- Department of Agroenvironmental Chemistry and Plant Nutrition, Faculty of Agrobiology, Food, and Natural Resources, Czech University of Life Sciences Prague, Prague, Czech Republic
Excess potentially toxic elements (PTEs), including arsenic (As), cadmium (Cd), lead (Pb), and zinc (Zn), above permissible limits in the environment, have detrimental effects on trophic levels. Hence, imperative to devise advertent measures to address this situation, especially in the soil ecosystem: the major reservoir of many PTEs. Using aerial plant parts (shoot) to accumulate As, Cd, Pb, and Zn - hyperaccumulators are considered a permanent approach to PTE removal from soils. This communication expatiated the principles that govern the hyperaccumulation of plants growing on As, Cd, Pb, and Zn-contaminated soils. The contribution of soil microbial communities during hyperaccumulation is well-elaborated to support the preference for this remediation approach. The study confirms a flow direction involving PTE uptake–translocation–tolerance–detoxification by hyperaccumulators. Rhizosphere microbes exhibit a direct preference for specific hyperaccumulators, which is associated with root exudations, while the resultant formation of chelates and solubility of PTEs, with soil physicochemical properties, including pH and redox potential, promote uptake. Different compartments of plants possess specialized transporter proteins and gene expressions capable of influx and efflux of PTEs by hyperaccumulators. After PTE uptake, many hyperaccumulators undergo cellular secretion of chelates supported by enzymatic catalysis and high transport systems with the ability to form complexes as tolerance and detoxification mechanisms. The benefits of combining hyperaccumulators with beneficial microbes such as endophytes and other rhizosphere microbes for PTE removal from soils are vital in enhancing plant survival and growth, minimizing metal toxicity, and supplying nutrients. Inoculation of suitable rhizosphere microbes can promote efficient cleaning of PTEs contaminated sites utilizing hyperaccumulator plants.
1 Introduction
Potentially toxic elements (PTEs), not excluding arsenic (As), cadmium (Cd), zinc (Zn), and lead (Pb), exert antagonistic behavior in the soil ecosystem, associated growing plants, and biomagnifications in animals and humans through ingestion (Knabb et al., 2016; Landrigan et al., 2018). Elements such as copper (Cu) and Zn are ubiquitous and exhibit direct physiological and metabolic relevance in plants as micronutrients, while excess accumulation above threshold limits incurs growth dysfunctions and death (Liu et al., 2018). Arsenic, Pb, and Cd have no benefit to plants and humans with possible health disorders, e.g., cardiovascular disease and diabetes upon accumulation (Fuller et al., 2022), as their environmental exposure increases.
Despite the geogenic occurrence of PTEs, anthropogenic interferences, including mining and smelting (Knabb et al., 2016; Boente et al., 2022), the extended application of untreated organic wastes (e.g., sewage sludge and compost) and mineral fertilizers (e.g., phosphate fertilizers) application to arable lands (Bisht and Chauhan, 2020), and coal ash deposition (Shin et al., 2017) often release enormous contents in soils. Industrialization, war, and intensification of agriculture have left a legacy of soil contamination throughout, with 80,000 sites (in Australia), 1,300 in the United States, 3 million in Europe and the western Balkans, and 16% in China’s soils representing the hot spot of global polluted sites (FAO/UN, 2018). Culminating these data reveals the extent of loss of land resources for agricultural sustainability and calls for more effective, efficient, and affordable removal methods. The vicinity of point sources of potentially toxic elements (PTEs), e.g., As, Cd, Pb, and Zn, become contaminated, affecting the quality of the water table via infiltration and the metabolism of soil microfauna/microflora (Cempel and Nikel, 2006; Ji et al., 2021). For example, mining and metallurgy of the 19th century in the Linares district (Jaén, South Spain) accumulated (mg kg−1) As (42), Cd (140), Pb (4,244—35,899), and up to 768 Zn compared to threshold limits of <20, 2, 100, and 200, respectively (Boente et al., 2022). Environmental exposure to PTEs results in hazardous effects as they are non-degradable (Woldetsadik et al., 2017; Landrigan et al., 2018). These raise significant awareness about the cleaning of affected soils as increases in human population parallel metalliferous activities (Figure 1). Considering climate change in recent decades and the consequences of its impacts (Hardy, 2003; National Academy of Sciences, 2020), removing PTEs in soils by plants offer adequate results over conventional technologies. Conventional strategies for cleaning up contaminated lands, e.g., soil washing (Shen et al., 2019), solidification (Matec industries, 2021), landfilling, soil flushing, and washing, capping, and vitrification (Koul and Taak, 2018; Liu et al., 2018; Khan et al., 2021), are often ex-situ and involve high treatment costs. These approaches often result in losing the fertility of soils and biodiversity, e.g., microfauna (Liu et al., 2022).
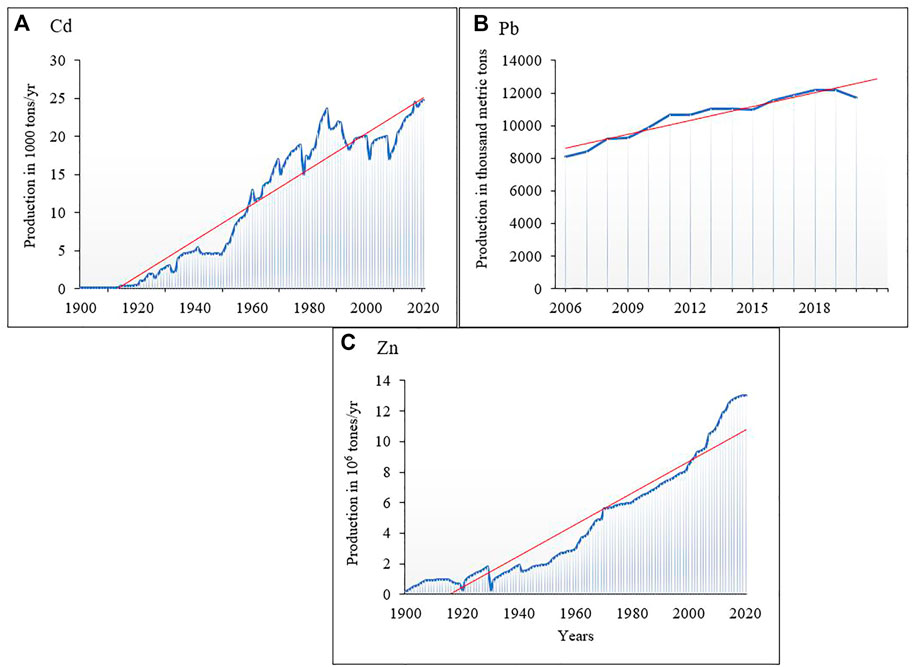
FIGURE 1. World production trend of (A) As, (B) Cd (adopted from Lucía Fernández, 2022, (C) Pb (Statista Research Department), and (D) Zn (U.S. Geological Survey, http://minerals.usgs.gov/ds/2005/140).
Some plants demonstrate Cd, Pb, and Zn accumulation in tissues and organs, especially shoots, providing evidence of their suitability in cleaning contaminated soils (Berhongaray et al., 2015; Yan et al., 2020). Hence, plant-based remediation techniques: phytoextraction, phytostabilization, phytodegradation, and phytovolatilization, most recently, have been adopted based on their success in revegetating PTE-polluted soils at an optimized cost and providing a greener ecosystem. Phytoremediation supports large field reclamations, prevents erosion and leaching by stabilizing metals, enhances organic matter (OM) accumulation, and builds up soil fertility (Jacob et al., 2018; Yan et al., 2020). During the reclamation of contaminated lands, a selection of phytoremediation type depends on the contaminants and the intended purpose of the field.
Some plant species extract and accumulate PTEs in aboveground organs (stem, appendages, leaves, lateral buds, flowering stems, and flower buds), with minimal or no signs of toxicity, termed hyperaccumulators (Suman et al., 2018; Ashraf et al., 2019). In recent times, PTE-polluted soils have been well-used to grow high-biomass woody plants, e.g., Salix and Populus spp allowing the reduction of environmental mobility of PTE and producing polluted harvested woody biomass to produce income (Guerra et al., 2011; Mleczek et al., 2018). Extraction of PTEs in contaminated soils to aboveground organs of plants (phytoextraction) prevents surface soils from contaminating other areas via runoff and erosion (Yan et al., 2020). Many hyperaccumulators grow faster, with high-biomass, fast root-to-shoot translocation, PTE-tolerant, and detoxify PTEs (Balafrej et al., 2020). The possibility of other low biomass and slow-growth plant hyperaccumulators, e.g., Solanum nigrum L., exhibit high PTE accumulation under the assistance of microorganisms (Zhang et al., 2021). Plant species from families including Brassicaceae (e.g., Brassica sp. and Noccaea sp.), Pteridaceae (e.g., Pteris vittate L.), Lamiaceae (Lamium maculatum L.), Poaceae (Deschampsia caespitosa L.), and Crassulaceae, are often characterized by high accumulation of PTEs aboveground biomass (Małecka et al., 2019; Castaňares and Lojka, 2020). For example, out of 970 mg kg−1 total Zn content in soils, the leaf, stem, and root of Cratoxylum sumatranum (Jack) accumulated 223, 329, and 210 mg kg−1 of Zn, respectively, in the mountain of Magdiwata, Philippines (Castaňares and Lojka, 2020).
Furthermore, the extension of roots creates a microbe-rhizosphere community with bacteria, fungi, actinomycetes, protozoa, and algae that regulate the mobility and bioavailability of PTE (Jacob et al., 2018; DalCorso et al., 2019). Microbes that tolerate PTEs contribute to plant survival and growth in the rhizosphere. For example, rhizobium represents bacteria that capture groups of plant rhizosphere with symbiotic nitrogen (N) fixation in soils (Visioli et al., 2015) and promote OM decomposition (Geng et al., 2022). Generally, root exudates capture microbes in soils and colonize root surfaces or infiltrate root cortexes (Visioli et al., 2015).
Organic acids, phenolics, and siderophores affect the acidification process, and the dynamics of redox conditions change the potential in rhizospheres (Glick, 2010). Hence, adsorbed, precipitated, and residual fractions of PTEs solubilize by increasing acidity, chelation, and ligand-induced dissolution by microbes to become accessible to plants (Sessitsch et al., 2013). For example, under Cd, Pb, and Zn stress, Enterobacter sp. and Klebsiella sp. cause high accessibility in the root rhizosphere of Brassica napus L. (Jing et al., 2014). It is vital to understand microbial input during hyperaccumulation of PTEs and their extent of effects on hyperaccumulating plants. Such studies enable specific microbes culturing for plants suitable to alleviate PTEs contaminated soils. Although there is individual scattered literature on soil PTEs, hyperaccumulation, and microbial effects on PTE cleaning by plants, a compilation of such studies remains scarce. It is pertinent to treat environmental contamination with urgent concern as the production rate of these metals, e.g., Cd, Pb, and Zn, keep increasing over the years (Figure 1). The end-products, such as litharge, after ore processing, are often disposed of and contribute to high PTE contents, especially in soils.
The current study presents an overview that describes in detail the principles governing the uptake, translocation, accumulation, and detoxification of As, Cd, Pb, and Zn by hyperaccumulating plants. We also answer questions that pertain to the extent of microbial participation in the accessibility of PTEs by hyperaccumulators. The implications of specific rhizosphere microbes in reworking PTEs during phytoextraction by hyperaccumulators deserve critical attention. Knowledge of the intensity of accumulation of exaggerated content of As, Cd, Pb, and Zn by plant hyperaccumulators is essential in avoiding the detrimental effects on soils and living things.
2 Data acquisition and proceedings
Relevant peer-reviewed publications covering sub-headings of this study were critically elucidated from ScienceDirect, Google Scholar, Web of Science, ResearchGate, and Scopus in the last decades to date to satisfy the flow chart in Figure 2. We used the search engines to elaborate on keywords, including the impacts of PTEs on the environment, processes of PTE uptake and translocation in plants, and effects of plant hyperaccumulators on microbes growing on PTE-contaminated soils.
3 Fractionation and speciation of PTEs in soils and their interactions with plants
Different elements are well-categorized by their physicochemical forms in soils, especially in organic complexes, adsorbed in solid phases or constituents of solid/mineral phases, with diverse solubility (Wu et al., 2019; Salman et al., 2021). Plant-accessible forms represent the exchangeable complexes (organic and inorganic components) and soluble forms in soil solution taken predominantly by plants as divalent ions.
The principles underlying the mobility (e.g., high pH, redox dynamics) and immobilization (e.g., high OM contents, soil colloids) may individually or collectively complement the dynamic of elements in soils (Wu et al., 2019; Zemanová et al., 2021). For example, PTEs undergo reactive dissolution leading to bioavailability in highly acidic soil (Kicińska et al., 2021). Again, OM reduces the mobility of PTE due to high negative charges that adsorb cationic elements (Sarkar et al., 2021).
(i) Arsenic (As): Various forms of As in soils include free ionic species, precipitated as solids, adsorbed on organic or inorganic constituents, and the exchangeable and structural composition of primary and secondary minerals (Shahid et al., 2014; Joseph et al., 2015). Inorganic species of As (As (III) and As (V)) are present in forms such as fully protonated As or arsenous acids (Fayiga and Saha, 2016). Arsenate (AsO3−4) is more stable and quickly adsorbed to surfaces of clay minerals and Fe/Mn (hydro)oxides (Khalid et al., 2017), while under reduced soil conditions, arsenite (As (OH)30) becomes highly toxic, soluble, and mobile for easy plants availability (Zemanová et al., 2021). Both As (V) and As (III) are highly soluble in H2O and may change valency states depending on the pH (Parvin et al., 2021) and Eh (da Silva et al., 2018).
In As contaminated soils, organoarsenicals, e.g., monometylarsenic (MMA), dimethylarsenic (DMA), tetramethylarsonium (TMA), trimethylarsine oxide (TMAO), and tetramethylarsonium ion (TETRA) usually represent a minor component or are not detected in many soils (Shrivastava et al., 2015). Arsenic can undergo oxidation, reduction, methylation, and demethylation in soils under the modulation of microorganisms (Di et al., 2019). Arsenic mobility, availability, and toxicity in the soil-to-plant system depend on the oxidation states of As (da Silva et al., 2018).
(ii) Cadmium (Cd): Cadmium occurs in a soluble and exchangeable portion, precipitated with carbonates, occluded in amorphous Fe/Mn oxides, complexed with OM, and residual forms (Wiggenhauser et al., 2021). In soils, Cd can bind with colloids and organic amendments (e.g., hydroxyapatite-phosphate materials) to reduce mobility (Zeng et al., 2020). High alkaline soils constitute Cd precipitates (Cd (OH)2), a condition for low accumulation by plants (Gong et al., 2021; Asare et al., 2023). Additionally, low Eh can induce reductive dissolution of Fe and Mn oxides, which release bonded Cd into soil solution and increase bioaccessibility (Izquierdo et al., 2017; Wu et al., 2019).
(iii) Lead (Pb): Lead in soils exists as a free metal ion, mostly complexed with inorganic components (e.g., PbCO32–, PbHCO3 –, PbSO42–, and PbCl2−), or occurs as organic ligands (e.g., humic and amino acids) (Lodygin et al., 2020). Ionic Pb, PbII, Pb oxides and hydroxides, and Pb-metal oxyanion complexes are the general forms released into soils. Organo-Pb compounds of commercial and toxicological value are predominantly limited to the alkyl (methyl and ethyl) Pb compounds and their salts (e.g., diethyl-Pb-dichloride, dimethyl-diethyl-Pb, and trimethyl-Pb-chloride) (Wang and Mulligan, 2006). Lead shows a high affinity to soil OM and colloidal surfaces, thus, reduces, its availability in plants. Free Pb in soils often remains the only phyto-available form (Punamiya et al., 2010).
(iv) Zinc (Zn): The mobility and immobilization of Zn in soils are associated with chemical fractions; Fe/Mn oxide bound, residual (immobile), OM bound, carbonate bound, exchangeable, and soluble in H2O (Đurić et al., 2021). Soluble forms of Zn, such as ZnSO₄, are moderately mobile in many soils. Zinc content in contaminated soils occurs in immobile forms (Sharma et al., 2013). Studies show that most agricultural and industrial activities result in a high fraction (>70%) of inaccessible Zn by plants with availability (exchangeable and carbonate forms) of >30% (Mertens and Smolders, 2013; Liao et al., 2019).
In soils with free molecular oxygen, reductive dissolution of Fe/Mn (hydr)oxides releases Zn into the aqueous phase, which repartitions with Zn into sulfide and carbonate solids. In dry oxidized soils, Zn relates with (hydr)oxide phases, and in flooded systems, sulfides and carbonates (Mondillo et al., 2018). In general, the speciation and bioavailability of As, Cd, Pb, and Zn in soils are individually or collectively associated with soil reaction (pH), redox potential (Eh), dissolved organic carbon, clay content, Fe/Mn/Al (hydr)oxides, and microbial processes (Dary et al., 2010; Sungur et al., 2020).
Meanwhile, plants modulate the mobility and accessibility of PTEs by discharging root exudates: oxalic, fumaric, malic, and acetic acids (Lapie et al., 2019), which enhance solubilization. However, root exudates can remove PTEs by chelating and complexation, altering the numbers and activity of rhizosphere microbes (Jing et al., 2014; Lapie et al., 2019). Uptake of PTE is partly possible via diffusion into the root epidermis. However, the primary passage occurs via the apoplast and symplast (Yan et al., 2020). Apoplastic movement involves several transporter proteins for uptake and translocation (Guerinot, 2000). Casparian strips represent an apoplastic restriction of PTE, especially Pb (Wang et al., 2015; Wilkins et al., 2016). The uptake of PTEs through the symplast is well-governed by metal carriers or complexing agents (Asare et al., 2023). Chelation resulting from the production of phytochelatins can result in the immobilization of PTEs in roots (Flores-Cáceres et al., 2015) while remaining PTEs sequester in cellular organelles. Cadmium, As, Pb, and Zn sequestered inside the vacuoles can transport into the stele and enter the xylem via the root symplast (Thakur et al., 2016). In the apoplast, many precipitated forms of compounds, e.g., CO2-3, get immobilized. However, the mobile fractions of PTEs at this point are translocated via the apoplast or symplast movement into leaves and sequestered in extracellular parts to stop compartmental cellular accumulation (Tong et al., 2004).
4 PTE hyperaccumulators and their protein transporters
4.1 PTE hyperaccumulators
Several plants can accumulate As, Cd, Pb, and Zn above threshold levels in aboveground organs. At the organ level, leaves are well-considered in establishing the hyperaccumulating statuses of plants (Yan et al., 2020; Lima et al., 2022). Hyperaccumulators of PTEs have distinguished physiological properties capable of sequestering high contents of PTEs at a high rate (Lima et al., 2022).
Due to health and ecotoxicological reasons, PTE contents in plants have assigned global and regional permissible limits. According to WHO (1996), the content of (in mg kg−1) As- 0.1, Cd- 0.02, Pb- 2, and Zn- 0.60 should not exceed the standard reference in plants. The use of translocation (root-to-shoot quotient, TF) and bioaccumulation (plant-to-soil quotient, BF) factors over 1 are vital parameters when associated with permissible limits of PTEs determining plant hyperaccumulating abilities (Ghori et al., 2016; Souri et al., 2017). Many hyperaccumulating plant species, including Lysimachia deltoids L., Viola baoshanensis (W. S. Shu), Silene gracilicanlis L., and Gentiana sp. exhibit translocation factors >1 for Cd, Zn, and Pb (Reeves and Baker, 2000; Table 1).
Studies show that Cd, Pb, and Zn-hyperaccumulator can accumulate (in mg kg-1 dry weight) > 100 (Baker et al., 2000), >1,000 (Baker et al., 2000), and 3,000 (Reeves and Baker, 2000), respectively, in any above-ground organ. According to Baker and Whiting (2002), these high contents only provide guidelines in recognition of the extreme behavior of plants during PTE uptake. High consideration is, thus, given to the content of PTEs in contaminated soils and their respective accumulation by the aboveground biomass, especially in the case where BF > 1. Plant species such as Pteris vittata L. (Danh et al., 2014) and Isatis cappadocica Desv (Karimi et al., 2009), hyperaccumulate As. For example, Pteris vittata adopts a mechanism of arsenate and arsenite uptake, translocation, and vacuolar sequestration (Danh et al., 2014), while the stem and shoot remain unaffected by As stress (Sridhar et al., 2011). Many hyperaccumulators possess multiple abilities in accumulating several PTEs. Wu et al. (2010) observed that V. baoshanensis accumulated (in mg kg−1) 1,090 of Cd, 1,902 Pb, and 3,428 Zn in the shoots.
4.2 PTE transporters
Plant uptake and subsequent transport of PTEs are well-mediated by complex sequences. Many specialized protein transporters in different plant parts exhibit diverse abilities for PTE translocation, maintaining homeostasis, and controlling ion movements across cellular channels from roots to shoots (Ricachenevsky et al., 2013; Mishra et al., 2017). These transporters enable the re-mobilization of sugar molecules from photosynthetic tissues in leaves to roots, stems, and seeds through phloem loading. Primary protein transporter families for PTEs include;
Zinc/iron protein transporter (ZIP): Most divalent cationic elements (Cd, Ni, Cu, Co, and Fe), especially Zn, undergo uptake and active translocation in the plant system by ZIP and associated transcription genes (Table 2). Another subfamily, the iron-regulated transporters (IRTs), are implicated mainly in the transport of Fe (Verret et al., 2004). The IRT is also responsible for Cd transport in the Arabidopsis (Lee and An, 2009).
Metal tolerance protein (MTPs): This transporter transports metals at the whole plant level (Desbrosses-Fonrouge et al., 2005) and regulates homeostasis and active Zn translocation (Ricachenevsky et al., 2013). The MTP transporters have different sub-families; Fe/Zn-MTP, Zn-MTP, and Mn-MTP (Shirazi et al., 2019), and can exist in several assessions from MTP1 to MTP12 (Shirazi et al., 2019).
Members of this family and their accessions partly mediate Co, Ni, and Cd transport. Studies have previously shown MTP1 in Hordeum vulgare (HvMTP1), Oryza sativa- (OsMTP1), and Cucumis sativus (CsMTP1), with MTP3 and MTP4 in A. thaliana and Cucumis sativus, respectively, into different compartments (Shahzad et al., 2010; Yuan et al., 2012).
Yellow Strip-like transporter family (YSL): YSL is well-known for the transport of Zn and Cd; described as an essential regulator of excess Zn together with Ferric Reductase Defective3 (FRD3), a MATE (multi-drug and toxin efflux) transporter (Pineau et al., 2012). They mediate the cellular uptake of metals that form complex to non-proteinogenic amino acids (Socha and Guerinot, 2014). According to DiDonato Jr et al. (2004), YSL2 is expressed in many cell types in roots and shoots of Arabidopsis and represents a metal-regulated gene encoding transporter of nicotianamine–metal complexes. In A. thaliana, YSL4 and YSL6 buffer the adverse effects of excess Fe (Divol et al., 2013).
Natural resistance-associated macrophage protein (NRAMP): The functions of the NRAMP were previously related to Fe2+ uptake and translocation (Williams and Mills, 2005; Mishra et al., 2017). The NRAMPs are well-identified in varied compartments of Arabidopsis, and other monocot and dicot, governing the transport of divalent PTEs, e.g., Cd, Zn, and Pb (Bastow et al., 2018).
Nodulin 26-like intrinsic proteins (NIP): NIPs are cationic element transporters, including Si, Se, As, and Sb. Various species of As in soils are loaded into plants by NIP (Zhao et al., 2008; Chen et al., 2016). Evidence of As(V) species transport in P. vittate and I. cappadocica was well-demonstrated by Su et al. (2008) and Karimi and Souri (2015).
Different groups of transporters control the transport of specific PTE (Figure 3).
i. Arsenic transporters
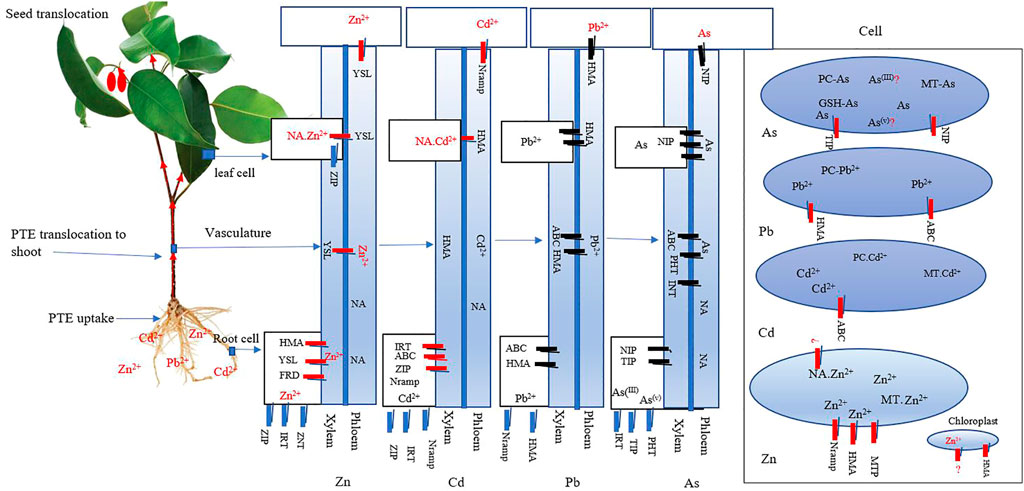
FIGURE 3. Schematic diagram showing various transporters are involved in As, Cd, Pb, and Zn uptake, transport, and sequestration in plants. Potentially toxic elements (PTEs) are taken up by root cell membrane transporters as free cations diverse transporters followed by Xylem–phloem Zn allocation occurs. The different PTEs also translocate to the leaf and seed tissues of NA-PTE complexes. Furthermore, MTs, PC, and GSH have been implicated in PTE homeostasis, transport, and sequestration in plants. Abbreviations: FRD ferric reductase defective; HMA heavy metal ATPase; IRT Fe-regulated transporter; MT metallothionein’s; MTP metal tolerance protein; NA nicotianamine; NRAMP natural resistance-associated macrophage protein; YSL yellow strip like; ZIP zinc-ion permease; ZNT zinc transporter and TIP tonoplast intrinsic protein (adopted and modified from Tao Lu, 2022).
Plant roots select As species by distinct transporters. For instance, in A. thaliana, As (v) uptake is mediated by phosphate (PHT) transporters AtPHT1, 4, 5, 7,8, and 9, while the NIP1-3, 5, and 7 for As (III) (Xu et al., 2015). Moreover, NIP has shown evidence in root-to-shoot translocation of As (III) (LeBlanc et al., 2013), with ACCC1 and 2 responsible for cellular transport of As (III) in the cytosol while in the presence of phytochelatins (Song et al., 2010).
Another transporter pertinent in As (III) is the tonoplast intrinsic protein (TIP) determined in P. vittate (He et al., 2016). Gene expressions, such as NIP1;1, NIP1;2, NIP3;1, NIP5;1, NIP6;1, and NIP7;1, are capable of coordinating H3AsO3 from the root and their subsequent transport (Bienert et al., 2008; Xu et al., 2015). The unanswered question so far is, are the same transporters solely for As species transport in hyperaccumulating plants?
ii. Cadmium transporters
Transporters Nramp, HMA, ZIP, ATP, YSL, and ABC (ATP Binding Cassette) families are involved in Cd distribution. The Nramps are well-identified in different compartments of Arabidopsis, and other monocots and dicots, Cd transport (Bastow et al., 2018). Meanwhile, Nramp1, 3, and 4 are often in charge of Cd above-organ accumulation in A. thaliana. Nramp6 is a Cd transporter, which mediates Cd transport from storage into the toxic cellular compartment. Gene transcription NcNramp1 in N. caerulescens regulates Cd influx across the endodermal plasma membrane, which implies possible root-to-shoot transport (Milner et al., 2014).
HMA1 is an efflux transporter localized in the chloroplast and controls the export of Cd from the chloroplast. HMA2 is a plasma membrane transporter involved in Cd root-to-shoot translocation, while overexpression of AtHMA3 enhanced Cd tolerance and increased its accumulation (Morel et al., 2009). AtHMA2 and AtHMA4, localized in the plasma membrane, are responsible for the xylem loading of Zn/Cd and play a key role in their accumulation in the shoots (Takahashi et al., 2012). Heavy-metal ATPase transporters are responsible for the long-distance transport of Cd from root to shoot. Additionally, gene expressions HMA4 and HMA2 regulate the influx of Cd into the stele to promote root-to-shoot transport in Arabidopsis Mishra et al. (2017).
YSL family involved in Cd transport include YSL1, YSL3, YSL6, and YSL7 (Curie et al., 2009). An excess of Cd can stimulate the expression of YLS. Overexpression of YSL1 or YSL3 in Arabidopsis increases the Cd translocation ratio under Cd stress (Tao and Lu, 2022).
iii. Lead transporters
NRAMP transporter family has been noted with the uptake of Pb, e.g., in O. sativa (Qiao et al., 2021). HMA3, a vacuolar P1B-ATPase, mediates the sequestration of Pb2+ and other cationic metals, into the vacuole of different plant species (Liu et al., 2018). ABC transporters and their subfamilies also modulate Pb transport. For example, the transcription gene ABCG36 of poplar hybrid located on the plasma membrane transports Pb2+ from the cytoplasm across the plasma membrane (Abolghassem et al., 2015). Expression ABCG48 was upregulated in the shoots and roots of plants treated with Pb2+ (Abolghassem et al., 2015). A homolog of HMA5 in poplar, PtHMA4, was also found to be localized at the plasma membrane. Meanwhile, the upregulation of Pb in the root by PtHMA4, suggests Pb from roots to shoots (Qiao et al., 2021). In Arabidopsis helleri L, AtHMA3 participates in Pb2+ sequestration by transporting it to the vacuoles (Hasan et al., 2017).
iv. Zinc transporters
ZIP transfers Zn to the stele in roots. For example, Noccaea caerulescen (J. Presl. & C. Presl.) and A. halleri exhibit improved Zn uptake (Assunção et al., 2001) and are represented by ZNT1 (Van de Mortel et al., 2006). The NcZNT1 gene expression and AtZIP4 for A. thaliana are active in the cortex, endodermis, and pericycle cells. These expressions are also in the same tissues of N. caerulescens but are not limited to Zn-deficient conditions (Van de Mortel et al., 2006; Lin et al., 2016). Zinc transporter 2 (ZNT2) and ZNT5 are also responsible for Zn2+ transport in the root cortex or by diffusion (Lin et al., 2016). The Zn ion is sequestered for vacuole storage and transported to the endodermis by ZIP23, ZIP19, ZIP5, and IRT3. Zinc ion transport stops by the apoplastic barrier (e.g., Casparian strip) and enters the endodermis via ZNT1/ZIP4. Transcriptional-level analysis shows that HMA2 and HMA4 form vital components of Zn hyperaccumulation and hyper-tolerance in A. halleri - AhHMA2 and AhHMA4 (Hanikenne et al., 2008; Frérot et al., 2018). Moreover, in the root pericycle, AhHMA2 and AhHMA4 promote Zn2+ efflux and loading in the root xylem (Hussain et al., 2004). Again, HMA3 mediates the sequestration of cationic Zn into the vacuole.
Zinc can transport from the root to shoot by ZIP4 and IRT3, form chelates, or possibly diffuse into the pericycle cells (the outermost part of the stele). Enhanced accumulation of Zn in the shoot by overexpression of IRT3 was suggested by Balafrej et al. (2020). Meanwhile, Zn enters the leaf cell either in chelation with low-molecular-weight ligands or as free Zn2+ complemented by ZIP4 and ZIP6 (Sinclair and Kramer, 2012). Several gene transcriptions from different transporters (e.g., MTP1 and 8, HMA3 and 4, and NRAMP3) promote the transport of Zn into the vacuole. The long-distance root-shoot translocation of Cd and Zn is solely responsible for HMA4 (Verret et al., 2004). Additionally, overexpression of HMA4 enhances Zn2+ efflux from the root symplast into the xylem vessels and promotes metal tolerance (Verret et al., 2004). After Zn loading in the xylem by different transporters: HMA, ZIP, and YLS, Zn reaches the leaves, where it binds to organic acids, e.g., malate and citrate, and sequesters in vacuoles. Unchelated Zn at the apoplastic barrier reaches the xylem through YSL or direct diffusion. Zn2+ crosses the xylem as free Zn or coupled with histidine, citrate, or malate (Tao and Lu, 2022). YSL transporters are also known for loading and unloading Zn in the xylem (Curie et al., 2009).
5 Persistence of hyperaccumulators under PTE stress
Plants, especially hyperaccumulators, either tolerate or detoxify PTEs to persist under high PTE (Dalvi and Bhalerao, 2013). Already taken ionic PTEs must undergo intracellular mechanisms to tolerance, including (a) cellular and subcellular compartmentalization of PTEs, (b) formation of chelates-reducing the toxicity of ionic forms of PTEs (Dalvi and Bhalerao, 2013), and (c) transportation capability. Meanwhile, other detoxification approaches involved in PTE tolerance in plants, such as PTE-immobilization in cell walls, impeded permeation across cell membranes, and active export into the apoplast (DalCorso et al., 2019).
Stress from PTEs triggers the production and accumulation of bioactive substances in plants. For instance, proline accumulation results from Cd, Pb, and Zn stress on plants (Roy and Bera, 2002). The detoxification process begins as proline (comprised of amino acids) chelates with PTE (Rai, 2002). Inadequate detoxification increases PTEs accumulation in the cytoplasm, enabling the release of reactive oxygen species (ROS). Excess production of ROS results in oxidative stress, may disrupt cell homeostasis, inhibit cellular processes, DNA damage, and protein oxidation (DalCorso et al., 2019). In PTE-induced oxidative damage, plant cells activate the ROS-scavenging system, which induces antioxidant enzymes (e.g., superoxide dismutase, catalase, peroxidase, and glutathione reductase) and non-enzymatic antioxidant compounds, e.g., metabolites (DalCorso et al., 2019).
(i) Arsenic (As): In the cells of roots, As is converted to less toxic forms, transported to vacuoles as AsIII, and forms complexes with glutathione/phytochelatins (Souri et al., 2017). The formation of As (III) complexes with γ-glutamyl-cysteinyl-glycine and phytochelatins (PC) and their transport into roots and shoots forms the dynamics of As coping (Souri et al., 2017). In the vacuole, As is predominantly sequestered in the form of phytochelatins (PC)-As or GSH (glutathione) conjugates (Kumar et al., 2015). Thus, the transporters responsible for vacuole sequestration of PC-As or GSH-As conjugate control As detoxification. Volatile fractions of reduced organo-arsenicals taken up by plants partly disappear via stomatal openings during phytovolatilization (Limmer and Burken, 2016).
For instance, As uptake by P. vittata is achieved through a high-affinity phosphate transport system, having the ability to store complexes in vacuoles of leaf cells (Singh et al., 2016). Ionic forms of PTE can sequestrate into petioles, sheathes, and trichomes of leaves (Eapen and D’souza, 2005; Yan et al., 2020). Transport systems with the ability to store complexes in vacuoles of leaf cells (Singh et al., 2016). Meanwhile, organic acids within cells prevent PTEs as free ions in the cytoplasm by forming complexes. However, this reduces the further availability y of PTEs. For example, Malate is involved in the chelation of Zn in A. halleri (Shanmugam et al., 2013). Translocated As species are partly detoxified by ABC and ACR3 (arsenite transporters), an arsenic transporter (Thounaojam et al., 2021).
(ii) Cadmium (Cd): Plants exhibit efficient strategies to respond to Cd in their environments. In the root, Cd forms chelate with ligands in the cell wall, cytoplasm, and vacuole resulting in its immobilization, thereby losing toxicity (Ali et al., 2013). Root vacuole storage of Cd reduces its toxicity and long-distance transport to shoot (Thakur et al., 2016). Cd is sequestered in shoots, and detoxification occurs in cell walls or plant vacuoles. The main mechanism of PC-mediated Cd detoxification is chelation by PCs to form a complex, which is then transported into the vacuoles by ABC transporters (Vatamaniuk et al., 2000; Zhang et al., 2018). Again, metallothionein, minute peptides that contain cysteine also act as cytoplasmic Cd chelation proteins (Zhang et al., 2013). Studies show that the cell wall, particularly pectin increases plant Cd tolerance by preventing Cd from entering root cells (Gutsch et al., 2018). The studies by Luo and Zhang, (2021) indicate that the expression of different genes occurs in the cell wall, which supports Cd hyperaccumulation and detoxification, e.g., in Sedum plumbizincicola.
InBrassica napus (Bna), ABCC sub-transporters BnaABCC3 and BnaABCC4 were upregulated under Cd stress and enhanced Cd tolerance by limiting the entry of Cd inside the cells and their phytochelatins-mediated detoxification (Yamaji et al., 2013).
(iii) Lead (Pb): Pb in plant tissue activates cellular responses and changes in signaling mechanisms and gene expression (Kumar and Prasad, 2018). These mechanisms trigger the release of specific metabolites, e.g., phytochelatins (PC), glutathione, and metallothionein (Estrella-Gómez et al., 2009). These bioactive materials, e.g., PC effectively bind with Pb and transport it to the vacuole, where detoxification of Pb occurs. Reportedly, Pb accumulation in plant tissues increases the expression of the PCS gene with a concomitant increase in PCs in aquatic fern Salvinia minima, a known Pb-hyperaccumulator (Estrella-Gómez et al., 2009). Increased synthesis of PCs reduces free Pb content in the cytoplasm and shows a strong correlation with the suppression of stress-related responses in plants (Estrella-Gómez et al., 2009). Finally, PTE complexation with glutathione, amino acids, and PCs is transported to the tonoplast or vacuole, where active detoxification and cell removal occur.
(iv) Zinc (Zn): Firstly, Zn storage in root vacuoles is used as a mechanism of Zn exclusion from the shoot in the presence of excess Zn influx into roots or excess Zn supply in the soil (references). The accumulation of Zn in different cells, e.g., trichomes, is thought to act as a detoxification and storage strategy under excess Zn growth conditions (Ricachenevsky et al., 2021). Zinc import into subcellular compartments is also a vital detoxification strategy. Meanwhile, studies show that AtHMA3 is involved in the vacuole sequestration of Zn2+ and the overexpression of AtHMA3 resulting in hyper-tolerance in A. thaliana (Miyadate et al., 2010).
6 The rhizosphere definition and its role
The rhizosphere elaborates on the root system and its close soils that foster biological and chemical activities, e.g., by exudation, for plant security and growth (Hartmann et al., 2009; Chamkhi et al., 2022). Rhizosphere represents nutrient-rich zones in soils that exhibit the capacity to perform extended functions for plants during stress (Chamkhi et al., 2022). However, the region supports beneficial and non-cooperative associations between rhizosphere microbes and plants (Figure 4). Due to the diverse root structure, the rhizosphere may exhibit varied size ranges. Hence, the properties of this region change only along roots. Meanwhile, about 90% of plants have roots extending from 0.27 to 0.9 m in soils, with a few reaching 1.82 m (Gilman, 1990).
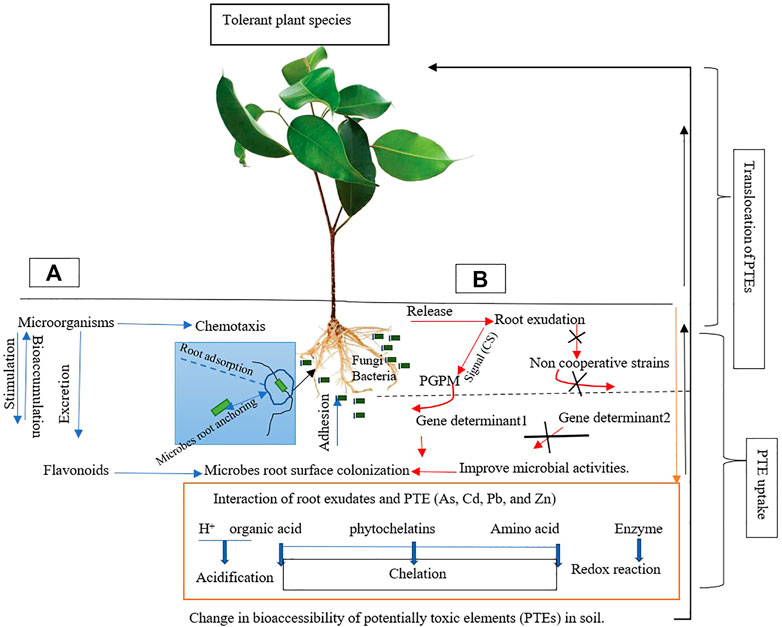
FIGURE 4. Shows the root rhizosphere region and the relationship between microbes and plants during the uptake of potentially toxic elements (PTEs) in soils. The process is well-supported by the production of root exudates. Plant growth-promoting microbes (PGPM), with a high preference for root exudation, colonize root surfaces and rework PTEs (adopted and modified from Ma et al., 2016). (A) indicates microbial activities; (B) indicates root exudation + microbial interaction.
The secretion of different bioactive (metabolites) compounds directing depends on the type of plant (genotype) and stress elicitor (e.g., abiotic and biotic). For example, roots can discharge their photosynthetically fixed C- into the soil (Sasse et al., 2018). The composition of exudates significantly impacts the activities and microbial count and diversity. Increased microbial count and activities in rhizosphere regions are a function of metabolite discharge (Sasse et al., 2018). Microbial preferences in the rhizosphere also depend on the influence of root exudates, which can affect the dissolution of PTEs for effective uptake. Hence, exudate patterns are vital drivers that shape the rhizosphere microbiome (Zhalnina et al., 2018) and are plant species-specific (Gianfreda, 2015).
Knowledge of the soil–root interface is pertinent to managing microorganisms, increasing plant growth, and reducing the effect of plant production. However, with the identification of plant genomes and the genes induced under different PTE stress, e.g., encoding for transporter proteins, metal sequestering peptides, and enzymes metabolism in plants, the principles governing PTEs uptake, accumulation, transport, chelation, and detoxification provide vital information for effective soil decontamination.
6.1 Rhizosphere microbe-plant interactions
Rhizosphere microbes exhibit movement to discharge pulses along plant roots (Hünninghaus et al., 2019) and play a vital role in regulating nutrition in plants, e.g., N fixation (Xiong et al., 2021). These microbes can compete and populate the root rhizosphere (Schreiter et al., 2018; Mulero-Aparicio et al., 2019). The attraction of microbes to the rhizosphere depends on the characteristics of the roots. Microbe-plant interaction in the rhizosphere involves chemical processes that partly account for the chelation and solubilization/dissolution of PTEs to promote mobility or immobilization (Figure 4). Although some microbes may not show a direct affinity for root exudates and many physicochemical properties of soils, especially toward the dissolution of PTEs, but contribute to their detoxification in soils. For example, Bacillus subtilis shows high tolerance and adsorption for Cd and Pb, a means of detoxification and elimination (Li et al., 2022). Soil properties, e.g., OM, organic C, and clay contents, influence microbial functional diversity (Li et al., 2022).
Microbes exhibiting resistance to the long-term effect of PTE contamination provide a basis for selecting suitable microbial species to assist in phytoremediation (Zubair et al., 2016; Table 2). Bacteria can help plants to resist stress and improve plant growth and productivity. For example, resistant bacteria can transform PTEs into less toxic forms and alter their availability for possible plant uptake or chelation (Whiting et al., 2001). Rhizosphere microbes such as Acinetobacter, Bacillus, Gluconacetobacter, and Pseudomonas affect the bioavailability of Zn (Costerousse et al., 2017), which involves pH reduction (acidic) and root growth. Additionally, the presence of mycelium fungi contributes to the increase in plants’ tolerance to excess Cd and Zn in Salix sp (Hrynkiewicz et al., 2012). Some rhizosphere microbes promote plant growth by direct interactions with plants or indirect antagonistic activities against plant pathogens. Pathogens affect many bacteria and fungi.
A typical example is endophyte resistance toward pathogenic space and nutrients in the rhizosphere and plant tissues (Vogel-Mikuš et al., 2006). The apical roots are involved in the active selection of specific microbe. Studies show that the polysaccharide composition of root mucilage for microbes with suitable glycosyl hydrolase composition (Amicucci et al., 2019) and further by extracellular DNA, antimicrobial proteins, and secondary metabolites (Haichar et al., 2014). Meanwhile, arbuscular mycorrhiza and ectomycorrhiza avoid PTEs from root uptake via absorption or chelation, indicating resistance (Hall, 2002).
Loading of PTEs in plant cell walls represents an approach to metal avoidance (Memon and Schroder, 2009; Krzeszowska et al., 2021). Cell wall pectin made of negative carboxylic groups of polygalacturonic acids can bond with cationic PTEs. The cation exchange reactions prevent free metallic ions from entering plant cells. Various interacting microbes produce phytohormones, which inhibit or promote root growth, protect plants against abiotic stress, and improve nutrient acquisition by roots (Gupta et al., 2014). One pertinent role of microbes in plant nutrition and health is the association between rhizosphere fluorescent pseudomonas and plants, while others suppress plant diseases and fungal pathogens from OM (Kumari and Kumar, 2018). For example, in soils suppressive to the fungal pathogen Rhizoctonia solani, Raza et al. (2016) reported that proteobacteria, firmicutes, and actinobacteria were prominent taxa involved in disease suppression.
Stable isotope study of plants and root-associated microbes shows dynamics in taxonomic composition and activities of microbial consumers. For example, Hünninghaus et al. (2019) pointed out that bacterial taxa with the highest abundance in the root of Zea mays L. were not necessarily those showing the highest enrichment of 13C from rhizodeposition, indicating substantial differences in consumption of exudates. Active and passive exudation of low molecular weight carbon compounds, e.g., sugars and organic acids, during root expansion and root hair zones (Canarini et al., 2019) constitutes microbial community modulation (Hu et al., 2018). These locations also modulate enzymatic activities (Zhang et al., 2020) and affect PTEs in soils and plant accessibility.
Arsenic reduction, methylation, and demethylation depend on the type of microbes in the soil. Under anaerobic conditions, organoarsenicals reduce to volatile arsine, such as monomethylarsonous acid [MMA(III)], dimethylarsinous acid [DMA(III)], and trimethylarsine TMA(III) (Zhang et al., 2021). Microbial As methylation is an effective method for As detoxification in microbes as a bioremediation approach.
6.2 Effects of PTEs on rhizosphere microbes during hyperaccumulation
Many rhizosphere microbiomes can tolerate high contents of Zn, As, Cd, and Pb (Abou-shanab et al., 2019), which contribute significantly to the mobility and immobilization of PTE in soils.
Rhizosphere microbes, such asbacterial siderophores, can increase PTE accumulation and induce tolerance during phytoremediation (Benizri and Kidd, 2018). For instance, rhizobacteria, endophytes, siderophores, carboxylic acids, and phosphate solubilizers can contribute to the dissolution of As for plant uptake (He et al., 2013; Cabello-Conejo et al., 2014). Plant-associated microbes protect host plants by releasing phytohormones to combat biotic and abiotic elicitors that cause adverse effects on plants.
Moreover, the secretion of root exudates by hyperaccumulating plants and the attraction of rhizosphere microorganisms can significantly affect the availability of PTEs (Figure 4). For example, a Cd content of 7 mg kg-1 in soil caused a reduction in rhizobium populations (Chaudri et al., 1992). Thus, high contents of some PTE can reduce microbial diversity and abundance (Abou-Shanab et al., 2005). For instance, the inoculation of Bacillus sp., Delftia sp., Pseudomonas sp., Pseudoxanthomonas sp., and Variovorax sp. on P. vittata plants under As stress resulted in increased plant biomass and As removal efficiency in soils (Yang et al., 2012).
Changes in pH affect microbial abundance and diversity, which are directly associated with the solubility of PTEs. According to Delorme et al. (2001), a high alkaline pH by adding lime increased bacteria count reduced Zn toxicity and enabled the growth of N. caerulescens and Trifolium pratense L. Meanwhile, increasing acidity promotes the high solubility of PTE for effective bioaccumulation (Becker and Skaar, 2014). Microbial counts also affect hyperaccumulators, probably because of the different secretions.
For example, N. caerulescens recorded a higher microbial population in Zn-contaminated soil than Trifolium pratense L. (Delorme et al., 2001) via acidification by microbes, which improves Zn availability, uptake, and selection for metal-resistant bacteria. Bacterial strains with Hexa-, Penta-, Tetra-, and Trivalent-metal ions tolerant are often abundant in the rhizosphere zone, where they can resist high Cd and Pb contents (Abou-Shanab et al., 2005). A unified multi-microbial influence on PTEs may result in fast stabilization or dissolution. These multi-facet abilities of diversified microbes in the rhizosphere are helpful during high levels of PTEs, as this can affect the uptake (Gremion et al., 2004). Antibiotics, phosphate solubilizers, hydrocyanic and indoleacetic acids, and siderophores obtained from some rhizobacteria increase Cd availability and facilitate absorption in plant roots (Sharma and Archana, 2016; He et al., 2020). Microbial populations often establish some positive relationship with the host plant system. Soil contaminated with PTEs can lead to the appearance of resistant rhizobacteria (Henao and Ghneim-Herrera, 2021; Table 3). Hyperaccumulating plant species of PTEs can significantly shape the rhizosphere ecosystem and impact the microbial community to favor the reduction of As, Cd, Pb, Zn, and many other pollutants. For example, Actinobacteria, Bacteroidetes, and genus Streptomyces were well-detected as dominant traits in Cd-stressed hyperaccumulator Sedum alfredii compared to non-hyperaccumulating ecotypes (Hou et al., 2018). Thus, S. alfredii and Cd exposure select suitable microbes for hyperaccumulation. According to Lu et al. (2023), Acidobacteria, Bacteroidetes, Deltaproteobacteria, and Gemmatimonadetes regulate excess Cd uptake and accumulation in Triticum aestivumL. with microbes partly influenced by soils with high OM.
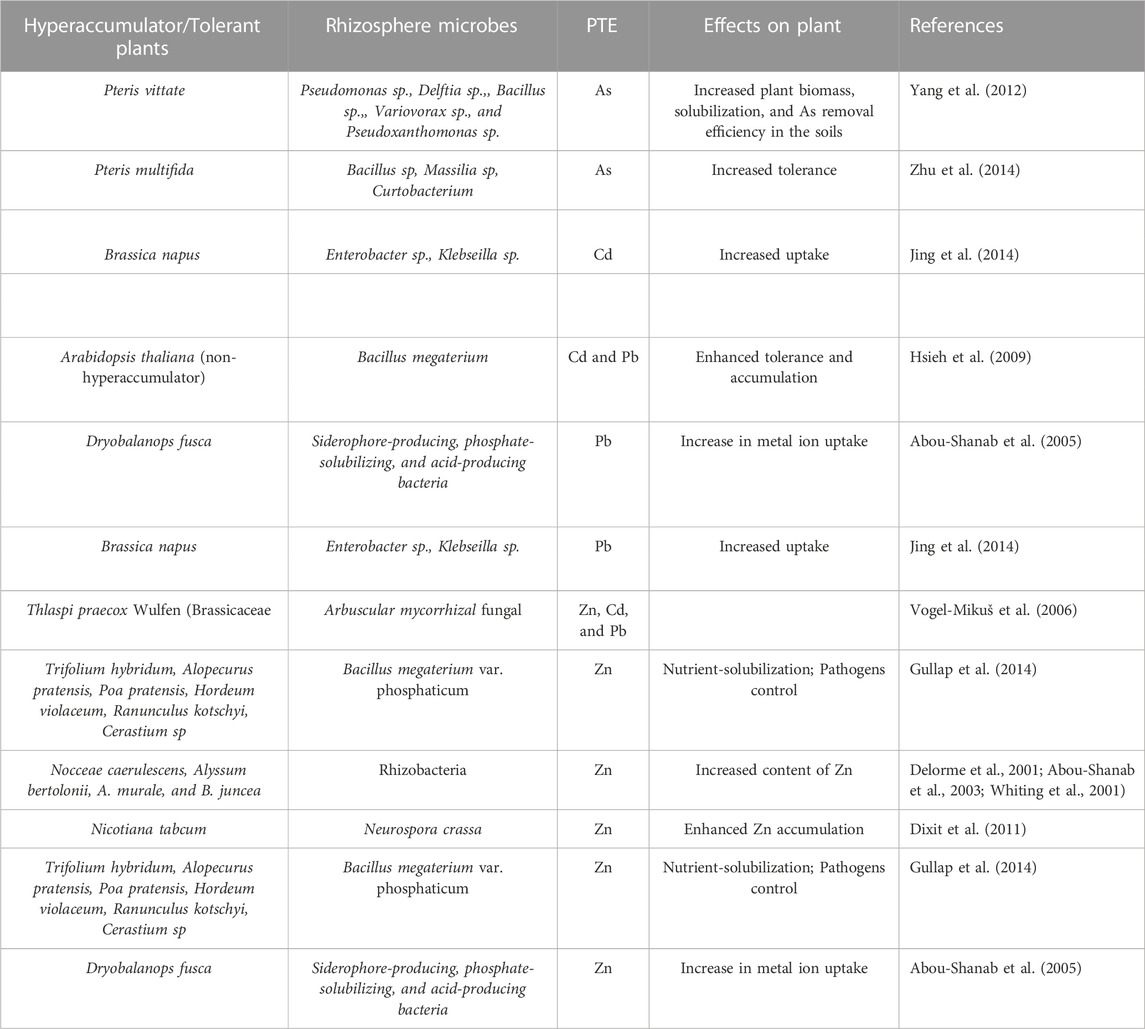
TABLE 3. Effects of rhizosphere microbes on plant hyperaccumulators/tolerant of Potentially toxic elements (PTE).
Studies have shown that a high proportion of Zn-resistant bacteria persist in the rhizosphere of the hyperaccumulator N. caerulescens (Delorme et al., 2001; Whiting et al., 2001) and Alyssum bertolonii Desv (Sessitsch et al., 2013) or Alyssum murale M. Bieb (Abou-Shanab et al., 2003) grown in soil contaminated with Zn. The addition of bacteria increased the shoot Zn content in N caerulescens about two times and the rate of soluble Zn transport compared to axenic controls (Whiting et al., 2001). Enzymatic processes during detoxification and activation of chemicals in plants are similar to microbial biotransformation pathways in soils.
The comparison of biochemical isolates such as phosphate solubilizers, siderophore, and acids showed that phosphate solubilizers dissolve 82.2% Zn and 68.2% of Pb, siderophores (71.02% Zn and 61.6% Pb), and acids—53.3% Zn and 42.9% Pb (Abou-Shanab et al., 2005). Hence, several organic materials released by microbes in the root rhizospheres are responsible for the dissolution of PTEs for plants’ availability. According to Singh et al. (2022), the presence of siderophores decreased the uptake of metals by plants. Siderophores produced by Pseudomonas sp., Serratia marcescens, and Streptomyces sp. had either no effect or negatively affected Zn uptake by Salix capreastrum. These effects indicate that the principles underlying metal uptake are also plant-dependent. The efficiency of siderophore producers in mobilizing or immobilizing soil PTEs depends on the binding form of metal/(loid)s, the charge of siderophores, soil pH, mineral composition, and organic content (Jing et al., 2007). Soil microbiome, particularly bacteria, containing enzymes, such as 1-aminocyclopropane-1-carboxylic acid (ACC) deaminase, limits secretion in stressed plants (Tiwari et al., 2018). Moreover, arbuscular mycorrhizal fungi contribute to plant PTE uptake (Kranner and Colville, 2011). Therefore, mycorrhizal fungi with high metal contents tolerance and biomass production can be applied for phytoextraction (Sagardoy et al., 2010). Thus, it is pertinent to co-inoculate PTE hyperaccumulators with desired microbes, e.g., Arthrobacter and other Microbacterium, that can improve uptake and translocation (Visioli et al., 2015).
7 Future perspectives
Microbes exhibit preference for specific PTE during hyperaccumulation. These attractions result from the secretion of exudates into the root rhizosphere region. Meanwhile, the entire microbial community may not necessarily benefit from the chelation or dissolution of PTEs for effective uptake by plants. Culturomics purposed on adapting beneficial microbes can improve the accessibility of PTEs.
Gene encoding involved in the uptake and translocation of Pb remains less published among crops such as Oryza sativa (Gong et al., 2022), while so far neglected in the hyperaccumulation of risk elements from contaminated sites. The induction of many of these transporter families and the use of suitable nano/microparticles remain the next level of concern for the remediation of PTE-contaminated sites. Mutation and ionome modifications of plant species with effective uptake and above-ground accumulation offer another innovative approach to PTE management in contaminated soils for sustainable use (Navarrete and De La Fuente, 2015).
8 Conclusion
Anthropogenic interferences contribute significantly to excess potentially toxic elements (PTES; As, Cd, Pb, and Zn) above the threshold of agricultural soils. Hyperaccumulating plants (above-ground accumulators of PTEs) possess uptake ability and accumulate the accessible portion of PTEs associated with the exchange complexes and soluble forms in the soil solution.
Additionally, soil characteristics, including high organic matter, carbon, and clay content increases microbial biomass and functionality and induce changes that regulate soil microbial community. These properties affect microbial abundance and increase the chances of high PTE reworking to ease uptake.
Root exudations of hyperaccumulators contribute to microbes’ selection. Microbial stimulation (chemotaxis and excretion), e.g., flavonoids, also provide an anchor to colonize the root surface to support the solubilization and uptake of PTEs. The production rate of root exudate by hyperaccumulators and the attraction of rhizosphere microbes can significantly affect the bioavailability and bioaccumulation of PTEs, which enhances phytoextraction by hyperaccumulators. Changes in soil physicochemical properties (e.g., pH and redox potential) and root exudation affect microbial abundance and diversity, which also influence the solubility, mobility, and accessibility of PTEs. Multiple resistance of As, Cd, Pb, and Zn by microbes can assist many hyperaccumulators during PTE uptake. Diversified microbes in the root rhizosphere are vital during high levels of PTEs, as this can increase metal uptake while plants utilize them to avoid toxicity.
Meanwhile, different transporters, including Zinc-iron protein (ZIP), Metal Tolerance Protein (MTP), Heavy metal ATPases (HMA), Yellow Strip-like (YSL), and Natural resistance-associated macrophage proteins (NRAMPs) and their transcription genes are responsible for the influx/efflux of PTEs to plant compartments. Many hyperaccumulators show unique transcriptional transporters associated with fast uptake of high contents of PTEs and subsequent translocation to the aerial parts. Part of the bioavailable fractions of the PTEs is chelated with intracellular organs of hyperaccumulators as a means of detoxification, while exudation contributes to tolerance.
Thus, hyperaccumulation depends on the availability of mobilized metal ions in soils, enhanced activity of metal transporters, and metal chelates/solubilizers provided by plants or their associated microbes.
Incorporating different transporter genes into plants to improve the ability for PTEs hyperaccumulation needs attention. The microbial community in the root rhizosphere during PTE hyperaccumulation is not well-studied. The inoculation of suitable transporter genes and rhizosphere microbes for an effective hyperaccumulation of PTEs requires critical studies.
Author contributions
Conceptualization: MA, JS, and PT, Data curation: MA and JS, Formal Analysis: MA, JS, and PT, Funding acquisition: JS and PT, Methodology: MA, JS, and PT, Project administration: JS and PT, Resources: JS and PT, Supervision: JS and PT, Validation: JS and PT Writing–original draft: MA and JS, Writing–review and editing: MA, JS, and PT.
Funding
The study received support from the Nutrisk project (European Regional Development Fund–Project No. CZ.02.1.01/0.0/0.0/16_019/0000845).
Conflict of interest
The authors declare that the research was conducted in the absence of any commercial or financial relationships that could be construed as a potential conflict of interest.
Publisher’s note
All claims expressed in this article are solely those of the authors and do not necessarily represent those of their affiliated organizations, or those of the publisher, the editors and the reviewers. Any product that may be evaluated in this article, or claim that may be made by its manufacturer, is not guaranteed or endorsed by the publisher.
References
Abolghassem, E., Ding, Y., Mokhberdoran, F., and Xie, Y. F. (2015). Heavy metal stress and some mechanisms of plant defense response. Sci. World J. 2015, 756120. doi:10.1155/2015/756120
Abou-Shanab, R. A., Angle, J. S., Delorme, T. A., Chaney, R. L., Van Berkum, P., Moawad, H., et al. (2003). Rhizobacterial effects on nickel extraction from soil and uptake by Alyssum murale. New Phytol. 158, 219–224. doi:10.1046/j.1469-8137.2003.00721.x
Abou-Shanab, R. A., Ghozlan, H., Ghanem, K., and Moawad, H. (2005). Behaviour of bacterial populations isolated from rhizosphere of diplachne fusca dominant in industrial sites. World J. Microbiol. Biotechnol. 21, 1095–1101. doi:10.1007/s11274-004-0005-6
Abou-shanab, R., El-Sheekh, M., and Sadowsky, M. J. (2019). ““Role of rhizobacteria in phytoremediation of metal-impacted sites”,” in Emerging and eco-friendly approaches for waste management. Editors R. Bharagava, and P. Chowdhar (Singapore: Springer). doi:10.1007/978-981-10-8669-4_14
Ali, H., Khan, E., and Sajad, M. A. (2013). Phytoremediation of heavy metals-concepts and applications. Chemosphere 91, 869–881. doi:10.1016/j.chemosphere.2013.01.075
Amicucci, M. J., Galermo, A. G., Guerrero, A., Treves, G., Nandita, E., Kailemia, M. J., et al. (2019). Strategy for structural elucidation of polysaccharides: Elucidation of a maize mucilage that harbors diazotrophic bacteria. Anal. Chem. 91, 7254–7265. doi:10.1021/acs.analchem.9b00789
Asare, M. O., Száková, J., and Tlustoš, P. (2023). The fate of secondary metabolites in plants growing on Cd-As-and Pb-contaminated soils-a comprehensive review. Environ. Sci. Pollut. Res. Int. 30, 11378–11398. doi:10.1007/s11356-022-24776-x
Ashraf, S., Ali, Q., Zahir, Z. A., Ashraf, S., and Asghar, H. N. (2019). Phytoremediation: Environmentally sustainable way for reclamation of heavy metal polluted soils. Ecotoxicol. Environ. Safe 174, 714–727. doi:10.1016/j.ecoenv.2019.02.068
Assunção, A., Martins, P. D. C., De Folter, S., Vooijs, R., Schat, H., and Aarts, M. (2001). Elevated expression of metal transporter genes in three accessions of the metal hyperaccumulator Thlaspi caerulescens: Zinc transporters of Thlaspi caerulescens. Thlaspi Caerulescens. Plant, Cell. Environ. 24, 217–226. doi:10.1111/j.13653040.2001.00666.x
Baker, A. J. M., and Whiting, S. N. (2002). In search of the Holy Grail - a further step in understanding metal hyperaccumulation? New Phytol. 155, 1–4. doi:10.1046/j.1469-8137.2002.00449_1.x
Baker, A. J. M., McGrath, S. P., Reeves, R. D., and Smith, J. A. C. (2000). “Hyperaccumulator plants: A review of the ecology and physiology of a biological resource for phytoremediation of metal-polluted soils,” in Phytoremediation of contaminated soil and water. Editors N. Terry, and G. Banuelos (London: Lewis Publishers), 85–107.
Balafrej, H., Bogusz, D., Triqui, Z. A., Guedira, A., Bendaou, N., Smouni, A., et al. (2020). Zinc hyperaccumulation in plants: A review. Plants 9, 562. doi:10.3390/plants9050562
Bastow, E. L., Garcia De La Torre, V. S., Maclean, A. E., Green, R. T., Merlot, S., Thomine, S., et al. (2018). Vacuolar iron stores gated by NRAMP3 and NRAMP4 are the primary source of iron in germinating seeds. Plant Physiol. 177, 1267–1276. doi:10.1104/pp.18.00478
Becker, K. W., and Skaar, E. P. (2014). Metal limitation and toxicity at the interface between host and pathogen. FEMS. Microbiol. Rev. 38, 1235–1249. doi:10.1111/15746976.12087
Benizri, E., and Kidd, P. (2018). The role of the rhizosphere and microbes associated with hyperaccumulator plants in metal accumulation, in Agromining: Farming for metals. Mineral resource reviews. Editors A. Van der Ent, G. Echevarria, A. Baker, and J. Morel (Cham: Springer). doi:10.1007/978-3-319-61899-9_9
Berhongaray, G., Verlinden, M. S., Broeckx, L. S., and Ceulemans, R. (2015). Changes in belowground biomass after coppice in two Populus genotypes. Forest Ecology and Management 337, 1–10.
Bienert, G. P., Thorsen, M., Schüssler, M. D., Nilsson, H. R., Wagner, A., Tamás, M. J., et al. (2008). A subgroup of plant aquaporins facilitate the bi-directional diffusion of As(OH)3 and Sb(OH)3across membranes. Bmc. Biol. 6, 26. doi:10.1186/1741-7007-6-26
Bisht, N., and Chauhan, P. S. (2020). “Excessive and disproportionate use of chemicals cause soil contamination and nutritional stress,” in Soil contamination - threats and sustainable solutions. Editors M. L. Larramendy, and S. Soloneski (Rijeka: IntechOpen). doi:10.5772/intechopen.94593
Boente, C., Sierra, C., Martínez, J., Rodríguez-Valdés, E., Afif, E., Rey, J., et al. (2022). Impact of old Pb mining and metallurgical production in soils from the Linares mining district (Spain). Environ. 9, 24. doi:10.3390/environments9020024
Bridgwater, A. V., Meier, D., and Radlein, D. (1999). An overview of fast pyrolysis of biomass. Org. Geochem. 30, 1479–1493. doi:10.1016/s0146-6380(99)00120-5
Cabello-Conejo, M., Becerra-Castro, C., Prieto-Fernández, A., Monterroso, C., Saavedra-Ferro, A., Mench, M., et al. (2014). Rhizobacterial inoculants can improve nickel phytoextraction by the hyperaccumulator Alyssum pintodasilvae. Alyssum Pintodasilvae. Plant Soil 379, 35–50. doi:10.1007/s11104-014-2043-7
Canarini, A., Kaiser, C., Merchant, A., Richter, A., and Wanek, W. (2019). Root exudation of primary metabolites: Mechanisms and their roles in plant responses to environmental stimuli. Front. Plant Sci. 10, 157. doi:10.3389/fpls.2019.00157
Castaňares, E., and Lojka, B. (2020). .Potential hyperaccumulator plants for sustainable environment in tropical habitats. IOP. Conf. Ser. Earth. Environ. Sci. 528, 012045. doi:10.1088/17551315/528/1/012045
Cempel, M., and Nikel, G. (2006). Nickel: A review of its sources and environmental toxicology. Pol. J. Environ. Stud. 15, 375–382.
Chamkhi, I., El Omari, N., Balahbib, A., El Menyiy, N., Benali, T., and Ghoulam, C. (2022). Is the rhizosphere a source of applicable multi-beneficial microorganisms for plant enhancement? Saudi J. Biol. Sci. 29, 1246–1259. doi:10.1016/j.sjbs.2021.09.032
Chaudri, A. M., McGrath, S. P., and Giller, K. E. (1992). Survival of the indigenous population of Rhizobium leguminosarum biovar trifolii in soil spiked with Cd, Zn, Cu, and Ni salts. Soil Biol. biochem. 24, 625–632. doi:10.1016/0038-0717(92)90040-5
Chen, Y., Fu, J. W., Han, Y. H., Rathinasabapathi, B., and Ma, L. Q. (2016). High as exposure induced substantial arsenite efflux in As-hyperaccumulator Pteris vittata. Chemosphere 144, 2189–2194. doi:10.1016/j.chemosphere.2015.11.001
Costerousse, B., Schönholzer-Mauclaire, L., Frossard, E., and Thonar, C. (2017). Identification of heterotrophic zinc mobilization processes among bacterial strains isolated from wheat rhizosphere (Triticum aestivum L.). Appl. Environ. Microbiol. 84, e01715–e01717. doi:10.1128/AEM.01715-17
Curie, C., Cassin, G., Couch, D., Divol, F., Higuchi, K., Le Jean, M., et al. (2009). Metal movement within the plant: Contribution of nicotianamine and yellow stripe 1-like transporters. Ann. Bot. 103, 1–11. doi:10.1093/aob/mcn207
da Silva, E. B., de Oliveira, L. M., Wilkie, A. C., Liu, Y., and Ma, L. Q. (2018). Arsenic removal from As-hyperaccumulator Pteris vittata biomass: Coupling extraction with precipitation. Chemosphere 193, 288–294. doi:10.1016/j.chemosphere.2017.10.116
DalCorso, G., Fasani, E., Manara, A., Visioli, G., and Furini, A. (2019). Heavy metal pollutions: State of the art and innovation in phytoremediation. Int. J. Mol. Sci. 20 (14), 3412. doi:10.3390/ijms20143412
Dalvi, A. A., and Bhalerao, S. A. (2013). Response of plants towards heavy metal toxicity: An overview of avoidance, tolerance, and uptake mechanism. Ann. Plant. Sci. 2, 362–368.
Danh, L. T., Truong, P., Mammucari, R., and Foster, N. (2014). A critical review of the arsenic uptake mechanisms and phytoremediation potential ofPteris vittata. Pteris Vittata. Int. J. Phytoremed. 16, 429–453. doi:10.1080/15226514.2013.798613
Dary, M., Chamber-Pérez, M., Palomares, A. J., and Pajuelo, E. (2010). “Insitu” phytostabilisation of heavy metal polluted soils using Lupinus luteus inoculated with metal-resistant plant-growth-promoting rhizobacteria. J. Hazard. Mat. 177, 323–330. doi:10.1016/j.jhazmat.2009.12.035
Delorme, T. A., Gagliardi, J. V., Angle, J. S., and Chaney, R. L. (2001). Influence of the zinc hyperaccumulator Thlaspi caerulescens J. & C. Presl. and the nonmetal accumulator Trifolium pratense L. on soil microbial populations. Can. J. Microbiol. 47, 773–776. doi:10.1139/w01-067
Desbrosses-Fonrouge, A-G., Voigt, K., Schröder, A., Arrivault, S., Thomine, S., and Krämer, U. (2005). Arabidopsis thaliana MTP1 is a Zn transporter in the vacuolar membrane which mediates Zn detoxification and drives leaf Zn accumulation. Febs. Lett. 579, 4165–4174. doi:10.1016/j.febslet.2005.06.046
Di, X., Beesley, L., Zhang, Z., Zhi, S., Jia, Y., and Ding, Y. (2019). Microbial arsenic methylation in soil and uptake and metabolism of methylated arsenic in plants: A review. Int. J. Environ. Res. Public Health 16, 5012. doi:10.3390/ijerph16245012
DiDonato, R. J., Roberts, L. A., Sanderson, T., Robynn Eisley, B., and Walker, E. L. (2004). Arabidopsis yellow stripe-like2 (YSL2): A metal-regulated gene encoding a plasma membrane transporter of nicotianamine-metal complexes. Plant J Cell. microbiol. 39 (3), 403–414. doi:10.1111/j.1365313X.2004.02128.x
Divol, F., Couch, D., Conéjéro, G., Roschzttardtz, H., Mari, S., and Curie, C. (2013). The Arabidopsis YELLOW STRIPE LIKE4 and 6 transporters control iron release from the chloroplast. Plant Cell. 25 (3), 1040–1055. doi:10.1105/tpc.112.107672
Dixit, P., Mukherjee, P. K., Ramachandran, V., and Eapen, S. (2011). Glutathione transferase from Trichoderma virens enhances cadmium tolerance without enhancing its accumulation in transgenic Nicotiana tabacum. PLoS One. 6 (1), e16360. doi:10.1371/journal.pone.0016360
Đurić, M., Oprčkal, P., Zalar, S. V., Pranjić, A. M., Ščančar, J., Milačič, R., et al. (2021). Environmental impacts and immobilization mechanisms of cadmium, lead, and zinc in geotechnical composites made from contaminated soil and paper-ash. Appl. Sci. 11, 11822. doi:10.3390/app112411822
Eapen, S., and D’souza, S. (2005). Prospects of genetic engineering of plants for phytoremediation of toxic metals. Biotechnol. Adv. 23, 97–114. doi:10.1016/j.biotechadv.2004.10.001
Estrella-Gómez, N., Mendoza-Cózatl, D., Moreno-Sánchez, R., González-Mendoza, D., Zapata-Pérez, O., Martínez-Hernández, A., et al. (2009). The Pb-hyperaccumulator aquatic fern Salvinia minima Baker, responds to Pb2+ by increasing phytochelatins via changes in SmPCS expression and phytochelatin synthase activity. Aquat. Toxicol. 91, 320–328. doi:10.1016/j.aquatox.2008.11.002
FAO/UN (2018). Report sounds alarm on soil pollution. https://www.fao.org/news/story/en/item/1126971/icode/ (Assessed 29 October 2022).
Fayiga, A. O., and Saha, U. K. (2016). Arsenic hyperaccumulating fern: Implications for remediation of arsenic contaminated soils. Geoderma. 284, 132–143. doi:10.1016/j.geoderma.2016.09.003
Flores-Cáceres, M. L., Hattab, S., Hattab, S., Boussetta, H., Banni, M., and Hernández, L. E. (2015). Specific mechanisms of tolerance to copper and cadmium are compromised by a limited concentration of glutathione in alfalfa plants. Plant Sci. 233, 165–173. doi:10.1016/j.plantsci.2015.01.013
Frérot, H., Hautekèete, N-C., Decombeix, I., Bouchet, M-H., Créach, A., Saumitou-Lapradeet, P., et al. (2018). Habitat heterogeneity in the pseudometallophyte Arabidopsis halleri and its structuring effect on natural variation of zinc and cadmium hyperaccumulation. Plant Soil. 423, 157–174. doi:10.1007/s11104-017-3509-1
Fuller, R., Landrigan, P. J., Balakrishnan, K., Bathan, G., Bose-O’Reilly, S., Brauer, M., et al. (2022). Pollution and health: A progress update. Lancet 6, E535–E547. doi:10.1016/s2542-5196(22)00090-0
Geng, H., Wang, F., Yan, C., Ma, S., Zhang, Y., Qin, Q., et al. (2022). Rhizosphere microbial community composition and survival strategies in oligotrophic and metal(loid) contaminated iron tailings areas. Hazard. Mat. 436, 129045. doi:10.1016/j.jhazmat.2022.129045
Ghori, Z., Iftikhar, H., Bhatti, M. F., Nasar-um-Minullah, Sharma, I., Kazi, A. G., et al. (2016). “Phytoextraction: The use of plants to remove heavy metals from the soil,” in Plant metal interaction: Emerging remediation techniques. Editor P. Ahmad (Amsterdam: Elsevier), 385–409.
Gianfreda, L. (2015). Enzymes of importance to rhizosphere processes. J. Soil Sci. Plant Nutr. 15, 0. doi:10.4067/S0718-95162015005000022
Gilman, E. F. (1990). Tree root growth and development. I. Form, spread, depth, and periodicity. J. Environ. Hort. 8, 215–220. doi:10.24266/0738-2898-8.4.215
Glick, B. R. (2010). Using soil bacteria to facilitate phytoremediation. Biotechnol. Adv. 28, 367–374. doi:10.1016/j.biotechadv.2010.02.001
Gong, L., Wang, J., Abbas, T., Zhang, Q., Cai, M., Tahir, M., et al. (2021). Immobilization of exchangeable Cd in soil using mixed amendment and its effect on soil microbial communities under paddy upland rotation system. Chemosphere 262, 127828. doi:10.1016/j.chemosphere.2020.127828
Gong, X., Yang, F., Pan, X., and Shao, J. F. (2022). Accumulation of silicon in shoots is required for reducing lead uptake in rice. Crop J. 262, 127828 doi:10.1016/j.cj.2022.09.014
Gremion, F., Chatzinotas, A., Kaufmann, K., von Sigler, W., and Harms, H. (2004). Impacts of heavy metal contamination and phytoremediation on a microbial community during a twelve-month microcosm experiment. FEMS. Microbiol. Ecol. 48 (2), 273–283. doi:10.1016/j.femsec.2004.02.004
Guerinot, M. L. (2000). The ZIP family of metal transporters. Biochimica Biophysica. Acta (BBA). – Biomemb. 1465, 190–198. doi:10.1016/S00052736(00)00138-3
Guerra, F. P., Gainza-Cortés, F. I., Pérez-Castro, R., and Zamudio, F. (2011). “Phytoremediation of heavy metals using poplars (Populus spp.): A glimpse of the plant responses to copper, cadmium and zinc stress,” in Handbook of phytoremediation (Chile: Nova Science Publishers Inc.), 387–414.
Gullap, M. K., Dasci, M., Erkovanİ., H., Koc, A., and Turan, M. (2014). Plant Growth-Promoting Rhizobacteria (PGPR) and phosphorus fertilizer-assisted phytoextraction of toxic heavy metals from contaminated soils. Commun. Soil Sci. Plant Anal. 45, 2593–2606. doi:10.1080/00103624.2014.929702
Gupta, A., Gopal, M., Thomas, G. V., Manikandan, V., Gajewski, J., Thomas, G., et al. (2014). Whole-genome sequencing and analysis of plant growth-promoting bacteria isolated from the rhizosphere of plantation crops coconut, cocoa, and arecanut. PLoS ONE. 9, e104259. doi:10.1371/journal.pone.0104259
Gutsch, A., Zouaghi, S., Renaut, J., Cuypers, A., Hausman, J. F., and Sergeant, K. (2018). Changes in the proteome of medicago sativa leaves in response to long-term cadmium exposure using a cell-wall targeted approach. Int. J. Mol. Sci. 19, 2498. doi:10.3390/ijms19092498
Haichar, F. E. Z., Santaella, C., Heulin, T., and Achouak, W. (2014). Root exudates mediated interactions belowground. Soil Biol. Biochem. 77, 69–80. doi:10.1016/j.soilbio.2014.06.017
Hall, J. (2002). Cellular mechanisms for heavy metal detoxification and tolerance. J. Exp. Bot. 53, 1–11. doi:10.1093/jexbot/53.366.1
Hanikenne, M., and Baurain, D. (2014). Origin and evolution of metal P-type ATPases in plantae (archaeplastida). Front. Plant Sci. 4, 544. doi:10.3389/fpls.2013.00544
Hanikenne, M., Talke, I. N., Haydon, M. J., Lanz, C., Nolte, A., Motte, P., et al. (2008). Evolution of metal hyperaccumulation required cis-regulatory changes and triplication of HMA4. Nature 453, 391–395. doi:10.1038/nature06877
Hardy, J. T. (2003). Climate change: Causes, effects, and solutions (1st ed.). New Jersey: Wiley, Chischester.
Hartmann, A., Schmid, M., van Tuinen, D., and Berg, G. (2009). Plant-driven selection of microbes. Plant Soil 321, 235–257. doi:10.1007/s11104-008-9814-y
Hasan, M. K., Cheng, Y., Kanwar, M. K., Chu, X.-Y., Ahammed, G. J., and Qi, Z.-Y. (2017). Responses of plant proteins to heavy metal stress—a review. Front. Plant Sci. 8, 1492. doi:10.3389/fpls.2017.01492
He, H., Ye, Z., Yang, D., Yan, J., Xiao, L., Zhong, T., et al. (2013). Characterization of endophytic Rahnella sp. JN6 from Polygonum pubescens and its potential in promoting growth and Cd, Pb, Zn uptake by Brassica napus. Chemosphere 90, 1960–1965. doi:10.1016/j.chemosphere.2012.10.057
He, Z., Yan, H., Chen, Y., Shen, H., Xu, W., Zhang, H., et al. (2016). An aquaporin PvTIP4;1 from Pteris vittata may mediate arsenite uptake. New Phytol. 209, 746–761. doi:10.1111/nph.13637
He, X., Xu, M., Wei, Q., Tang, M., Guan, L., Lou, L., et al. (2020). Promotion of growth and phytoextraction of cadmium and lead in Solanum nigrum L. mediated by plant-growth promoting rhizobacteria. Ecotoxicol. Enviro. Saf. 205, 111333. doi:10.1016/j.ecoenv.2020.111333
Henao, S. G., and Ghneim-Herrera, T. (2021). Heavy metals in soils and the remediation potential of bacteria associated with the plant microbiome. Front. Environ. Sci. 9. doi:10.3389/fenvs.2021.604216
Hou, D., Lin, Z., Wang, R., Ge, J., Wei, S., Xie, R., et al. (2018). Cadmium exposure-Sedum alfredii planting interactions shape the bacterial community in the hyperaccumulator plant rhizosphere. Appl. Environ. Microbiol. 84, e02797–17. doi:10.1128/AEM.02797-17
Hrynkiewicz, K., Dabrowska, G., Baum, C., Niedojadlo, K., and Leinweber, P. (2012). Interactive and single effects of ectomycorrhiza formation and Bacillus cereus on metallothionein MT1 expression and phytoextraction of Cd and Zn by willows. Water Air Soil Pollut. 223 (3), 957–968. doi:10.1007/s11270-011-0915-5
Hsieh, T. F., Ibarra, C. A., Silva, P., Zemach, A., Eshed-Williams, L., Fischer, R. L., et al. (2009). Genome-wide demethylation of Arabidopsis endosperm. Sci. 324, 1451–1454. doi:10.1126/science.1172417
Hu, L., Robert, C. A. M., Cadot, S., Zhang, X., Ye, M., Li, B., et al. (2018). Root exudate metabolites drive plant-soil feedbacks on growth and defense by shaping the rhizosphere microbiota. Nat. Comm. 9, 2738. doi:10.1038/s41467-018-05122-7
Hünninghaus, M., Dibbern, D., Kramer, S., Koller, R., Pausch, J., Schloter-Hai, B., et al. (2019). Disentangling carbon flow across microbial kingdoms in the rhizosphere of maize. Soil Biol. biochem. 134, 122–130. doi:10.1016/j.soilbio.2019.03.007
Hussain, D., Haydon, M. J., Wang, Y., Wong, E., Sherson, S. M., Young, J., et al. (2004). P-type ATPase heavy metal transporters with roles in essential zinc homeostasis in Arabidopsis. Plant Cell. 16 (5), 1327–1339. doi:10.1105/tpc.020487
Izquierdo, M., Tye, A. M., and Chenery, S. R. (2017). Using isotope dilution assays to understand speciation changes in Cd, Zn, Pb, and Fe in a soil model system under simulated flooding conditions. Geoderma 295, 41–52. doi:10.1016/j.geoderma.2017.02.006
Jacob, J. M., Karthik, C., Saratale, R. G., Kumar, S. S., Prabakar, D., Kadirvelu, K., et al. (2018). Biological approaches to tackle heavy metal pollution: A survey of literature. J. Environ. Manage. 217, 56–70. doi:10.1016/j.jenvman.2018.03.077
Ji, X., Abakumov, E., Chigray, S., Saparova, S., Polyakov, V., Wang, W., et al. (2021). Response of carbon and microbial properties to risk elements pollution in arctic soils. J. Hazard. Mat. 408, 124430. doi:10.1016/j.jhazmat.2020.124430
Jing, Y. D., He, Z. L., and Yang, X. E. (2007). Role of soil rhizobacteria in phytoremediation of heavy metal contaminated soils. J. Zhejiang Univ. Sci. B 8, 192–207. doi:10.1631/jzus.2007.B0192
Jing, Y. X., Yan, J. L., He, H. D., Yang, D. J., Xiao, L., Zhong, T., et al. (2014). Characterization of bacteria in the rhizosphere soils of Polygonum pubescens and their potential in promoting growth and Cd. Pb. Zn. uptake by Brassica Napus. Int. J. Phytol. 16, 321–333. doi:10.1080/15226514.2013.773283
Joseph, T., Dubey, B., and McBean, E. A. (2015). Human health risk assessment from arsenic exposures in Bangladesh. Sci. Total. Environ. 527, 552–560. doi:10.1016/j.scitotenv.2015.05.053
Karimi, N., and Souri, Z. (2015). Effect of phosphorus on arsenic accumulation and detoxification in arsenic hyperaccumulator, Isatis cappadocica. J. Plant. Growth Reg. 34, 88–95. doi:10.1007/s00344-014-9445-x
Karimi, N., Ghaderian, S. M., Raab, A., Feldmann, J., and Meharg, A. A. (2009). An arsenic-accumulating, hypertolerant brassica, Isatis capadocica. Isatis Cappadocica. New Phytol. 184, 41–47. doi:10.1111/j.14698137.2009.02982.x
Khalid, S., Shahid, M., Dumat, C., Niazi, N. K., Bibi, I., Bakhat, G. H. F. S., et al. (2017). Influence of ground-water and wastewater irrigation on lead accumulation in soil and vegetables: Implications for health risk assessment and phytoremediation. Int. J. Phytoremed. 19, 1037–1046. doi:10.1080/15226514.2017.1319330
Khan, S., Naushad, M., Lima, E. C., Zhang, S., Shaheen, S. M., and Rinklebe, J. (2021). Global soil pollution by toxic elements: Current status and future perspectives on the risk assessment and remediation strategies – a review. J. Hazard. Mater 417, 126039. doi:10.1016/j.jhazmat.2021.126039
Kicińska, A., Pomykałam, R., and Izquierdo-Diaz, M. (2021). Changes in soil pH and mobility of heavy metals in contaminated soils. Eur. J. Soil. Sci. 73, e13203. doi:10.1111/ejss.13203
Knabb, K. A., Erel, Y., Tirosh, O., Rittenour, T., Laparidou, S., Najjar, M., et al. (2016). Environmental impacts of ancient copper mining and metallurgy: Multi-proxy investigation of human-landscape dynamics in the Faynan valley, southern Jordan. J. Archaeol. Sci. 74, 85–101. doi:10.1016/j.jas.2016.09.003
Koptsik, G. N. (2014). Problems and prospects concerning the phytoremediation of heavy metal polluted soils: A review. Eurasian Soil Sci. 47, 923–939. doi:10.1134/S1064229314090075
Koul, B., and Taak, P. (2018). Biotechnological strategies for effective remediation of polluted soils. Springer. doi:10.1007/978-981-13-2420
Kranner, I., and Colville, L. (2011). Metals and seeds: Biochemical and molecular implications and their significance for seed germination. Environ. Exp. Bot. 7, 93–105. doi:10.1016/j.envexpbot.2010.05.005
Krzeszowska, E., Kokowska-Pawłowska, M., and Kandrzeszowski, S. (2021). Distribution of selected critical elements in the carboniferous coal-bearing series of the upper silesian and lublin coal basins (Poland). Acta. Geol. Sin. 96, 273–292. doi:10.1111/17556724.14811
Kumar, A., and Prasad, M. N. V. (2018). Plant-lead interactions: Transport, toxicity, tolerance, and detoxification mechanisms. Ecotoxicol. Environ. Saf. 166, 401–418. doi:10.1016/j.ecoenv.2018.09.113
Kumar, S., Dubey, R. S., Tripathi, R. D., Chakrabarty, D., and Trivedi, P. K. (2015). Omics and biotechnology of arsenic stress and detoxification in plants: Current updates and prospective. Environ. Int. 7, 221–230. doi:10.1016/j.envint.2014.10.019
Kumari, A., Kumar, R., shmi, R., and Jangra, R. (2018). Exploring phyllosphere bacteria for growth promotion and yield of potato (Solanum tuberosum L.). Int. J. Curr. Microbiol. Appl. Sci. 7 (4), 1065–1071. doi:10.20546/ijcmas.2018.704.117
Landrigan, P. J., Fuller, R., Acosta, N. J. R., Adeyi, O., Arnold, R., Basu, N., et al. (2018). The Lancet Commission on pollution and health. Lancet 39, 462–512. doi:10.1016/S0140-6736(17)32345-0
Lapie, C., Leglize, P., and Paris, C. (2019). Profiling of main metabolites in root exudates and mucilage collected from maize submitted to cadmium stress. Environ. Sci. Pollut. Res. 26, 17520–17534. doi:10.1007/s11356-019-05168-0
LeBlanc, M. S., McKinney, E. C., Meagher, R. B., and Smith, A. P. (2013). Hijacking membrane transporters for arsenic phytoextraction. J. Biotechnol. 163, 1–9. doi:10.1016/j.jbiotec.2012.10.013
Lee, S., and An, G. (2009). Over−expression of OsIRT1 leads to increased iron and zinc accumulations in rice. Plant Cell. Environ. 32, 408–416. doi:10.1111/j.1365-3040.2009.01935.x
Li, X., Sun, M., Zhang, L., Finlay, R. D., Liu, R., and Lian, B. (2022). Widespread bacterial responses and their mechanism of bacterial metallogenic detoxification under high concentrations of heavy metals. Environ. Saf. 246, 114193. doi:10.1016/j.ecoenv.2022.114193
Liao, Z., Chen, Y., Ma, J., Islam, M. S., Weng, L., and Li, Y. (2019). Cd, Cu, and Zn accumulations caused by long-term fertilization in greenhouse soils and their potential risk assessment. Int. J. Environ. Res. Public Health 16, 2805. doi:10.3390/ijerph16152805
Lima, L. W., Castleberry, M., Wangeline, A. L., Aguirre, B., Dall’Acqua, S., Pilon-Smits, E. A. H., et al. (2022). HyperaccumulatorStanleya pinnata: In situ fitness in relation to tissue selenium concentration. Plants 11, 690. doi:10.3390/plants11050690
Limmer, M., and Burken, J. (2016). Phytovolatilization of organic contaminants. Environ. Sci. Technol. 50, 6632–6643. doi:10.1021/acs.est.5b04113
Lin, Y-F., Hassan, Z., Talukdar, S., Schat, H., and Aarts, M. G. (2016). Expression of the ZNT1 zinc transporter from the metal hyperaccumulator Noccaea caerulescens confers enhanced zinc and cadmium tolerance and accumulation to Arabidopsis thaliana. PLoS ONE 11, e0149750. doi:10.1371/journal.pone.0149750
Liu, W., Shu, W., and Lan, C. (2004). Viola baoshanensis, a plant that hyperaccumulates cadmium. Chin. Sci. Bull. 49, 29–32. doi:10.1007/BF02901739
Liu, L., Li, W., Song, W., and Guo, M. (2018). Remediation techniques for heavy metal-contaminated soils: Principles and applicability. Sci. Total. Environ. 633, 206–219. doi:10.1016/j.scitotenv.2018.03.161
Liu, J., Zhao, L., Liu, Q., Li, J., Qiao, Z., Sun, P., et al. (2022). A critical review on soil washing during soil remediation for heavy metals and organic pollutants. Int. J. Environ. Sci. Technol. 19, 601–624. doi:10.1007/s13762-021-03144-1
Lodygin, E. D., Alekseev, I. I., Vasilevich, R. S., and Abakumov, E. V. (2020). Complexation of lead and cadmium ions with humic acids from arctic peat soils. Environ. Res. 191, 110058. doi:10.1016/j.envres.2020.110058
Lombi, E., Tearall, K. L., Howarth, J. R., Zhao, F. J., Hawkesford, M. J., and McGrath, S. P. (2002). Influence of iron status on cadmium and zinc uptake by different ecotypes of the hyperaccumulator Thlaspi caerulescens . Thlaspi Caerulescens. Plant Physiol. 128, 1359–1367. doi:10.1104/pp.010731
Lu, M., Huang, L., Wang, Q., Cao, X., Lin, Q., He, Z., et al. (2023). Soil properties drive the bacterial community to cadmium contamination in the rhizosphere of two contrasting wheat (Triticum aestivum L.) genotypes. J. Environ. Sci. 128, 117–128. doi:10.1016/j.jes.2022.07.028
Luo, J-S., and Zhang, Z. (2021). Mechanisms of cadmium phytoremediation and detoxification in plants. Crop J 9, 521–529. doi:10.1016/j.cj.2021.02.001
Ma, L., Komar, K., Tu, C., Zhang, W., Cai, Y., and Kennelley, E. D. (2001). A fern that hyperaccumulates arsenic. Nature 409, 579. doi:10.1038/35054664
Ma, L., Li, J., Zhan, Z., Chen, L., Li, D., Bai, Q., et al. (2016). Specific histone modification responds to arsenic-induced oxidative stress. Toxicol. Appl. Pharmacol. 302, 52–61. doi:10.1016/j.taap.2016.03.015
Małecka, A., Konkolewsk, A., Hanć, A., Barałkiewicz, D., Ciszewska, L., Ratajczak, E., et al. (2019). Insight into the phytoremediation capability of Brassica juncea (v. Malopolska): Metal accumulation and antioxidant enzyme activity. Int. J. Mol. Sci. 20, 4355. doi:10.3390/ijms20184355
Matec Industries (2021). Soil washing – remediation of contaminated sites. https://www.matecindustries.com/en/soil-washing-remediation-of-contaminated-sites/ ([Accessed 21 February 2023].
Memon, A. R., and Schröder, P. (2009). Implications of metal accumulation mechanisms to phytoremediation. Environ. Sci. Pollut. Res. Int. 16, 162–175. doi:10.1007/s11356-008-0079-z
Mertens, J., and Smolders, E. (2013). “Zinc,” in Heavy metals in soils. Environmental pollution. Editor B. Alloway (Dordrecht: Springer), 22. doi:10.1007/978-94-007-4470-7_17
Mesjasz-Przybyłowicz, J., Nakonieczny, M., Migula, P., Augustyniak, M., Tarnawska, M., Reimold, W. U., et al. (2004). Uptake of Cadmium, Lead, Nickel, and Zinc from soil and water solutions by the nickel hyperaccumulator. Berkheya Coddii. Acta Biol. Cracoviensia Ser. Bot. 46, 75–85.
Milner, M. J., Mitani-Ueno, N., Yamaji, N., Yokosho, K., Craft, E., Fei, Z., et al. (2014). Root and shoot transcriptome analysis of two ecotypes of Noccaea caerulescens uncovers the role of NcNramp1 in Cd hyperaccumulation. Plant J. 78, 398–410. doi:10.1111/tpj.12480
Mishra, S., Mishra, A., and Küpper, H. (2017). Protein biochemistry and expression regulation of cadmium/zinc pumping ATPases in the hyperaccumulator plantsArabidopsis halleri and. Noccaea Caerulescens. Front. Plant. Sci. 8, 835. doi:10.3389/fpls.2017.00835
Miyadate, H., Adachi, S., Hiraizumi, A., Tezuka, K., Nakazawa, N., Kawamoto, T., et al. (2010). OsHMA3, a P1B-type of ATPase affects root-to-shoot cadmium translocation in rice by mediating efflux into vacuoles. New Phytol. 189, 190–199. doi:10.1111/j.1469-8137.2010.03459.x
Mleczek, M., Gąsecka, M., Waliszewska, B., Magdziak, Z., Szostek, M., Rutkowski, P., et al. (2018). Salix viminalis L. - a highly effective plant in phytoextraction of elements. Chemosphere 212, 67–78. doi:10.1016/j.chemosphere.2018.08.055
Mohiley, A., Laaser, T., Höreth, S., Clemens, S., Tielbörger, K., and Gruntman, M. (2021). Between the devil and the deep blue sea: Herbivory induces foraging for and uptake of cadmium in a metal hyperaccumulating plant. Proc. R. Soc. B 288, 20211682. doi:10.1098/rspb.2021.1682
Mondillo, N., Wilkinson, J. J., Boni, M., Weiss, D. J., and Mathur, R. (2018). A global assessment of Zn isotope fractionation in secondary Zn minerals from sulfide and non-sulfide ore deposits and model for fractionation control. Chem. Geol. 500, 182–193. doi:10.1016/j.chemgeo.2018.09.033
Morel, M., Crouzet, J., Gravot, A., Auroy, P., Leonhardt, N., Vavasseur, A., et al. (2009). AtHMA3, a P1B-ATPase allowing Cd/Zn/Co/Pb vacuolar storage in Arabidopsis. Plant Physiol. 149, 894–904. doi:10.1104/pp.108.130294
Mulero-Aparicio, A., Cernava, T., Turrà, D., Schaefer, A., Di Pietro, A., López-Escudero, F. J., et al. (2019). The role of volatile organic compounds and rhizosphere competence in mode of action of the non-pathogenic Fusarium oxysporum FO12 toward Verticillium wilt. Front. Microbiol. 10, 1808. doi:10.3389/fmicb.2019.01808
National Academy of Sciences (2020). Climate change: Evidence and causes: Update 2020. Washington, DC: The National Academies Press, 7. doi:10.17226/25733
Navarrete, F., and De La Fuente, L. (2015). Zinc detoxification is required for full virulence and modification of the host leaf ionome by Xylella fastidiosa. Mol. Plant Microbe. Interact. 28, 497–507. doi:10.1094/MPMI-07-14-0221-R
Parvin, S., Haque, M. E., Akhter, F., Ali, M., and Shafin, M. S. (2021). Determination of arsenic in minor cereals (barley, foxtail millet, proso-millet, finger- millet, pearl -millet, buckwheat. Oat, quinoa, and sorghum) in gazipur. Biomed. J. Sci. Tech. Res. 40, 32251–32253.
Pineau, C., Loubet, S., Lefoulon, C., Chalies, C., Fizames, C., Lacombe, B., et al. (2012). Natural variation at the FRD3 MATE transporter locus reveals cross-talk between Fe homeostasis and Zn tolerance in Arabidopsis thaliana. PLoS Gene. 8, e1003120. doi:10.1371/journal.pgen.1003120
Punamiya, P., Datta, R., Sarkar, D., Barber, S., Patel, M., and Das, P. (2010). Symbiotic role of Glomus mosseae in phytoextraction of lead in vetiver grass [Chrysopogon zizanioides (L.)]. J. Hazard Mat. 177, 465–474. doi:10.1016/j.jhazmat.2009.12.056
Qiao, S. Y., Tao, Y., Shan, Q. H., Wang, J. G., Chai, T. Y., Gong, S. F., et al. (2021). Physiological and gene expression responses of six annual ryegrass cultivars to cobalt, lead, and nickel stresses. Int. J. Mol. Sci. 22, 13583. doi:10.3390/ijms222413583
Rai, V. (2002). Role of amino acids in plant responses to stresses. Biol. Plant 45, 481–487. doi:10.1023/A:1022308229759
Raza, W., Yousaf, S., and Rajer, F. U. (2016). Plant growth-promoting activity of volatile organic compounds produced by Bio-control strains. Sci. Lett. 4, 40–43.
Reeves, R. D., and Baker, A. J. M. (2000). ““Metal accumulating plants”,” in Phytoremediation of toxic metals: Using plants to clean up the environment. Editors I. Raskin, and B. D. Finsley (New York: Wiley), 193–229.
Ricachenevsky, F. K., Menguer, P. K., Sperotto, R. A., Williams, L. E., and Fett, J. P. (2013). Roles of plant metal tolerance proteins (MTP) in metal storage and potential use in biofortification strategies. Front. Plant. Sci. 144, 144. doi:10.3389/fpls.2013.00144
Ricachenevsky, F. K., Punshon, T., Salt, D. E., Fett, J. P., and Guerinot, M. L. (2021). Arabidopsis thaliana zinc accumulation in leaf trichomes is correlated with zinc concentration in leaves. Sci. Rep. 11, 5278. doi:10.1038/s41598-021-84508-y
Roy, S. B., and Bera, A. (2002). Individual and combined effect of mercury and manganese on phenol and proline content in leaf and stem of mungbean seedlings. J. Environ. Biol. 23, 433–435.
Sagardoy, R., Morales, F. E., Rellán-Álvarez, R., Abadía, A., Abadía, J., and López-Millán, A. (2010). Carboxylate metabolism in sugar beet plants grown with excess Zn. J. Plant Physiol. 168, 730–733. doi:10.1016/j.jplph.2010.10.012
Salman, S. A., El-Anwar, E. A. A., Asmoay, A., Mekky, H., Ibrahem, W. A., and Elnazer, A. (2021). Chemical fractionation and risk assessment of some heavy metals in soils, assiut governorate, Egypt. Egypt. J. Chem.64. doi:10.21608/ejchem.2021.59371.3276
Sarkar, B., Mukhopadhyay, R., Ramanayaka, S., Bolan, N., and Ok, Y. S. (2021). The role of soils in the disposition, sequestration, and decontamination of environmental contaminants. Phil. Trans. R. Soc. B 376, 20200177. doi:10.1098/rstb.2020.0177
Sasse, J., Martinoia, E., and Northern, T. (2018). Feed your friends: Do plant exudates shape the root microbiome? Trends Plant Sci. 23, 25–41. doi:10.1016/j.tplants.2017.09.003
Schreiter, S. S., Babin, D. D., Smalla, K. K., and Grosch, R. R. (2018). Rhizosphere competence and biocontrol effect of Pseudomonas sp. RU47 independent from plant species and soil type at the field scale. Front. Microbiol. 9, 97. doi:10.3389/fmicb.2018.00097
Sessitsch, A., Kuffner, M., Kidd, P., Vangronsveld, J., Wenzel, W. W., Fallmann, K., et al. (2013). The role of plant-associated bacteria in the mobilization and phytoextraction of trace elements in contaminated soils. Soil Biol. Biochem. 60, 182–194. doi:10.1016/j.soilbio.2013.01.012
Shahid, M., Dumat, C., Pourrut, B., Silvestre, J., Laplanche, C., and Pinelli, E. (2014). Influence of EDTA and citric acid on lead-induced oxidative stress toVicia faba roots. J. Soils Sed. 14, 835–843. doi:10.1007/s11368-013-0724-0
Shahzad, Z., Gosti, F., Frérot, H., Lacombe, E., Roosens, N., Saumitou-Laprade, P., et al. (2010). The five AhMTP1 zinc transporters undergo different evolutionary fates towards adaptive evolution to zinc tolerance in Arabidopsis halleri. PLoS Genet. 6, e1000911. doi:10.1371/journal.pgen.1000911
Shanmugam, V., Lo, J-C., and Yeh, K-C. (2013). Control of Zn uptake in Arabidopsis halleri: A balance between Zn and Fe. Front. Plant Sci. 14, 281. doi:10.3389/fpls.2013.00281
Sharma, R. K., and Archana, G. (2016). Cadmium minimization in food crops by cadmium resistant plant growth promoting rhizobacteria. Appl. Soil Ecol. 107, 66–78. doi:10.1016/j.apsoil.2016.05.009
Sharma, A., Patni, B., Shankhdhar, D., and Shankhdhar, S. C. (2013). Zinc – An indispensable micronutrient. Physiol. Mol. Biol. Plants. 19, 11–20. doi:10.1007/s12298-012-0139-1
Shen, Z., Zhang, J., Hou, D., Tsang, D. W. C., Ok, Y. S., and Alessi, D. S. (2019). Synthesis of MgO-coated corncob biochar and its application in lead stabilization in a soil washing residue. Environ. Int. 122, 357–362. doi:10.1016/j.envint.2018.11.045
Shin, J., Natanson, A., Khun, J., Odorizzi, N., DeCreny-Jackson, J., Fowowe, H., et al. (2017). Research Article: Assessing the impact of coal ash exposure on soil microbes in the Dan River. BIOS. J. 88, 72–85. doi:10.1893/bios-d-16-00006.1
Shirazi, Z., Abedi, A., Kordrostami, M., Burritt, D. J., and Hossain, M. A. (2019). Genome-wide identification and characterization of the metal tolerance protein (MTP) family in grape (Vitis vinifera L.). 3 Biotech. 9, 199. doi:10.1007/s13205-019-1728-2
Shrivastava, A., Ghosh, D., Dash, A., and Bose, S. (2015). Arsenic contamination in soil and sediment in India: Sources, effects, and remediation. Curr. Pollut. Rep. 1, 35–46. doi:10.1007/s40726-015-0004-2
Sinclair, S. A., and Krämer, U. (2012). The zinc homeostasis network of land plants. Biochim. Biophys. Acta. 1823, 1553–1567. doi:10.1016/j.bbamcr.2012.05.016
Singh, S., Parihar, P., Singh, R., Singh, V. P., and Prasad, S. M. (2016). Heavy metal tolerance in plants: Role of transcriptomics, proteomics, metabolomics, and ionomics. Front. Plant Sci. 6, 1143. doi:10.3389/fpls.2015.01143
Singh, P., Chauhan, P. K., Upadhyay, S. K., Singh, R. K., Dwivedi, P., Wang, J., et al. (2022). Mechanistic insights and potential use of siderophores producing microbes in rhizosphere for mitigation of stress in plants grown in degraded land. Front. Microbiol. 13, 898979. doi:10.3389/fmicb.2022.898979
Socha, A. L., and Guerinot, M. L. (2014). Mn-euvering manganese: The role of transporter gene family members in manganese uptake and mobilization in plants. Front. Plant Sci. 5, 106. doi:10.3389/fpls.2014.00106
Song, W. Y., Park, J., Mendoza-Cózatl, D. G., Suter-Grotemeyer, M., Shim, D., Hörtensteiner, S., et al. (2010). Arsenic tolerance in Arabidopsis is mediated by two ABCC-type phytochelatin transporters. Proc. Natl. Acad. Sci. U. S. A. 107, 21187–21192. doi:10.1073/pnas.1013964107
Souri, Z., Karimi, N., and Sandalio, L. M. (2017). Arsenic hyperaccumulation strategies: An overview. Front. Cell. Dev. Biol. 5, 67. doi:10.3389/fcell.2017.00067
Sridhar, B. B. M., Han, F. X., Diehl, S. V., Monts, D. L., and Su, Y. (2011). Effect of phytoaccumulation of arsenic and chromium on structural and ultrastructural changes of brake fern (Pteris vittata). Bra. J. Plant. Physiol. 23, 285–293. doi:10.1590/S1677-04202011000400006
Su, Y. H., McGrath, S. P., Zhu, Y. G., and Zhao, F. J. (2008). Highly efficient xylem transport of arsenite in the arsenic hyperaccumulator Pteris vittata. New Phytol. 180, 434–441. doi:10.1111/j.14698137.2008.02584.x
Suman, J., Uhlik, O., Viktorova, J., and Macek, T. (2018). Phytoextraction of heavy metals: A promising tool for clean-up of polluted environment? Front. Plant Sci. 9, 1476. doi:10.3389/fpls.2018.01476
Sungur, A., Kavdir, Y., Özcan, H., İlay, R., and Soylak, M. (2020). Geochemical fractions of trace metals in surface and core sections of aggregates in agricultural soils. Catena 197, 104995. doi:10.1016/j.catena.2020.104995
Takahashi, R., Bashir, K., Ishimaru, Y., Nishizawa, N. K., and Nakanishi, H. (2012). The role of heavy-metal ATPases, HMAs, in zinc and cadmium transport in rice. Plant Signal Behav. 7, 1605–1607. doi:10.4161/psb.22454
Tao, J., and Lu, L. (2022). Advances in genes-encoding transporters for cadmium uptake, translocation, and accumulation in plants. Toxics 10, 411. doi:10.3390/toxics10080411
Thakur, S., Singh, L., Wahid, Z. A., Siddiqui, M. F., Atnaw, S. M., and Din, M. F. M. (2016). Plant-driven removal of heavy metals from soil: Uptake, translocation, tolerance mechanism, challenges, and future perspectives. Environ. Monit. Assess. 188, 206. doi:10.1007/s10661-016-5211-9
Thounaojam, T. C., Khan, Z., Meetei, T. T., Srivastava, S., Panda, K. S., and Upadhyaya, H. (2021). Transporters: The molecular drivers of arsenic stress tolerance in plants. J. Plant Biochem. Biotechnol. 30, 730–743. doi:10.1007/s13562-021-00748-z
Tiwari, G., Duraivadivel, P., Sharma, S., and Hariprasad, P. (2018). 1-Aminocyclopropane-1-carboxylic acid deaminase producing beneficial rhizobacteria ameliorate the biomass characters of Panicum maximum Jacq. by mitigating drought and salt stress. Sci. Rep. 8, 17513. doi:10.1038/s41598-018-35565-3
Tong, Y-P., Kneer, R., and Zhu, Y-G. (2004). Vacuolar compartmentalization: A second-generation approach to engineering plants for phytoremediation. Trends Plant Sci. 9, 7–9. doi:10.1016/j.tplants.2003.11.009
Van de Mortel, J. E., Villanueva, L. A., Schat, H., Kwekkeboom, J., Coughlan, S., Moerland, P. D., et al. (2006). Large expression differences in genes for iron and zinc homeostasis, stress response, and lignin biosynthesis distinguish roots of Arabidopsis thaliana and the related metal hyperaccumulator. Thlaspi Caerulescens. Plant Physiol. 142, 1127–1147. doi:10.1104/pp.106.082073
Vatamaniuk, O. K., Mari, S., Lu, Y. P., and Rea, P. A. (2000). Mechanism of heavy metal ion activation of phytochelatin (PC) synthase: Blocked thiols are sufficient for PC synthase catalyzed transpeptidation of glutathione and related thiol peptides. J. Biol. Chem. 275, 31451–31459. doi:10.1074/jbc.m002997200
Verret, F., Gravot, A., Auroy, P., Leonhardt, N., David, P., Nussaume, L., et al. (2004). Overexpression of AtHMA4 enhances root−to−shoot translocation of zinc and cadmium and plant metal tolerance. Febs. Lett. 576, 306–312. doi:10.1016/j.febslet.2004.09.023
Visioli, G., D'Egidio, S., and Sanangelantoni, A. M. (2015). The bacterial rhizobiome of hyperaccumulators: Future perspectives based on omics analysis and advanced microscopy. Front. Plant Sci. 5, 752. doi:10.3389/fpls.2014.00752
Visoottivisetha, P., Francesconib, K., and Sridokchan, W. (2002). The potential of Thai indigenous plant species for the phytoremediation of arsenic-contaminated land. Environ. Pollut. 118, 453–461. doi:10.1016/s0269-7491(01)00293-7
Vogel-Mikuš, K., Pongrac, P., Kump, P., Nečemer, M., and Regvar, M. (2006). Colonisation of a Zn, Cd and Pb hyperaccumulator Thlaspi praecox Wulfen with indigenous arbuscular mycorrhizal fungal mixture induces changes in heavy metal and nutrient uptake. Environ. Pollut. 139, 362–371. doi:10.1016/j.envpol.2005.05.005
Wang, S., and Mulligan, C. N. (2006). Effect of natural organic matter on arsenic release from soils and sediments into groundwater. Environ. Geochem. Health 28, 197–214. doi:10.1007/s10653-005-9032-y
Wang, S. L., Liao, W., Yu, F. Q., Liao, B., and Shu, W. S. (2009). Hyperaccumulation of lead, zinc, and cadmium in plants growing on a lead/zinc outcrop in Yunnan Province, China. Environ. Earth Sci. 58, 471–476. doi:10.1007/s00254-008-1519-2
Wang, X., Tam, N. F-Y., He, H., and Ye, Z. (2015). The role of root anatomy, organic acids, and iron plaque on mercury accumulation in rice. Plant Soil. 394, 301–313. doi:10.1007/s11104-015-2537-y
Whiting, S. N., de Souza, M. P., and Terry, N. (2001). Rhizosphere bacteria mobilize Zn for hyperaccumulation by Thlaspi caerulescens. Environ. Sci. Technol. 35, 3144–3150. doi:10.1021/es001938v
Wiggenhauser, M., Aucour, A. M., Bureau, S., Campillo, S., Telouk, P., Romani, M., et al. (2021). Cadmium transfer in contaminated soil-rice systems: Insights from solid-state speciation analysis and stable isotope fractionation. Environ. Pollut. 26, 115934. doi:10.1016/j.envpol.2020.115934
Wilkins, K. A., Matthus, E., Swarbreck, M. S., and Davies, J. M. (2016). Calcium-mediated abiotic stress signaling in roots. Front. Plant Sci. 7, 1296. doi:10.3389/fpls.2016.01296
Williams, L. E., and Mills, R. F. (2005). P1B ATPases—An ancient family of transition metal pumps with diverse functions in plants. Trends Plant Sci. 10, 491–502. doi:10.1016/j.tplants.2005.08.008
Wioleta, W., Anna, D., Ilona, B., Kamila, K., and Elżbieta, R. (2015). Lead-induced changes in phosphorylation of PSII proteins in low-light grown pea plants. Biometals 28, 151–162. doi:10.1007/s10534-014-9811-y
Wojcik, M., and Tukiendorf, A. (2005). Cadmium uptake, localization and detoxification in Zea mays. Zea Mays. Biol. Plant 49, 237–245. doi:10.1007/s10535-005-7245-7
Woldetsadik, D., Drechsel, P., Keraita, B., Itanna, F., and Gebrekidan, H. (2017). Heavy metal accumulation, and health risk assessment in wastewater-irrigated urban vegetable farming sites of Addis Ababa, Ethiopia. Int. J. Food. Contam. 4, 9. doi:10.1186/s40550-017-0053-y
World Health Organization (1996). Permissible limits of heavy metals in soil and plants. Geneva, Switzerland: WHO.
Wu, C., Liao, B., Wang, S-L., Zhang, J., and Li, J-T. (2010). Pb and Zn accumulation in a Cd-hyperaccumulator (Viola baoshanensis). Int. J. Phytol. 12, 574–585. doi:10.1080/15226510903353195
Wu, C., Shi, L., Xue, S., Li, W., Jiang, X., Rajendran, M., et al. (2019). Effect of sulfur-iron modified biochar on the available cadmium and bacterial community structure in contaminated soils. Sci. Total Environ. 647, 1158–1168. doi:10.1016/j.scitotenv.2018.08.087
Xiong, Q., Hu, J., Wei, H., Zhang, H., and Zhu, J. (2021). Relationship between plant roots, rhizosphere microorganisms, and nitrogen and its special focus on rice. Agric. 11, 234. doi:10.3390/agriculture11030234
Xu, W., Dai, W., Yan, H., Li, S., Shen, H., Chen, Y., et al. (2015). Arabidopsis NIP3;1 plays an important role in arsenic uptake and root-to-shoot translocation under arsenite stress conditions. Mol. Plant 8, 722–733. doi:10.1016/j.molp.2015.01.005
Yamaji, N., Sasaki, A., Xia, J., Yokosho, K., and Ma, J. F. (2013). A node-based switch for preferential distribution of manganese in rice. Nat. Commun. 4, 2442. doi:10.1038/ncomms3442
Yan, A., Wang, Y., Tan, S. N., Yusof, M. L., Ghosh, S., and Chen, Z. (2020). Phytoremediation: A promising approach for revegetation of heavy metal-polluted land. Front. Plant Sci. 11, 359. doi:10.3389/fpls.2020.00359Phytoremediation
Yang, Q., Tu, S., Wang, G., Liao, X., and Yan, X. (2012). Effectiveness of applying arsenate-reducing bacteria to enhance arsenic removal from polluted soils by Pteris vittata L. Int. J. Phytoremed. 14, 89–99. doi:10.1080/15226510903567471
Yuan, L., Yang, S., Liu, B., Zhang, M., and Wu, K. (2012). Molecular characterization of a rice metal tolerance protein, OsMTP1. Plant Cell. Rep. 31, 67–79. doi:10.1007/s00299-011-1140-9
Zemanová, V., Pavlíková, D., Hnilička, F., and Pavlík, M. (2021). Arsenic toxicity-induced physiological and metabolic changes in the shoots of Pteris cretica and spinacia oleracea. Plants 10, 2009. doi:10.3390/plants10102009
Zeng, X., Xu, H., Lu, J., Li, W., Wu, L., Tang, J., et al. (2020). The immobilization of soil cadmium by the combined amendment of bacteria and hydroxyapatite. Rep 10, 2189. doi:10.1038/s41598-020-58259-1
Zhalnina, K., Zengler, K., Newman, D., and Northern, T. R. (2018). Need for laboratory ecosystems to unravel the structures and functions of soil microbial communities mediated by chemistry. mBio 9, 011755–e1218. doi:10.1128/mBio.01175-18
Zhang, Y., Cougnon, F. B. L., Wanniarachchi, Y. A., Hayden, J. A., and Nolan, E. M. (2013). Reduction of human defensin 5 affords a high-affinity zinc chelating peptide. ACS Chem. Biol. 8, 1907–1911. doi:10.1021/cb400340k
Zhang, J., Martinoia, E., and Lee, Y. (2018). Vacuolar transporters for cadmium and arsenic in plants and their applications in phytoremediation and crop development. Plant Cell. Physiol. 59, 1317–1325. doi:10.1093/pcp/pcy006
Zhang, X., Kuzyakov, Y., Zang, H., Michaela, D. A., Lingling, S., Spielvogel, S., et al. (2020). Rhizosphere hotspots: Root hairs and warming control microbial efficiency, carbon utilization, and energy production. Soil Biol. biochem. 148, 107872. doi:10.1016/j.soilbio.2020.107872
Zhang, J., Chen, J., Wu, Y-F., Wang, Z. P., Qiu, J. G., Li, X. L., et al. (2021). Oxidation of organoarsenicals and antimonite by a novel flavin monooxygenase widely present in soil bacteria. Environ. Microbiol. 24, 752–761. doi:10.1111/1462-2920.15488
Zhao, F. J., Ma, J. F., Meharg, A. A., and McGrath, S. P. (2008). Arsenic uptake and metabolism in plants. New Phytol. 181, 777–794. doi:10.1111/j.14698137.2008.02716.x
Zhu, L-J., Guan, D. X., Luo, J., Rathinasabapathi, B., and Ma, L. Q. (2014). Characterization of arsenic-resistant endophytic bacteria from hyperaccumulators Pteris vittata and. Pteris Multifida. Chemosphere 113, 9–16. doi:10.1016/j.chemosphere.2014.03.081
Keywords: above-ground biomass, hyperaccumulators, microbes, phytoremediation, transporter protein
Citation: Asare MO, Száková J and Tlustoš P (2023) Mechanisms of As, Cd, Pb, and Zn hyperaccumulation by plants and their effects on soil microbiome in the rhizosphere. Front. Environ. Sci. 11:1157415. doi: 10.3389/fenvs.2023.1157415
Received: 02 February 2023; Accepted: 06 April 2023;
Published: 17 April 2023.
Edited by:
Prafulla Kumar Sahoo, Central University of Punjab, IndiaReviewed by:
Rakesh Kumar, Independent Researcher, Rajgir, IndiaTofan Kumar Rout, Central Drug Research Institute (CSIR), India
Saloni Sachdeva, Jaypee Institute of Information Technology, India
Copyright © 2023 Asare, Száková and Tlustoš. This is an open-access article distributed under the terms of the Creative Commons Attribution License (CC BY). The use, distribution or reproduction in other forums is permitted, provided the original author(s) and the copyright owner(s) are credited and that the original publication in this journal is cited, in accordance with accepted academic practice. No use, distribution or reproduction is permitted which does not comply with these terms.
*Correspondence: Michael O. Asare, YXNhcmVAZnpwLmN6dS5jeg==