- 1Soil Biodiversity and Ecosystem Function Lab, Department of Biology, University of Western Ontario, London, ON, Canada
- 2Plant and Soil Ecology Lab, Department of Biology, Algoma University, Sault Ste. Marie, ON, Canada
- 3Laboratório de Sistemática de Collembola e Conservação, Coleção de Referência de Fauna de Solo, Instituto de Biologia de Solo, Universidade Estadual da Paraíba, Campus V, João Pessoa, Brazil
- 4Northern Research Station, US Department of Agriculture Forest Service, Grand Rapids, MN, United States
- 5Oak Ridge National Laboratory, US Department of Energy, Oak Ridge, TN, United States
Boreal peatlands are important ecosystems for carbon cycling because they store 1/3 of the world’s terrestrial carbon in only ∼3% of the global landmass. This high carbon storage capacity makes them a key potential mitigation strategy for increased carbon emissions induced by global climate warming. In high-carbon storage systems like peatlands, soil faunal communities are responsible for secondary decomposition of organic matter and nutrient cycling, which suggests they play an important role in the carbon cycle. Experiments have shown that warming can affect plant and microbial communities in ways that potentially shift peatlands from carbon sinks to sources. Although previous studies have found variable effects of climate change manipulations on soil communities, warming is expected to affect soil community composition mainly through reductions in moisture content, whereas elevated CO2 atmospheric concentrations are expected to only indirectly and weakly do so. In this study we used a large-scale peatland field-based experiment to test how soil microarthropod (oribatid and mesostigmatid mite, and collembolan species abundance, richness and community composition) respond to a range of experimental warming temperatures (between 0°C and +9°C) crossed with elevated CO2 conditions over 4 years in the Spruce and Peatland Responses Under Changing Environments (SPRUCE) experiment. Here we found that warming significantly decreased surface peat moisture, which in turn decreased species microarthropod richness and abundance. Specifically, oribatid and mesostigmatid mite, collembolan, and overall microarthropod richness significantly decreased under lower moisture levels. Also, the abundance of microarthropods increased under higher moisture levels. Neither warming nor elevated [CO2] affected microarthropods when analysed together or separate, except for the richness of mesostigmatids that significantly increased under warming. At the community level, communities varied significantly over time (except collembolans), and moisture was an important driver explaining community species composition. While we expect that the cumulative and interactive effects of the SPRUCE experimental treatments on soil faunal biodiversity will continue to emerge, our results already suggest effects are becoming more observable over time. Taken together, the changes belowground indicate potential changes on carbon and nitrogen cycles, as microarthropods are important players of soil food webs.
1 Introduction
Soil biodiversity accounts for an estimate ¼ of all life on Earth and performs important ecosystem-level processes such as decomposition and nutrient cycling that facilitate many ecosystem services such as carbon transformations and storage that help regulate climate (Filser et al., 2016; FAO, 2020). At the same time, there is increasing concern that multiple anthropogenic stressors, including climate warming, are impacting soil biodiversity and function (Geisen et al., 2019; Yang et al., 2022). Global temperatures at northern latitudes are expected to increase between 2°C and 8°C over the next century (IPCC, 2018) alongside concomitant increases in atmospheric CO2 concentrations. Elevated atmospheric CO2 may not have direct effects on soil biodiversity, but can enhance aboveground productivity (Ainsworth and Long, 2005; but see Leuzinger and Hättenschwiler, 2013 for full discussion) leading indirectly to enhanced belowground biomass and microbial activity from increased root exudation (Fenner et al., 2007; Nie et al., 2015). Warming will directly increase metabolic rates of soil organisms, potentially increasing population growth rates and altering community composition that could increase decomposition and nutrient cycling rates (Campbell et al., 2009; Stuble et al., 2019); thus, both warming and elevated CO2 could accelerate decomposition rates and diminish the carbon storage potential of soils. At the same time, warming also indirectly affects soil systems through changes in soil moisture, alongside shifts in precipitation regimes and increased variability of annual precipitation events forecast under climate change. As such it is important to understand how multiple climate change factors will affect soil biodiversity and function (Rillig et al., 2019; Peng et al., 2022).
Previous studies of climate change (warming, elevated CO2, and changes in soil moisture conditions) on belowground communities have yielded mixed results that depend on the taxonomic group examined (Markkula et al., 2019; Meehan et al., 2020; Barreto and Lindo, 2022), the interaction between climate change factors (Kardol et al., 2011; Haimi et al., 2015; Lindo, 2015; Turnbull and Lindo, 2015; Peng et al., 2022), and other context specific environmental variables (Blankinship et al., 2011; Holmstrup et al., 2017; Meehan et al., 2020; Barreto et al., 2021). Both the direct and interacting effects of climate change are difficult to observe at ecosystem-level scales, especially given that changes in soil fauna communities may occur at fine-scale taxonomic resolution (i.e., genus or species-level), and may only emerge over multiple year time scales. Much of the information about the response of soil biodiversity to multiple climate change factors is known from grasslands or agricultural areas (e.g., O’Lear and Blair, 1999; Kardol et al., 2011; Yin et al., 2020) and forest systems (Meehan et al., 2020), with only a recent focus on northern peatlands, despite being high carbon storage systems (but see Barreto et al., 2021).
Peatland biodiversity, both above- and belowground, often contains unique species adapted to very moist, low nutrient, highly acidic environments (Rydin and Jeglum, 2006; Minayeva et al., 2017; Barreto and Lindo, 2021). Although covering a relatively small fraction of the Earth’s area, peatlands are globally important carbon stores (Frolking et al., 2011; Beaulne et al., 2021) that contain at least 550 Gt of carbon in their peat (i.e., partially decomposed plant matter) (Hugelius et al., 2020), which constitutes about 1/3 of the world’s terrestrial C (Limpens et al., 2008). At the same time, these deep accumulations of organic soil horizons provide habitat and food resources for a myriad of soil organisms; among which dominant groups include oribatid mites (Acari: Oribatida), collembolans (Entognatha: Collembola), and mesostigmatid mites (Acari: Mesostigmata). Oribatid mites are commonly the most diverse group of arthropods in terrestrial soils and most species are particle-feeding saprophages and mycophages (Behan-Pelletier and Lindo, 2023). Collembolans are soil and litter dwelling organisms that feed on a variety of resources, but mainly on soil microbes and decomposing organic matter (Bellinger et al., 2023). Mesostigmatid mites, on the other hand, are mostly free-living predators that are at the top of the soil food web (Lindquist et al., 2009).
Mesostigmatid and oribatid mites, and collembolans have been used as indicators of disturbance (Milano et al., 2017; Meehan et al., 2019; Manu et al., 2021), soil pollution (Fiera, 2009; Wierzbicka et al., 2019) and landfill restoration (Ashwood et al., 2022), while both oribatid mites and collembolans respond to warming (Lindo, 2015; Meehan et al., 2020; Barreto et al., 2021). In the case of peatlands, oribatid mites have been shown to negatively respond to disturbance-induced lower moisture levels (Silvan et al., 2000; Lehmitz, 2014). They are considered good predictors of moisture, suggesting these dominant microarthropod groups could form an important multi-taxa approach for climate bioindication in peatland systems (Lehmitz et al., 2020). However, specific responses to climate factors are often correlated or mitigated by other environmental conditions that suggest more permanent shifts in community composition may take longer to emerge.
Here we assessed the biodiversity (species abundance, richness) and composition of three dominant soil microarthropod groups (Oribatida, Mesostigmata, and Collembola) over 4 years across a range of experimental warming temperatures (between 0°C and +9°C) crossed with elevated CO2 conditions in the large-scale peatland experimental system called SPRUCE (Hanson et al., 2017). Established and ongoing multi-measurement approaches of SPRUCE further allows us to examine whether response to experimental treatments interact with other environmental variables (i.e., soil moisture). The novelty of this study lies on the long-term data we present, but more importantly, it is in line with recent calls for multiple and interactive global change factors studies in soils (e.g., Rillig et al., 2019). Following Blankinship et al. (2011), we hypothesize that the experimental treatments will significantly affect the peatland microarthropod communities. While we predict that elevated atmospheric CO2 effects would be generally weak across the three taxonomic groups as any effects are indirect through plant-mediated processes (including through root exudates), we predict that the direct effects of soil warming will increase microarthropod abundances due to increased metabolic rates (Brose et al., 2012), particularly among the small-bodied, parthenogenetic species. At the same time, warming-induced drying of peat-soils will decrease species richness due to losses in peatland-specific taxa while non-peatland specific organisms are restricted from entering the experimental system. We expect that the bi-directional nature of these responses will play out over time, and that these effects would be more observable over time.
2 Materials and methods
2.1 Site description and experimental design
The SPRUCE experiment (Spruce and Peatland Responses Under Changing Environments—https://mnspruce.ornl.gov/) is an unparalleled ecosystem-scale climate manipulation initiated by the US Department of Energy located at an ombrotrophic (Sphagnum-dominated) bog within the U.S. Department of Agriculture Forest Service’s Marcell Experimental Forest (Bog S1, N 47°30.476′; W 93°27.162′) in northern Minnesota, United States. The SPRUCE experiment consists of 10 large-scale (12.8 m diameter × 7 m tall) open-top octagonal enclosures (see Supplementary Figure S1) with belowground warming (to a depth of 2 m) initiated in 2014, followed by aboveground warming (enclosure air temperature) in 2015. Elevated CO2 treatments were initiated in June 2016. Five temperature treatments were obtained in each of two enclosures that range from ambient temperatures for the region to +9°C warming (target temperatures of +0°C, +2.25°C, +4.5°C, +6.75°C, and +9°C). Duplicate enclosures are subjected to either ambient (∼400 ppm) or elevated (∼900 ppm) atmospheric CO2 concentration during daytime hours. See Hanson et al. (2017) for full description of the SPRUCE experimental set-up.
A general description of the site is found elsewhere (e.g., Krassovski et al., 2015; https://mnspruce.ornl.gov/design), however, pertinent to the belowground biodiversity is that the ombrotrophic bog has an average pH of 4.1 (Iversen et al., 2014) and is covered by Sphagnum divinum (formerly S. magellanicum), S. fallax, S. angustifolium, and S. fuscum, Polytrichum sp. and Pleurozium schreberi (Norby et al., 2019) with other vegetation including Picea mariana (black spruce), Larix laricina (tamarack) and select shrub (e.g., Chamaedaphne calyculata (leatherleaf), Rhododendron groenlandicum (Labrador tea), and sedge (Eriophrium). Between 1961 and 2005, the average annual precipitation was 768 mm and the average air temperature was 3.3°C (Sebestyen et al., 2011), which has increased 0.4°C over the last 40 years.
We sampled all ten SPRUCE enclosures every October in the years 2015, 2017, 2018, and 2019. To account for within-chamber variability, three subsamples were collected from the top 10 cm Sphagnum moss layer (∼10 cm3; 88.04 g ± 1.02 g w.w.) of open Sphagnum areas (i.e., not directly under trees) of each chamber and combined into a composite sample; 10 samples were collected per year. Soil invertebrates were collected from the composite samples using dry extraction via portable Berlese extractors within 1 h of collection into 75% EtOH over 72 h. Moisture content of each composite sample was based on gravimetric mass loss of the sample weighed prior to and immediately following fauna extraction and is expressed as a ratio of moisture to dry peat mass.
After extraction, all microarthropods were enumerated and sorted into major taxonomic groups with the dominant taxa (i.e., Oribatida, Mesostigmata, Collembola) being further identified to genus or species level, where possible. Adult oribatid and mesostigmatid mites were identified and enumerated using a dissecting microscope (Nikon SMZ745T) with representative individuals of each morphotype slide mounted in Hoyer’s medium for examination under compound light microscope (Nikon Eclipse Ni-U); genera and species were identified based on the primary taxonomic literature. Oribatid mite species identifications were confirmed with the keys in Behan-Pelletier and Lindo (2023). All collembolan specimens were cleared in Nesbitt’s solution, mounted on glass slides in Hoyer’s medium and identified using a Zeiss Axio Scope A1 microscope; specimens were deposited in the Coleção de Referência de Fauna de Solo (CRFS) at the Universidade Estadual da Paraíba, João Pessoa, PB, Brazil. These three taxonomic groups comprised 89 species (48 spp. Oribatida, 20 spp. Mesostigmata, 21 spp. Collembola) and were >70% of the total microarthropods collected (Supplementary Table S1). The species richness and abundance within Oribatida, Mesostigmata, and Collembola, and species richness and abundance of all microarthropods were standardised by dry weight (dwt) of peat sampled, and are expressed as the number of species and the number of individuals per gram dry weight, respectively.
2.2 Statistical analyses
We assessed the annual response of peat microarthropod communities to warming and elevated atmospheric CO2 concentration using Linear Mixed-Effects models (LMM) in R program version 4.0.1 (R Core Team, 2020). Specifically, we examined the response of the total microarthropod species richness and abundance (including other groups like prostigmatid and astigmatid mites), and individual taxon species richness and abundance using the {lme} function in the “nlme” package (Pinheiro and Bates, 2000) and {Anova} function in the “car” package (Fox and Weiberg, 2019). These models account for the experimental treatment effects (warming and [CO2]) as well as peat moisture content, year of sampling and the specific enclosure sampled. The models we used were built as follows:
Both warming and elevated CO2 concentration were treated as fixed effects with warming being a numerical variable represented by the average annual (11th October—10th October) peat temperature measured at −10 cm and CO2 being a discrete variable (i.e., “ambient” or “elevated”). Year was also treated as a discrete and fixed variable in the models due to the progression of treatment application (deep peat heating was initiated during summer 2014, whole ecosystem warming was initiated in August 2015, and CO2 treatment started in June 2016). For this reason, the data collected in 2015 were entirely treated as “control” for CO2 treatments. Lastly, enclosures were included in the model as a random effect to account for variability among enclosures and repeated sampling.
To examine overall microarthropod, oribatid and mesostigmatid mite, and collembolan community composition we used the function {adonis2} in R to perform a three-way permutation multivariate Anova (PermAnova) based on Bray-Curtis dissimilarity of composition among samples to determine how [CO2], target temperature (as per experimental design) and year affected communities across all sampling events. Communities were further assessed visually through non-metric multidimensional scaling (Nmds) using the discrete variables [CO2], target temperature and year. To test the relationship between environmental variables with specific species (considering all microarthropods together), we implemented distance-based redundancy analyses (db-RDA) also based on Bray-Curtis dissimilarity of composition among samples (communities) using the {capscale} function in the “vegan” package (Oksanen et al., 2019). Specifically, we tested whether warming (actual temperatures), elevated [CO2], peat moisture content, year and enclosure help explain the variance of the top 50% of species that had the highest axis loadings across all sampling periods. To do that, we used the sum of the absolute value of the axis loadings for each species from the first two (i.e., dominant) axes (CAP1, CAP2) to select 45 species of microarthropods, 24 species of oribatid mites, 10 species of mesostigmatid mites and 10 species of collembolans (for further analysis see Supplementary Table S1). All models were initially written as:
We used the {ordistep} function to select the components of the models after confirming no collinearity between them using the function {vif.cca}. All analyses used an alpha of 0.05, and final plots were created in R with the packages “ggplot2” (Wickham, 2016) and “ggrepel” (Slowikowski et al., 2021).
3 Results
Neither warming (χ2 = 2.874; p = 0.579), nor elevated [CO2] (χ2 = 3.251, p = 0.516) significantly affected species richness when all microarthropods were analysed together. Total microarthropod abundance was also not significantly affected by warming (χ2 = 5.043, p = 0.282) or elevated [CO2] (χ2 = 6.755, p = 0.149). However, warming significantly decreased peat moisture (χ2 = 6.044, p = 0.048) (Figure 1) and thus indirect effects of warming on species richness and abundance through decreases in peat moisture were detected. In particular, total microarthropod richness was significantly higher under higher levels of moisture (χ2 = 22.781, p < 0.001) (Figure 2A). Peat moisture was also an important factor driving total microarthropod abundance, which also increased with moisture, albeit marginally significant (χ2 = 9.400, p = 0.052).
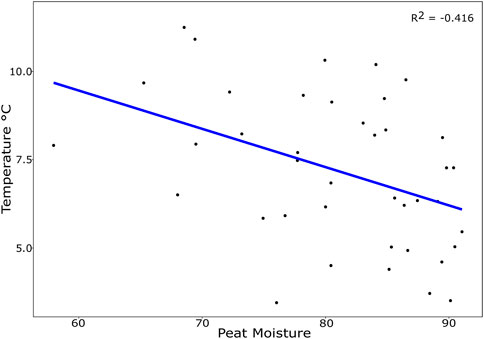
FIGURE 1. Relationship between peat moisture (%) and peat temperature (°C) with 10 samples collected per year in 2015, 2017, 2018, and 2019. Peat temperature is the annual average temperature (11th October to 10th October) at −10 cm measured within each enclosure with peat moisture reflects the moisture content of the peat sample based on gravimetric mass loss.
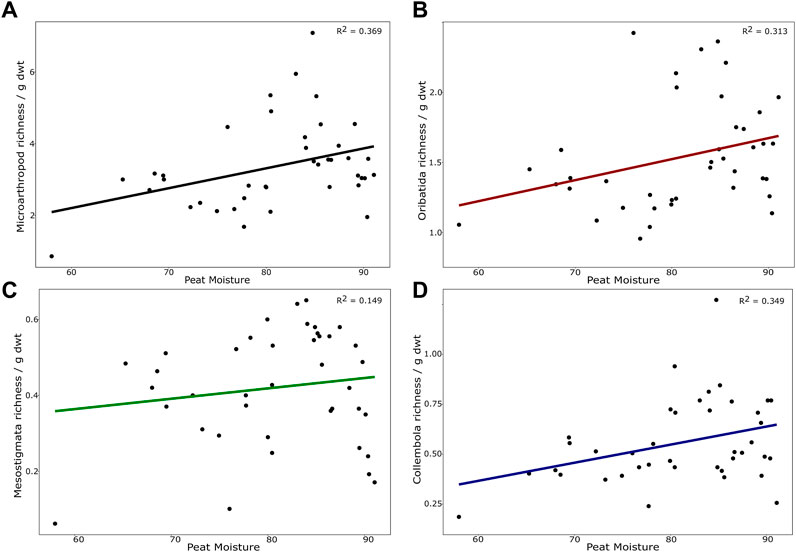
FIGURE 2. Number of species (richness) standardized per gram of dry weight peat sample in relation to peat moisture content (%). (A) Richness of all microarthropods considered together (oribatid, mesostigmatid, prostigmatid and astigmatid mites, and collembolans). Prostigmatid and astigmatid mites were not morphotyped and thus considered one “species” each. (B) Richness of adult oribatid mites; (C) Richness of adult mesostigmatid mites; (D) Richness of collembolans (juveniles were also identified to species level).
The reduction in peat moisture due to warming increased over time (χ2 = 12.140, p < 0.001), where only in the first year of whole ecosystem warming (i.e., 2015), peat moisture was not influenced by experimental treatment. Year was also a significant driver of microarthropod richness led by high variability in species richness in our model (χ2 = 7.959, p = 0.004) (particularly high in 2019), although similar effects of year are not observed in total microarthropod abundance (χ2 = 0.302, p = 0.582). Not surprisingly, as the dominant group in our samples, oribatid mites showed similar results. Oribatid mite species richness was not significantly related to warming (χ2 = 2.781, p = 0.594), elevated [CO2] (χ2 = 5.782, p = 0.216) or time (χ2 = 2.419, p = 0.119), although it was significantly higher under higher moisture levels (χ2 = 14.231, p = 0.006) (Figure 2B). Oribatid mite abundance (adults and immatures) did not respond significantly to the experimental treatments either (warming: χ2 = 5.182, p = 0.269; elevated [CO2]: χ2 = 4.698, p = 0.319), nor did they significantly change in response to moisture content levels (χ2 = 6.691, p = 0.153) nor time (χ2 = 0.028, p = 0.866). Nonetheless, when analysed separately, adult oribatid mite abundance significantly increased with moisture content levels (χ2 = 10.671, p = 0.030).
Mesostigmatid mite richness significantly increased with both warming (χ2 = 9.876, p = 0.042) and peat moisture level contents (χ2 = 19.836, p < 0.001) (Figure 2C), and it varied across years (χ2 = 2.659, p = 0.100), albeit only marginally; elevated [CO2] (χ2 = 5.264, p = 0.261) was not a significant factor. Mesostigmatid mite abundance did not significantly respond to warming (χ2 = 6.119, p = 0.190), year (χ2 = 0.178, p = 0.672), peat moisture (χ2 = 5.180, p = 0.269) or [CO2] (χ2 = 4.609, p = 0.329). Similarly, for Collembola, peat moisture was an important driver, increasing the richness of collembolans (χ2 = 15.331, p = 0.004) (Figure 2D), while the experimental treatments had no direct effects on collembolan richness (warming: χ2 = 4.136, p = 0.387; [CO2]: χ2 = 0.935, 0.919). Collembola species richness also significantly varied over time (χ2 = 10.209, p = 0.001), with the highest variability in collembolan richness observed in 2019. The abundance of collembolans did not significantly change with any of our variables (warming: χ2 = 1.396, p = 0.844; [CO2]: χ2 = 0.711, p = 0.949; peat moisture: χ2 = 1.219, p = 0.874; year: χ2 = 0.424, p = 0.514).
Further examination into the composition of the entire microarthropod community and all taxonomic groups also suggest that warming-induced peat soil drying affected the composition of microarthropod communities, as well as the variability across experimental treatment years. Community composition of adult microarthropods was significantly different across sampling years (PermAnova: F = 2.165, p = 0.022), and the Nmds plot suggests that variability in communities increased over time (Figure 3). Yet, neither warming (in this case, target temperature: F = 1.155, p = 0.343) nor elevated [CO2] significantly affected microarthropod communities (F = 0.964, p = 0.465) and there were no interactions between these factors (PermAnova: p results >0.05). However, the db-RDA model examining the 45 species that exhibited highest (absolute) axis scores in the preliminary analysis showed that warming (F = 2.042, p = 0.015), and peat moisture (F = 2.677, p = 0.005) were significant factors (Figure 4) (see Supplementary Table S2 for full model summaries). The overall model was significant in explaining the variance in the community (F = 2.101, p = 0.001), with the first axis CAP1 explaining 6.82% of the variance (F = 2.183, p = 0.008).
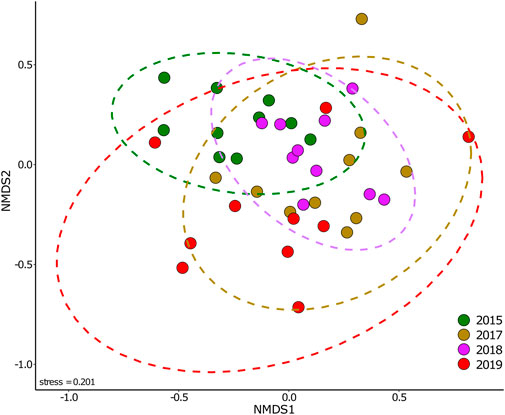
FIGURE 3. Compositional similarities of microarthropod communities sampled across 4 years. Forty samples were collected in October of 2015, 2017, 2018, and 2019. Communities are plotted by sampling event. Microarthropod communities are based on standardised abundance of individual species from each enclosure. Stress = 0.201, number of dimensions (k) = 3. The ellipses indicate 95% confidence intervals.
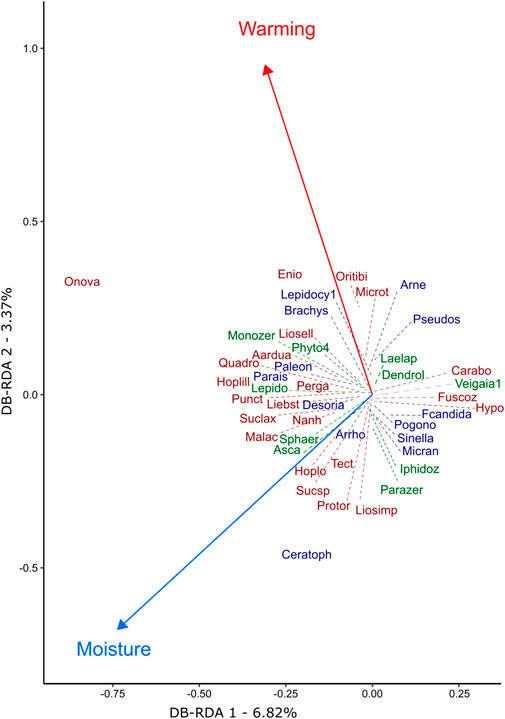
FIGURE 4. Abiotic factors driving bog microarthropod community composition (2015–2019) in peat soil samples analysed by db-RDA. The top 50% species with the highest axis loadings are plotted and related to peat moisture content and warming. Arrows indicate how the explaining variables are related to ordination space, and dashed lines represent the actual location of species in this multidimensional space. Species in green are Mesostigmata, in blue Collembola, and in dark red Oribatida.
Oribatid mite communities were also significantly different between sampling events (PermAnova: F = 2.838, p = 0.016), with composition generally increasing in variability over time. Likewise, the db-RDA model for oribatid mites showed the final model significantly explained the variance in the communities (F = 2.209, p = 0.001), and variance was explained by warming (F = 2.358, p = 0.005), and peat moisture (F = 2.771, p = 0.005) within the first axis CAP1 (F = 3.091, p = 0.001), which explained 7.46% of the final model results (Supplementary Figure S2).
Mesostigmatid mite communities showed a different pattern from oribatid mites where a significant change in mesostigmatid composition was observed with target temperature (PermAnova: F = 1.728, p = 0.030), with variability generally increasing with warming. Communities were also significantly different over time (PermAnova: F = 3.297, p = 0.001). The db-RDA model for the mesostigmatid mite communities (F = 2.271, p = 0.001) included warming (F = 1.779, p = 0.020) and year (F = 2.279, p = 0.005) as significant factors. The first axis CAP1 significantly explained 8.76% of the variance (F = 3.641, p = 0.001) (Supplementary Figure S3). Analyses at the community level showed that collembolan communities did not respond to any variables in the PermAnova (all p > 0.05), or could be explained by our factors in the db-RDA due to lack of constrained components in the model (Supplementary Table S2).
4 Discussion
Using an unparalleled ecosystem-scale climate experiment with interacting global change factors as treatments (warming and elevated [CO2]) and a long-term dataset that allowed us to investigate their cumulative effects, we observed significant changes in three soil microarthropod taxonomic groups, which we explored as potential climate indicators. We found the strongest driver of changes in species richness across all groups was indirectly related to the experimental (treatment) conditions, specifically warming-induced drying of the peatland surface soils. In line with our prediction, decreases in peat moisture content led to strong reductions in total microarthropod, collembolan, oribatid and mesostigmatid mite richness. Lower moisture content may have created unfavourable conditions to certain species, and this reduction in species richness could be explained by losses in taxa that inhabit wetter microhabitats such as Zetomimus setosus (Banks, 1895), previously collected from wet vegetation at edge of and on ponds, and Mycobates hyaleus (Behan-Pelletier, 1994), known from moss and lichen (Behan-Pelletier and Lindo, 2023) both species that we only collected in control temperature plots. Warming-induced reductions in soil moisture leading to decreased microarthropod richness and abundance have previously been observed (Kardol et al., 2011; Barreto et al., 2021), and in those studies, oribatid mites typically drove the trends. Soil moisture is a known driver of oribatid mite communities across a variety of terrestrial ecosystem types (Tsiafouli et al., 2005; Elo et al., 2018; Jakšová et al., 2020) with most studies showing a positive relationship of richness and abundance with soil moisture. Previous studies have shown that lowered levels of moisture have overall negative effects on peatland oribatid mite richness (Lehmitz, 2014) and proportional abundance (Silvan et al., 2000). Given that warming-induced drying of the experimental enclosures is increasing as the experiment continues, it is likely that the response of peatland microarthropods will continue to become more apparent over time, but consequences at the ecosystem level are yet to be investigated.
Warming and warming-induced drying of peatland systems can drive other biotic changes that can affect microarthropod communities. For instance, Barreto et al. (2021) also observed a reduction in soil moisture associated with experimental warming using both passive (open-top chambers) and active ground heating, and these combined effects led to reductions in Sphagnum moss cover (Lyons et al., 2020) alongside increases in sedge, shrub and tree cover. These trends are also observed at the SPRUCE site; high mortality of Sphagnum has been observed in warmed plots (Iversen et al., 2022) with Sphagnum cover declining from close to 100% of the ground area to <50% of the ground area in the warmest enclosures (Norby et al., 2019). As Sphagnum mosses provide habitat, and buffer changes in soil moisture due to their high water holding capacity (Bengtsson et al., 2020), reductions in Sphagnum cover could mechanistically explain the reductions in microarthropod richness that would potentially face unfavourable drier conditions. Contrary to what we found, Zhang et al. (2023) using open-top chambers (OTCs) in a wetland in China showed that warming did not influence the richness of Collembola under warming-induced drier conditions. We posit that our lower collembolan richness under drier conditions might be explained by migration of species downward, as seen for a blanket bog in Sweden in Krab et al. (2010), who also suggested that the combined effects of higher temperatures and lower moisture levels may cause desiccation stress and strongly influence the vertical stratification of collembolans. Nonetheless, peat moisture has been shown to be the most important driver determining the peatland collembolan communities in other studies (e.g., Sławski et al., 2022). At the same time, Barreto et al. (2021) observed increases in oribatid species richness under warming, which they attributed to the influx of nearby forest species colonizing their peat site. We posit that reductions in species richness are attributed to the warming-induced drying of the peat soils and the loss of peatland-specific taxa, while the experimental enclosures at SPRUCE would restrict dispersal of forest and more xeric species from entering the experimental system.
We predicted that the direct effects of soil warming, which started belowground in 2014, would increase the abundances of microarthropods, particularly among small-bodied and parthenogenetic species, as was observed by Lindo (2015). This is because increases in temperature have been shown to accelerate metabolic rates (Brose et al., 2012). However, we found no evidence that warming, or the indirect effects of peat drying, significantly affected total microarthropod or major taxon abundance. Nonetheless, when investigating species-level responses to warming and lowered moisture conditions, we detect treatment responses. Liochthonius sellnicki (Thor, 1930) and Eniochthonius mahunkai (Norton and Behan-Pelletier, 2007), for instance, both parthenogenetic species, increased in abundance under warming as previously seen (Lindo, 2015; Barreto et al., 2021). However, the most abundant mesostigmatid mite we collected, the sexually reproducing Parazercon radiatus Berlese, 1910, significantly decreased in abundance under warmer and drier conditions, which could be in response to a bottom-up effect caused by their food source, nematodes, known for living within the water film and being abundant under high moisture levels in peatlands (Kamath et al., 2022). Further, two parthenogenetic species of oribatid mites (E. mahunkai and Oppiella nova (Oudemans, 1902)) were associated with soil temperature as a variable in the db-RDA analysis (see Supplementary Table S1 for list of species identified as asexual). Oppiella nova is a cosmopolitan species found in almost all habitats sampled and sometimes associated with disturbance (Melekhina et al., 2021), while E. mahunkai is a peatland specialist (Behan-Pelletier and Lindo, 2023). Markkula et al. (2019) found that year-round warming, as with the SPRUCE experiment, had no effect on total abundance of oribatids, possibly because of contrasting effects of warming in summer versus winter seasons. While warming may create metabolically more favourable conditions over winter months, increases in temperature during summer months, where the field can exceed 37.5°C daytime air temperatures, could have a negative effect on many soil microarthropod species. We found no evidence for metabolic increases in reproduction under warming and observed no shifts in the proportion of juveniles that would infer greater reproduction rate under warming. While two previous studies have found warming significantly increased the abundance of small-bodied, non-sexually (parthenogenetic) reproducing species (Lindo, 2015; Markkula et al., 2019), the response of microarthropod abundance to warming in non-peatland systems have been mixed (Blankinship et al., 2011; Vestergård et al., 2015; Alatalo et al., 2017; Meehan et al., 2020).
Understandably, the effects of elevated atmospheric CO2 on microarthropods were weak as expected effects would be indirect through plant-mediated processes, which is consistent with other studies (Blankinship et al., 2011; Lindo, 2015; Peng et al., 2022) as well with our prediction. However, a few studies have found elevated CO2 affected oribatid and collembolan diversity through changes in litter C:N ratio (Holmstrup et al., 2017) while soil detritivores increased with increased inputs of labile soil carbon under elevated CO2 (Eisenhauer et al., 2012). Similar indirect effects of warming are anticipated, albeit also weakly potentially affecting soil organisms. Warming has strongly increased fine-root growth at the SPRUCE site by over an order of magnitude in the warmest treatment, with stronger responses in shrubs than in trees or graminoids (Malhotra et al., 2020). Growth of fine roots would provide extra labile carbon via root exudates that could help fuel the microbial community with bottom-up effects on soil microarthropods. The majority of oribatid mites and collembolans are expected to be feeding on fungi. Similarly, warming can shift peatland microbial communities of hollows towards communities present in hummocks (Maillard et al., 2021), potentially changing the resource base for microarthropods.
Overall and taxon-specific shifts in soil microarthropods at the SPRUCE site suggest treatment effects are taking time to emerge, and possibly leading to greater heterogeneous communities under warming. At the same time, Roos et al. (2020) found that the negative effects of warming on collembolan and oribatid community composition persisted even after 9 years after the experimental treatment ended, suggesting shifts in microarthropod composition as long-lasting and potentially not reversible. The work presented here is one component of larger results emerging from the SPRUCE experiment that suggest belowground biodiversity and ecosystem function are altered under warming. Specifically, warming has enhanced the degradation of organic matter and greenhouse gas production (Wilson et al., 2021), with linear increases in net carbon loss associated with warming treatments (Hanson et al., 2020), while additional losses in soil carbon are observed through increased methane (CH4) emissions (Hopple et al., 2020). Taken together the changes belowground indicate a loss of carbon storage potential, and changes in members of the soil food web will also alter how carbon and nitrogen are cycled, although this remains largely understudied (but see https://github.com/robertwbuchkowski/soilfoodwebs for an R package developed for soil food webs). While we expect that the cumulative and interactive effects of the SPRUCE experimental treatments on soil biodiversity will continue to emerge, the first 5 years of the experiment already suggest effects are becoming more observable over time. Resultingly, many researchers advocate for longer studies that incorporate multiple factors (Rillig et al., 2019; Barreto and Lindo, 2022), especially given the importance of soil biodiversity in belowground carbon transformations and storage that help regulate climate feedbacks.
Data availability statement
The original contributions presented in the study are included in the article/Supplementary Material, further inquiries can be directed to the corresponding author.
Author contributions
CB: Sample collection and processing, oribatid mite identification, data analysis, and writing. PC: Mesostigmatid mite identification. EdL: Collembolan identification. LS: Collembolan identification. DZ: Collembolan identification. RK: Sample collection. PH: Conceptualization and methodology. ZL: Sample collection, oribatid mite identification, data analysis and writing. All authors contributed to manuscript revision, read, and approved the submitted version.
Funding
Funding was provided from the Natural Sciences and Engineering Research Council of Canada (NSERC) Discovery Grant program (ZL #418241–2012 and 05901–2019) and the Ontario Ministry of Research, Innovation and Science Early Researcher Award (ZL #ER13–09-243).
Acknowledgments
The SPRUCE experiment is supported by the Biological and Environmental Research program in the United States Department of Energy’s Office of Science. We thank the USDA Forest Service for our use of the Marcell Experimental Forest facilities and thank Robert Nettles for the accommodation during the field campaigns. We thank Sharon Patterson (University of Minnesota) for helping with sampling and extracting microarthropods, Mirindi Eric Dusenge (Western University) for discussions involving the environmental data available for the SPRUCE project and Matthew Meehan (University of Manchester) for statistical suggestions.
Conflict of interest
The authors declare that the research was conducted in the absence of any commercial or financial relationships that could be construed as a potential conflict of interest.
Publisher’s note
All claims expressed in this article are solely those of the authors and do not necessarily represent those of their affiliated organizations, or those of the publisher, the editors and the reviewers. Any product that may be evaluated in this article, or claim that may be made by its manufacturer, is not guaranteed or endorsed by the publisher.
Supplementary material
The Supplementary Material for this article can be found online at: https://www.frontiersin.org/articles/10.3389/fenvs.2023.1153683/full#supplementary-material
References
Ainsworth, E. A., and Long, S. P. (2005). What have we learned from 15 years of free-air CO2 enrichment (face)? A meta-analytic review of the responses of photosynthesis, canopy. New Phytol. 165, 351–371. doi:10.1111/j.1469-8137.2004.01224.x
Alatalo, J. M., Jägerbrand, A. K., Juhanson, J., Michelsen, A., and Ľuptáčik, P. (2017). Impacts of twenty years of experimental warming on soil carbon, nitrogen, moisture and soil mites across alpine/subarctic tundra communities. Sci. Rep. 7, 44489. doi:10.1038/srep44489
Ashwood, F., Barreto, C., Butt, K. R., Lampert, M., Doick, K., and Vanguelova, E. I. (2022). Earthworms and soil mesofauna as early bioindicators for landfill restoration. Soil Res. doi:10.1071/SR21286
Barreto, C., Branfireun, B. A., McLaughlin, J. W., and Lindo, Z. (2021). Responses of oribatid mites to warming in boreal peatlands depend on fen type. Pedobiologia 89, 150772. doi:10.1016/j.pedobi.2021.150772
Barreto, C., and Lindo, Z. (2021). Checklist of oribatid mites (Acari: Oribatida) from two contrasting boreal fens: An update on oribatid mites of Canadian peatlands. Syst. Appl. Acarol. 26 (5), 866–884. doi:10.11158/saa.26.5.4
Barreto, C., and Lindo, Z. (2022). Response of soil biodiversity to global change. Pedobiologia 90, 150792. doi:10.1016/j.pedobi.2022.150792
Beaulne, J., Garneau, M., Magnan, G., and Boucher, É. (2021). Peat deposits store more carbon than trees in forested peatlands of the boreal biome. Sci. Rep. 11, 2657. doi:10.1038/s41598-021-82004-x
Behan-Pelletier, V., and Lindo, Z. (2023). Oribatid mites. Biodiversity, taxonomy and ecology. Boca Raton, Florida: Taylor and Francis, 508.
Bellinger, P. F., Christiansen, K. A., and Janssens, F. (2023). checklist of the Collembola of the world. Avaliable At: http://www.collembola.org.
Bengtsson, F., Granath, G., Cronberg, N., and Rydin, H. (2020). Mechanisms behind species-specific water economy responses to water level drawdown in peat mosses. Ann. Bot-London 126, 219–230. doi:10.1093/aob/mcaa033
Blankinship, J. C., Niklaus, P. A., and Hungate, B. A. (2011). A meta-analysis of responses of soil biota to global change. Oecologia 165, 553–565. doi:10.1007/s00442-011-1909-0
Brose, U., Dunne, J. A., Montoya, J. M., Petchey, O. L., Schneider, F. D., and Jacob, U. (2012). Climate change in size structured ecosystems. Philos. T R. Soc. B 367, 2903–2912. doi:10.1098/rstb.2012.0232
Campbell, J. L., Rustad, L. E., Lindsey, E., Boyer, E. W., Christopher, S. F., Driscoll, C. T., et al. (2009). Consequences of climate change for biogeochemical cycling in forests of northeastern north America. Can. J. Res 39, 264–284. doi:10.1139/X08-104
Eisenhauer, N., Cesarz, S., Koller, R., Worm, K., and Reich, P. B. (2012). Global change belowground: Impacts of elevated CO2, nitrogen, and summer drought on soil food webs and biodiversity. Glob. Change Biol. 18, 435–447. doi:10.1111/j.1365-2486.2011.02555.x
Elo, R. A., Penttinen, R., and Sorvari, J. (2018). Distribution of oribatid mites is moisture-related within red wood ant Formica polyctena nest mounds. Appl. Soil Ecol. 124, 203–210. doi:10.1016/j.apsoil.2017.11.013
FAO (2020). State of knowledge of soil biodiversity – status, challenges and potentialities. Summary for policy makers. Rome, Italy: FAO, 616.
Fenner, N., Freeman, C., Lock, M. A., Harmens, H., Reynolds, B., and Sparks, T. (2007). Interactions between elevated CO2 and warming could amplify DOC exports from peatland catchments. Environ. Sci. Technol. 41, 3146–3152. doi:10.1021/es061765v
Fiera, C. (2009). Biodiversity of Collembola in urban soils and their use as bioindicators for pollution. Pesqui. Agropecuária Bras. 44, 868–873. doi:10.1590/S0100-204X2009000800010
Filser, J., Faber, J. H., Tiunov, A. V., Brussaard, L., Frouz, J., De Deyn, G., et al. (2016). Soil fauna: Key to new carbon models. Soil 2, 565–582. doi:10.5194/soil-2-565-2016
Fox, J., and Weisberg, S. (2019). An R companion to applied regression. Third edition. Thousand Oaks CA: Sage. Avaliable At: https://socialsciences.mcmaster.ca/jfox/Books/Companion/.
Frolking, S., Talbot, J., Jones, M. C., Treat, C. C., Kauffman, J. B., Tuittila, E. S., et al. (2011). Peatlands in the Earth’s 21st century climate system. Environ. Rev. 19, 371–396. doi:10.1139/a11-014
Geisen, S., Wall, D. H., and van der Putten, W. H. (2019). Challenges and opportunities for soil biodiversity in the Anthropocene. Curr. Biol. 19 (19), PR1036–R1044. doi:10.1016/j.cub.2019.08.007
Haimi, J., Laamanen, J., Penttinen, R., Räty, M., Koponen, S., Kellomäki, S., et al. (2015). Impacts of elevated CO2 and temperature on the soil fauna of boreal forests. Appl. Soil Ecol. 30, 104–112. doi:10.1016/j.apsoil.2005.02.006
Hanson, P. J., Griffiths, N. A., Iversen, C. M., Norby, R. J., Sebestyen, S. D., Phillips, J. R., et al. (2020). Rapid net carbon loss from a whole-ecosystem warmed peatland. AGU Adv. 1, e2020AV000163. doi:10.1029/2020AV000163
Hanson, P. J., Riggs, J. S., Nettles, W. R., Phillips, J. R., Krassovski, M. B., Hook, L. A., et al. (2017). Attaining whole-ecosystem warming using air and deep-soil heating methods with an elevated CO2 atmosphere. Biogeosciences 14, 861–883. doi:10.5194/bg-14-861-2017
Holmstrup, M., Damgaard, C., Schmidt, I. K., Arndal, M. F., Beier, C., Mikkelsen, T. N., et al. (2017). Long-term and realistic global change manipulations had low impact on diversity of soil biota in temperate heathland. Sci. Rep. 7, 41388–41411. doi:10.1038/srep41388
Hopple, A. M., Wilson, R. M., Kolton, M., Zalman, C. A., Chanton, J. P., Kostka, J., et al. (2020). Massive peatland carbon banks vulnerable to rising temperatures. Nat. Comm. 11, 2373. doi:10.1038/s41467-020-16311-8
Hugelius, G., Loisel, J., Chadburn, S., Jackson, R. B., Jones, M., MacDonald, G., et al. (2020). Large stocks of peatland carbon and nitrogen are vulnerable to permafrost thaw. P Natl. Acad. Sci. U. S. A. 117, 20438–20446. doi:10.1073/pnas.1916387117
IPCC (2018). “Summary for policymakers,” in Global warming of 1.5°C: An IPCC special report on the impacts of global warming of 1.5°C above pre-industrial levels and related global greenhouse gas emission pathways, in the context of strengthening the global response to the threat of climate change, sustainable development, and efforts to eradicate poverty. Editors V. Masson-Delmotte, P. Zhai, H. -O. Pörtner, D. Roberts, J. Skea, and P. R. Shukla (Geneva: World Meteorological Organization), 32.
Iversen, C. M., Hanson, P. J., Brice, D. J., Phillips, J. R., McFarlane, K. J., Hobbie, E. A., et al. (2014). “SPRUCE peat physical and chemical characteristics from experimental plot cores,” in 2012. Oak Ridge national laboratory, TES SFA (Oak Ridge, Tennessee, U.S.A: U.S. Department of Energy). doi:10.3334/CDIAC/spruce.005
Iversen, C. M., Latimer, J., Brice, D. J., Childs, J., Stel, H. M. V., Defrenne, C. E., et al. (2022). Whole-ecosystem warming increases plant-available nitrogen and phosphorus in an ombrotrophic bog. Ecosystems 26, 86–113. doi:10.1007/s10021-022-00744-x
Jakšová, P., Ľuptáčik, P., Miklisová, D., Horváthová, F., and Hlavatá, H. (2020). Oribatida (Acari) communities in arable soils formed under waterlogged conditions: The influence of a soil moisture gradient. Biologia 75, 243–257. doi:10.2478/s11756-019-00291-2
Kamath, D., Barreto, C., and Lindo, Z. (2022). Nematode contributions to the soil food web trophic structure of two contrasting boreal peatlands in Canada. Pedobiologia 93–94, 150809. doi:10.1016/j.pedobi.2022.150809
Kardol, P., Reynolds, W. N., Norby, R. J., and Classen, A. T. (2011). Climate change effects on soil microarthropod abundance and community structure. Appl. Soil Ecol. 47, 37–44. doi:10.1016/j.apsoil.2010.11.001
Krab, E. J., Oorsprong, H., Berg, M. P., and Cornelissen, J. H. C. (2010). Turning northern peatlands upside down: Disentangling microclimate and substrate quality effects on vertical distribution of Collembola. Funct. Ecol. 24, 1362–1369. doi:10.1111/j.1365-2435.2010.01754.x
Krassovski, M. B., Riggs, J. S., Hook, L. A., Nettles, W. R., Hanson, P. J., and Boden, T. A. (2015). A comprehensive data acquisition and management system for an ecosystem-scale peatland warming and elevated CO2 experiment. Geosci. Instrum. Method Data Syst. 4, 203–213. doi:10.5194/gi-4-203-2015
Lehmitz, R., Haase, H., Otte, V., and Russel, D. (2020). Bioindication in peatlands by means of multi-taxa indicators (Oribatida, Araneae, Carabidae, vegetation). Ecol. Indic. 109, 105837. doi:10.1016/j.ecolind.2019.105837
Lehmitz, R. (2014). The oribatid mite community of a German peatland in 1987 and 2012 – effects of anthropogenic desiccation and afforestation. Soil Org. 86, 131–145.
Leuzinger, S., and Hättenschwiler, S. (2013). Beyond global change: Lessons from 25 years of CO2 research. Oecologia 171, 639–651. doi:10.1007/s00442-012-2584-5
Limpens, J., Berendse, F., Blodau, C., Canadel, J. G., Freeman, C., Holden, H., et al. (2008). Corrigendum to Peatlands and the carbon cycle: From local processes to global implications a synthesis published in biogeosciences, 5, 1475–1491, 2008. Biogeosciences 5, 1739–1491. doi:10.5194/bg-5-1739-2008
Lindo, Z. (2015). Warming favours small-bodied organisms through enhanced reproduction and compositional shifts in belowground systems. Soil Biol. Biochem. 91, 271–278. doi:10.1016/j.soilbio.2015.09.003
Lindquist, E. E., Krantz, G., and Walter, D. E. (2009). “Order Mesostigmata,” in A manual of Acarology (Lubbock: Texas Tech University Press), 124–232.
Lyons, C. L., Branfireun, B. A., McLaughlin, J., and Lindo, Z. (2020). Simulated climate warming increases plant community heterogeneity in two types of boreal peatlands in north–central Canada. J. Veg. Sci. 31, 908–919. doi:10.1111/jvs.12912
Maillard, F., Fernandez, C. W., Mundra, S., Heckman, K. A., Kolka, R. K., Kauserud, H., et al. (2021). Warming drives a ‘hummockification’ of microbial communities associated with decomposing mycorrhizal fungal necromass in peatlands. New Phytol. 234, 2032–2043. doi:10.1111/nph.17755
Malhotra, A., Brice, D., Childs, J., Graham, J. D., Hobbie, E. A., Stel, H. V., et al. (2020). Peatland warming strongly increases fine-root growth. PNAS 177, 17627–17634. doi:10.1073/pnas.2003361117
Manu, M., Băncilă, R. I., Bîrsan, C. C., Mountford, O., and Onete, O. (2021). Soil mite communities (Acari: Mesostigmata) as indicators of urban ecosystems in Bucharest, Romania. Sci. Rep. 11, 3794. doi:10.1038/s41598-021-83417-4
Markkula, I., Cornelissen, J. H. C., and Aerts, R. (2019). Sixteen years of simulated summer and winter warming have contrasting effects on soil mite communities in a sub-Arctic peat bog. Polar Biol. 42, 581–591. doi:10.1007/s00300-018-02454-4
Meehan, M. L., Barreto, C., Turnbull, M. S., Bradley, R. L., Bellenger, J. P., Darnajoux, R., et al. (2020). Response of soil fauna to simulated global change factors depends on ambient climate conditions. Pedobiologia 83, 150672. doi:10.1016/j.pedobi.2020.150672
Meehan, M. L., Song, Z., Lumley, L. M., Cobb, T. P., and Proctor, H. (2019). Soil mites as bioindicators of disturbance in the boreal forest in northern Alberta, Canada: Testing taxonomic sufficiency at multiple taxonomic levels. Ecol. Indic. 102, 349–365. doi:10.1016/j.ecolind.2019.02.043
Melekhina, E. N., Belykh, E. S., Markarova, M. Y., Taskaeva, A. A., Rasova, E. E., Baturina, O. A., et al. (2021). Soil microbiota and microarthropod communities in oil contaminated sites in the European Subarctic. Sci. Rep. 11, 19620. doi:10.1038/s41598-021-98680-8
Milano, V., Cortet, J., Baldantoni, D., Bellino, A., Dubs, F., Nahmani, J., et al. (2017). Collembolan biodiversity in mediterranean urban parks: Impact of history, urbanization, management and soil characteristics. Appl. Soil Ecol. 119, 428–437. doi:10.1016/j.apsoil.2017.03.022
Minayeva, T. Y., Bragg, O. M., and Sirin, A. A. (2017). Towards ecosystem-based restoration of peatland biodiversity. Mires Peat 19, 1–36. doi:10.19189/MaP.2013.OMB.150
Nie, M., Bell, C., Wallenstein, M. D., and Pendall, E. (2015). Increased plant productivity and decreased microbial respiratory C loss by plant growth-promoting rhizobacteria under elevated CO2. Sci. Rep. 5, 9212. doi:10.1038/srep09212
Norby, R. J., Childs, J., Hanson, P. J., and Warren, J. M. (2019). Rapid loss of an ecosystem engineer: Sphagnum decline in an experimentally warmed bog. Ecol. Evol. 9 (22), 12571–12585. doi:10.1002/ece3.5722
Oksanen, J., Blanchet, F. G., Friendly, M., Kindt, R., Legendre, P., McGlinn, D., et al. (2019). vegan: Community ecology package. The R Project for Statistical Computing. Available at: https://www.mcglinnlab.org/publication/2019-01-01_oksanen_vegan_2019/.
O’Lear, H. A., and Blair, J. M. (1999). Responses of soil microarthropods to changes in soil water availability in tallgrass prairie. Biol. Fert. Soils 29, 207–217. doi:10.1007/s003740050546
Peng, Y., Peñuelas, J., Vesterdal, L., Yue, K., Peguero, G., Fornara, D. A., et al. (2022). Responses of soil fauna communities to the individual and combined effects of multiple global change factors. Ecol. Lett. 25, 1961–1973. doi:10.1111/ele.14068
Pinheiro, J. C., and Bates, D. M. (2000). Mixed-effects models in S and S-plus. New York: Springer. doi:10.1007/b98882
R Core Team (2020). Computing, R: A language and environment for statistical computing. R Foundation for Statistical. Available at: https://www.eea.europa.eu/data-and-maps/indicators/oxygen-consuming-substances-in-rivers/r-development-core-team-2006.
Rillig, M. C., Ryo, M., Lehmann, A., Aguilar-Trigueros, C. A., Buchert, S., Wulf, A., et al. (2019). The role of multiple global change factors in driving soil functions and microbial biodiversity. Science 366, 886–890. doi:10.1126/science.aay2832
Roos, R. E., Birkemoe, T., Asplund, J., Ľuptáčik, P., Raschmanová, N., Alatalo, J. M., et al. (2020). Legacy effects of experimental environmental change on soil micro-arthropod communities. Ecosphere 11, e03030. doi:10.1002/ecs2.3030
Rydin, H., and Jeglum, J. K. (2006). “The Biology of peatlands,” in Biology of habitats. 1st ed (Oxford Academic, Online edition). doi:10.1093/acprof:oso/9780198528722.001.0001
Sebestyen, S. D., Dorrance, C., Olson, D. M., Verry, E. S., Kolka, R. K., Elling, A. E., et al. (2011). Long-term monitoring sites and trends at the Marcell experimental Forest in Peatland biogeochemistry and watershed hydrology at the Marcell experimental forest. Editors R. K. Kolka, S. D. Sebestyen, E. S. Verry, and K. N. Brooks (Boca Raton, Florida: CRC Press), 16–71.
Silvan, N., Laiho, R., and Vasander, H. (2000). Changes in mesofauna abundance in peat soils drained for forestry. For. Ecol. Manag. 133, 127–133. doi:10.1016/S0378-1127(99)00303-5
Sławski, M., Stebel, A., and Sławska, M. (2022). Spontaneous regeneration of Collembola assemblages in a raised bog after human-induced disturbance. Appl. Soil Ecol. 169, 104233. doi:10.1016/j.apsoil.2021.104233
Slowikowski, K., Schep, A., Hughes, S., Dang, T. K., Lukauskas, S., Irisson, J. O., et al. (2021). Automatically position non-overlapping text labels with “ggplot2. RDRR. Available at: https://ggrepel.slowkow.com/authors.html#citation.
Stuble, K. L., Ma, S., Liang, J., Luo, Y., Classen, A. T., and Souza, L. (2019). Long-term impacts of warming drive decomposition and accelerate the turnover of labile, not recalcitrant, carbon. Ecosphere 10, e02715. doi:10.1002/ecs2.2715
Tsiafouli, M. A., Kallimanis, A. S., Katana, E., Stamou, G. P., and Sgardelis, S. P. (2005). Responses of soil microarthropods to experimental short-term manipulations of soil moisture. Appl. Soil Ecol. 29, 17–26. doi:10.1016/j.apsoil.2004.10.002
Turnbull, M. S., and Lindo, Z. (2015). Combined effects of abiotic factors on Collembola communities reveal precipitation may act as a disturbance. Soil Biol. Biochem. 82, 36–43. doi:10.1016/j.soilbio.2014.12.007
Vestergård, A. M., Dyrnum, K., Michelsen, A., Damgaard, C., and Holmstrup, M. (2015). Long-term multifactorial climate change impacts on mesofaunal biomass and nitrogen content. Appl. Soil Ecol. 92, 54–63. doi:10.1016/j.apsoil.2015.03.002
Wickham, H. (2016). ggplot2: elegant graphics for data analysis. Springer. Available at: https://cran.r-project.org/web/packages/ggplot2/citation.html.
Wierzbicka, A., Dyderski, M. K., Kamczyc, J., Rączka, G., and Jagodziński, A. M. (2019). Responses of soil mite communities (Acari: Oribatida, Mesostigmata) to elemental composition of mosses and pine needles and long-term air pollution in Scots pine (Pinus sylvestris L.) stands. Sci. Total Environ. 691, 284–295. doi:10.1016/j.scitotenv.2019.07.138
Wilson, R. M., Tfaily, M. M., Kolton, M., Johnston, E. R., Petro, C., Zalman, C. A., et al. (2021). Soil metabolome response to whole-ecosystem warming at the Spruce and peatland responses under changing environments experiment. PNAS 118 (25), e2004192118. doi:10.1073/pnas.2004192118
Yang, G., Ryo, M., Roy, J., Lammel, D. R., Ballhausen, M., Jing, K., et al. (2022). Multiple anthropogenic pressures eliminate the effects of soil microbial diversity on ecosystem functions in experimental microcosms. Nat. Commun. 13, 4260. doi:10.1038/s41467-022-31936-7
Yin, R., Kardol, P., Thakur, M. P., Gruss, I., Wu, G., Eisenhauer, R., et al. (2020). Soil functional biodiversity and biological quality under threat: Intensive land use outweighs climate change. Soil Biol. Biochem. 147, 107847. doi:10.1016/j.soilbio.2020.107847
Keywords: climate change, boreal zone, microarthropods, community ecology, bog, wetland
Citation: Barreto C, Conceição PHS, de Lima ECA, Stievano LC, Zeppelini D, Kolka RK, Hanson PJ and Lindo Z (2023) Large-scale experimental warming reduces soil faunal biodiversity through peatland drying. Front. Environ. Sci. 11:1153683. doi: 10.3389/fenvs.2023.1153683
Received: 29 January 2023; Accepted: 06 April 2023;
Published: 20 April 2023.
Edited by:
Balázs Deák, Hungarian Academy of Science, HungaryReviewed by:
Borbála Szabó, University of Bremen, GermanyMalak M. Tfaily, University of Arizona, United States
Copyright © 2023 Barreto, Conceição, de Lima, Stievano, Zeppelini, Kolka, Hanson and Lindo. This is an open-access article distributed under the terms of the Creative Commons Attribution License (CC BY). The use, distribution or reproduction in other forums is permitted, provided the original author(s) and the copyright owner(s) are credited and that the original publication in this journal is cited, in accordance with accepted academic practice. No use, distribution or reproduction is permitted which does not comply with these terms.
*Correspondence: Carlos Barreto, Y2JhcnJldG9AdXdvLmNh
†Present address: Carlos Barreto, Ontario Forest Research Institute, Ministry of Natural Resources and Forestry, Sault Ste. Marie, ON, Canada