- 1Shandong Provincial Key Laboratory of Applied Microbiology, Ecology Institute, Qilu University of Technology (Shandong Academy of Sciences), Jinan, China
- 2EnviroCORE, Dargan Research Centre, South East Technological University, Carlow Campus, Carlow, Ireland
Introduction: Bioremediation has been shown to be an effective strategy for removing toxic pollutants from the environment, particularly organic chemicals such as petroleum hydrocarbons. This paper investigates the changes in toxicity of petroleum-contaminated soil as a result of microbial remediation processes.
Methods: Changes in the ecotoxicity of the contaminated soil were examined using a plant, earthworm, enzyme activity and luminescent bacteria toxicity tests.
Results: The results showed that bioremediation could effectively degrade petroleum hydrocarbon (C10–C40) pollutants. After 42 days of remediation, the petroleum hydrocarbon (C10–C40) content of Group A (bioaugmented polluted wetland soil) decreased from 1.66 g/kg to 1.00 g/kg, and the degradation rate was 40.6%. The petroleum hydrocarbon (C10–C40) content of Group B (bioaugmented polluted farmland soil decreased from 4.00 g/kg to 1.94 g/kg, and the degradation rate was 51.6%. During the microbial remediation progress, the ecological toxicity of petroleum-contaminated soil first increased and then decreased. The photosynthetic pigment content index in the higher plant toxicity test, the earthworm survival index and the soil catalase activity all showed good agreement with the relative luminescence index of extracted DCM/DMSO in the luminescent bacterial toxicity test. The soil toxicity decreased significantly after remediation. Specifically, the photosynthetic pigment content of wheat were inhibited in the soil during the whole process (remediation for 42 days), and decreased to the minimum on remediation day 21. The 7-day and 14-day survival rate of earthworms in Group A and Group B gradually decreased in the soil remediation process, and then gradually increased, survival rate at the end of remediation was higher than at the beginning. Soil catalase activity was significantly negatively correlated with petroleum hydrocarbon (C10–C40) content (−0.988, −0.989). The ecological toxicity of contaminated soil reached to the maximum on the 21st day of remediation, relative luminosity of luminescent bacteria in dichloromethane/dimethyl sulfoxide extracts from Group A and Group B were 26.3% and 16.3%, respectively.
Conclusion: Bioremediation could effectively degrade petroleum hydrocarbon (C10–C40) pollutants. Wheat photosynthetic pigment content, earthworm survival rate, soil catalase activity and relative luminescence of luminescent bacteria can better indicate the ecological toxicity of petroleum-contaminated soil in bioremediation process.
Highlights
• The degradation rate of petroleum-contaminated soil was 40.64% and 51.56% after microbial remediation.
• During the remediation of petroleum-contaminated soil, the toxicity increased first and then decreased.
• Soil enzyme activity is affected by petroleum content, organic matter content and soil respiration.
• Ecotoxicity test of contaminated soil is helpful to determine the best remediation strategy.
1 Introduction
Petroleum is the world’s largest energy source, accounting for up to a third of global energy demand (Komarova et al., 2022). Data show that global petroleum production has reached more than four billion tons per year, while annual consumption is five billion tons (Wang et al., 2022). However, almost every process of petroleum exploration and petrochemical production pollutes the surrounding environment (Alex et al., 2021). In recent years, petroleum spills have occurred frequently around the world. Due to the leakage of petroleum pipe holes, ship transportation accidents and industrial sewage, the environment of groundwater, ocean and soil has been polluted to varying degrees (Kuria et al., 2017; Shamshad et al., 2021). In China, as a result of petroleum exploitation, 100, 000 tons of contaminated soil is generated annually, the exceeding points of petroleum hydrocarbons (TPHs) accounted for 23.6%, in the sewage irrigation area (MEEPPC, 2014). Contamination by petroleum hydrocarbons (PHs) is a great threat to environment due to the higher persistence and bio-toxicity of PHs. Landing crude oil not only pollutes the environment, but also wastes resources and diminishes natural habitats. Therefore, removal of PHs from contaminated environment and strategies to reduce their toxic effects on living organisms are crucial for environmental safety and human health (Alex et al., 2021; Ali et al., 2021).
In recent years, there are more and more researches on the harmless remediation technology of petroleum-contaminated soil, including physical treatment, chemical treatment, bioremediation and phytoremediation (Son et al., 2021; Chang et al., 2022). Among them, microbial mediated bioremediation has been widely studied because of its advantages of no secondary pollution, high efficiency and low cost. Many studies often use the total concentration of pollutants in the soil as the basis for calculating the risk to human and environmental health (Sample et al., 1997) without considering the bioavailability of soil. However, due to the complexity of soil ecosystem and the complexity of petroleum composition, the actual risk exposure of soil depends on the bioavailability of these pollutants. Single chemical analysis cannot accurately reflect the ecological toxicity of soil (Stella et al., 2015). Therefore, it is recommended to combine chemical analysis with different toxicity assays to characterize the toxicity level of the contaminated soil (Plaza et al., 2010; Kanaji et al., 2014), which can reflect the complexity and superposition of pollutant toxicity. Assessment of ecological risks associated with soil contamination has become an important area of research in toxicological studies (Khan et al., 2013).
Previous studies have shown that the change of soil ecotoxicity can be monitored by a variety of test methods such as higher plant toxicity test, luminescent bacteria toxicity test, earthworm toxicity test and soil enzyme activity test (Khan et al., 2012; Libralato et al., 2016; Chae et al., 2020; Yla et al., 2020; Alabi et al., 2022). Due to the strong adhesion and low emulsification ability of petroleum, it is easy to adhere to the surface of soil particles or plant roots, which will affect the permeability of soil (Devatha et al., 2019), and further affect the absorption, transpiration and respiration of water, mineral nutrients, function of enzymes in plants, photosynthesis and membrane permeability (Mansur et al., 2015). And the plant species-specific differences found in seed germination and tolerance to pollutants are very large (Khan et al., 2014). Therefore, higher plants can comprehensively reflect the degree of soil pollution and its ecological toxicity by using their growth and development status and leaf physiological and biochemical indexes (Haq and Kalamdhad, 2021). Rivera-Cruz and Trujillo-Narcía (2004) used five temperate plants to study the effects of different concentrations of oil-contaminated soil on their germination rate, plant height, root length, and total biomass. Liu et al. (2021) studied the joint toxicity of polyethylene microplastics and phenanthrene on wheat seedlings and observed their effects on wheat biomass, stem height, root length and photosynthesis. Soil invertebrates are useful soil quality indicators due to their sensitivity to disturbances in edaphic conditions (Julie et al., 2002). These tests are also relatively simple, cost effective and quick to execute. Earthworms are one of the most vulnerable soil invertebrates to environmental toxins and have been successfully used to evaluate the toxicity of heavy metals, antibiotics, pesticides and petroleum in soil (Wu et al., 2012; Harmanpreet et al., 2019; Sheng et al., 2021; Chen et al., 2022). Because they are bioindicators of soil pollution, Eisenia foetida (Pérez-Losada et al., 2005) has long been used as a key indicator organism of ecotoxicology diagnosis (Amorim et al., 2011; Gainer et al., 2019). As an important part of soil, soil enzymes mainly come from soil microorganism, plant roots and decomposition of soil animal and plant residues (Ma et al., 2014). They are involved in various biochemical processes in soil, which can reflect the overall health status of soil and have potential in assessing the impact of hydrocarbons on soil health (Dick, 1997). They have been listed as one of the important means of testing the ecological toxicity of soil pollutants by the International Organization for Economic Cooperation and Development (OECD) and the International Organization for Standardization (ISO) (Tan et al., 2017). In order to evaluate the effect of crude oil on soil enzyme activity, Dindar et al. (2017) conducted a 150-day biostimulation remediation experiment on crude oil-contaminated soil. The results showed that the urease activity (UA) level after remediation of crude oil-contaminated soil was generally higher than the initial UA level of soil. Luminescent bacteria toxicity test has the advantages of high sensitivity, high throughput, low cost and automated analysis, which is suitable for preliminary screening of soil toxicity (Heinlaan et al., 2007; Khan et al., 2012). It has been widely used in the evaluation and monitoring of soil ecological toxicity in petroleum-contaminated soil remediation process (Wu et al., 2021; Wang et al., 2023). Studies have shown that there is a quantitative relationship between soil pollutant concentration and relative luminous intensity of luminous bacteria (Yu et al., 2021).
The above methods have their own advantages in ecological toxicity assessment. The indication and evaluation of soil ecological toxicity by different indicator organisms can effectively integrate the overall toxicity effect of different food chain organisms on toxic and harmful substances in soil, and can comprehensively reflect the soil contamination. Therefore, the microbial remediation process of petroleum-contaminated soil needs to integrate various ecological toxicity indicators and evaluation methods to make a comprehensive and scientific judgment on the safety of soil ecosystems (Wcislo et al., 2016). Based on this, this study used higher plant toxicity test, earthworm toxicity test, soil enzyme activity test and luminescent bacteria toxicity test to further explore any changes in the toxicity of two petroleum-contaminated soils as a result of a bioremediation treatment.
2 Materials and methods
2.1 Experimental materials
Test soil: The contaminated surface soil (0–10 cm) near the abandoned oil wells (mined in 2,000 s–2010 s) in Gudao Town, Dongying City, Shandong Province was collected, and the clean surface soil (0–10 cm) near the selected sampling point was used as the blank control. The samples were collected according to ‘The Technical Specification for soil Environmental monitoring’ (HJ/T 166–2004) (National Environmental Protection Standards of the People's Republic of China, 2004). Each soil sample is evenly mixed with four soil subsamples in equal amount, and the subsamples are distributed in four directions around the wellhead of the oil well, all about 5 m away from the oil well. One soil sample originated from a nearby polluted wetland system (N 37° 50′ 20″, E 118° 44′ 4″): Polluted Wetland Soil (PWS), a visibly clean oil sample was taken from this site: Clean Wetland Soil (CWS). Another soil sample was taken from a polluted farmland site (N 37° 51′ 4″, E 118° 45′ 15″): Polluted farmland Soil (PFS) and a clean sample were taken from this farmland: Clean Farmland soil (CFS). The soil samples were taken back to the laboratory, the plant residues and large grained gravel were removed and the soil was dried in the shade, and then mixed evenly through 2 mm sieve. The physical and chemical properties and TPHs content were determined. The results of this soil analysis are shown in Table 1.
Preparation of petroleum degrading inoculum: In this study, SWH-2 (Sphingobacterium multivorum) (Li et al., 2007), WG-6 (Actnetobacter calcoaceticus) (Zhang et al., 2014) and PPZ-1 (Pseudomonas sp.) (Zhang et al., 2018) were used to create a hydrocarbon degrading inoculum. These strains were previously isolated in author’s laboratory and have a certain ability to degrade crude oil or PAHs (Li et al., 2007; Zhang et al., 2017; Wang et al., 2018). The above three strains were enriched and cultured, and then the isolated and purified strains were inoculated into conical flasks containing 100 mL Luria-Bertani medium, and 0.3 g crude oil (non-sterilized) was added to them. The bacteria were cultured in a shaker at 28 °C for 5 days. Finally, the three strain cultures (109 CFU/mL) were mixed in a 1: 1: 1 (V/V/V) to create the TPHs degrading consortia. The consortia were then added to peat samples in a 1:5 (mL/g).
Wheat (Triticum aestivum) seeds of “Jimai 22” were purchased from Fuyichun Seed Company. The seed germination rate reached 100% in the pre-experiment. Jimai 22 is the new wheat variety with the largest planting area in China, which is suitable for planting in the Huanghuai Plain (Ma et al., 2019). Eisenia foetida (Savigny, 1826), one of the experimental earthworm species in international standard OECD 207, was purchased from an earthworm breeding base in Jurong, China. Photobacterium phosphoreum T3 spp. freeze-dried powder was purchased from Nanjing Soil Research Institute, Chinese Academy of Sciences.
2.2 Remediation scheme design of petroleum-contaminated soil
In order to study the effect of microbial treatment on ecotoxicity of petroleum-contaminated soil, the experiment was carried out in a plastic basin of 36 cm × 25 cm × 11 cm. A total of six groups were set up in triplicate. Each plastic basin was filled with 5 kg dry soil, and the prepared microbial agent was inoculated into petroleum-contaminated soil in a proportion of 30 g per 1,000 g (3%) soil (the final concentration of inoculated consortia was 6 × 106 CFU/mL per g of contaminated soil). An appropriate amount of KNO3 was added to adjust C: N to 20: 1 (Margesin and Schinner, 2000), and according to preliminary laboratory experimental results, the soil moisture content was adjusted to 20%. The plastic basin was placed in a constant temperature incubator at 25°C. The soil was mixed every 3 days and water was supplemented to maintain the moisture content at 20%. The treatments were treated as follows.
- Group A: PWS + 3% bacteria agent, C: N = 20:1, soil moisture content 20%, 25°C
- Group B: PFS + 3% bacteria agent, C: N = 20:1, soil moisture content 20%, 25°C
- Group C: CWS + 3% bacteria agent, C: N = 20:1, soil moisture content 20%, 25°C
- Group D: CFS + 3% bacteria agent, C: N = 20:1, soil moisture content 20%, 25°C
- Group E: Untreated PWS, 25°C
- Group F: Untreated PFS, 25°C
On the 0th, 7th, 14th, 21st, 28th, 35th and 42nd day of the experiment, the soil samples of each group were collected for toxicity test.
2.3 Experimental method
2.3.1 Extraction and content determination of petroleum hydrocarbons in soil
Determination method of soil TPHs (C10–C40) content referred to ‘Soil and sediment-Determination of petroleum hydrocarbons (C10–C40)-Gas chromatography’ (HJ 1021-2019) (He et al., 2021). Moisture content of sediment samples was determined according to ‘The specification for marine monitoring—Part 5: Sediment analysis’ (GB 17378.5) (National Standards of the People's Republic of China, 2007). TPHs were extracted by soxhlet extraction method.
2.3.2 Higher plant toxicity test
The ecotoxicity of petroleum-contaminated soil in remediation process was evaluated with the wheat growth test (based on OECD 208). In brief, 10 wheat seeds were evenly embedded in different soil samples (220 g soil in each sample), with a moisture content of 60% field capacity (FC). Environmental conditions are set according to OECD 208 standard (Organization for Economic Co-operation and Development, 2006). The seed germination rate was recorded on the 5th day, and the plant height, fresh weight of above-ground and root fresh weight of wheat seedlings were measured on the 14th day after 50% of the control wheat emerged. Five plants for each parallel sample were selected. Photosynthetic pigment determination method was determined as previously described (Saric, 1978).
2.3.3 Earthworm toxicity test
Eisenia foetida were raised 1 week in advance according to the OECD 207. Experiment on earthworm mortality: Earthworms are washed and weighed before being transferred into the soil samples of different remediation periods to be tested (30 organisms per 500 g of soil). The raising conditions are set according to OECD 207 standard (Organization for Economic Co-operation and Development, 1984). After 7 days and 14 days mortalities are registered. Earthworm avoidance experiment: A 25 cm long, 17 cm wide and 13 cm high container was divided into two parts with a partition plate placed in the middle of the container. One part was placed in the clean soil, and the other part was placed in the contaminated soil at different remediation periods. The partition was removed and 10 earthworms were added. The earthworms were cultured in 20°C lightless incubator. After 48 h, the earthworms on both sides were counted. The calculation formula of avoidance rate was described (Achazi, 2002).
2.3.4 Detection of soil enzyme activity
Urease (UE) activity: By an indophenol blue method, the soil samples were incubated at 38°C for 24 h. The product NH3-N reacted with hypochlorite and phenol in strong alkaline medium to form water-soluble dye indophenol blue, which was determined at 578 nm (Feng et al., 2008). Catalase (CAT) activity: By a colorimetric method, CAT catalyzes hydrogen peroxide to produce water and oxygen, residual hydrogen peroxide reacts with a highly sensitive chromogenic probe to produce colored substances, which was determined at 510 nm (Trasar et al., 1999). Sucrase (SC) activity: By a 3, 5-dinitrosalicylic acid (DNS) method, soil samples were incubated at 37°C for 24 h and the reaction product reacted with 3, 5-dinitrosalicylic acid, then the resultant was determined at 540 nm (Zhu et al., 2011). Polyphenol oxidase (PPO) activity: By a colorimetric method, using levodopa as substrate. Soil samples were incubated at 25°C for 60 min and the reaction product red quinones was determined at 475 nm (Bartosz et al., 2009). Soil moisture content was measured by gravimetric method.
2.3.5 Acute toxicity test of luminescent bacteria
Extraction method of water extract from petroleum-contaminated soil referred to ‘Solid waste—Extraction procedure for leaching toxicity—Horizontal vibration method’ (HJ 557-2010) (National Environmental Protection Standards of the People's Republic of China, 2010). The preparation of dichloromethane (DCM)/dimethyl sulfoxide (DMSO) extract from contaminated soil was described (Liu et al., 2007). Determination and calculation of biological toxicity were elaborated (Bundy et al., 2004).
2.4 Data analysis
All data were sorted and drawn using Excel 2022 (Microsoft, United States) and Origin 2022 (OriginLab, United States), and analyzed using SPSS Statistics 20 (IBM, United States).
3 Results and discussion
3.1 Microbial remediation of petroleum-contaminated soil
Microbial remediation was carried out by adding microbial agents and appropriate nutrients to petroleum-contaminated soils. TPHs concentrations were monitored at 0th, 7th, 14th, 21st, 28th, 35th and 42nd day after inoculation. The results showed that TPHs in the contaminated soil were rapidly degraded within 0–7 days after the addition of microbial agents, and the degradation rate of TPHs gradually slowed down after 7 days (Figure 1). The degradation rate of Group A reached 25.6% after 7 days, and the degradation rate reached 40.6% at the end of the experiment. While the degradation rate of Group B was 31.1% on day 7, and 51.6% at the end. The degradation rate of Group B during the whole process was higher than that of Group A, probably because the high soil TPHs content of Group B could provide more abundant nutrients for microorganisms, which was conducive to microbial metabolism and reproduction. At the end of Group A degradation, the TPHs content increased, which may be due to the small change of degradation rate, resulting in insignificant change of oil content. In the early stage of remediation, microorganisms in microbial agents used carbon in petroleum as nutrients to multiply, and TPHs components degraded rapidly. During the experiment process, the degradation rate greatly slowed down. On the one hand, it may be because the n-alkanes and naphthenic hydrocarbons with less carbon were consumed in large quantities at the beginning of remediation, and then the microorganisms began to degrade the refractory aromatic hydrocarbons (Chaineau et al., 1995; Langbehn and Steinhart, 1995). On the other hand, it may be that the TPH intermediate metabolites accumulated in the degradation process, such as aldehydes, ketones, fatty acids (Jorgensen et al., 2000), and metabolites produced by microorganisms change the soil environment, and affect the microorganisms with TPH degradation ability, resulting in slow degradation rate. The overall concentration of group E and group F without microbial agents for natural degradation did not change significantly. The degradation rates of Group E and Group F were 1.02% and 2.03% after 42 days, respectively, which may be due to the abiotic dissipation of TPHs in soils.
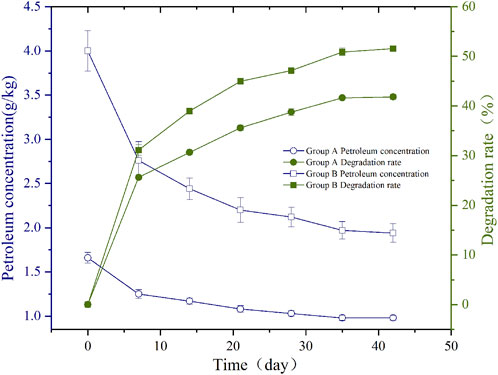
FIGURE 1. Concentrations and degradation efficiencies of petroleum hydrocarbons (C10-C40) in different contaminated soils. The data points represent the mean values of the parameters, the error bars are the standard error of the values, n = 3.
3.2 Phytotoxicity of remediated soils on wheat
3.2.1 Effect on germination rate of wheat seeds
In this study, the germination rate of wheat seeds in clean soils (Group C and Group D) was 83.3% ± 5.8% and 90.0% ± 0.0%, respectively (Supplementary Table S1). The germination rate of wheat seeds in the contaminated soils (PWS and PFS) was not significantly different from those in the clean soils (p > 0.05), which indicated that wheat germination was not affected by the concentration of TPH present in these soils. There are no significant correlation between the germination rate of wheat seeds in the soil at different remediating stages and the TPHs content in the soil, p = 0.254 in Group A and p = 0.312 in Group B. This may be because the nutrients required for seed germination of higher plants are mainly derived from the energy in their endosperm, which makes them less sensitive to soil environmental pollution (Chouychai et al., 2007). At the same time, seed rind plays a protective role on the internal structure of seeds, which can prevent the entry of toxic and harmful substances (Liu et al., 2001).
Many researches have reported that low levels may not have been high enough to have phytotoxic effects at this stage of wheat growth. Tang et al. (2011) also showed that contaminated soils from the Shengli oilfield with a TPH level of around 0.1% had little effect on wheat germination, whereas at 0.5%–3.0% TPH they observed significant inhibition of wheat germination (10%–60%). The group A (PWS) soil in our study had TPH levels of 0.16% and in the group B soils (PFS) had TPH levels of 0.4%. Other researchers have shown that the inhibition rate was 48.4% at TPH content of 3%, suggesting strong tolerance of wheat on TPH (Oboh et al., 2007). Studies have shown that only TPHs concentrations higher than 0.5% can significantly reduce seed germination (Gaskin et al., 2008). So some studies have speculated that high concentrations of TPHs will affect the germination rate of seeds (Varjani et al., 2020). Meantime, the phytotoxicity of TPHs may be affected by differences in absorption, nutrient availability and cell wall structure of petroleum compounds in different plant species or tissues (Banks and Schultz, 2005). High concentrations of salt and organic pollutants inhibit seed germination (Kumari et al., 2016; Kaboosi, 2017). There was no change in the germination rate of wheat in the soil of group E and F (83.3% and 86.7%) at different remediation stages.
3.2.2 Effects on growth and development of wheat seedlings
The results of toxicity experiment of higher plants showed a significant ecotoxicity of the soil during the whole period of bioremediation (Table 2). The plant height of Group B wheat in the 7th and 14th days of soil remediation showed significant inhibition compared with the non-pollution control group (p < 0.01). There were also significant differences (p < 0.01) in the above-ground fresh biomass between the 7th and 14th days of remediation and the non-polluting soil control. The above-ground fresh weight was significantly lower than that of the pollution control (p < 0.01), and it was significantly inhibited (p < 0.05) in the 21st day. Root fresh weight in 0 days, 28 days, 35 days and 42 days soil, compared with non-pollution control showed a significant increase (p < 0.01), and in 7 days and 14 days soil showed a significant inhibition (p < 0.01). At the same time, the growth index of wheat in Group B was slightly higher than that of Group A, probably because the farmland soil had greater nutrients P, organic carbon and less salt, so more suitable for plant growth, the extra nutrients probably lead to enhanced plant growth that compensated for the slightly higher levels of TPH. TPHs will be degraded by plants and generate energy for their own growth (Košnář et al., 2020). The correlation analysis between the growth and development indexes of wheat seeds in the soil at different restoration stages and the TPHs content in the soil was carried out, and the P values were all greater than 0.05. In general, a higher sensitivity of root elongation was found when compared with shoot length and seed germination test (Khan et al., 2014). There was greater root growth of the wheat in the soil samples after 28 days of bioremediation than at the beginning of the bioremediation process, indicating some reduction in phytotoxicity.
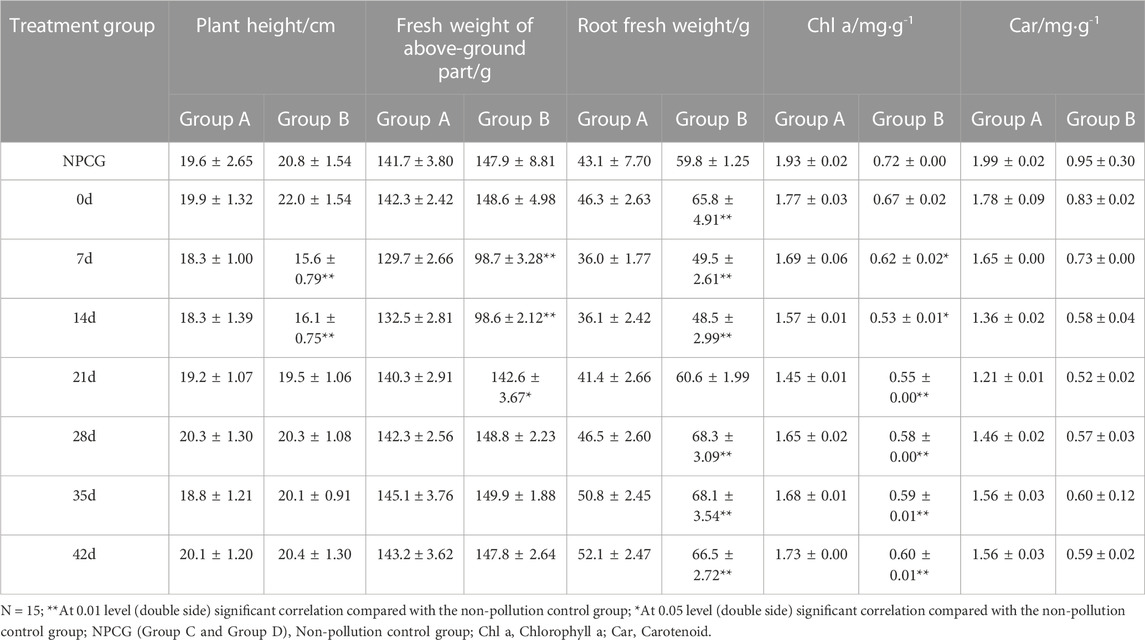
TABLE 2. The effects of different soil remediation periods on wheat seedling growth and photosynthetic pigments in wheat seedling leaves.
3.2.3 Effect of photosynthetic pigment synthesis in wheat seedlings
TPHs can interfere with chloroplast arrangement, thereby interfering with electron transport and photosynthesis (Singh Tomar and Jajoo, 2013; Jajoo et al., 2014). Some high molecular hydrocarbon substances in petroleum can enter cells through the cell wall, affecting chlorophyll synthesis, thereby affecting the photosynthesis process, and producing serious toxic effects on plants (Plaza et al., 2010). It can be seen that the synthesis of light and pigments is affected. Chlorophyll a content of wheat showed inhibitory effect in soil at different remediation time-points. Group A and Group B had the greatest inhibitory effect on soil on day 21, had the least inhibitory effect on soil on day 7 (Table 2). Carotenoid content of wheat was also inhibited throughout the remediation process. Carotenoid content of Group A was significantly inhibited, most severely inhibited in soil on day 14, and Group B was most severely inhibited in soil on day 21. After statistical analysis, the chlorophyll a content and carotenoid content of wheat had a very significant positive correlation, r = 0.917 (p < 0.01) in Group A and r = 0.541 (p < 0.01) in Group B, indicating that the content of chlorophyll a and carotenoid content had good consistency under petroleum pollution stress. In the correlation analysis of wheat photosynthetic pigment content and TPHs content in soil at different restoration stages, only the carotenoid content of wheat in Group B was extremely significantly correlated with TPHs content, r = 0.897 (p < 0.01).
Studies have shown that, petroleum pollutants into the soil will reduce the bioavailability of soil nutrients, weaken the uptake of soil nutrients by plants, thereby affecting the synthesis of photosynthetic pigments in plants (Karthikeyan et al., 2014); on the other hand, petroleum in the soil and intermediate metabolites during microbial remediation destroy the chloroplast structure (Xie et al., 2014) and inhibit some enzyme functions in the cells, resulting in a decline in photosynthetic pigment content in plants. Some components of TPHs, other than metabolite, have direct toxic effects, such as the discovery in wheat that fluoranthene inhibits both light and dark photosynthesis (Tomar and Jajoo, 2014). Research has demonstrated that PAHs inhibit OEC and electron transport chain of PS-II donor side and acceptor side (Kummerová et al., 2008). The toxicity increased and then decreased during the remediation process, probably due to the production and accumulation of toxic metabolites during petroleum degradation, and then the intermediate products were partially degraded. Or while the bioremediation removed many of the organic compounds, less degradation occurred for fluoranthene and other polycyclic aromatic hydrocarbons that affect leaf photosynthesis.
Among the ecological toxicity evaluation indexes of higher plants in this study, the growth and development indexes of wheat seedlings (plant height, above-ground fresh weight, root fresh weight) indicated that the soil had the strongest toxicity on the 7th and 14th days of remediation. The biochemical indexes of wheat leaves (chlorophyll a and carotenoids) showed that the ecological toxicity of soil in the early to middle stages of remediation increased. The soil toxicity was the strongest on the 21st day, and then decreased, but there was a certain ecological toxicity in the soil during the whole remediation period.
3.3 Effect of petroleum-contaminated soil in different remediation timepoints on earthworm (Eisenia foetida)
3.3.1 Effect on earthworm (Eisenia foetida) survival
As a biological indicator, earthworm mortality bioassay is an important tool for ecotoxicological research (Khan et al., 2012). Survival of earthworms in the soils at different time-points in the remediation process was examined. Earthworms were placed into the clean soils from both sites (CWS and CFS) survived until the end of the experiment. There were death and poisoning symptoms of earthworms in contaminated soil at different remediation periods. The poisoning symptoms were as follows: body dehydration, in vivo peristalsis and stretching ability was significantly weakened.
It can be seen that the survival rate of E. foetida (Savigny, 1826) decreased significantly with the prolongation of exposure time, indicating a chronic toxicity of petroleum-contaminated soil on the earthworms. There was a gradual decrease in the survival rate between 7-day exposure and 14-day exposure in both group A and B soils probably due to the bioaccumulation of toxic compounds over time (Figure 2A). There was an increase in earthworm survival rates in Group A soils after more than 7 days of bioremediation. Whereas in the Group B soils survival rates initially increased but then decreased at around day 21 but by the end of the bioremediation experiments the survival levels were much greater than at the beginning. This dip in survival rate could be due to increase levels of toxic metabolites that were later degraded. The survival rate of earthworms increased at the end of remediation of both contaminated soils, suggesting that bioremediation ultimately succeeded in reducing soil toxicity. While the mortality of earthworms in the earthworm toxicity experiments at different petroleum concentrations showed a dose-effect relationship (Lucia et al., 2012), it is different from the results of this paper, indicating that some toxic intermediates will be produced in the process of soil remediation, and these intermediates cannot be simply expressed by chemical units, but their toxicity will be reflected in organisms (Dallinger and Horn, 2014; Zhao and Zhu, 2017).
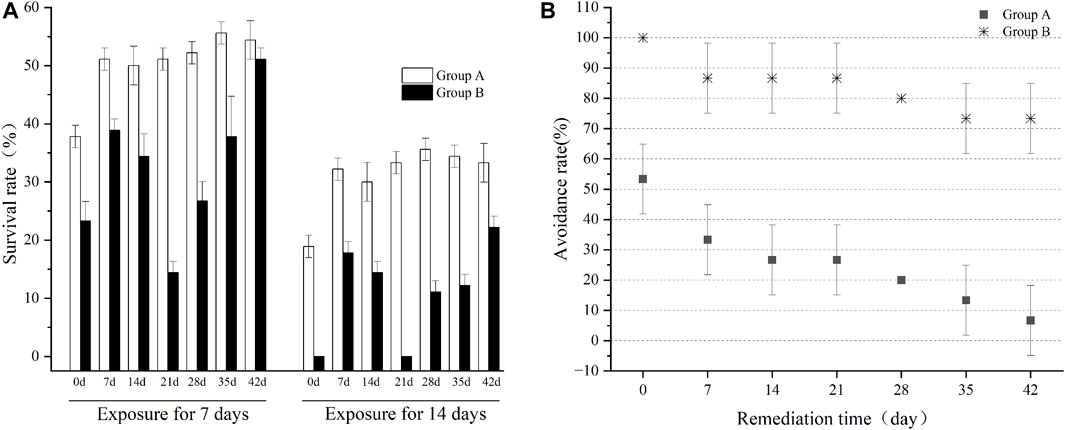
FIGURE 2. Effect of petroleum-contaminated soil on earthworm (E. foetida). (A) Survival rates of earthworms (E. foetida) in soil at different remediation time-points. (B) Avoidance rates of earthworms (E. foetida) in soil at different remediation periods. The columns represent the mean values of the parameters, the error bars are the standard error of the values, n = 3.
In the correlation analysis between earthworm survival rate and TPHs content in soil at different restoration stages, only earthworm survival rate in Group A was significantly correlated with TPHs content, r = −0.967 (p < 0.01) at 7 days and r = −0.958 (p < 0.01) at 14 days.
3.3.2 Avoidance test of Eisenia foetida
TPH may cause death, swelling, body lesions, stiffening, coiling and low reproduction of earthworm (Oboh et al., 2007). In the avoidance test for 48 h, no earthworm death was observed in the control group C and D and the treatment group, but the avoidance rates of E. foetida in all treatment groups were positive after 48 h (Figure 2B). In the avoidance test throughout the remediation period, most earthworms chose the clean soil, and those which chose to enter the TPH contaminated soil would escape and re-enter, indicating that E. foetida are very sensitive to the toxicity of petroleum pollutants. With the decrease of petroleum pollution concentration, the avoidance rate also decreased. In Group A, even if the TPHs content in the soil at the late restoration stage had dropped to a low level (1.00 g/kg), E. foetida still have a 6.67% avoidance rate, indicating that E. foetida are very sensitive to soil toxicity. When the number of earthworms in clean soil accounted for 80% of the total number of earthworms added in the experiment, it showed that contaminated soil was not suitable for earthworm survival, that is, there was avoidance behavior (ISO) (International Organization for Standardization, 2004). Therefore, in Group B, only the soil on the 35th day (1.97 g/kg) and the 42nd day (1.94 g/kg) was suitable for earthworm survival, and the E. foetida avoidance rate was 73.3%. The avoidance rate is higher in the Group B (PFS) soil with the higher (4 g/kg) TPH level. In the correlation analysis of earthworm avoidance rate and TPHs content in soil at different restoration stages, the earthworm avoidance rate of group A and group B were extremely significantly correlated with TPHs content, r = 0.947 (p < 0.01) in group A, r = 0.910 (p < 0.01) in group B. The data shows that avoidance rates in both contaminated soils dropped as the bioremediation time delayed, again indicating that bioremediation reduced the ecotoxicity in these soils.
3.4 Changes of enzyme activities in petroleum-contaminated soils in different remediation periods
3.4.1 Changes of enzyme activity
Catalase is a kind of oxidoreductase, which is formed during the biochemical oxidation reaction of biological respiration and organic matter. Its activity can characterize the soil humus intensity and the conversion rate of organic matter (Zhou and Zhang, 1980). It can be seen from Figure 3A that the catalase activity of Group B was higher than that of Group A, indicating that the increase in organic matter content would enhance the enzyme activity, there is a very significant positive correlation between soil catalase activity and soil organic matter content (Wang et al., 2012). The increase in TPH content would not inhibit the catalase activity within a certain range. The growth rate of catalase activity in contaminated soil with microbial agents at the early stage of soil remediation was significantly higher than that at the middle and late degradation stages, and the catalase activity steadily increased over 28 days and then plateaued, which was consistent with the results of Margesin and Schinner (2000). The enhancement of catalase activity is due to the large-scale reproduction of microorganisms after adapting to the environment in the early stage of remediation, which increases the decomposition of H2O2 generated during the degradation of TPHs, promotes the secretion of catalase, and increases the enzyme activity. The production of toxic intermediates and the accumulation of excess H2O2 can inhibit the growth and activity of microorganisms, and reduce the secretion of enzymes (Sarkar et al., 2005).
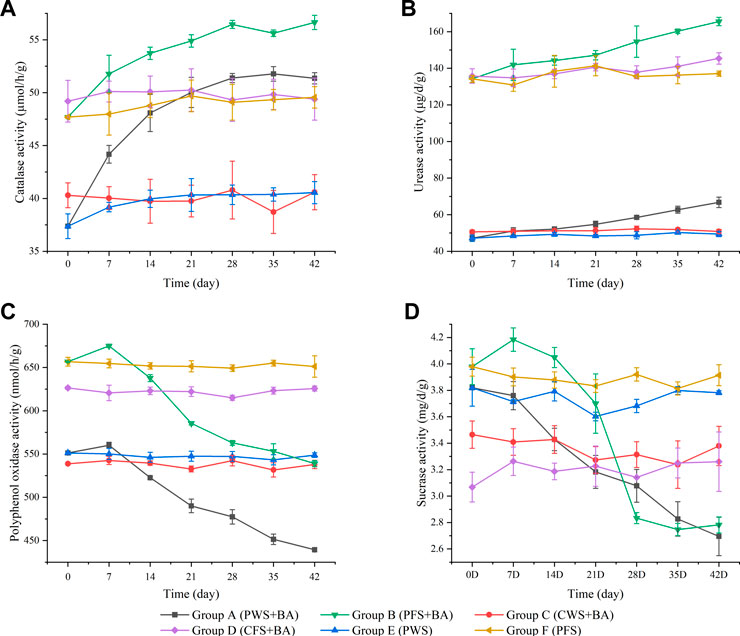
FIGURE 3. Changes of enzyme activities in soil. The data points represent the mean values of the parameters, the error bars are the standard error of the values, n = 3.
Urease is an amide enzyme that promotes the hydrolysis of carbon-nitrogen bonds (CO-NH) in organic matter. It can reflect the status of soil nitrogen transformation and nutrient level in bioremediation of petroleum-contaminated soil. It can be seen from Figure 3B that the urease activity in soil increased with the extension of remediation time, possibly due to the inoculated microorganisms using the TPH as a carbon source for reproduction and growth after adapting to the soil environment, which promoted the transformation of soil nitrogen and thus promoted the secretion of urease (Makoi and Ndakidemi, 2008). At the same time, some studies showed that soil urease activity was not only closely related to nitrogen level, but also positively correlated with soil organic matter content, total nitrogen and available phosphorus (Niu et al., 2021).
The degradation and metabolism of TPHs will also produce a certain amount of polyphenols, which are the substrates for PPO enzymes. The PPO activity in soil can be used to characterize the biodegradation of some TPHs (Riah et al., 2014). It can be seen from Figure 3C that during the remediation of petroleum-contaminated soil, the activity of PPO increased first and then decreased continuously, with the highest activity on the 7th day of remediation. The increase of PPO activity within 0–7 days may be due to the addition of microbial agents and supplement of nitrogen, which is conducive to the degradation of TPHs (Xu et al., 2020). Chen et al. (2017) also observe a similar PPO profile. However, as the TPHs content decreased and became less available for microbial degradation then PPO activity decreased. Previous studies showed that there is a certain correlation between PPO activity and petroleum degradation rate (Wang et al., 2011).
Sucrase is involved in the decomposition of organic matter in soil, which is closely related to soil carbon cycle (Chen et al., 2010). In this study, the sucrase activity (Figure 3D) of Group A continued to decrease and the sucrase activity during day 0–14 of Group B was higher than that before remediation, and then continued to decrease. The increase of sucrase activity in the early stage of Group B remediation may be because low concentrations of organic pollutants in petroleum-contaminated soil would increase sucrase activity (Dindar et al., 2015). And sucrase and microorganisms use hydrocarbons as substrates and carbon sources (Baran et al., 2004; Labud et al., 2007). A sharp reduction in the activity of this enzyme in the contaminated soil may indicate reduced content of sucrose and other sugar-based organic molecules. The increase respiration rate, in the remediated soil have led to rapid utilization of the organic matter in the soil, and so to a drop in sucrase activity.
3.4.2 Correlation between soil enzyme activity and petroleum content
Through the correlation analysis between soil enzyme activity and petroleum residue in the remediation process of petroleum-contaminated soil, the results are shown in Table 3. In Group A and Group B, catalase activity was significantly negatively correlated with TPHs content (p < 0.01), urease activity was significantly negatively correlated with TPHs content (p < 0.05), and PPO activity was significantly positively correlated with TPHs content (p < 0.05). Sucrase activity was positively correlated with TPHs content in Group A (p < 0.05), but not in Group B (p > 0.05).
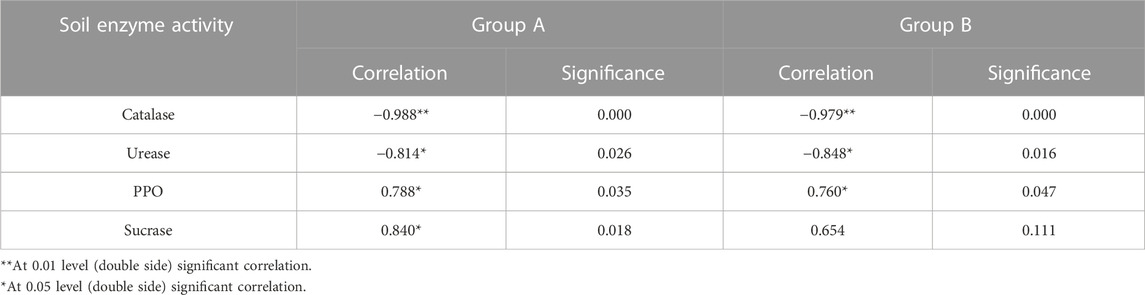
TABLE 3. Correlation analysis between petroleum concentration and soil enzyme activity during remediation of petroleum-contaminated soil.
TPHs can cause changes in soil enzyme activity. Firstly, TPHs affects the growth and reproduction of microorganisms in soil, thereby affecting the synthesis and secretion of enzymes in microorganisms. Secondly, TPHs affect the growth and metabolism of plants and the secretion of root exudates and related enzymes are also affected. Thirdly, petroleum pollutants directly act on soil enzymes, changes the structure of enzymes, and inhibits or promotes the activity of enzymes (Tabatabai and Dick, 2002; Andreoni et al., 2004). From the perspective of the four enzyme activities in the six soils during the whole microbial remediation process, the indigenous microorganisms in the soil have little effect on relieving the ecological toxicity of petroleum-contaminated soil, which is manifested by the fact that the enzyme activity in the contaminated soil without the addition of the microbial agent changes little throughout the remediation process. This also verifies the consistency of the previous test: the test exogenously added composite microbial agents play a leading role in the removal of petroleum pollutants. The difference of enzyme activity between Group A and Group B indicated that soil enzyme activity was significantly affected by topography, land use and soil type (Alvarenga et al., 2008).
3.5 Luminescent bacteria toxicity test
The experimental results show that the aqueous extract of the two petroleum-contaminated soil samples with remediation has no significant inhibitory effect on the relative luminescence intensity of luminescent bacteria, and the relative luminescence intensity of soil aqueous extract during the whole remediation process is above 85%, belonging to the level of micro-toxicity (Figure 4). This is because the main pollutants in the test soil are TPHs insoluble in water, and these toxic substances in aqueous extract may be water-soluble intermediate metabolites produced by hydrocarbons during degradation (Lin et al., 2001).
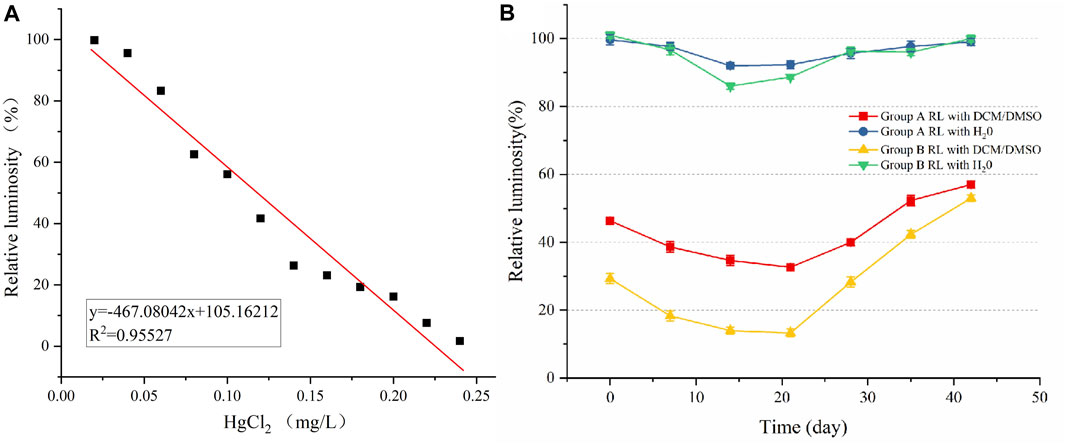
FIGURE 4. Changes of relative luminosity of luminescent bacteria in soil at different remediation periods. (A) Mercury chloride luminescence scale. (B) Relative luminosity of soil water and DCM/DMSO extraction liquid phase. The data points represent the mean values of the parameters, the error bars are the standard error of the values, n = 3.
It can be seen that Group A chart that the luminosity was increased from 46.3% before remediation to 57% after remediating, and the toxicity was reduced by 18.8%. Group B luminosity increased from 29.3% before remediating to 53% after remediating, and the toxicity decreased by 44.7%. Both Group A and Group B showed the lowest relative luminescence, i.e., the highest toxicity, on the 21st day of remediation, indicating the accumulation of toxic metabolites in the first half of remediation, and studies have shown that the oxidation intermediates of PAHs have stronger biological toxicity than their parent hydrocarbons (Wang et al., 2021). With the remediating process, the toxicity decreased gradually from 21 days to 42 days, which may be due to the growth and reproduction of microorganisms using intermediate metabolites, and the toxicity decreased slowly. Correlation analysis between soil TPHs content and relative luminosity showed that for both group A (r = −0.104), and group B (r = −0.262), the correlation was not significant (p > 0.05). Although the TPHs content in the Group B soil at day 42 was just a little higher than that at day 0 for the Group A soil, the corresponding relative luminescence’s differed greatly. Group B at day 42 had a TPH concentration of 1.94 g/kg and a luminosity of 53%, while Group A at day 0 had a TPH level of 1.66 g/kg and a luminosity of 46%. The reason for this is unclear, but it may be related to differences in the hydrocarbon profiles in the two soils. At day 0 group A soil may have contained more toxic components or indeed it could be related to other physic-chemical differences in the two soils.
The luminescence intensity of the DCM/DMSO extract of petroleum-contaminated soil demonstrated a consistent trend with the amount of photosynthetic pigment in wheat and the survival rate of earthworms, which gradually decreased in the early stage, reached the lowest level on the 21st day, and then gradually increased. This indicates that the ecological toxicity of the soil gradually increased, with the highest toxicity on day 21, and then the toxicity gradually decreased, which is consistent with the experimental results of Phillips et al. (2000). This may be because in the early stages of remediation, the intermediate metabolites of hydrocarbon degradation can be more toxic than the parent compounds (Phillips et al., 2000), and the early rapid proliferation of microorganisms capable of degrading PHs significantly increased the rate of degradation, resulting in the early rapid accumulation of toxic intermediates, leading to increased soil toxicity. As the remediation process progressed, the rate of PH degradation, their concentration, the amount of metabolites produced and the toxicity of the soil decreased. Several studies claim that sorption, which is regulated by time and the physicochemical properties of specific soils, is a commonly accessible factor in the bioavailability, degradation and toxicity of soil contaminants (Rivas, 2006; Guo et al., 2022). Day 21 may be in the early stage of the actual whole degradation process. At this stage, toxic intermediate metabolites are continuously generated and accumulated. Therefore, if the scaled-up toxicity portion of our experiment had been prolonged to a point where contaminant levels were even further reduced, we might have seen a more complete process of toxicity changes.
4 Conclusion
In this paper, four ecotoxicity indices were combined to evaluate the ability of bioremediation to reduce the ecotoxicity of two petroleum-contaminated soils, including plant, earthworm, soil enzyme and bacterial toxicity assays. The results suggest that wheat seed germination may not be appropriate to be used as a monitoring tool for petroleum-contaminated soils. Nevertheless, the relative luminosity of luminescent bacteria can be a sensitive indicator of the ecotoxicity of petroleum-contaminated soil, which is consistent with the results of the plant seedling growth and development index, the photosynthetic pigment toxicity test, the earthworm survival rate test and the soil catalase activity test. In addition, in the process of microbial remediation of petroleum-contaminated soil, the reduction of target TPH contaminant residues does not imply a reduction in soil ecotoxicity. The results also show that the ecological toxicity of petroleum-contaminated soil reached the maximum in the early and middle stages of remediation, and gradually weakened in the later stage, and there was a certain ecological toxicity throughout the microbial remediation process. The changes of ecotoxicity index were not always related to the concentration of pollutants, and for each soil type the trend from one test to another may not be consistent. Each test demonstrated a different ability to detect a reduction in soil contamination. Further experimental investigations are needed to explore the correlation between the changes of these indicators and the toxicity of petroleum-contaminated soil.
Data availability statement
The raw data supporting the conclusions of this article will be made available by the authors, without undue reservation.
Author contributions
XC and JW designed the study; XC analyzed samples; XC, WZ, and KG designed the graphics; XC, XF, JW, MW, and KG wrote the manuscript; YH supervised the study; and all authors contributed to previous versions and approved the final manuscript.
Funding
This study was supported by programs of the National Key R&D Program of China (2019YFC1804103), and European Union’s Horizon 2020 research and innovation programme “GREENER” under grant agreement number 826312.
Conflict of interest
The authors declare that the research was conducted in the absence of any commercial or financial relationships that could be construed as a potential conflict of interest.
Publisher’s note
All claims expressed in this article are solely those of the authors and do not necessarily represent those of their affiliated organizations, or those of the publisher, the editors and the reviewers. Any product that may be evaluated in this article, or claim that may be made by its manufacturer, is not guaranteed or endorsed by the publisher.
Supplementary material
The Supplementary Material for this article can be found online at: https://www.frontiersin.org/articles/10.3389/fenvs.2023.1141562/full#supplementary-material
References
Achazi, R. K. (2002). Invertebrates in risk assessment development of a test battery and of short term biotests for ecological risk assessment of soil. J. Soils Sediments 2, 174–178. doi:10.1007/BF02991037
Alabi, O. A., Olukunle, O. F., Ojo, O. F., Oke, J. B., and Adebo, T. C. (2022). Comparative study of the reproductive toxicity and modulation of enzyme activities by crude oil-contaminated soil before and after bioremediation. Chemosphere 299, 134352. doi:10.1016/j.chemosphere.2022.134352
Alex, A. E., Osikemekha, A. A., Oluwafunke, J., Bawo, F. K., Emmanuel, T. O., Charming, O. A., et al. (2021). Mapping soil susceptibility to crude oil pollution in the region of delta, south-south Nigeria: A proportional study of environmetrics, health, ecological risks, and geospatial evaluation. Sci. Afr. 14, e01012. doi:10.1016/j.sciaf.2021.e01012
Ali, M. H., Khan, M. I., Bashir, S., Azam, M., Naveed, M., Qadri, R., et al. (2021). Biochar and Bacillus sp. MN54 assisted phytoremediation of diesel and plant growth promotion of maize in hydrocarbons contaminated soil. Agronomy 11 (9), 1795. doi:10.3390/agronomy11091795
Alvarenga, P., Palma, P., Goncalves, A. P., Baião, N., Fernandes, R. M., Varennes, A., et al. (2008). Assessment of chemical, biochemical and ecotoxicological aspects in a mine soil amended with sludge of either urban or industrial origin. Chemosphere 72 (11), 1774–1781. doi:10.1016/j.chemosphere.2008.04.042
Amorim, M. J. B., Oliveira, E., Teixeira, A. S., Gravato, C. S., Loureiro, S., Guilhermino, L. C., et al. (2011). Toxicity and bioaccumulation of phenanthrene in enchytraeus albidus (Oligochaeta: Enchytraeidae). Environ. Toxicol. Chem. 30, 967–972. doi:10.1002/etc.464
Andreoni, V., Cavalca, L., Rao, M. A., Nocerino, G., Bernasconi, S., DellAmico, E., et al. (2004). Bacterial communities and enzyme activities of PAHs polluted soils. Chemosphere 57, 401–412. doi:10.1016/j.chemosphere.2004.06.013
Banks, M. K., and Schultz, K. E. (2005). Comparison of plants for germination toxicity tests in petroleum-contaminated soils. Water, Air, Soil Pollut. 167 (1/4), 211–219. doi:10.1007/s11270-005-8553-4
Baran, S., Bielińska, J. E., and Oleszczuk, P. (2004). Enzymatic activity in an airfield soil polluted with polycyclic aromatic hydrocarbons. Geoderma 118 (3-4), 221–232. doi:10.1016/S0016-7061(03)00205-2
Bartosz, A., Veikko, K., and Aino, S. (2009). Polyphenol oxidase, tannase and proteolytic activity in relation to tannin concentration in the soil organic horizon under silver birch and Norway spruce. Soil Biol. Biochem. 41 (10), 2085–2093. doi:10.1016/j.soilbio.2009.07.018
Bundy, J. G., Paton, G. I., and Campbell, C. D. (2004). Combined microbial community level and single species biosensor responses to monitor recovery of oil polluted soil. Soil Biol. Biochem. 36 (7), 1149–1159. doi:10.1016/j.soilbio.2004.02.025
Chae, Y., Cui, R., Lee, J., and An, Y. J. (2020). Effects on photosynthesis and polyphenolic compounds in crop plant mung bean (Vigna radiata) following simulated accidental exposure to hydrogen peroxide. J. Hazard. Mater. 383 (5), 121088–121088.7. doi:10.1016/j.jhazmat.2019.121088
Chaineau, C. H., Morel, J. L., and Oudot, J. (1995). Microbial degradation in soil microcosms of fuel oil hydrocarbons from drilling cuttings. Environ. Sci. Technol. 29 (6), 1615–1621. doi:10.1021/es00006a027
Chang, Y. C., Peng, Y. P., Chen, K. F., Chen, T. Y., and Tang, C. T. (2022). The effect of different in situ chemical oxidation (ISCO) technologies on the survival of indigenous microbes and the remediation of petroleum hydrocarbon-contaminated soil. Process Saf. Environ. Prot. 163, 105–115. doi:10.1016/j.psep.2022.05.019
Chen, F. S., Zeng, D. H., Fahey, T. J., and Liao, P. F. (2010). Organic carbon in soil physical fractions under different-aged plantations of Mongolian pine in semi-arid region of Northeast China. Appl. Soil Ecol. 44 (1), 42–48. doi:10.1016/j.apsoil.2009.09.003
Chen, K. L., Wu, M. L., Ye, X. Q., Li, W., and Yuan, J. (2017). Impacts of bioremediation on microbial activities in petroleum contaminated soil[J]. J. Agro-Environment Sci. 36 (2), 279–285. doi:10.11654/jaes.2016-0991
Chen, K. Y., Tang, R. G., Luo, Y. M., Chen, Y. C., Ali, E. N., Du, J. H., et al. (2022). Transcriptomic and metabolic responses of earthworms to contaminated soil with polypropylene and polyethylene microplastics at environmentally relevant concentrations. J. Hazard. Mater. 427, 128176. doi:10.1016/j.jhazmat.2021.128176
Chouychai, W., Thongkukiatkul, A., Upatham, S., Lee, H., Pokethitiyook, P., and Kruatrachue, M. (2007). Phytotoxicity assay of crop plants to phenanthrene and pyrene contaminants in acidic soil. Environ. Toxicol. 22 (6), 597–604. doi:10.1002/tox.20285
Dallinger, A., and Horn, M. A. (2014). Agricultural soil and drilosphere as reservoirs of new and unusual assimilators of 2,4-dichlorophenol carbon. Environ. Microbiol. 16, 84–100. doi:10.1111/1462-2920.12209
Devatha, C. P., Vishal, A. V., and Rao, J. P. C. (2019). Investigation of physical and chemical characteristics on soil due to crude oil contamination and its remediation. Appl. Water Sci. 9 (4), 89. doi:10.1007/s13201-019-0970-4
Dick, R. P. (1997). Soil enzyme activities as integrative indicators of soil biological indicators for detecting changes in soil quality, 121–157.
Dindar, E., Şağban, F. O. T., and Başkaya, H. S. (2015). Variations of soil enzyme activities in petroleum-hydrocarbon contaminated soil. Int. Biodeterior. Biodegrad. 105, 268–275. doi:10.1016/j.ibiod.2015.09.011
Dindar, E., Topac, F. O., Baskaya, H. S., and Kaya, T. (2017). Effect of wastewater sludge application on enzyme activities in soil contaminated with crude oil[J]. J. Soil Sci. Plant Nutr. 17. doi:10.4067/S0718-95162017005000014
Feng, X., Duan, J. P., Pu, X. P., Zou, Y., and Li, C. R. (2008). Comparative analyses between two methods of measuring soil urease activity[J]. Grassl. turf (02), 70–72. doi:10.13817/j.cnki.cyycp.2008.02.004
Gainer, A., Hogan, N., and Siciliano, S. D. (2019). Soil invertebrate avoidance behavior identifies petroleum hydrocarbon contaminated soils toxic to sensitive plant species. J. Hazard. Mater. 361 (5), 338–347. doi:10.1016/j.jhazmat.2018.08.086
Gaskin, S., Soole, K., and Bentham, R. (2008). Screening of Australian native grasses for rhizoremediation of aliphatic hydrocarbon-contaminated soil. [J]. Int. J. Phytoremediation 10 (5), 378–389. doi:10.1080/15226510802100465
Guo, S. S., Liu, X. M., and Tang, J. C. (2022). Enhanced degradation of petroleum hydrocarbons by immobilizing multiple bacteria on wheat bran biochar and its effect on greenhouse gas emission in saline-alkali soil. Chemosphere 286 (2), 131663. doi:10.1016/j.chemosphere.2021.131663
Haq, I., and Kalamdhad, A. S. (2021). Phytotoxicity and cyto-genotoxicity evaluation of organic and inorganic pollutants containing petroleum refinery wastewater using plant bioassay. Environ. Technol. Innovation 23, 101651. doi:10.1016/j.eti.2021.101651
Harmanpreet, S., George, O., Andrew, O., and Kumar, K. (2019). Bioavailability of biosolids-borne ciprofloxacin and azithromycin to terrestrial organisms: Microbial toxicity and earthworm responses. Sci. Total Environ. 650, 18–26. doi:10.1016/j.scitotenv.2018.09.004
He, X., Guan, X., Meng, X. P., Wu, C. H., and Zhong, L. M. (2021). Rapid determination of total petroleum hydrocarbons by gas chromatography (C10∼C40) [J]. Shanxi Chem. Ind. 41 (05), 62–64. doi:10.16525/j.cnki.cn14-1109/tq.2021.05.21
Heinlaan, M., Kahru, A., Kasemets, K., Kurvet, I., Douay, F., Sepp, K., et al. (2007). Rapid screening for soil ecotoxicity with a battery of luminescent bacteria tests. Altern. Laboratory Animals Atla 35 (1), 101–110. doi:10.1177/026119290703500109
International Organization for Standardization (2004). Draft: Soil quality-avoidance test for evaluating the quality of soils and the toxicity of chemicals-Part 1: Test with earth-worms (Eisenia fetida/andrei) [S]. Geneva: International Organization for Standardization.
Jajoo, A., Mekala, N. R., Tomar, R. S., Grieco, M., and Tikkanen, M. (2014). Inhibitory effects of polycyclic aromatic hydrocarbons (PAHs) on photosynthetic performance are not related to their aromaticity. J. Photochem. Photobiol. B Biol. 137, 151–155. doi:10.1016/j.jphotobiol.2014.03.011
Jorgensen, K. S., Puustinen, J., and Suortti, A. M. (2000). Bioremediation of petroleum hydrocarbon-contaminated soil by composting in biopiles. Environ. Pollut. 107 (2), 245–254. doi:10.1016/S0269-7491(99)00144-X
Julie, K. B., Deborah, A. N., and Alison, L. S. (2002). Soil invertebrate and microbial communities, and decomposition as indicators of polycyclic aromatic hydrocarbon contamination. Appl. Soil Ecol. 21 (1), 71–88. doi:10.1016/s0929-1393(02)00023-9
Kaboosi, K. (2017). The assessment of treated wastewater quality and the effects of mid-term irrigation on soil physical and chemical properties (case study: Bandargaz-treated wastewater). Appl. Water Sci. 7, 2385–2396. doi:10.1007/s13201-016-0420-5
Kanaji, M., Yao, J., Radhika, C., Liu, H. J., Liu, W. J., Cai, M. M., et al. (2014). A combined approach of physicochemical and biological methods for the characterization of petroleum hydrocarbon-contaminated soil[J]. Environ. Sci. Pollut. Res. 21, 454. doi:10.1007/s11356-013-1923-3
Karthikeyan, M., Hussain, N., Gajalakshmi, S., and Abbasi, S. A. (2014). Effect of vermicast generated from an allelopathic weed lantana (Lantana camara) on seed germination, plant growth, and yield of cluster bean (Cyamopsis tetragonoloba)[J]. Environ. Sci. Pollut. R. 21, 12539–12548. doi:10.1007/s11356-014-3103-5
Khan, M. I., Cheema, S. A., Tang, X. J., Shen, C. F., Sahi, S. T., Jabbar, A., et al. (2012). Biotoxicity assessment of pyrene in soil using a battery of biological assays. Archive Environ. Contam. Toxicol. 63, 503–512. doi:10.1007/s00244-012-9793-0
Khan, M. I., Cheema, S. A., Tang, X. J., Hashmi, M. Z., Shen, C. F., Park, J., et al. (2013). A battery of bioassays for the evaluation of phenanthrene biotoxicity in soil. Archive Environ. Contam. Toxicol. 65, 47–55. doi:10.1007/s00244-013-9879-3
Khan, M. I., Cheema, S. A., Shen, C., Hassan, I., and Chen, Y. X. (2014). Phytotoxicity assessment of phenanthrene and pyrene in soil using two barley genotypes. Toxicol. Environ. Chem. 96 (1-2), 94–105. doi:10.1080/02772248.2014.923425
Komarova, A. V., Filimonova, I. V., and Kartashevich, A. A. (2022). Energy consumption of the countries in the context of economic development and energy transition. Energy Rep. 8 (9), 683–690. doi:10.1016/j.egyr.2022.07.072
Košnář, Z., Mercl, F., and Pavel, T. (2020). Long-term willows phytoremediation treatment of soil contaminated by fly ash polycyclic aromatic hydrocarbons from straw combustion[J]. Environ. Pollut. 264, 114787. doi:10.1016/j.envpol.2020.114787
Kumari, V., Yadav, A., Haq, I., Kumar, S., Bharagava, R. N., Singh, S. K., et al. (2016). Genotoxicity evaluation of tannery effluent treated with newly isolated hexavalent chromium reducing Bacillus cereus. J. Environ. Manag. 183 (1), 204–211. doi:10.1016/j.jenvman.2016.08.017
Kummerová, M., Lucie, V., Jana, K., and Zezulka, S. (2008). The use of physiological characteristics for comparison of organic compounds phytotoxicity. Chemosphere 71 (11), 2050–2059. doi:10.1016/j.chemosphere.2008.01.060
Kuria, N., Björnar, A. B., André, S., Sigurd, Ø., John, A. B., Hans, F. V. B., et al. (2017). Petroleum oil and mercury pollution from shipwrecks in Norwegian coastal waters. Sci. Total Environ. 593-594, 624–633. doi:10.1016/j.scitotenv.2017.03.213
Labud, V., Garcia, C., and Hernandez, T. (2007). Effect of hydrocarbon pollution on the microbial properties of a sandy and a clay soil. Chemosphere 66 (10), 1863–1871. doi:10.1016/j.chemosphere.2006.08.021
Langbehn, A., and Steinhart, H. (1995). Biodegradation studies of hydrocarbons in soils by analyzing metabolites formed. Chemosphere 30 (5), 855–868. doi:10.1016/0045-6535(94)00446-2
Li, C. M., Wang, J. N., Qiu, W. Z., and Chi, J. G. (2007). Isolation and identification of highly efficient oil-degrading bacteria and study of their degradation capability[J]. Biotechnology 17 (04), 80–82. doi:10.16519/j.cnki.1004-311x.2007.04.019
Libralato, G., Costa Devoti, A., Zanella, M., Sabbioni, E., Mičetić, I., Manodori, L., et al. (2016). Phytotoxicity of ionic, micro- and nano-sized iron in three plant species[J]. Ecotoxicol. Environ. Saf. 123, 81. doi:10.1016/j.ecoenv.2015.07.024
Lin, Z. F., Yu, H. X., Xu, S. F., and Wang, L. (2001). Modification of the Photobacterium phosphoreum toxicity test method. Environ. Sci. 22 (02), 114–117. doi:10.13227/j.hjkx.2001.02.024
Liu, W., Song, Y. F., Zhou, Q. X., Li, P. J., Sun, T. H., and Yao, D. M. (2001). Effect of chloroben-zene-stress on seed germination and seedling growth of wheat[J]. Agro-environmental Prot. 20 (2), 65–68. doi:10.3321/j.issn:1672-2043.2001.02.001
Liu, W. X., Luo, Y. M., Teng, Y., Li, Z. G., and Wu, L. H. (2006). Advances in bioremediation of petroleum contaminated soil[J]. Soil 38 (5), 634–639. doi:10.13758/j.cnki.tr.2006.05.023
Liu, W. X., Luo, Y. M., Teng, Y., Li, Z. G., and Wu, L. H. (2007). Eco-risk assessment and bioremediation of petroleum contaminated soil II. Changes in physico-chemical properties and microbial ecology of petroleum contaminated soil[J]. Acta Pedol. Sin. 44 (5), 848–853. doi:10.3321/j.issn:0564-3929.2007.05.011
Liu, S., Wang, J., Zhu, J., Wang, J., Wang, H., and Zhan, X. (2021). The joint toxicity of polyethylene microplastic and phenanthrene to wheat seedlings. Chemosphere 282, 130967. doi:10.1016/j.chemosphere.2021.130967
Loureiro, S., Soares, A., and Nogueira, A. (2005). Terrestrial avoidance behaviour tests as screening tool to assess soil contamination. Environ. Pollut. 138 (1), 121–131. doi:10.1016/j.envpol.2005.02.013
Lucia, S., Cornelis, V. G., Annamaria, R., and Giulia, M. (2012). Soil invertebrates as bioindicators of urban soil quality. Environ. Pollut. 161 (1), 57–63. doi:10.1016/j.envpol.2011.09.042
Ma, J., Shen, J., Liu, Q., Fang, F., Cai, H., and Guo, C. (2014). Risk assessment of petroleum-contaminated soil using soil enzyme activities and genotoxicity to Vicia faba. Ecotoxicology 23 (4), 665–673. doi:10.1007/s10646-014-1196-8
Ma, Y. F., Liang, Q., Ge, J. Z., and Xin, D. C. (2019). Comparison of yield formation between winter wheat jimai 22 and spring wheat jinqiang 8[J]. Crops 35 (5), 192–195. doi:10.16035/j.issn.1001-7283.2019.05.031
Makoi, J. H. J. R., and Ndakidemi, P. A. (2008). Selected soil enzymes: Examples of their potential roles in the ecosystem[J]. Afr. J. Biotrchnology 7 (3), 181–191. doi:10.5897/AJB07.590
Mansur, A. A., Adetutu, E. M., Makadia, T., Morrison, P. D., and Ball, A. S. (2015). Assessment of the hydrocarbon degrading abilities of three bioaugmentation agents for the bioremediation of crude oil tank bottom sludge contaminated Libyan soil. Int. J. Environ. Bioremediation Biodegrad. 3 (1), 1–9. doi:10.12691/ijebb-3-1-1
Margesin, R., and Schinner, F. (2000). The impact of hydrocarbon remediation on enzyme activities and microbial properties of soil[J]. Acta Biotechnol. 20 (3-4), 313–333. doi:10.1002/abio.370200312
MEEPPC (2014). Report on national general survey of soil contamination [EB/OL]. Beijing: Ministry of Ecology and Environmental of the People’s Republic of China. Available at: https://www.mee.gov.cn/gkml/sthjbgw/qt/201404/t20140417_270670_wh.htm.
National Environmental Protection Standards of the People's Republic of China (2004). The technical specification for soil environmental monitoring (HJ/T 166-2004)[S]. Available at: https://www.mee.gov.cn/image20010518/5406.pdf.
National Environmental Protection Standards of the People's Republic of China (2010). Solid waste-Extraction procedure for leaching toxicity-Horizontal vibration method (HJ 557-2010)[S]. Available at: https://www.mee.gov.cn/ywgz/fgbz/bz/bzwb/jcffbz/201002/W020111115364167460414.pdf.
National Standards of the People's Republic of China (2007). The specification for marine monitoring—Part 5: Sediment analysis (GB 17378.5) [S].
Niu, H., Wu, H., Chen, K., Sun, J., Cao, M., and Luo, J. (2021). Effects of decapitated and root-pruned Sedum alfredii on the characterization of dissolved organic matter and enzymatic activity in rhizosphere soil during Cd phytoremediation. J. Hazard. Mater. 417, 125977. doi:10.1016/j.jhazmat.2021.125977
Oboh, B. O., Adeyinka, Y., Awonuga, S., and Akinola, M. O. (2007). Impact of soil types and petroleum effluents on the earthworm, Eudrilus eugeniae. J. Environ. Biol. 28 (2), 209–212. doi:10.1016/j.jconhyd.2006.08.012
Organization for Economic Co-operation and Development (OECD) (1984). Guidelines for testing of chemicals No. 207. Earthworm, acute toxicity test[S]. Paris: Organization for Economic Co-operation and Development.
Organization for Economic Co-operation and Development (OECD) (2006). Guidelines for testing of chemicals No. 208. Terrestrial plant test: Seedling emergence and seedling growth test [S]. Paris: Organization for Economic Co-operation and Development.
Phillips, T. M., Liu, D., Seech, A. G., Lee, H., and Trevors, J. T. (2000). Monitoring bioremediation in creosote-contaminated soils using chemical analysis and toxicity tests. J. Ind. Microbiol. Biot. 24, 132–139. doi:10.1038/sj.jim.2900789
Plaza, G. A., Nalecz-Jawecki, G., Pinyakong, O., Illmer, P., and Margesin, R. (2010). Ecotoxicological and microbiological characterization of soils from heavy-metal- and hydrocarbon-contaminated sites. Environ. Monit. Assess. 163 (1-4), 477–488. doi:10.1007/s10661-009-0851-7
Riah, W., Laval, K., Laroche-Ajzenberg, E., Mougin, C., Latour, X., and Trinsoutrot-Gattin, I. (2014). Effects of pesticides on soil enzymes: A review. Environ. Chem. Lett. 12 (2), 257–273. doi:10.1007/s10311-014-0458-2
Rivas, F. J. (2006). Polycyclic aromatic hydrocarbons sorbed on soils: A short review of chemical oxidation based treatments. J. Hazard. Mater. 138 (2), 234–251. doi:10.1016/j.jhazmat.2006.07.048
Rivera-Cruz, M. C., and Trujillo-Narcía, A. (2004). Estudio de toxicidad vegetal en suelos con petroleos nuevo e intemperizado[J]. Interciencia 29 (7), 369–376.
Sample, B., Aplin, M., Efroymson, R., Suter li, G., and Welsh, C. (1997). Methods and tools for estimation of the exposure of terrestrial wildlife to contaminants. Oak Ridge, TN: Oak Ridge National Laboratory.
Sarkar, D., Ferguson, M., Datta, R., and Birnbaum, S. (2005). Bioremediation of petroleum hydrocarbons in contaminated soils: Comparison of biosolids addition, carbon supplementation, and monitored natural attenuation. Environ. Pollut. 136 (1), 187–195. doi:10.1016/j.envpol.2004.09.025
Pérez-Losada, M., Eiroa, J., and Mato, S. (2005). Phylogenetic species delimitation of the earthworms Eisenia fetida (Savigny, 1826) and Eisenia andrei Bouché, 1972 (Oligochaeta, Lumbricidae) based on mitochondrial and nuclear DNA sequences[J]. Pedobiologia 49 (4), 317–324. doi:10.1016/j.pedobi.2005.02.004
Shamshad, K., Mu, N., Eder, C. L., Zhang, S. X., Sabry, M. S., and Jorg, R. (2021). Global soil pollution by toxic elements: Current status and future perspectives on the risk assessment and remediation strategies – a review. J. Hazard. Mater. 417, 126039. doi:10.1016/j.jhazmat.2021.126039
Sheng, Y. F., Liu, Y., Wang, K. W., James, V. C., Wu, Y. C., and Zhou, Y. (2021). Ecotoxicological effects of micronized car tire wear particles and their heavy metals on the earthworm (Eisenia fetida) in soil. Sci. Total Environ. 793 (1), 148613. doi:10.1016/j.scitotenv.2021.148613
Singh Tomar, R., and Jajoo, A. (2013). A quick investigation of the detrimental effects of environmental pollutant polycyclic aromatic hydrocarbon fluoranthene on the photosynthetic efficiency of wheat (Triticum aestivum). Ecotoxicology 22 (8), 1313–1318. doi:10.1007/s10646-013-1118-1
Son, A. H., Dane, L., Balaji, S., Binoy, S., Girish, C., Kirkham, M. B., et al. (2021). Rhizoremediation as a green technology for the remediation of petroleum hydrocarbon-contaminated soils. J. Hazard. Mater. 401, 123282. doi:10.1016/j.jhazmat.2020.123282
Stella, T., Covino, S., Burianova, E., Filipová, A., Křesinová, Z., Voříšková, J., et al. (2015). Chemical and microbiological characterization of an aged PCB-contaminated soil. Sci. Total Environ. 533, 177–186. doi:10.1016/j.scitotenv.2015.06.019
Tan, X., Wang, Z., Lu, G., He, W., Wei, G., Huang, F., et al. (2017). Kinetics of soil dehydrogenase in response to exogenous Cd toxicity. J. Hazard. Mater. 329, 299–309. doi:10.1016/j.jhazmat.2017.01.055
Tang, J., Min, W., Fei, W., Sun, Q., and Zhou, Q. (2011). Eco-toxicity of petroleum hydrocarbon contaminated soil. J. Environ. Sci. 23 (5), 845–851. doi:10.1016/S1001-0742(10)60517-7
Tomar, R. S., and Jajoo, A. (2014). Fluoranthene, a polycyclic aromatic hydrocarbon, inhibits light as well as dark reactions of photosynthesis in wheat (Triticum aestivum). Ecotoxicol. Environ. Saf. 109, 110–115. doi:10.1016/j.ecoenv.2014.08.009
Trasar, C. C., Camiña, A. F., Leirós, M. C., and Gil-Sotres, F. (1999). An improved method to measure catalase activity in soils. Soil Biol. Biochem. 31 (3), 483–485. doi:10.1016/s0038-0717(98)00153-9
Varjani, S., Upasani, V. N., and Pandey, A. (2020). Bioremediation of oily sludge polluted soil employing a novel strain of Pseudomonas aeruginosa and phytotoxicity of petroleum hydrocarbons for seed germination. Sci. Total Environ. 737, 139766. doi:10.1016/j.scitotenv.2020.139766
Wang, H., Li, H. B., and Sun, T. (2011). Bioremediation of PAHs contaminated soil and its impacts on soil enzyme activity[J]. Ecol. Environ. Sci. 20 (4), 691–695. doi:10.3969/j.issn.1674-5906.2011.04.018
Wang, B., Xue, S., Liu, G. B., Zhang, G. H., Li, G., and Ren, Z. P. (2012). Changes in soil nutrient and enzyme activities under different vegetations in the Loess Plateau area, Northwest China. CATENA 92 (3), 186–195. doi:10.1016/j.catena.2011.12.004
Wang, R. J., Wang, J. L., Sun, P., Wang, D. X., and Sun, G. R. (2018). Acinetobacter calcoaceticus for petroleum degradation and its preparation method and application[P]. CN107904184A.
Wang, Y., Nie, M., Diwu, Z., Chang, F., Yin, Q., Zhang, B., et al. (2021). Toxicity evaluation of the metabolites derived from the degradation of phenanthrene by one of a soil ubiquitous PAHs-degrading strain Rhodococcus qingshengii FF. J. Hazard. Mater. 415, 125657. doi:10.1016/j.jhazmat.2021.125657
Wang, Z. Q., Fan, Z. F., Zhang, X. Y., Liu, B. L., and Chen, X. (2022). Status, trends and enlightenment of global oil and gas development in 2021. Petroleum Explor. Dev. 49 (5), 1210–1228. doi:10.1016/S1876-3804(22)60344-6
Wang, S., Cheng, F. L., Shao, Z. G., Wu, B., and Guo, S. H. (2023). Effects of thermal desorption on ecotoxicological characteristics of heavy petroleum-contaminated soil. Sci. Total Environ. 857 (1), 159405. doi:10.1016/j.scitotenv.2022.159405
Wcislo, E., Bronder, J., Bubak, A., Rodríguez-Valdés, E., and Gallego, J. L. R. (2016). Human health risk assessment in restoring safe and productive use of abandoned contaminated sites. Environ. Int. 94, 436–448. doi:10.1016/j.envint.2016.05.028
Wu, S. J., Zhang, H. X., Zhao, S. L., Wang, J. L., Li, H. L., and Chen, J. M. (2012). Biomarker responses of earthworms (Eisenia fetida) exposured to phenanthrene and pyrene both singly and combined in microcosms. Chemosphere 87 (4), 285–293. doi:10.1016/j.chemosphere.2011.11.055
Wu, B., Guo, S. H., and Wang, J. N. (2021). Spatial ecological risk assessment for contaminated soil in oiled fields. J. Hazard. Mater. 403, 123984. doi:10.1016/j.jhazmat.2020.123984
Xie, Y., Tao, G., Chen, Q., and Tian, X. (2014). Effects of perchlorate stress on growth and physiological characteristics of rice (oryza sativa L.) seedlings[J]. Water, air. Soil Pollut. 225 (8), 1–8. doi:10.1007/s11270-014-2077-8
Xu, C. B., Yang, W. J., Wei, L. S., Huang, Z. Y., Li, A. J., and Lin, A. (2020). Enhanced phytoremediation of PAHs-contaminated soil from an industrial relocation site by Ochrobactrum sp. Environ. Sci. Pollut. Res. 27 (9), 8991–8999. doi:10.1007/s11356-019-05830-7
Yla, B., Xing, W., and Zsa, B. (2020). Ecotoxicological effects of petroleum-contaminated soil on the earthworm Eisenia fetida. J. Hazard. Mater. 393, 122384. doi:10.1016/j.jhazmat.2020.122384
Yu, X. Y., Jiang, P., Zhang, H., Chen, W. Y., Xue, Y. G., and Zhang, W. Y. (2021). Assessment of acute soil toxicity and ecological risk in microwave remediation of petroleum hydrocarbon contaminated sites[J]. Environ. Chem. 40 (11), 3413–3420. doi:10.7524/j.issn.0254-6108.2020070502
Zhang, Q., Chi, J. G., Qiu, W. Z., Huang, Y. J., and Zheng, L. W. (2014). Optimization of ultrasonic disruption for PAHs-depredating enzymes from actnetobacter calcoaceticus[J]. Chin. Agric. Sci. Bull. 30 (17), 180–185. doi:10.11924/j.issn.1000-6850.2013-2325
Zhang, W., Wang, J. N., Zheng, L. W., Huang, Y. J., and Zhang, J. (2017). Study on the effect of the distribution of degrading bacteria in PAHs contaminated soil on the phytoremediation effect of microbial agents[R]. Beijing: Shandong Province, Ecology Institute of Shandong Academy of Sciences. 2023-02-23. Available at: https://kns.cnki.net/KCMS/detail/detail.aspx?dbname=SNAD&filename=SNAD000001791133.
Zhang, W., Zheng, L., Wang, J., Huang, Y., Zhang, S., Gao, Y., et al. (2018). Real-time fluorescence quantitative PCR was used to specifically detect exogenous degrading bacteria in PAHs contaminated soil[J]. Jiangsu Agric. Sci. 46 (11), 15–18. doi:10.15889/j.issn.1002-1302.2018.11.004
Zhao, S. Y., and Zhu, L. Y. (2017). Uptake and metabolism of 10:2 fluorotelomer alcohol in soil-earthworm (Eisenia fetida) and soil-wheat (Triticum aestivum L.) systems. Environ. Pollut. 220, 124–131. doi:10.1016/j.envpol.2016.09.030
Zhou, L. K., and Zhang, Z. M. (1980). Determination method of soil enzyme activity[J]. Chin. J. Soil Sci. (05), 37–38. doi:10.19336/j.cnki.trtb.1980.05.014
Keywords: soil, petroleum, bioremediation, ecotoxicity, soil enzyme activity, luminescent bacteria
Citation: Chai X, Wang M, Fu X, Zhang W, Huang Y, Germaine KJ and Wang J (2023) Shift of combined ecotoxicity index in petroleum polluted soils during a bacterial remediation. Front. Environ. Sci. 11:1141562. doi: 10.3389/fenvs.2023.1141562
Received: 10 January 2023; Accepted: 28 February 2023;
Published: 09 March 2023.
Edited by:
Guanlong Yu, Changsha University of Science and Technology, ChinaReviewed by:
Muhammad Imran Khan, University of Agriculture, Faisalabad, PakistanGaurav Saxena, Shoolini University of Biotechnology and Management Sciences, India
Copyright © 2023 Chai, Wang, Fu, Zhang, Huang, Germaine and Wang. This is an open-access article distributed under the terms of the Creative Commons Attribution License (CC BY). The use, distribution or reproduction in other forums is permitted, provided the original author(s) and the copyright owner(s) are credited and that the original publication in this journal is cited, in accordance with accepted academic practice. No use, distribution or reproduction is permitted which does not comply with these terms.
*Correspondence: Jianing Wang, d2FuZ2puQHNkYXMub3Jn