: cyanobacteria, potassium-solubilizing bacteria, saline-alkali soil, wastewater, wheat growth promotion
Citation: Duan H, Liu W, Zhou L, Han B, Huo S, El-Sheekh M, Dong H, Li X, Xu T and Elshobary M (2023) Improving saline-alkali soil and promoting wheat growth by co-applying potassium-solubilizing bacteria and cyanobacteria produced from brewery wastewater. Front. Environ. Sci. 11:1170734. doi: 10.3389/fenvs.2023.1170734
Received: 21 February 2023; Accepted: 04 May 2023;
Published: 15 May 2023.
Edited by:
Xiaokai Zhang, Jiangnan University, ChinaReviewed by:
Evrim Elcin, Adnan Menderes University, TürkiyeTong Pang, Chinese Academy of Sciences (CAS), China
Conghui Liu, Chinese Academy of Agricultural Sciences, China
Copyright © 2023 Duan, Liu, Zhou, Han, Huo, El-Sheekh, Dong, Li, Xu and Elshobary. This is an open-access article distributed under the terms of the Creative Commons Attribution License (CC BY). The use, distribution or reproduction in other forums is permitted, provided the original author(s) and the copyright owner(s) are credited and that the original publication in this journal is cited, in accordance with accepted academic practice. No use, distribution or reproduction is permitted which does not comply with these terms.
*Correspondence: Wei Liu, bGl1d2VpY3NAcWx1LmVkdS5jbg==