- Department of Integrative Biology, University of Guelph, Guelph, ON, Canada
Nitrogen (N) and phosphorus (P) are fundamental for plant biomass production in grasslands, are often co-limiting, and have become major freshwater pollutants. By factorially applying gradients of N and P to field-based grassland mesocosms, we tested for saturating thresholds of plant uptake as nutrients increase and whether simultaneous and potentially additive growing-season demand reduces flows of dissolved nutrients to subsurface leachate. We quantified the seasonality of nutrient losses, differences in uptake by functional group (grasses, forbs), the impacts of increasing nutrients on root:shoot ratios, and contrasted vegetated and unvegetated treatments to isolate edaphic influences. Overall, most added nutrients were retained by plants and soil–80% for N and 99% for P. Co-limitation dynamics were powerful but asymmetrical with N additions reducing P in leachate, but P having little influence on N. N retention was primarily influenced by season—most N was lost prior to peak biomass when plant demand was presumably lower. Nutrients reduced root:shoot ratios by increasing foliage but with no detectable effect on retention, possible because root biomass remained unchanged. Similarly, there was no impact of functional group on nutrient loss. Despite substantial plant uptake, leachate concentrations of N and P still exceeded regional levels for safe drinking water and prevention of algal blooms. This work reveals how nutrient co-limitation can accelerate the capture of P by N in grasslands, indicating that plant uptake can significantly reduce dissolved subsurface nutrients. However, the offseason flows of N and the failure to meet regional water-quality standards despite capture levels as high as 99% reveal that vegetative-based solutions to nutrient capture by grasslands are important but likely insufficient without complimentary measures that reduce inputs.
Introduction
Nutrient retention by plants is a critical ecosystem function, especially given dramatic anthropogenic increases in the inputs of nitrogen (N) and phosphorous (P) (Cordell et al., 2009; Stevens et al., 2015). This includes agricultural fertilization, which has increased crop yields in the past century but with accelerating environmental impacts (Sobota et al., 2015; McCann et al., 2021). The sole intent of fertilization is to trigger yield increases in crops, but a “retention gap” often occurs where upwards of 30%–50% of annually applied soluble inorganic fertilizer can be lost to leaching or volatilization (Bouwman et al., 2002; Good and Beatty, 2011; Mueller et al., 2012). This gap has resulted in N and P becoming major global pollutants, driven in part by their yearly addition to often nutrient-saturated farm soils (Drinkwater and Snapp, 2007). Reducing nutrient losses on farms will require a more complete understanding of the capacity for maximum plant uptake, by crops and by downslope amendments such as permanent-cover buffers (Blann et al., 2009; Noble et al., 2023).
The retention of N and P by plant uptake is often stoichiometrically regulated—they are required in combination and in different ratios, and are typically co-limiting such that the availability of one affects the uptake of the other (Elser et al., 2007; Harpole et al., 2011; Li et al., 2016). As such, the maximum capacity for plants to retain nutrients on farms may be determined in part by the availability of one or both nutrients (Greenwood et al., 2008; Hou et al., 2020). The power of N-P co-limitation for plant growth has been illustrated in global grasslands, with N-P additions on five continents increasing annual biomass by 48% compared to N alone (21% increase) and P alone (no detectable impact) (Carroll et al., 2022). This co-dependency suggests the potential for synergistic uptake, where their combined addition (as typically occurs in agriculture) triggers higher retention than would occur by adding one or the other in isolation. A challenge for maximizing co-dependent uptake, however, is that the magnitude and timing of uptake can vary widely by seasonal life stage (Niklas et al., 2005; Li et al., 2016; Deng et al., 2017; Jiang et al., 2019). Unfortunately, most farm nutrients are applied in single early-season pulses when plant demand is low. As well, uptake at peak biomass can be constrained in nutrient-saturated soils because resource limitations often shift to water, light, or other nutrients—declining root:shoot ratios may be associated with less nutrient demand (Harpole et al., 2017; Cleland et al., 2019). Given these complexities, the occurrence of “retention gaps” on farms is unsurprising, with more efficient and possibly complete utilization of soil nutrients by plants being dependent on the temporal dynamics of stochiometric uptake that can be difficult to determine (e.g., Penuelas et al., 2012).
Here, we use field-based grassland mesocosms to test these issues, exploring resource co-limitation, plant uptake, and the retention of nutrients from entering subsurface leachate. Foremost we measure nutrient loss in leachate across factorial gradients of added N and P, using application rates comparable to intensive agriculture (range: 0–20 g m−2). We quantify whether co-limitation impacts on retention are explicitly related to how N and P combine to affect overall plant production, on allocation to roots versus shoots, on whether N and P demands vary seasonally based on reductions of nutrient loads in leachate, and whether community composition (7 species of grasses, 7 species of forbs including 4 legumes, or a 14 species mix of both) alters these outcomes. We partner these treatments with unvegetated mesocosms with or without nutrients, to untangle background effects of soil processes on N and P flow rates (Burwell et al., 1977; Noble et al., 2023). Finally, we explore management implications, focusing on how early-season application, temporal patterns of biomass production by grassland, and species composition variously shape leachate concentrations, relative to established groundwater standards derived to protect drinking water and prevent algal blooms.
Materials and methods
Experiment design
Two hundred grassland mesocosms were established in 2018 with differing plant communities (4 levels including unvegetated) and nutrient additions (10 levels) at the rare Charitable Research Reserve (Cambridge, ON, Canada; 43 22 N, 80 21 W). Mesocosms were established in 19 L plastic buckets designed to capture leachate from the experimental plant communities (Supplementary Figure S1). A drainage spout was installed using a 50 cm 5/8″ inner diameter hose at the base of each mesocosm. These mesocosms were filled 30 cm of soil on top of a 5 cm layer of 3/4” inch aggregate stone to facilitate drainage. Sieved soil was acquired from the top 20 cm of an agricultural field, retired in 2007 from long-term corn-soy-wheat rotations (Harvey and MacDougall, 2014; 2015; 2018). After 2007, succession happened naturally, and the site is now characterized by oldfield taxa especially Solidago, Trifolum, and Symphyotrichum spp. The soils are well-drained luvisolic St Jacobs Loams (Agriculture and Agri-Food Canada, 2004). Filled mesocosms were arranged so that the drainage hose fed via gravity into a hand-dug hole (Supplementary Figure S1). Collection bags were attached to the end of each drainage hose.
Mesocosms were seed planted on 5 June 2018, with one of three randomly allocated experimental grassland communities plus a no-plant control. Grassland communities were all grasses (7 species: Bromus inermis, Dactylis glomerata, Echinochloa esculenta, Lolium multiflorum, Lolium perenne, Phleum pratense, Sorghum drummondii), all grassland forbs including legumes (7 species: Achillea millefolium, Brassica napus, Brassica oleracea, Medicago sativa, Trifolium hybridum, Trifolium pratense, Trifolium repens), or a 14-species combination of both (referred to as “both” for brevity in the figures). The unvegetated control treatment was unplanted. Mesocosms were hand weeded to maintain community composition. After planting, all mesocosms were watered daily for 2 weeks including the unvegetated controls.
Ten nutrient treatments began on 19 June 2018 once seeds had germinated. The treatments had variable N levels with constant P (0, 5, 10, or 15 g N m−2; 20 g P m−2), variable P levels with constant N (20 g N m−2; 0, 5, 10, or 15 g P m−2), both N and P at high levels (20 g N m−2; 20 g P m−2), or control levels with no N and P added (0 g N m−2; 0 g P m−2). The gradients of nutrient addition were designed to encapsulate i) recommended rates for crop fertilization [16.2 g N m−2 for corn (Ontario Ministry of Agriculture Food and Rural Affairs, 2018)], ii) levels of N and P commonly used in ecological nutrient studies [10 g N m−2 and 10 g P m−2 (Borer et al., 2017)], and iii) to maintain symmetrical levels of N and P addition. Fertilizer was added as urea (N) or triple super phosphate (P). After fertilizing, mesocosms were only watered to ensure plant survival. This was necessary in July which experienced a period without precipitation (Figure 1). As before, all mesocosms including the no-plant controls were equally watered. All watering events during this frame were adjusted to ensure that no water would percolate through the entire mesocosm and be lost as leachate. Treatments with plants (all grasses, all forbs, grasses and forbs) had 2 replicates per each nutrient treatment across 3 blocks for a total of 180 mesocosms (3 vegetated treatments X 10 nutrients treatments X 2 replicates X 3 blocks = 180). Treatments without plants had 2 replicates per nutrient treatment within only 1 block for a total of 20 mesocosms (180 + 20 = 200 total).
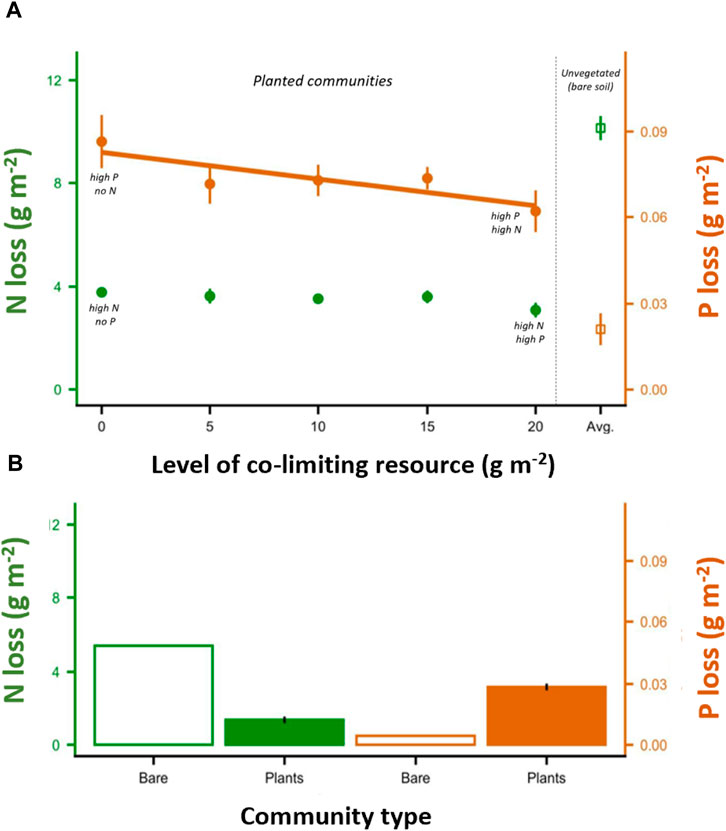
FIGURE 1. Nutrient loss with shifting levels of co-limiting resources, for high P with increasing levels of N and for high N with increasing levels of P. For P, increasing levels of N reduces P levels in subterranean leachate (A). In contrast, N levels in leachate are unaffected by increased levels of P addition (A). For unvegetated mesocosms, N losses are significantly higher compared to vegetated mesocosms, indicating the importance of plant uptake on N (A,B). Unexpectedly, P levels in leachate were lower in mesocosms lacking vegetation (A,B). Error bars represent = 1 SE, and trendlines show significant linear relationships. In (A), N = 9 for mesocosms with plants, and N = 5 for bare soil mesocosms. In (B), N = 9 for mesocosms with plants, and N = 1 for bare soil.
Leachate sampling and processing
Leachate collection began starting 19 June 2018 and occurred three times, approximately every 3 weeks (Figure 1). Collections encompassed i) 19 June–10 July (21 days, 9.5 cm ambient precipitation), ii) 10 July–5 August (26 days, 8.0 cm), and iii) 5 August–22 August (17 days, 21.1 cm). Leachate was processed by lowering the pH to 2.0 with hydrochloric acid to preserve the sample until processing, and then refrigerated up to 48 h until analysis.
Leachate was analyzed for total N and total P at SGS Agri-Food Laboratories (Guelph, ON, Canada). Nitrogen was determined by NO—cadmium reduction (Standard Methods, 2006) and Kjeldahl N (Standard Methods, 2005) to 0.05 mg/L. In some instances, the level
Of N present in the samples was below the detectable level of 0.5. In these cases, N was recorded
As 0 mg/L. Total P was determined by inductively coupled plasma—mass spectrometry (U.S. EPA. 1994) to 0.003 mg/L. Below the detection limit, P was recorded as 0 mg/L.
Total N and total P leached throughout the experiment was approximated by taking the product of each collection period’s leachate nutrient concentrations by the maximum volume of leachate collected within the collection period. There was no difference in mean volume of leachate collected between the 4 communities at any collection date, and there was high correlation between the mean and maximum volume collected (r = 0.97). Given that this experiment was not designed to precisely measure the volume of leachate, but rather the nutrient concentrations, the maximum volume at each date is used to reduce any error associated with spillage of leachate in transportation to the laboratory. Statistical significance remained unchanged regardless if volume mean or max was used, or if total potential leachate (based on rainfall amount during each collection period) was used. This value is equivalent to a “loadings” basis to facilitate comparisons between this experiment and water quality reports and recommendations.
Biomass harvesting
At the conclusion of the experiment, above- and below-ground biomass were harvested separately to obtain the aboveground: belowground ratio (AG: BG). The aboveground fraction was clipped at the soil surface, oven dried at 40°C for 48 h and weighed. Roots were collected by passing the entire mesocosm’s soil through a sieve (1 mm diameter), after which collected roots were washed free of dirt, oven dried, and weighed. Biomass measurements are g per mesocosm, which is equivalent to g 0.07 m−2.
Soil and plant chemistry
On a subset of the treatments (treatments = 0 g N m−2 + 0 g P m−2; 0 g N m−2 + 20 g P m−2, 20 g N m−2 + 0 g P m−2, 20 g N m−2 + 20 g P m−2), two soil cores (2.5 cm diameter, 10 cm depth) were collected at the conclusion of the experiment. Cores were stored at 4°C until analysis, sieved to remove roots and plant biomass, air dried, and homogenized using a coffee grinder. After processing, soil available P was determined using the Mehlich 3 procedure (Frank et al., 2012). Aboveground biomass collected from the same subset of treatments was homogenized using a Wiley Mill, after which we used a sulfuric acid digestion and determined foliar % P colorimetrically with ammonium molybdate (Ogdahl et al., 2013).
Statistical analysis
Analyses were performed at the block-level, averaging over two replicate mesocosms for a total n = 100 (90 experimental, 10 no-plant controls). Averaging was necessary to account for missing data, particularly during the second leachate collection time point during which multiple mesocosms did not produce leachate.
Analyses were performed on two different subsets of the 10 experimental fertilizer levels. The “variable N” experiment consisted of plots where N fertilizer levels varied (0, 5, 10, 15, 20 g N m−2) and P fertilizer was held constant (always at 20 g P m−2). The “variable P” experiment consisted of plots where P fertilizer levels varied (0, 5, 10, 15, 20 g P m−2) and N fertilizer was held constant (always at 20 g N m−2). Treatments where no N nor P was added were used as a comparative measure, similarly with plots with a no-plant addition control.
For the leachate analysis in mesocosms grown with plants, concentration of N and P at each time point was analyzed in a 2-way ANOVA where community type and fertilizer treatment were fixed factors. Above- and below-ground biomass and their ratio were also evaluated within the same 2-way ANOVA. Significance of all factors was evaluated with Type II tests using the Anova function in the car package (Fox and Weisberg, 2011). When there was a significant community type × nutrient interaction, separate linear models evaluating the effect of the variable nutrient on a response variable were run each community type. When there was a significant, main effect of community type, Tukey’s post-hoc tests were run evaluating the response variable against the 3 community types.
We used a piecewise structural equation modeling (SEM) approach to assess the importance of biomass and leachate responses to fertilizer addition for each community type. Given that biomass production could play both explanatory and response roles, we used piecewise SEMs to disentangle the role of each variable (Grace et al., 2010). Specifically, we used the piecewiseSEM package (Lefcheck, 2016) to investigate how added N and P impacts nutrient retention via direct (fertilizer rate) and indirect (mediated by biomass uptake) pathways. We tested if community composition significantly varied, and finding a better association with an unconstrained model, we fitted unique models to each community type. In all cases, the initial model included the following links: 1) Total N leached is affected by N fertilizer rate, biomass production, and above-:belowground ratio, 2) Total P leached is affected by P fertilizer rate, biomass production, and above-:Belowground ratio, 3) biomass production is affected by N and P fertilizer rates 4) above-:Belowground ratio is affected by N and P fertilizer rates. Importantly, our initial model did not include links between N addition and P loss, or P addition and N loss, because we had no a priori expectation, or any biological hypothesis, that would directly link these outcomes. After specifying our initial model, we refined our model by dropping non-significant links (p < 0.05) in a stepwise fashion beginning with the least significant until the 𝚫AICc between subsequent models was <2. Final model fits indicated good fit with the data for all communities (grasses: Fisher’s C = 13.52, p = 0.484; forbs: Fisher’s C = 10.5, p = 0.398; both: Fisher’s C = 16.74, p = 0.403) where p-value >0.05 indicates good model fit (Shipley, 2009). The relative importance of direct effects is given by standardized coefficients for each path, and indirect effects can be determined by multiplying standardized coefficients.
Data analyses were performed using R version 3.5.1 (R Core Team, 2018). Leachate levels were compared with regional standards for standing non-running surface water (Canada, 2004), of 10 ppm (comparable to 10 mg L−1) for N and 0.02 ppm (comparable to 0.02 mg L−1) for P. Both have been shown to be minimum thresholds for triggering significant impacts on drinking water quality and aquatic processes including algal blooms (see Noble et al., 2023).
Results
Regulation of N loss by treatment
Overall N loss was not influenced by P additions (Figure 1; Supplementary Table S1). Temporally, nearly all N loss occurred before grassland plants had reached maturity (Figure 2). The period of greatest N loss was during the first month (average 105.0 mg L−1 in leachate across all treatments with 20 g N m−2 added—Figure 2). During this period, we did see evidence of co-limitation dynamics where P additions slightly reduced N losses (Figure 2; Supplementary Table S1), but the effect was not sufficiently strong to influence overall N loss (Figure 1; Supplementary Table S1). Following the first month, leaching losses of N dropped (Figure 2, average 0.5 mg L−1 loss for both the second and third month). During the second month, we saw that communities dominated by grasses had less leaching than communities with both grasses and forbs (Supplementary Table S1, Tukey’s post-hoc, p = 0.046). During the third month, adding P actually increased N leaching losses (Supplementary Table S1, linear post-hoc p = 0.009). The nearly undetectable levels of N leaching during the second and third months, however, left the total N leaching losses unaffected by adding P or manipulating community composition (Supplementary Table S1). Supplemental results show that greater N additions meant more N loss overall, but the magnitude of this effect was much smaller than the overall temporal patterns linked to plant development and uptake (Supplementary Figure S2A; Supplementary Table S1). Bare soil mesocosms lost more N than did any treatment with plants present (Figure 1). N loss occurred even in mesocosms where no N was applied (∼2 g m−2 total throughout the experiment; Figure 1B, Figure 2A).
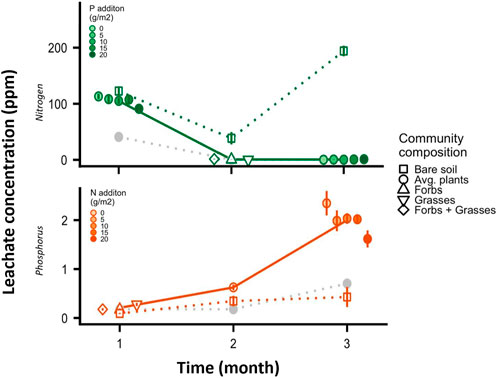
FIGURE 2. Average concentration by time of nitrogen and phosphorus in leachate, for N losses with increasing P addition and P losses with increasing N addition. Temporal patterns for bare soil mesocosms (square points) and nutrient control mesocosms (grey points, 0 g N m−2 and 0 g P m−2) are shown for comparative purposes. Error bars = 1 SE, and trendlines show the average trend in nutrient losses for the focal mesocosms (solid line) as well as the bare soil and nutrient controls (dashed). Most N was lost early in the year prior to peak plant growth, regardless of P levels. Most P was lost later in the growing season, when mesocosm biomass was at its peak.
Regulation of P loss by treatment
Overall P leaching was reduced by N additions (Supplementary Table S1; Figure 1A, brown points). This trend emerged after the first month (Supplementary Table S1). Temporally, P loss accelerated throughout the growing season (Figure 2B). During the first month, we found that grass communities had higher P losses than those with only forbs (Supplementary Table S1, Tukey’s post-hoc p = 0.027) or with grasses and forbs growing together (Tukey’s post-hoc p = 0.038). Supplemental results show that adding more P meant greater P loss (Supplementary Figure S2B; Supplementary Table S1). Unexpectedly, bare soil mesocosms lost less P than did any treatment even with plants present—rather than retaining P by plant uptake, the presence of vegetation increased P loss to leachate (Figure 1).
Functional groups and root:shoot allocation with nutrients
There was no overall effect of functional group on nutrient loss across our three experimental plant communities, although vegetation (compared to bare soil controls) consistently reduced N losses and facilitated P losses (Figure 1). The different functional groups did vary in the magnitude to which biomass production and allocation patterns mechanistically influenced overall nutrient leaching (Figure 3). In grass communities, N additions increased plant biomass that subsequently led to higher P demand and less P lost in leachate (Figure 3). In contrast, N additions had little direct effect on forb biomass production, and therefore did not influence overall P leaching (Figure 3). In all cases, fertilizer application shifted allocation towards greater relative investment in shoots (Figure 3). While relative reduced root allocation was a consequence of N addition, this did not result in increased N leaching likely because total root biomass did not change—changes to root:shoot ratios strictly involved changes in foliar biomass.
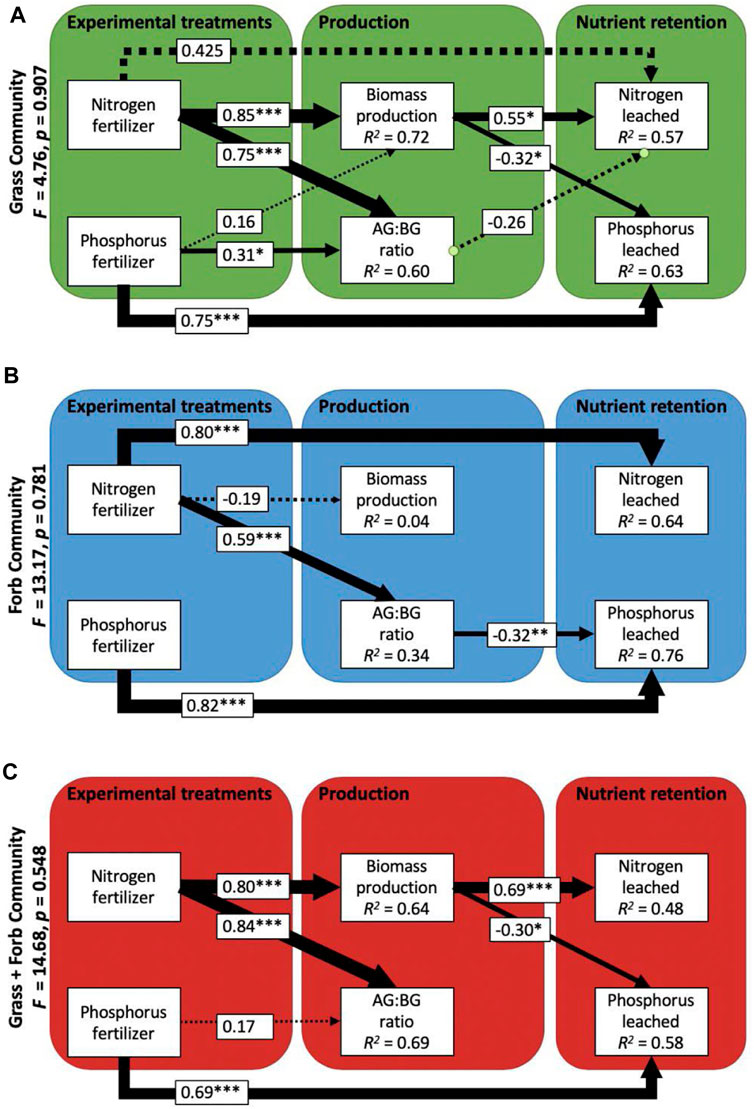
FIGURE 3. Best-fit path models showing the links between experimental N and P additions, biomass production, ratios between above and belowground biomass (AG: BG), and amounts of N and P leached within the grass (A), forb (B) and grass + forb (C) communities. Arrow thickness is proportional to the standardized path coefficients, with the directionality and size given within boxes along the arrow. Asterisks indicate significance level of linkages (*<0.05, **<0.01, ***<0.001), and dashed lines are used when significance of paths is >0.05. R2 values are given within the boxes containing endogenous variables.
Discussion
Overall, we observed significant retention of nutrients in mesocosm grassland, based on nutrients added as a one-time early-season input comparable to typical agricultural management. Nutrient concentrations detected in leachate during the growing season revealed the capture of ∼80% added N and ∼99% retention of added P. The mechanisms of retention, however, differed substantially and involved both vegetative and soil-based processes. First, the interaction between N and P were highly asymmetric—N additions reduced P leaching but there was no reciprocal effect of P additions on N loss. Second, there were significant temporal influences on both nutrients but in the opposite direction: nearly all N loss occurred before the mesocosm grassland had reached maturity while P loss was highest at peak biomass. Finally, we observed proportional linkages between higher application rates and greater leaching losses, especially for N. There was no overall effect of functional group among our three experimental plant communities. In total, our work shows that terrestrial nutrient retention can be immediately increased by establishing permanent vegetation cover and leveraging co-limitation dynamics to maximize biomass production–plants reduced N leaching to negligible levels as they matured, with N-driven biomass growth creating greater P demands and therefore reduced P leaching. On the other hand, despite these trends, nutrient concentrations in leachate still exceeded regional standards for water quality (even with ∼99% capture of P). These results strongly suggest that nutrient uptake by grasslands, while highly effective (e.g., planted riparian grassland buffers), is unlikely to stem nutrient losses in conventional agriculture, without complementary measures that reduce the amounts added and adjust the time of application towards periods of peak plant activity.
We observed strikingly different models of nutrient retention for N and P, both functionally and temporally. For N, plant maturity was the largest factor controlling retention, such that low plant biomass early in the growing season coincided with the greatest losses to leachate. Early season leachate concentrations for N, sometimes exceeding 105 mg L−1, were well above recommended guidelines for safe drinking water (<10 mg L−1) (Canada, 2019). Following the first month, leaching losses of N dropped to negligible levels (average 0.5 mg L−1 loss for both) in accordance with previous work showing that inorganic N is readily taken up by phenologically active plants (Hooper and Vitousek, 1998).
During the second month, we saw that communities dominated by grasses had slightly lower leaching than communities with both grasses and forbs, potentially because grasses may have had faster root biomass production than forbs although this was not tested. The nearly undetectable levels of N leaching during the second and third months, however, left the total N leaching losses unaffected by adding P or community composition. Our soils do not appear to be strongly P limited given that P addition did not significantly increase biomass production, so perhaps it is not surprising that P additions did not reduce N loss. The ability of plants to uptake N, and therefore reduce leaching losses throughout a growing season, was contrasted by the patterns seen in the bare soil mesocosms. Not surprisingly, fertilized bare soil lost up to 2.5 x more N than any treatment with plants present.
Temporally, total N loss corresponded in part with precipitation, with the first and third collection dates associated with appreciably more rainfall than the second (Supplementary Figure S3). This observation aligns with previous work showing that precipitation pulses can facilitate nutrient movement during the growing season (Congreves et al., 2016; Bowles et al., 2018; Noble et al., 2023). Finally, N loss to leachate occurred even in mesocosms where no N was applied. Soil used in this experiment had been farmed and fertilized for decades until 2007, suggesting nutrient legacies given that nutrient cessation occurred more than a decade prior to our experiment (see also Mazzorato et al., 2022). They may also reflect N loss associated with microbial decomposition (e.g., Craine et al., 2007), although we did not quantify N volatilization rates. Regardless, these results for N loss suggest that retention is best maximized by the presence of mature, phenologically active vegetation.
For P, in nearly all cases including both vegetated and unvegetated mesocosms, retention was at least 99% based on the difference between levels of fertilization and levels measured in leachate. This finding supports previous reports of P being typically less mobile than N via binding to soil (Burwell et al., 1977; Noble et al., 2023). However, three notable findings emerged from our work, despite the high levels of retention for P. Foremost, we found that overall P loss was reduced by N additions suggesting strong nutrient co-limitation. There were lower levels of P remaining in the soil at the conclusion of the experiment when N was added (Supplementary Figure S4), showing that N additions further limit P leaching losses. Mechanistically, more plant biomass (driven by N additions) likely meant higher demand, and thus uptake, for P. Alternatively, N additions have been shown to increase root and soil phosphatase activity (Marklein and Houlton 2012; Deng et al., 2017), which can also facilitate plant P uptake although we did not evaluate enzyme production here. While foliar plant %P was reduced with added N (Supplementary Figure S5), accounting for additional biomass production meant that P held in plant tissues was still greater when N was added. The temporal signature of P loss reinforces that biomass production demands P, and compliments previous findings of widespread N and P co-limitation on primary production (Elser et al., 2007; Harpole et al., 2017; Carroll et al., 2022). It also provides new evidence that co-limitation dynamics can impact nutrient retention in nutrient-rich systems.
Second, we found that plants appeared to facilitate small amounts of P loss based on comparisons with bare-soil mesocosms. Given the sensitivity of P solubility to pH (e.g., Penn and Camberato, 2019), one possible explanation is the acidification of the rhizosphere by plant activity. Plant-derived acidification cans occur by a range of possible mechanisms, including ammonium uptake, root exudation, and oxidization (Hinsinger, 2001). Consequentially, this may lead to greater P loss later once plants have developed root systems, an effect that may be magnified in the relatively confined area of the mesocosm containers (Supplementary Figure S1). This effect also appears to vary by vegetation type. During the first month, we found that grasses had higher P losses than communities grown with only forbs or with grasses and forbs, potentially because grass dominated communities established roots more quickly. An alternative possibility for unexpectedly high flows of P to subsurface water is macropores, which can form on clay-dominated soils during periods of summer drought (Noble et al., 2023). However, none were observed in our mesocosms.
Third, regardless of P retention approaching 100% the quantity of P leached from the system still exceeded levels necessary to maintain uncontaminated freshwater based on a threshold of 0.02 mg L−1 (Canada, 2004). This was true even when supplemental P was not added to the mesocosms—during the third month, P averaged 2.0 mg L−1 in leachate with fertilization and 0.60 mg L−1 without. While surface flows and sediment loss are the primary mechanisms by which P moves from terrestrial to aquatic systems (Gaynor and Findlay, 1995), these results show that P loss via leaching pathways cannot be disregarded as a threat to water quality (Noble et al., 2023). Given that we saw that adding more P fertilizer meant more P loss (Supplementary Figure S1B), we conclude that P leaching can be lowered by reducing P inputs.
The strong connection between plant growth and nutrient uptake is to be expected, given the need for both N and P for photosynthesis. However, we did not observe higher retention with greater functional group richness despite predictions that niche complementarity may elevate community-level nutrient foraging (Tilman et al., 1997; Cardinale et al., 2011). The greatest driver of vegetative-based retention was whether the plant community was present or absent, with the functional composition of our three communities being relatively unimportant. We did see some limited trends relating the presence of grasses or forbs. Communities grown with grasses had stronger biomass responses to N fertilizer additions, which subsequently was linked to less P loss via greater plant demand and uptake for P. In contrast, N additions had little direct effect on forb biomass production, and therefore did not influence overall P leaching. The forb community contained several N fixing species, which may have contributed to the lower sensitivity of biomass production to added N (You et al., 2017). Fertilizer application also shifted allocation towards greater relative investment in shoots, would be expected as mineral nutrient additions increased (Bloom et al., 1985; Ziter and MacDougall, 2013; Borer et al., 2014; Cleland et al., 2019; Eskelinen et al., 2022). However, this did not result in increased N leaching possibly because root biomass remained unchanged.
To conclude, these results reveal the potential for herbaceous vegetation to capture large percentages on dissolved plant-available forms of N and P at shallow depths in soils (30 cm deep), including feedbacks where higher N triggers elevated uptake of P (Cooper et al., 2017). This is consistent with numerous studies showing the effectiveness of farm and forest buffers for nutrient capture (e.g., Blann et al., 2009; Weigelhofer et al., 2012; Noble et al., 2023). It also reveals a paradoxical dynamic where nutrient additions could potentially act to stem nutrient losses in some circumstances, at least for dissolved P (Yang et al., 2018). Our factorial isolation of the relative effects of vegetation and soil was helpful to isolate retention mechansims, given that non-vegetative factors can also seasonally affect nutrient flows (e.g., drought-soil interactions in summer). Our non-vegetative plots showed that higher N flows occurred even without N addition, while also showing unexpectedly lower flows with P compared to vegetated mesocosms. The former is consistent with findings of high influxes of dissolved nutrients into open waters when plants are seasonally inactive (Noble et al., 2023). Finally, we also observed that nutrient concentrations in leachate often exceeded regional water-quality standards even with P capture at 99%. Clearly, vegetation alone cannot solve nutrient losses on managed landscapes unless partnered with measures that better match peak uptake with the timing, amount, and ratio of added nutrients (Noble et al., 2023).
Data availability statement
The raw data supporting the conclusion of this article will be made available by the authors, without undue reservation.
Author contributions
All authors contributed to the study conception and design. EE, assisted by AM, conceived the ideas and designed methodology; EE collected and analysed the data; EE and AM co-wrote the manuscript. All authors contributed critically to the drafts and gave final approval for publication.
Acknowledgments
We thank Kristin Doherty, Bernal Arce, Lauren Janke, Daniel Noble, Brock Roth, and the rare Charitable Research Reserve. Support provided by the Food From Thought research program funded by a Canada First Research Excellence Fund to the University of Guelph.
Conflict of interest
The authors declare that the research was conducted in the absence of any commercial or financial relationships that could be construed as a potential conflict of interest.
Publisher’s note
All claims expressed in this article are solely those of the authors and do not necessarily represent those of their affiliated organizations, or those of the publisher, the editors and the reviewers. Any product that may be evaluated in this article, or claim that may be made by its manufacturer, is not guaranteed or endorsed by the publisher.
Supplementary material
The Supplementary Material for this article can be found online at: https://www.frontiersin.org/articles/10.3389/fenvs.2023.1144268/full#supplementary-material
References
Agriculture and Agri-Food Canada (2004). Canadian soil information system: National soil database. Canada: Agriculture and Agri-Food Canada.
Blann, K. L., Anderson, J. L., Sands, G. R., and Vondracek, B. (2009). Effects of agricultural drainage on aquatic ecosystems: A review. Crit. Rev. Environ. Sci. Tech. 39, 909–1001. doi:10.1080/10643380801977966
Bloom, A. J., Chapin, F. S., and Mooney, H. A. (1985). Resource limitation in plants - an economic analogy. Annu. Rev. Ecol. Syst. 16, 363–392. doi:10.1146/annurev.es.16.110185.002051
Borer, E. T., Grace, J. B., Harpole, W. S., MacDougall, A. S., and Seabloom, E. W. (2017). A decade of insights into grassland ecosystem responses to global environmental change. Nat. Ecol. Evol. 1, 0118. doi:10.1038/s41559-017-0118
Borer, E. T., Seabloom, E. W., Gruner, D. S., Harpole, W. S., Hillebrand, H., Lind, E. M., et al. (2014). Herbivores and nutrients control grassland plant diversity via light limitation. Nature 508, 517–520. doi:10.1038/nature13144
Bouwman, A. F., Boumans, L. J. M., and Batjes, N. H. (2002). Estimation of global NH3volatilization loss from synthetic fertilizers and animal manure applied to arable landsand grasslands. Glob. Biogeochem. Cycles 16, 8-1–8-14. doi:10.1029/2000gb001389
Bowles, T. M., Atallah, S. S., Campbell, E. E., Gaudin, A. C. M., Wieder, W. R., and Grandy, A. S. (2018). Addressing agricultural nitrogen losses in a changing climate. Nat. Sustain. 1, 399–408. doi:10.1038/s41893-018-0106-0
Burwell, R. E., Schuman, G. E., Heinemann, H. G., and Spomer, R. G. (1977). Nitrogen and phosphorus movement from agricultural watersheds. J. Soil Water Conservation 32, 226–230.
Canada, E. (2004). Canadian guidance framework for the management of phosphorus in freshwater systems. Ottawa: Environment Canada.Ecosystem health: Science-based solutions
Canada, G. (2019). Guidelines for Canadian drinking water quality: Guideline technical document-nitrate and nitrite. Canada: Agriculture and Agri-Food Canada.
Cardinale, B. J., Matulich, K. L., Hooper, D. U., Byrnes, J. E., Duffy, E., Gamfeldt, L., et al. (2011). The functional role of producer diversity in ecosystems. Am. J. Bot. 98 (3), 572–592. doi:10.3732/ajb.1000364
Carroll, O., Batzer, E., Bharath, S., Borer, E. T., Campana, S., Esch, E., et al. (2022). Nutrient identity modifies the destabilising effects of eutrophication in grasslands. Ecol. Letts 25, 754–765. doi:10.1111/ele.13946
Cleland, E. E., Lind, E. M., DeCrappeo, N. M., DeLorenze, E., Wilkins, R. A., Adler, P. B., et al. (2019). Belowground biomass response to nutrient enrichment depends on light limitation across globally distributed grasslands. Ecosystems 22, 1466–1477. doi:10.1007/s10021-019-00350-4
Congreves, K. A., Dutta, B., Grant, B. B., Smith, W. N., Desjardins, R. L., and Wagner-Riddle, C. (2016). How does climate variability influence nitrogen loss in temperate agroecosystems under contrasting management systems? Agric. Ecosyst. Environ. 227, 33–41. doi:10.1016/j.agee.2016.04.025
Cooper, R. J., Hama-Aziz, Z., Hiscock, K. M., Lovett, A. A., Dugdale, S. J., Sunnenberg, G., et al. (2017). Assessing the farm-scale impacts of cover crops and non-inversion tillage regimes on nutrient losses from an arable catchment. Agric. Ecosyst. Environ. 237, 181–193. doi:10.1016/j.agee.2016.12.034
Cordell, D., Drangert, J. O., and White, S. (2009). The story of phosphorus: Global food security and food for thought. Glob. Environ. Change-Human Policy Dimensions 19, 292–305. doi:10.1016/j.gloenvcha.2008.10.009
Craine, J. M., Morrow, C., and Fierer, N. (2007). Microbial nitrogen limitation increases decomposition. Ecology 88, 2105–2113. doi:10.1890/06-1847.1
Deng, Q., Hui, D. F., Dennis, S., Reddy, K. C., and Xu, X. (2017). Responses of terrestrial ecosystem phosphorus cycling to nitrogen addition: A meta-analysis. Glob. Ecol. Biogeogr. 26, 713–728. doi:10.1111/geb.12576
Drinkwater, L. E., and Snapp, S. (2007). Nutrients in agroecosystems: Rethinking the management paradigm. Adv. Agron. 92, 163–186. doi:10.1016/S0065-2113(04)92003-2
Elser, J. J., Bracken, M. E. S., Cleland, E. E., Gruner, D. S., Harpole, W. S., Hillebrand, H., et al. (2007). Global analysis of nitrogen and phosphorus limitation of primary producers in freshwater, marine and terrestrial ecosystems. Ecol. Lett. 10, 1135–1142. doi:10.1111/j.1461-0248.2007.01113.x
Eskelinen, A., Harpole, W. S., Jessen, M. T., Virtanen, R., and Hautier, Y. (2022). Light competition drives herbivore and nutrient effects on plant diversity. Nature 611, 301–305. doi:10.1038/s41586-022-05383-9
Fay, P. A., Prober, S. M., Harpole, W. S., Knops, J. M., Bakker, J. D., Borer, E. T., et al. (2015). Grassland productivity limited by multiple nutrients. Nat. Plants 1, 15080. doi:10.1038/nplants.2015.80
Fox, J., and Weisberg, S. (2011). An R companion to applied regression. second edition. Thousand Oaks, CA: Sage.
Frank, K., Beegle, D., and Denning, J. (2012). “Phosphorus. North central regional research publication No. 221 (revised),” in Recommended chemical soil test procedures for the North Central Region (United States: University Of Missouri).
Gaynor, J. D., and Findlay, W. I. (1995). Soil and phosphorus loss from conservation and conventional tillage in corn production. Am. Soc. Agron. Crop Sci. Soc. Am. Soil Sci. Soc. Am. 24, 734–741. doi:10.2134/jeq1995.00472425002400040026x
Good, A. G., and Beatty, P. H. (2011). Fertilizing nature: A tragedy of excess in the commons. Plos Biol. 9, e1001124. doi:10.1371/journal.pbio.1001124
Grace, J. B., Anderson, T. M., Olff, H., and Scheiner, S. M. (2010). On the specification of structural equation models for ecological systems. Ecol. Monogr. 80, 67–87. doi:10.1890/09-0464.1
Greenwood, D. J., Karpinets, T. V., Zhang, K., Bosh-Serra, A., Boldrini, A., and Karawulova, L. (2008). A unifying concept for the dependence of whole-crop N: P ratio on biomass: Theory and experiment. Ann. Bot. 102 (6), 967–977. doi:10.1093/aob/mcn188
Harpole, W. S., Ngai, J. T., Cleland, E. E., Seabloom, E. W., Borer, E. T., Bracken, M. E. S., et al. (2011). Nutrient co-limitation of primary producer communities. Ecol. Lett. 14, 852–862. doi:10.1111/j.1461-0248.2011.01651.x
Harpole, W. S., Sullivan, L. L., Lind, E. M., Firn, J., Adler, P. B., Borer, E. T., et al. (2017). Out of the shadows: Multiple nutrient limitations drive relationships among biomass, light and plant diversity. Funct. Ecol. 31, 1839–1846. doi:10.1111/1365-2435.12967
Harvey, E., and MacDougall, A. S. (2018). Non-interacting impacts of fertilization and habitat area on plant diversity via contrasting assembly mechanisms. Divers. Distributions 24 (4), 509–520. doi:10.1111/ddi.12697
Harvey, E., and MacDougall, A. S. (2015). Spatially heterogeneous perturbations homogenize the regulation of insect herbivores. Am. Nat. 186 (5), 623–633. doi:10.1086/683199
Harvey, E., and MacDougall, A. S. (2014). Trophic island biogeography drives spatial divergence of community establishment. Ecology 95, 2870–2878. doi:10.1890/13-1683.1
Hinsinger, P. (2001). Bioavailability of soil inorganic P in the rhizosphere as affected by root-induced chemical changes: A review. Plant Soil 237, 173–195. doi:10.1023/a:1013351617532
Hooper, D. U., and Vitousek, P. M. (1998). Effects of plant composition and diversity on nutrient cycling. Ecol. Monogr. 68, 121–149. doi:10.1890/0012-9615(1998)068[0121:eopcad]2.0.co;2
Hou, E. Q., Luo, Y. Q., Kuang, Y. W., Chen, C. R., Lu, X. K., Jiang, L. F., et al. (2020). Global meta-analysis shows pervasive phosphorus limitation of aboveground plant production in natural terrestrial ecosystems. Nat. Commun. 11, 637. doi:10.1038/s41467-020-14492-w
Jiang, J., Wang, Y. P., Yang, Y. H., Yu, M. X., Wang, C., and Yan, J. H. (2019). Interactive effects of nitrogen and phosphorus additions on plant growth vary with ecosystem type. Plant Soil 440, 523–537. doi:10.1007/s11104-019-04119-5
Lefcheck, J. S. (2016). piecewiseSEM: piecewise structural equation modelling in R for ecology, evolution, and systematics. Methods Ecol. Evol. 7, 573–579. doi:10.1111/2041-210x.12512
Li, Y., Niu, S. L., and Yu, G. R. (2016). Aggravated phosphorus limitation on biomass production under increasing nitrogen loading: A meta-analysis. Glob. Change Biol. 22, 934–943. doi:10.1111/gcb.13125
Mazzorato, A. C., Esch, E. H., and MacDougall, A. S. (2022). Prospects for soil carbon storage on recently retired marginal farmland. Sci. Total Environ. 806, 150738. doi:10.1016/j.scitotenv.2021.150738
McCann, K. S., Cazelles, K., MacDougall, A. S., Fussmann, G. F., Bieg, C., Cristescu, M., et al. (2021). Landscape modification and nutrient-driven instability at a distance. Ecol. Lett. 24 (3), 398–414. doi:10.1111/ele.13644
Mueller, N. D., Gerber, J. S., Johnston, M., Ray, D. K., Ramankutty, N., and Foley, J. A. (2012). Closing yield gaps through nutrient and water management. Nature 490, 254–257. doi:10.1038/nature11420
Niklas, K. J., Owens, T., Reich, P. B., and Cobb, E. D. (2005). Nitrogen/phosphorus leaf stoichiometry and the scaling of plant growth. Ecol. Lett. 8, 636–642. doi:10.1111/j.1461-0248.2005.00759.x
Noble, D., MacDougall, A. S., and Levison, J. (2023). Seasonal dynamics of dissolved nutrient flows in riparian farm buffers. Netherlands: Science of the Total Environment.
Ogdahl, M., Buyarski, C., and contributors, P. W. (2013). Plant phosphorus protocol -sulfuric acid digestion. Minneapolis, MN, USA: PrometheusWiki.
Ontario Ministry of Agriculture Food and Rural Affairs (2018). Field crop budgets 2018. Canada: Ontario Ministry of Agriculture Food and Rural Affairs.
Penn, C. J., and Camberato, J. J. (2019). A critical review on soil chemical processes that control how soil pH affects phosphorus availability to plants. Agriculture 9 (6), 120. doi:10.3390/agriculture9060120
Penuelas, J., Sardans, J., Rivas-Ubach, A., and Janssens, I. A. (2012). The human-induced imbalance between C, N and P in Earth's life system. Glob. Change Biol. 18, 3–6. doi:10.1111/j.1365-2486.2011.02568.x
Pretty, J., Benton, T. G., Bharucha, Z. P., Dicks, L. V., Flora, C. B., Wratten, ., et al. (2018). Global assessment of agricultural system redesign for sustainable intensification. Nat. Sustain. 1, 441–446. doi:10.1038/s41893-018-0114-0
R Core Team (2018). R: A language and environment for statistical computing. Vienna, Austria: R Foundation for Statistical Computing.
Shipley, B. (2009). Confirmatory path analysis in a generalized multilevel context. Ecology 90, 363–368. doi:10.1890/08-1034.1
Sobota, D. J., Compton, J. E., McCrackin, M. L., and Singh, S. (2015). Cost of reactive nitrogen release from human activities to the environment in the United States. Environ. Res. Lett. 10, 025006. doi:10.1088/1748-9326/10/2/025006
Stevens, C. J., Lind, E. M., Hautier, Y., Harpole, W. S., Borer, E. T., Hobbie, S., et al. (2015). Anthropogenic nitrogen deposition predicts local grassland primary production worldwide. Ecology 96 (6), 1459–1465. doi:10.1890/14-1902.1
Tilman, D., Knops, J., Wedin, D., Reich, P., Ritchie, M., and Siemann, E. (1997). The influence of functional diversity and composition on ecosystem processes. Science 277, 1300–1302. doi:10.1126/science.277.5330.1300
U.S. EPA (1994). “Method 200.8: Determination of trace elements in waters and wastes by inductively coupled plasma-mass spectrometry,”. Revision 5.4.
Weigelhofer, G., Fuchsberger, J., Teufl, B., Welti, N., and Hein, T. (2012). Effects of riparian forest buffers on in-stream nutrient retention in agricultural catchments. J. Environ. Qual. 41, 373–379. doi:10.2134/jeq2010.0436
Yang, Y., Tilman, D., Lehman, C., and Trost, J. J. (2018). Sustainable intensification of high-diversity biomass production for optimal biofuel benefits. Nat. Sustain. 1, 686–692. doi:10.1038/s41893-018-0166-1
You, C., Wu, F., Gan, Y., Yang, W., Hu, Z., Xu, Z., et al. (2017). Grass and forbs respond differently to nitrogen addition: A meta-analysis of global grassland ecosystems. Sci. Rep. 7, 1563. doi:10.1038/s41598-017-01728-x
Keywords: buffer strips, co-limitation, mesocosm, nutrient leaching, nutrient retention, sustainable agriculture
Citation: Esch E and MacDougall AS (2023) Nitrogen addition enhances terrestrial phosphorous retention in grassland mesocosms. Front. Environ. Sci. 11:1144268. doi: 10.3389/fenvs.2023.1144268
Received: 14 January 2023; Accepted: 06 April 2023;
Published: 13 April 2023.
Edited by:
Kaibo Wang, Chinese Academy of Sciences (CAS), ChinaReviewed by:
Zhenhong Wang, Guizhou University, ChinaYi Wang, Chinese Academy of Sciences (CAS), China
Copyright © 2023 Esch and MacDougall. This is an open-access article distributed under the terms of the Creative Commons Attribution License (CC BY). The use, distribution or reproduction in other forums is permitted, provided the original author(s) and the copyright owner(s) are credited and that the original publication in this journal is cited, in accordance with accepted academic practice. No use, distribution or reproduction is permitted which does not comply with these terms.
*Correspondence: Andrew S. MacDougall, asm@uoguelph.ca