- 1State Key Laboratory of Soil Erosion and Dryland Farming on the Loess Plateau, Northwest A&F University, Shaanxi, China
- 2Institute of Soil and Water Conservation, Chinese Academy of Sciences, Shaanxi, China
Applying biochar to soil has been advocated as an effective measure to improve soil fertility and increase carbon (C) sequestration. Biochar is often co-applied with nitrogen (N) fertilizers in agricultural ecosystems, however, the interactive effects of biochar and N addition (BN) on soil greenhouse gases (GHGs) fluxes, soil C and N fractions, and yield has not been investigated. Here, we manipulated a global meta-analysis to explore the effects of biochar and N addition and their interaction on the GHGs, soil C and N fractions, and yield by assembling 75 articles. Results indicate that across all studies, biochar, N, and BN additions all increased soil CO2 emissions (8.5%–29.6%), yield (4.2%–58.2%), soil organic C (SOC, 1.8%–50.4%), dissolved organic C (DOC, 2.7%–30.0%), and total N (TN, 6.8%–15.6%), but had no significant effect on CH4 fluxes. Biochar addition reduced N2O emissions (−21.3%), global warming potential (GWP, −19.8%), greenhouse gas intensity (GHGI, −28.2%), NH4+ (−17.8%) and NO3− (−10.7%), whereas N addition increased these indexes. The interaction effects of BN on CO2 and N2O emissions, GWP, TN, and NH4+ contents were antagonistic, while CH4 emissions, DOC, MBC, NO3−, and yield exhibited synergistic responses. Notably, soil GHGs responses varied depending on geo-climatic factors, edaphic properties, biochar and N treatment parameters, and experimental scenarios. These findings indicate that the co-addition of biochar and N has the potential to mitigate climate change and improve yield, providing a valuable reference for the improvement of climate-smart agriculture.
1 Introduction
As the three major greenhouse gases (GHGs) resulting from human activities, the annual average concentrations of carbon dioxide (CO2), methane (CH4), and nitrous oxide (N2O) have risen to alarming levels of 410 ppm, 1866 ppb, and 332 ppb, respectively (IPCC, 2021). Consequently, the excessive GHGs emissions have led to a 1.09°C increase in global surface temperature between the period of 2011–2020 compared to 1850–1900 (IPCC, 2021). Nitrogen (N) addition is a common agricultural practice used to improve soil quality and crop yield (Guo et al., 2010; Shakoor et al., 2020). However, excessive N addition can trigger N leaching and enormous GHGs emissions, leading to further climate warming (Liu and Greaver, 2009; Li and Chen, 2020). Biochar, a chemically stable and highly adsorptive carbon-rich material produced by pyrolysis of biological organic materials, has demonstrated promising results in mitigating GHGs emissions (Woolf et al., 2010; Lehmann et al., 2021). Woolf et al. (2010) estimated that applying biochar to soil can offset 12% of human-induced GHGs emissions. Thus, adding biochar to N-fertilized soils could be an effective strategy to mitigate GHGs emissions while maintaining agricultural productivity.
Numerous independent studies have investigated the responses of soil GHGs to combined biochar and N (BN) addition, including positive, negative, and insignificant effects. One study reported that the application of biochar to mineral N-fertilizer soils caused a 45% increase in CO2 emissions, but had no significant effect on organic N-fertilizer soils (Liu et al., 2016). He et al. (2016) verified that biochar addition significantly promoted CH4 emissions by 11.6% in N-treated plots, but no significant change in bare soils. During the middle and late stages of maize growth, both increased and decreased N2O emissions were observed due to biochar and N addition, respectively (Edwards et al., 2018). Research has also demonstrated that a low biochar addition rate (20 t ha−1) could increase the global warming potential (GWP), whereas a high biochar addition rate (40 t ha−1) decreased it in N-fertilized soils (Liu et al., 2019b). Previous studies have suggested that biochar and N applied to soil could improve more yield (35.0%–48.4%) compared to N addition alone (Biederman and Harpole, 2013; Hu et al., 2021). The above contradictory responses may be attributed to heterogeneity in geo-climatic factors, edaphic properties, and experimental conditions of these studies, indicating the importance of considering these factors during the practical application of biochar and N addition.
The impacts of biochar and N addition on soil GHGs fluxes is widely acknowledged to be influenced by both biotic and abiotic pathways (Liu and Greaver, 2009; Zhong et al., 2016; Lehmann et al., 2021). The addition of biochar and N stimulates crop growth, leading to increased root sediments and more litter return, which in turn elevates soil respiration substrates and facilitates CO2 emissions (Alvarez, 2005; Troy et al., 2013; Jia et al., 2020). Conversely, toxic substances present in biochar (phenolic compounds and furans, etc.) and N addition-induced soil acidification may impede microbial and extracellular enzyme activities, ultimately suppressing microbial respiration (Lehmann et al., 2011; Phoenix et al., 2012). The release of CH4 is dependent on the interplay between CH4 production and oxidation, which is governed by both methanogens and methanotrophs (Inubushi et al., 2005). The application of biochar and N to soil provides additional carbon (C) sources for methanogens, resulting in higher CH4 emissions (Liu and Greaver, 2009; Singla and Inubushi, 2014). However, in N-limited regions, N addition may also alleviate the adverse effects of N limitation on methanotrophic activities, thereby accelerating CH4 uptake (Peng et al., 2019; Deng et al., 2020). Studies have also confirmed that microbial autotrophic nitrification and denitrification mechanisms are the primary pathways responsible for N2O formation (Baggs, 2011; Duan et al., 2019). Most studies suggest that N addition generally stimulates N2O emissions (Liu and Greaver, 2009; Deng et al., 2020; Du et al., 2021), while biochar addition hinders N2O production (Song et al., 2016; Liu et al., 2019a; He et al., 2021b). The addition of N fertilizer to the soil can increases the substrate availability for nitrifying and denitrifying bacteria and promote N2O emissions (Liu and Greaver, 2009), while the adsorption of biochar on soil NH4+ and NO3− reduces their availability and thus suppress N2O emissions (Cayuela et al., 2013). Therefore, understanding the impact of biochar and N interaction on soil GHGs fluxes and soil properties is critical for accurately predicting C and N cycles in terrestrial ecosystems.
To date, several meta-analyses have primarily focused on the isolated effects of N or biochar addition on soil GHGs fluxes (Ji et al., 2018; Liu et al., 2019a; Deng et al., 2020; Du et al., 2021). Only one study, with certain restriction, investigated the soil GHGs fluxes responses to the interaction between biochar and N (He et al., 2021b). It is noteworthy that soil GHGs reactions are strongly tied to both soil C and N conversion processes, thus, data on soil C and N contents are equally relevant. Additionally, the assessment of yield should not be disregarded since N and biochar additions are frequently utilized in agroecosystems. He et al. (2021b) attempted to explore the impacts of various factors on soil GHGs, but their research did not quantify the relative importance of the involved factors, nor the direct and indirect effects of predictors on soil GHGs. Hence, a global analysis examining the combined effects of biochar and N addition on soil GHGs, as well as soil C and N content, yield, and greenhouse gas intensity (GHGI), while quantifying the key factors, would be more conducive to an accurate assessment of the global soil GHGs budget.
In this study, we synthesized 870 paired observations from 75 peer-reviewed papers to address the following questions: 1) How do soil C and N contents respond to biochar and N additions worldwide? 2) What are the global responses and sensitivities of soil GHGs, yield, and GHGI to biochar and N additions? 3) What is the effect of various factors (geo-climatic factors, edaphic properties, biochar and N treatment parameters, and experimental scenarios) on soil GHGs fluxes?
2 Materials and methods
2.1 Data preparation
The relevant literature was searched using the Google Scholar, Web of Science, and CNKI databases (2010–2021) using the search terms (char/biochar) AND (nitrogen/N) AND (greenhouse gas/CO2/CH4/N2O). The selection criteria were as follows: 1) included at least four treatments simultaneously (control, N addition, biochar addition, and co-addition of biochar and N at the same site); 2) the means and standard deviations (SDs) could be obtained either directly or indirectly from the text. Ultimately, 75 peer-reviewed papers containing 870 paired observations from 71 sites worldwide were screened and analyzed (Supplementary Figure S1).
Additionally, geo-climatic factors, soil variables, biochar and N treatment parameters, and experimental scenarios were also recorded from the text (Supplementary Table S1, S2). The GWP was assessed by CH4 and N2O with conversion factors of 25 and 298, respectively (Wang et al., 2012; Liu et al., 2014). The greenhouse gas intensity (GHGI) was evaluated by dividing the GWP by yield (t ha−1). The original data in the graphs were obtained using the Get-Data software (ver. 2.20, Russian Federation).
2.2 Data analysis
2.2.1 Individual effects
The response ratio (RR, natural logs of the ratio of the mean of the treatment group to the control group) were used to evaluate the individual effect of N addition, biochar addition, or both combined (BN) (Hedges et al., 1999). The specific calculation of variance (vi) and weight (w) for each RR and the weighted mean RR (RR++) were as described by Jia et al. (2020).
The meta-analysis was conducted using the R (v.4.0.2). The 95% confidence interval (CI) for RR did not overlap with zero, indicating a significant individual effect. The percentage change of RR++ was calculated as (e RR++ −1) × 100%. Additionally, a model selection analysis was performed by using the ‘glmulti’ package within R to specify the relative importance of each predictor. Predictors with a sum of Akaike weights greater than 0.8 were considered to be the most important. Moreover, publication bias was examined by calculating the fail-safe number (Supplementary Table S3).
2.2.2 Main and interactive effects
The main effect of a factor represents the difference obtained by comparing its net effect in the presence and absence of a second factor. Following the methods of Gurevitch et al. (1992) and Crain et al. (2008), Hedge’s d was used to assess the main effect sizes of either N (dN) or biochar addition (dB) on the variables as well as their interactions (dI), calculated as follows:
where
The variance of dI (
where i was the number of groups, k was the number of comparisons in the ith group, and w was the weight (reciprocal of the variances).
The interaction effects of biochar and N addition showed three types: additive, antagonistic, and synergistic. If the 95% CI overlap was zero, the interactive effect was considered additive. When the individual effects were in the opposite direction or both negative, the interactions >0 were denoted antagonistic (<0 were synergistic). If both individual effects were positive, the interactions >0 were denoted synergistic (<0 were antagonistic) (Crain et al., 2008; Yue et al., 2017).
3 Results
3.1 The impact of biochar and N additions on GHGs fluxes and soil properties
The results showed that the addition of biochar, N, and BN all significantly increased soil CO2 emissions (+8.5%, +28.9%, and +29.6%) and yield (+4.2%, +53.7%, and +58.2%), respectively, but had no significant effect on CH4 fluxes (Figure 1). The soil N2O emissions and GWP were significantly increased under the N and BN treatments, while biochar addition reduced them.
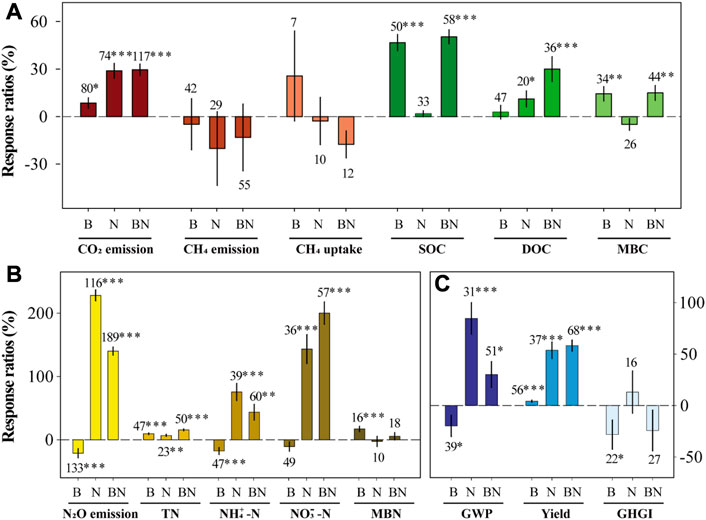
FIGURE 1. Effects of biochar, nitrogen (N), and combined biochar and N (BN) additions on soil carbon cycle (A), N cycle (B) and GHGI (C).
The addition of biochar, N, and BN also significantly increased the soil SOC, dissolved organic carbon (DOC), and TN (Figure 1). Biochar addition significantly increased soil pH, microbial biomass C (MBC), microbial biomass N (MBN), cation exchange capacity (CEC), ammonia-oxidizing bacteria (AOB), nirS, and nosZ, but decreased NH4+-N (Supplementary Figures S1, S2). N addition alone increased soil NH4+-N and NO3−-N, but decreased soil pH and nosZ. The combined addition of BN significantly increased soil MBC, CEC, AOB, and nosZ, but reduced soil bulk density.
3.2 Main and interactive effects of two factors on GHGs fluxes and soil properties
The main effects results showed that biochar addition significantly promoted CO2 emissions and yield, while reducing CH4 uptakes, N2O emissions, GWP, and GHGI (Figure 2). The main effects of N addition were increased CO2 and N2O emissions, GWP, and yield, in tandem to decreased soil CH4 fluxes and GHGI.
The interactive effects showed that the combined addition of biochar and N had antagonistic effects on CO2 and N2O emissions and GWP, synergistic effects on CH4 emissions and yield, and additive effects on soil CH4 uptakes and GHGI (Figure 2). The antagonistic effects of CO2 and N2O emissions and GWP accounted for 46.5%, 51.1%, and 30.0%, respectively. The synergy ratios of CH4 emissions and yield were 2.1% and 36.0%, respectively. Moreover, BN addition produced synergistic effects on DOC, MBC, and NO3−-N, but produced antagonistic effects on TN and NH4+-N.
3.3 Drivers of soil GHGs, GWP, yield, and GHGI
The results showed that the climate and soil factors, biochar and N treatment parameters, and experimental scenarios (land-use, experimental method, and duration) were closely related to soil GHGs (Figures 3, 4), GWP, yield, and GHGI (Supplementary Table S4 and Supplementary Figure S3). The model selection analysis revealed that the N forms, SOC, STN, biochar pyrolysis temperature, and soil pH were key predictors involved in BN treatment, explaining 47% of the CO2 emissions variability in the structural equation model (SEM) (Figures 5A, D). Additionally, BN addition stimulated higher CO2 emissions in paddy fields added with organic fertilizers (Figure 3A).
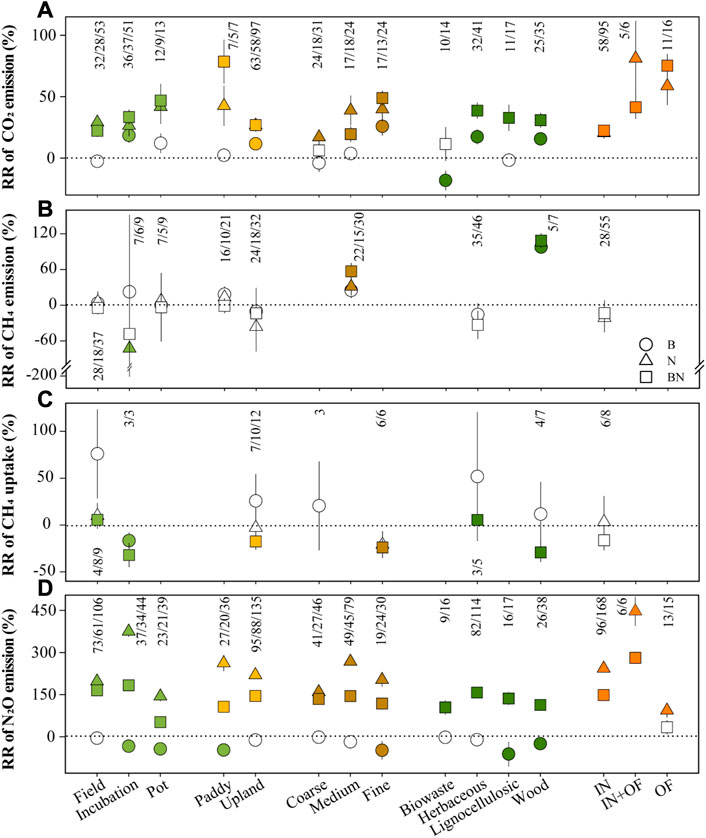
FIGURE 3. The responses of soil CO2 emission (A), CH4 emission (B), CH4 uptake (C) and N2O emission (D) to predictors under BN addition.
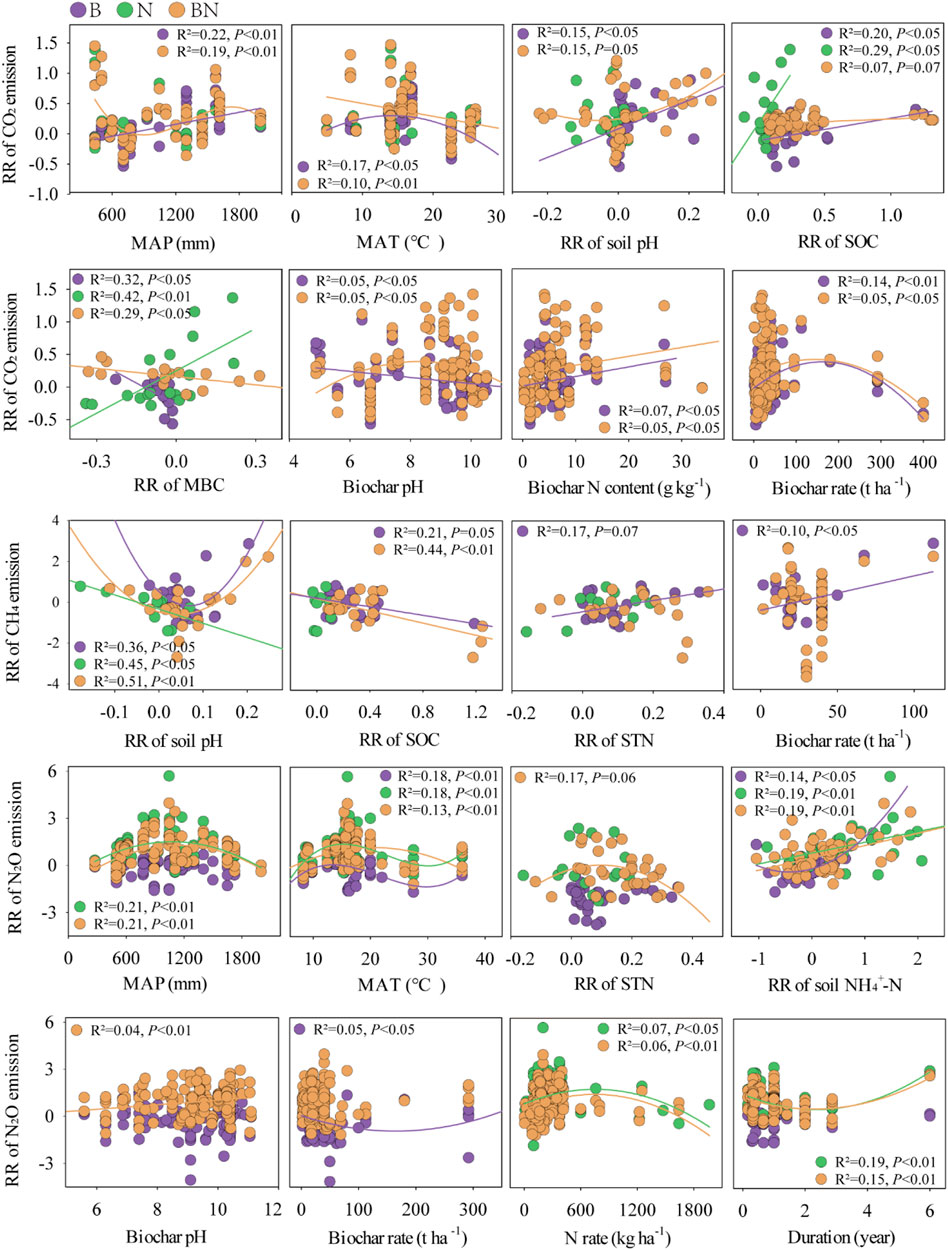
FIGURE 4. Relationships between the predictors and the responses of soil GHGs. MAP, mean annual precipitation; MAT, mean annual temperature.
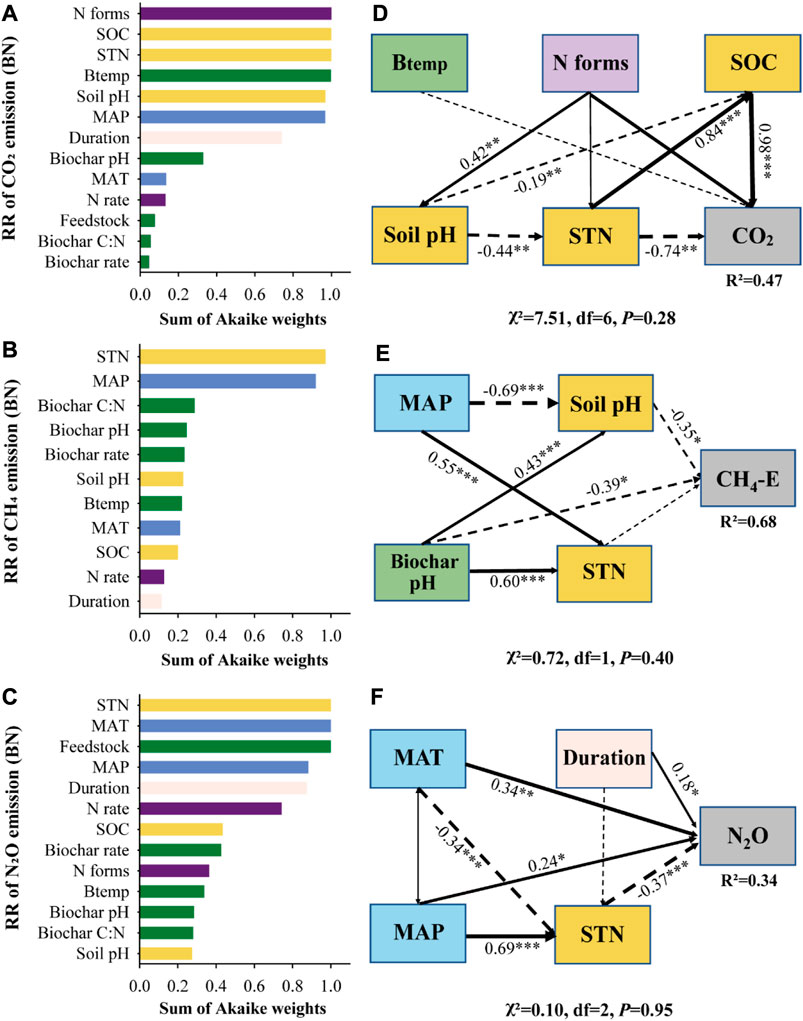
FIGURE 5. Model-averaged importance of the predictors of BN addition impacts on soil GHGs (A–C). The structural equation model (SEM) examining the indirect and direct effects of predictors on soil GHGs (D–F). MAP, mean annual precipitation; MAT, mean annual temperature; SOC: soil organic carbon; B temp: biochar pyrolysis temperature.
The SEM indicated that MAP, soil pH, biochar pH, and STN explained 68% of the variability in CH4 emissions, with STN and MAP being the dominant factors in the context of BN treatment (Figures 5B, E). Similarly, STN was also found to be the key factor determining CH4 emissions under N treatment (Supplementary Figure S4B). In medium-textured soils, BN addition produced more CH4 emissions than N addition alone (Figure 3B). Herbaceous-derived biochar significantly increased CH4 uptakes, while wood-derived biochar suppressed it in N-fertilized soils (Figure 3C).
The MAT, MAP, duration, and STN directly affected 34% of the N2O emissions variability under BN treatment (Figure 5F). The MAT was a key predictor of N2O emissions under the N and BN treatments (Figure 5 and Supplementary Figure S4), and a significant relationship between MAT and N2O emissions was also observed (Figure 4). The results showed that following the addition of BN, the pot experiments resulted in lower N2O emissions compared to the field experiments (Figure 3D).
Both MAP and MAT were significantly negatively correlated with GWP, yield, and GHGI under the N and BN treatments (Supplementary Table S4). The GWP decreased with increasing soil pH under the biochar and BN treatments. Adding biochar and N to paddy fields or fine-textured soil could greatly boost their yield (Supplementary Figure S3).
4 Discussion
4.1 Individual effects of the single or combined addition of biochar and N on soil GHGs, yield, and GHGI
Overall, biochar addition significantly promoted CO2 emissions, which was a finding consistent with earlier meta-analyses (He et al., 2016; Song et al., 2016). Functioning as exogenous C input, biochar can enrich soil organic matter status, promote the release of inorganic C, and stimulate microbial metabolic activity, thereby augmenting available substrates for soil respiration (Smith et al., 2010; Luo et al., 2011; Omondi et al., 2016; He et al., 2021b). Although our results showed that biochar application significantly increased SOC (46.7%) and MBC (14.4%) and insignificantly increased DOC content (2.7%) (Figure 1), we only observed a significant positive relationship between SOC and CO2 emissions (Figure 4), suggesting that biochar-induced increase in CO2 emissions were mainly related to changes in SOC content in the current study. Compared with biochar addition alone, N and BN additions facilitated more CO2 emissions, potentially due to the significant increase in soil DOC content (11.2% for N addition; 30.0% for BN addition; Figure 1). In addition, our findings indicated that N and BN additions also greatly enriched soil NH4+-N and NO3−-N (Figure ), leading to higher N availability and thus, promoting microbial C mineralization in the soil (Lu et al., 2011; Zhou et al., 2013).
The current meta-analysis showed that biochar addition mitigated N2O emissions, and conversely, N and BN additions greatly stimulated it, consistent with the results of He et al. (2021b). It is well understood that N-fertilized soils provide abundant available substrates for nitrifying and denitrifying bacteria, which ultimately results in increased N2O emissions (Deng et al., 2020). This possibility is supported by the current positive relationship between the increased soil NH4+-N contents and N2O emissions under the N and BN treatments (Figures 1, 4). Moreover, the reduction in N2O emissions caused by biochar may be attributed to its liming effect. Increased soil pH may promote N2O reductase activities, resulting in lower N2O emissions (Clough et al., 2013), and we actually found an increase in nosZ (Supplementary Figure S2). Alternatively, biochar adsorbed soil NH4+-N and NO3−-N contents, thus reducing the available substrates for soil nitrifying and denitrifying microbes, ultimately hindering soil N2O emissions rates (Cheng et al., 2008; Cayuela et al., 2013). The results showed that biochar addition alone actually reduced soil NH4+-N and NO3−-N (Figure 1), and the RRs of NH4+-N and NO3−-N decreased with increasing biochar application rates (Supplementary Figure S5).
The soil GWP was assessed jointly by the CH4 and N2O fluxes, and the results suggest that biochar addition did not significantly alter CH4 fluxes (Figure 1), thus, the reduction in biochar-induced GWP was mainly due to less N2O emissions. Similarly, the increase in GWP resulting from the addition of N or BN stems from higher N2O emissions (Figure 1). Moreover, the results found that the addition of biochar, N, and BN all significantly boosted crop yield, which was closely related to improved N availability (Supplementary Table S4). Consequently, the positive effects of crop yield (4.2%) coupled with decreased soil GWP (−19.8%) led to lower soil GHGI (−28.2%) in the biochar-amended plots.
4.2 Interactive effects of biochar and N application on soil GHGs, yield, and GHGI
Antagonistic effects indicate that the combined effect of two factors is weaker than the sum of their individual effects (Coors and Meester, 2010; Zhou et al., 2016). Our study showed that combined BN addition promoted CO2 emissions by 29.6%, which was less than the sum of the two individual effects (37.4%, Figure 1). Antagonistic effects of the biochar-N interaction on CO2 emissions aligned with the meta-analysis of He et al. (2021b), which may be attributable to accompanying reduced soil microbial respiration rates (Iqbal et al., 2009; Zhang et al., 2012; Zhang et al., 2021). Previous research has found that N inhibition of white-rot fungi could reduce phenoloxidase activity, thereby impairing soil microbial respiration (Frey et al., 2004). The adsorption of inorganic N by biochar can reduce N availability for microbes (Steiner et al., 2008; Clough et al., 2013). The current indeed found a significant decrease in soil NH4+-N content due to the biochar-N interaction (Figure 2).
Our findings reveal a synergistic effect of biochar-N interaction on CH4 emissions, contradicting those of a recent meta-analysis (He et al., 2021b). This discrepancy may be attributed to the differences in sample size between the studies, and our results may be more convincing science our observations (n = 48) are larger than theirs (n = 33). Non-etheless, there were some similar results where the interaction of biochar with N significantly reduced CH4 emissions. Other studies also demonstrated that biochar reduced CH4 emissions in rice fields, especially in N-fertilized plots (Xie et al., 2013; Qin et al., 2016; Liu et al., 2019b). The existing empirical evidence suggests that biochar-induced increases in soil pH and aeration favor methanotrophs, thereby reducing mcrA/pmoA and ultimately suppressing CH4 emissions (Feng et al., 2012). Our study demonstrated that BN addition slightly increased the soil pH by 2.0% and significantly decreased the soil bulk density by 4.7% (Supplementary Figure S2).
The observed mitigative and antagonistic effects of the biochar-N interaction on N2O emissions indicate that the biochar-induced reduction in N2O emissions exceeded the N-induced increase in N2O emissions, possibly due to the alteration in soil pH and NH4+-N levels (Figure 2). The abundant alkaline cations provided by biochar raised the soil pH, which stimulated N2O reductase activities and facilitated the complete denitrification of N2O to N2 (Cayuela et al., 2013). Our results showed that BN addition slightly increased soil pH and greatly increased nosZ by 40.3% (Supplementary Figure S2). The above-mentioned immobilization of soil NH4+-N by biochar also reduced the available substrates for soil nitrifying bacteria, ultimately hindering the N2O emissions rate (Cheng et al., 2008). The reduced soil bulk density caused by the biochar-N interaction increased the soil aeration (Supplementary Figure S2), thereby inhibiting the soil denitrification process and slowing down N2O production (Simek et al., 2002).
The biochar-N interaction exhibited an antagonistic effect on GWP, but a synergistic effect on yield. It is well understood that reduced soil GWP results from lower CH4, and N2O emissions (Figure 2). Adding N fertilizer to biochar-treated soil can enrich the nutrient supply and improve nutrient use efficiency, thus promoting crop yield (Hu et al., 2021; Kotuš et al., 2022). In this study, the findings of increased DOC, MBC, and NO3−-N provide some support for this explanation under the interactive addition of biochar and N (Figure 2).
4.3 Factors affecting the response of soil GHGs fluxes
Existing empirical evidence suggests that the effects of N and biochar addition on soil GHGs fluxes are diverse, with the degree and direction of responses varying with geo-climatic factors, edaphic properties, biochar and N treatment parameters, and experimental scenarios (Liu et al., 2018; Deng et al., 2020; He et al., 2021b).
4.3.1 Climates and edaphic factors
Model selection analysis revealed that the MAP and MAT were key predictors of soil GHGs emissions under BN treatment (Figure 5). The MAP and MAT could change the soil microhabitats by altering the soil temperature and moisture, thus affecting GHGs fluxes (Yan et al., 2022; Yang et al., 2022). Most preexisting studies have concluded that SOC decreases with increasing temperature (Hontoria et al., 1999; Lal, 2004), leading to reduced available substrates for soil respiration (Brye et al., 2016). Similarly, we observed that CO2 emissions decreased with increasing MAT under BN treatment (Figure 4), possibly related to temperature-induced changes in SOC. The emission and uptake of CH4 are influenced by the joint activities of methanotrophs and methanogens (Inubushi et al., 2005), and changes in soil moisture caused by rainfall affect soil aeration status (Yang et al., 2022). The SEM showed that MAP and MAT directly affected N2O emissions (Figure 5), and both displayed a parabolic relationship with N2O emissions (Figure 4), indicating the threshold effects of MAP and MAT on N2O emissions under the BN treatment.
The addition of biochar and N each stimulated greater CO2 emissions in fine-textured soils compared to coarse-textured soils (Figure 3), consistent with the finding of He et al. (2021b). Existing empirical evidence posits that SOC increases with silt and clay contents but decreases with sand content (Zinn et al., 2005; Gami et al., 2009). Consequently, fine-textured soil can provide more available substrates for soil organic matter mineralization and microbial survival, thereby resulting in higher CO2 emissions (Figure 4), and the SEM actually revealed the direct impact of SOC on CO2 emissions (Figure 5A). Our results showed that the initial N content of the soil was also a key factor affecting CH4 emissions under biochar and BN additions (Figure 5 and Supplementary Figure S4), and higher soil N content resulted in more CH4 emissions (Figure 4), as N-rich soil provides a greater nutrient supply for methanogens. It is well understood that N2O emissions increase with increasing soil NH4+-N due to more available substrates for the nitrification process (Figure 4).
4.3.2 Biochar and N treatment parameters
Biochar feedstock and its application rates can modulate soil feedback, thereby affecting soil GHGs fluxes (Li et al., 2018; Zhang et al., 2019). Our results showed that biowaste-derived biochar suppressed CO2 emissions, whereas biochar produced from herbaceous or wood pyrolysis stimulated it (Figure 3). The poor C content of biowaste-derived biochar can impair the mineralization rate of easily decomposable C from this biochar itself (Liu et al., 2019a). We observed a threshold effect of the biochar application rates on CH4 emissions, with biochar application rates exceeding 22.7 t ha−1 promoting CH4 emissions (Figure 4), as more biochar supply enriches the available substrates for methanogens (Singla and Inubushi, 2014). Additionally, the lignocellulosic/wood-derived biochar mitigated N2O emissions, but biowaste-derived biochar had no significant effect on it, which is likely attributable to the lesser surface area and weaker aromatic structure of biowaste-derived biochar (Mandal et al., 2016).
Moreover, N forms and their application rates were also crucial predictors of GHGs responses (Figure 5 and Supplementary Figure S4). The combined addition of organic and inorganic N greatly promoted CO2 and N2O emissions compared to inorganic N addition alone (Figure 3). The abundant C produced by the decomposition of organic fertilizers can provide nutrient and cellular energy for microbial respiration and denitrifying bacteria, thereby promoting the rate of denitrification (Clough et al., 2013; Xie et al., 2013). Furthermore, the meta-regression analysis showed a significant negative relationship between CH4 uptake and the N application rates (data not shown), which is similar to previous findings (Robertson, 2006; Zhang et al., 2008). The accumulation of soil NO3−-N and NH4+-N due to high N input reduces soil pH, which is toxic to methanotrophs, and thus inhibits CH4 uptakes (Gulledge et al., 2004; Deng et al., 2020).
4.3.3 Experimental scenarios
Different experimental scenarios (including alterations in the experimental method, duration, and land use) affect the soil bulk density, oxygen partial pressure, and nutrient availability, and ultimately alter soil GHGs (Vargas et al., 2012; Li et al., 2018). Our results showed that BN addition stimulated more CO2 emissions in incubation/pot experiments than in the fields (Figure 3), which is in line with the findings of Song et al. (2016), suggesting that the increased CO2 emissions was mainly contributed by the incubation/pot experiment. This discrepancy may be attributed to differences in fertilizer application rates, experimental durations, and soil hydrology between the field and incubation/pot experiments.
The result showed that CH4 uptake decreased with increasing trial duration (data not shown). Spokas (2013) found that the stable structure of field-aged biochar was partially destroyed, making it easier for methanogens to decompose and utilize, thus promoting CH4 production and reducing CH4 absorption. Similarly, a longer experimental duration was found to be not conducive to N2O emissions mitigation (Figure 4). He et al. (2021a) found that continuous N application for 30 years had a stronger stimulating effect on the gross rate of nitrogen mineralization and autotrophic nitrification. Conversely, The destruction of the aromatic structure of the weathered biochar and the increased content of acidic functional groups weakened the electron transfer ability in the denitrification process (Jose et al., 2018). Therefore, the stimulation of N2O emissions by aging biochar may be attributed to the nitrification pathway.
Moreover, adding biochar and N to the paddy field stimulated more CO2 emissions than in upland fields (Figure 3), which is consistent with an earlier meta-analysis (Liu et al., 2016). It is generally accepted that paddy field soils have higher initial organic matter and N application rates, which may account for the more positive CO2 emissions response. Consequently, high N addition alone stimulated more N2O emissions in paddy fields compared to upland fields. However, adding N to biochar-treated soil produced more N2O in the upland. These heterogeneous results imply that both fertilization methods and land use types need to be considered when formulating effective climate mitigation strategies.
5 Conclusion
In the current meta-analysis, the individual effects, main effects, and interaction effects of biochar and N addition were examined simultaneously (Figure 6). The results showed that biochar, N, and BN additions all increased CO2 emissions, SOC, DOC, TN, and yield, respectively, but caused no change in CH4 fluxes. N and BN additions stimulated N2O emissions and GWP, while biochar addition inhibited them. In addition, the effect of the biochar-N interaction on the soil GHGs was mainly antagonistic, while its effect on yield was synergistic. It is worth noting that the responses of soil GHGs were also affected by geo-climatic factors, edaphic properties, biochar and N treatment parameters, and experimental scenarios. Applying herbal biochar and N to fine-textured soils may produce better economic and environmental benefits. Furthermore, global climate change factors (such as warming, drought events, and elevated CO2 concentrations) could interact with biochar/N addition to jointly affect soil GHGs, and the long-term persistence of biochar-N interactions also needs to be further verified.
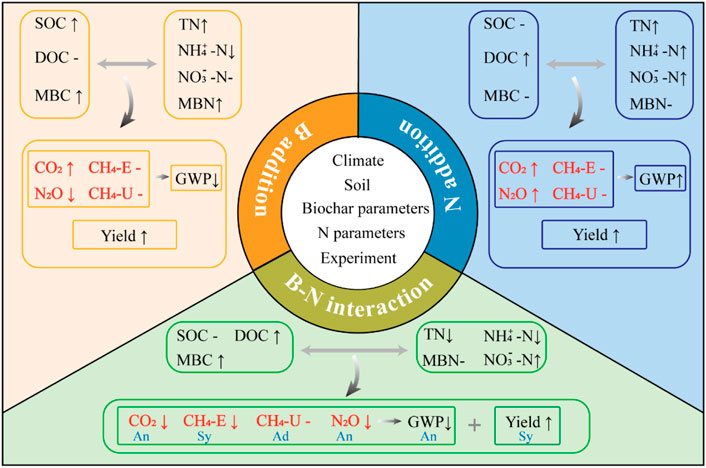
FIGURE 6. Potential mechanisms for the effects of biochar-N interactions on soil GHGs fluxes. An, Sy, and Ad indicate antagonistic, synergistic, and additive, respectively.
Data availability statement
The original contributions presented in the study are included in the article/Supplementary Material, further inquiries can be directed to the first author.
Author contributions
XJ: Conceptualization, Methodology, formal analysis, investigation, writing—original draft. WY: Software, validation, supervision, writing—review and editing. HM: Investigation, resources. ZS: Conceptualization, investigation, writing—review and editing.
Funding
The National Natural Science Foundation of China (42077452), the Natural Science Basic Research Plan in Shaanxi Province of China (2023-JC-YB-182) and the Fundamental Research Funds for the Central Universities (2452017233) provided financial support for this study.
Conflict of interest
The authors declare that the research was conducted in the absence of any commercial or financial relationships that could be construed as a potential conflict of interest.
Publisher’s note
All claims expressed in this article are solely those of the authors and do not necessarily represent those of their affiliated organizations, or those of the publisher, the editors and the reviewers. Any product that may be evaluated in this article, or claim that may be made by its manufacturer, is not guaranteed or endorsed by the publisher.
Supplementary material
The Supplementary Material for this article can be found online at: https://www.frontiersin.org/articles/10.3389/fenvs.2023.1123897/full#supplementary-material
References
Alvarez, R. (2005). A review of nitrogen fertilizer and conservation tillage effects on soil organic carbon storage. Soil Use Manag. 21, 38–52. doi:10.1111/j.1475-2743.2005.tb00105.x
Baggs, E. M. (2011). Soil microbial sources of nitrous oxide: Recent advances in knowledge, emerging challenges and future direction. Curr. Opin. Environ. Sustain. 3, 321–327. doi:10.1016/j.cosust.2011.08.011
Biederman, L. A., and Harpole, W. S. (2013). Biochar and its effects on plant productivity and nutrient cycling: A meta-analysis. GCB Bioenergy 5, 202–214. doi:10.1111/gcbb.12037
Brye, K. R., Mcmullen, R. L., Silveira, M. L., Motschenbacher, J., Smith, S. F., Gbur, E. E., et al. (2016). Environmental controls on soil respiration across a southern US climate gradient: A meta-analysis. Geoderma Reg. 7, 110–119. doi:10.1016/j.geodrs.2016.02.005
Cayuela, M. L., Sánchez-Monedero, M., Roig, A., Hanley, K., Enders, A., and Lehmann, J. (2013). Biochar and denitrification in soils: When, how much and why does biochar reduce N2O emissions? Sci. Rep. 3, 1732. doi:10.1038/srep01732
Cheng, C. H., Lehmann, J., and Engelhard, M. H. (2008). Natural oxidation of black carbon in soils: Changes in molecular form and surface charge along a climosequence. Geochimica Cosmochimica Acta 72, 1598–1610. doi:10.1016/j.gca.2008.01.010
Clough, T., Condron, L., Kammann, C., and Müller, C. (2013). A review of biochar and soil nitrogen dynamics. Agronomy 3, 275–293. doi:10.3390/agronomy3020275
Coors, A., and Meester, L. D. (2010). Synergistic, antagonistic and additive effects of multiple stressors: Predation threat, parasitism and pesticide exposure in Daphnia magna. J. Appl. Ecol. 45, 1820–1828. doi:10.1111/j.1365-2664.2008.01566.x
Crain, C. M., Kroeker, K., and Halpern, B. S. (2008). Interactive and cumulative effects of multiple human stressors in marine systems. Ecol. Lett. 11, 1304–1315. doi:10.1111/j.1461-0248.2008.01253.x
Deng, L., Huang, C., Kim, D., Shangguan, Z., Wang, K., Song, X., et al. (2020). Soil GHG fluxes are altered by N deposition: New data indicate lower N stimulation of the N2O flux and greater stimulation of the calculated C pools. Glob. Change Biol. 26, 2613–2629. doi:10.1111/gcb.14970
Du, Y., Ke, X., Li, J., Wang, Y., Cao, G., Guo, X., et al. (2021). Nitrogen deposition increases global grassland N2O emission rates steeply: A meta-analysis. Catena 199, 105105. doi:10.1016/j.catena.2020.105105
Duan, P., Zhang, Q., Zhang, X., and Xiong, Z. (2019). Mechanisms of mitigating nitrous oxide emissions from vegetable soil varied with manure, biochar and nitrification inhibitors. Agric. For. Meteorology 278, 107672. doi:10.1016/j.agrformet.2019.107672
Edwards, J. D., Pittelkow, C. M., Kent, A. D., and Yang, W. H. (2018). Dynamic biochar effects on soil nitrous oxide emissions and underlying microbial processes during the maize growing season. Soil Biol. Biochem. 122, 81–90. doi:10.1016/j.soilbio.2018.04.008
Feng, Y., Xu, Y., Yu, Y., Xie, Z., and Lin, X. (2012). Mechanisms of biochar decreasing methane emission from Chinese paddy soils. Soil Biol. Biochem. 46, 80–88. doi:10.1016/j.soilbio.2011.11.016
Frey, S. D., Knorr, M., Parrent, J. L., and Simpson, R. T. (2004). Chronic nitrogen enrichment affects the structure and function of the soil microbial community in temperate hardwood and pine forests. For. Ecol. Manag. 196, 159–171. doi:10.1016/s0378-1127(04)00198-7
Gami, S. K., Lauren, J. G., and Duxbury, J. M. (2009). Influence of soil texture and cultivation on carbon and nitrogen levels in soils of the eastern Indo-Gangetic Plains. Geoderma 153, 304–311. doi:10.1016/j.geoderma.2009.08.003
Gulledge, J., Hrywna, Y., Cavanaugh, C., and Steudler, P. A. (2004). Effects of long-term nitrogen fertilization on the uptake kinetics of atmospheric methane in temperate forest soils. Fems Microbiol. Ecol. 49, 389–400. doi:10.1016/j.femsec.2004.04.013
Guo, J. H., Liu, X. J., Zhang, Y., Shen, J. L., Han, W. X., Zhang, W. F., et al. (2010). Significant acidification in major Chinese croplands. Science 327, 1008–1010. doi:10.1126/science.1182570
Gurevitch, J., Morrow, L. L., Wallace, A., and Walsh, J. S. (1992). A meta-analysis of competition in field experiments. Am. Nat. 140, 539–572. doi:10.1086/285428
He, M., Xin, X., Meng, L., Yan, X., Zhao, C., Cai, Z., et al. (2021a). Long-term appropriate N management can continuously enhance gross N mineralization rates and crop yields in a maize-wheat rotation system. Biol. Fertil. Soils, 1–11. doi:10.1007/s00374-021-01595-9
He, Y., Yao, Y., Jia, Z., Chen, X., Zhou, L., Shao, J., et al. (2021b). Antagonistic interaction between biochar and nitrogen addition on soil greenhouse gas fluxes: A global synthesis. GCB Bioenergy 13, 1636–1648. doi:10.1111/gcbb.12878
He, Y., Zhou, X., Jiang, L., Li, M., Du, Z., Zhou, G., et al. (2016). Effects of biochar application on soil greenhouse gas fluxes: A meta-analysis. GCB Bioenergy 9, 743–755. doi:10.1111/gcbb.12376
Hedges, L. V., Gurevitch, J., and Curtis, P. S. (1999). The meta-analysis of response ratios in experimental ecology. Ecology 80, 1150–1156. doi:10.1890/0012-9658(1999)080[1150:tmaorr]2.0.co;2
Hontoria, C., Saa, A., and Rodríguez-Murillo, J. C. (1999). Relationships between soil organic carbon and site characteristics in peninsular Spain. Soil Sci. Soc. Am. J. 63, 614–621. doi:10.2136/sssaj1999.03615995006300030026x
Hu, X., Cai, A., Wu, D., Liang, G., Xiao, J., Xu, M., et al. (2021). Effects of biochar application on crop productivity, soil carbon sequestration, and global warming potential controlled by biochar C: N ratio and soil pH: A global meta-analysis. Soil Tillage Res. 213, 105125. doi:10.1016/j.still.2021.105125
Inubushi, K., Otake, S., Furukawa, Y., Shibasaki, N., Ali, M., Itang, A. M., et al. (2005). Factors influencing methane emission from peat soils: Comparison of tropical and temperate wetlands. Nutrient Cycl. Agroecosyst. 71, 93–99. doi:10.1007/s10705-004-5283-8
IPCC (2021). Summary for policymakers. Clmate change 2021: The physical science basis. Cambridge, UK: Cambridge University Press.
Iqbal, J., Hu, R., Shan, L., Hatano, R., Du, L., Lu, L., et al. (2009). CO2 emission in a subtropical red paddy soil (Ultisol) as affected by straw and N-fertilizer applications: A case study in southern China. Agric. Ecosyst. Environ. 131, 292–302. doi:10.1016/j.agee.2009.02.001
Ji, C., Jin, Y. G., Li, C., Chen, J., Kong, D. L., Yu, K., et al. (2018). Variation in soil methane release or uptake responses to biochar amendment: A separate meta-analysis. Ecosystems 21, 1692–1705. doi:10.1007/s10021-018-0248-y
Jia, X., Zhong, Y., Liu, J., Zhu, G., and Yan, W. (2020). Effects of nitrogen enrichment on soil microbial characteristics: From biomass to enzyme activities. Geoderma 366, 114256. doi:10.1016/j.geoderma.2020.114256
Jose, M. d. l. R., Rosado, M., Paneque, M., Miller, A. Z., and Knicker, H. (2018). Effects of aging under field conditions on biochar structure and composition: Implications for biochar stability in soils. Sci. Total Environ. 613, 969–976. doi:10.1016/j.scitotenv.2017.09.124
Kotuš, T., Šimanský, V., Drgoňová, K., Illéš, M., Wójcik-Gront, E., Balashov, E., et al. (2022). Combination of biochar with N-fertilizer affects properties of soil and N2O emissions in maize crop. Agronomy-Basel 12, 1314. doi:10.3390/agronomy12061314
Lal, R. (2004). Soil carbon sequestration to mitigate climate change. Geoderma 123, 1–22. doi:10.1016/j.geoderma.2004.01.032
Lehmann, J., Cowie, A., Masiello, C. A., Kammann, C., Woolf, D., Amonette, J. E., et al. (2021). Biochar in climate change mitigation. Nat. Geosci. 14, 883–892. doi:10.1038/s41561-021-00852-8
Lehmann, J., Rillig, M. C., Thies, J., Masiello, C. A., Hockaday, W. C., and Crowley, D. (2011). Biochar effects on soil biota - a review. Soil Biol. Biochem. 43, 1812–1836. doi:10.1016/j.soilbio.2011.04.022
Li, S., and Chen, G. (2020). Contemporary strategies for enhancing nitrogen retention and mitigating nitrous oxide emission in agricultural soils: Present and future. Environ. Dev. Sustain. 22, 2703–2741. doi:10.1007/s10668-019-00327-2
Li, Y., Hu, S., Chen, J., Müller, K., Li, Y., Fu, W., et al. (2018). Effects of biochar application in forest ecosystems on soil properties and greenhouse gas emissions: A review. J. Soils Sediments 18, 546–563. doi:10.1007/s11368-017-1906-y
Liu, L., and Greaver, T. L. (2009). A review of nitrogen enrichment effects on three biogenic GHGs: The CO2 sink may be largely offset by stimulated N2O and CH4 emission. Ecol. Lett. 12, 1103–1117. doi:10.1111/j.1461-0248.2009.01351.x
Liu, Q., Liu, B., Zhang, Y., Hu, T., Lin, Z., Liu, G., et al. (2019a). Biochar application as a tool to decrease soil nitrogen losses (NH3 volatilization, N2O emissions, and N leaching) from croplands: Options and mitigation strength in a global perspective. Glob. Change Biol. 25, 2077–2093. doi:10.1111/gcb.14613
Liu, Q., Zhang, Y., Liu, B., Amonette, J. E., Liu, Z., Liu, G., et al. (2018). How does biochar influence soil N cycle? A meta-analysis. Plant Soil 426, 211–225. doi:10.1007/s11104-018-3619-4
Liu, S., Zhang, Y., Zong, Y., Hu, Z., Wu, S., Zhou, J., et al. (2016). Response of soil carbon dioxide fluxes, soil organic carbon and microbial biomass carbon to biochar amendment: A meta-analysis. GCB Bioenergy 8, 392–406. doi:10.1111/gcbb.12265
Liu, X., Ye, Y., Liu, Y., Zhang, A., Zhang, X., Li, L., et al. (2014). Sustainable biochar effects for low carbon crop production: A 5-crop season field experiment on a low fertility soil from central China. Agric. Syst. 129, 22–29. doi:10.1016/j.agsy.2014.05.008
Liu, X., Zhou, J., Chi, Z., Zheng, J., Li, L., Zhang, X., et al. (2019b). Biochar provided limited benefits for rice yield and greenhouse gas mitigation six years following an amendment in a fertile rice paddy. Catena 179, 20–28. doi:10.1016/j.catena.2019.03.033
Lu, M., Zhou, X., Luo, Y., Yang, Y., Fang, C., Chen, J., et al. (2011). Minor stimulation of soil carbon storage by nitrogen addition: A meta-analysis. Agric. Ecosyst. Environ. 140, 234–244. doi:10.1016/j.agee.2010.12.010
Luo, Y., Durenkamp, M., De Nobili, M., Lin, Q., and Brookes, P. C. (2011). Short term soil priming effects and the mineralisation of biochar following its incorporation to soils of different pH. Soil Biol. Biochem. 43, 2304–2314. doi:10.1016/j.soilbio.2011.07.020
Mandal, S., Sarkar, B., Bolan, N., Novak, J., Yong, S., Van Zwieten, L., et al. (2016). Designing advanced biochar products for maximizing greenhouse gas mitigation potential. Crit. Rev. Environ. Sci. Technol. 46, 1367–1401. doi:10.1080/10643389.2016.1239975
Omondi, M. O., Xia, X., Nahayo, A., Liu, X., Korai, P. K., and Pan, G. (2016). Quantification of biochar effects on soil hydrological properties using meta-analysis of literature data. Geoderma 274, 28–34. doi:10.1016/j.geoderma.2016.03.029
Peng, Y., Wang, G., Li, F., Yang, G., Fang, K., Liu, L., et al. (2019). Unimodal response of soil methane consumption to increasing nitrogen additions. Environ. Sci. Technol. 53, 4150–4160. doi:10.1021/acs.est.8b04561
Phoenix, G. K., Emmett, B. A., Britton, A. J., Caporn, S. J. M., Dise, N. B., Helliwell, R., et al. (2012). Impacts of atmospheric nitrogen deposition: Responses of multiple plant and soil parameters across contrasting ecosystems in long-term field experiments. Glob. Change Biol. 18, 1197–1215. doi:10.1111/j.1365-2486.2011.02590.x
Qin, X., Wang, H., Liu, C., Li, J., Wan, Y., Gao, Q., et al. (2016). Long-term effect of biochar application on yield-scaled greenhouse gas emissions in a rice paddy cropping system: A four-year case study in SouthSouth China. Sci. Total Environ. 569, 1390–1401. doi:10.1016/j.scitotenv.2016.06.222
Robertson, P. A. P., and Robertson, G. P. (2006). The effect of increased N deposition on nitrous oxide, methane and carbon dioxide fluxes from unmanaged forest and grassland communities in Michigan. Biogeochemistry 79, 315–337. doi:10.1007/s10533-005-5313-x
Shakoor, A., Shahzad, S. M., Farooq, T. H., and Ashraf, F. (2020). Future of ammonium nitrate after Beirut (Lebanon) explosion. Environ. Pollut. 267, 115615. doi:10.1016/j.envpol.2020.115615
Simek, M., Jisova, L., and Hopkins, D. W. (2002). What is the so-called optimum pH for denitrification in soil? Soil Biol. Biochem. 34, 1227–1234. doi:10.1016/s0038-0717(02)00059-7
Singla, A., and Inubushi, K. (2014). Effect of biochar on CH4 and N2O emission from soils vegetated with paddy. Paddy Water Environ. 12, 239–243. doi:10.1007/s10333-013-0357-3
Smith, J. L., Collins, H. P., and Bailey, V. L. (2010). The effect of young biochar on soil respiration. Soil Biol. Biochem. 42, 2345–2347. doi:10.1016/j.soilbio.2010.09.013
Song, X., Pan, G., Zhang, C., Zhang, L., and Wang, H. (2016). Effects of biochar application on fluxes of three biogenic greenhouse gases: A meta-analysis. Ecosyst. Health Sustain. 2, e01202. doi:10.1002/ehs2.1202
Spokas, K. (2013). Impact of biochar field aging on laboratory greenhouse gas production potentials. GCB Bioenergy 5, 165–176. doi:10.1111/gcbb.12005
Steiner, C., Glaser, B., Geraldes Teixeira, W., Lehmann, J., Blum, W. E., and Zech, W. (2008). Nitrogen retention and plant uptake on a highly weathered central Amazonian Ferralsol amended with compost and charcoal. J. plant Nutr. soil Sci. 171, 893–899. doi:10.1002/jpln.200625199
Troy, S. M., Lawlor, P. G., O' Flynn, C. J., and Healy, M. G. (2013). Impact of biochar addition to soil on greenhouse gas emissions following pig manure application. Soil Biol. Biochem. 60, 173–181. doi:10.1016/j.soilbio.2013.01.019
Vargas, R., Collins, S. L., Thomey, M. L., Johnson, J. E., Friggens, M. T., Natvig, D. O., et al. (2012). Precipitation variability and fire influence the temporal dynamics of soil CO2 efflux in an arid grassland. Glob. Change Biol. 18, 1401–1411. doi:10.1111/j.1365-2486.2011.02628.x
Wang, J., Pan, X., Liu, Y., Zhang, X., and Xiong, Z. (2012). Effects of biochar amendment in two soils on greenhouse gas emissions and crop production. Plant soil 360, 287–298. doi:10.1007/s11104-012-1250-3
Woolf, D., Amonette, J. E., Street-Perrott, F. A., Lehmann, J., and Joseph, S. (2010). Sustainable biochar to mitigate global climate change. Nat. Commun. 1, 56–59. doi:10.1038/ncomms1053
Xie, Z., Xu, Y., Liu, G., Liu, Q., Zhu, J., Tu, C., et al. (2013). Impact of biochar application on nitrogen nutrition of rice, greenhouse-gas emissions and soil organic carbon dynamics in two paddy soils of China. Plant Soil 370, 527–540. doi:10.1007/s11104-013-1636-x
Yan, W., Zhong, Y., Shangguan, Z., and Torn, M. S. (2022). Response of soil greenhouse gas fluxes to warming: A global meta-analysis of field studies. Geoderma 419, 115865. doi:10.1016/j.geoderma.2022.115865
Yang, J., Jia, X., Ma, H., Chen, X., Liu, J., Shangguan, Z., et al. (2022). Effects of warming and precipitation changes on soil ghg fluxes: A meta-analysis. Sci. Total Environ. 827, 154351. doi:10.1016/j.scitotenv.2022.154351
Yue, K., Fornara, D. A., Yang, W., Peng, Y., Peng, C., Liu, Z., et al. (2017). Influence of multiple global change drivers on terrestrial carbon storage: Additive effects are common. Ecol. Lett. 20, 663–672. doi:10.1111/ele.12767
Zhang, A., Liu, Y., Pan, G., Hussain, Q., Li, L., Zheng, J., et al. (2012). Effect of biochar amendment on maize yield and greenhouse gas emissions from a soil organic carbon poor calcareous loamy soil from Central China Plain. Plant Soil 351, 263–275. doi:10.1007/s11104-011-0957-x
Zhang, C., Zeng, G., Huang, D., Lai, C., Chen, M., Cheng, M., et al. (2019). Biochar for environmental management: Mitigating greenhouse gas emissions, contaminant treatment, and potential negative impacts. Chem. Eng. J. 373, 902–922. doi:10.1016/j.cej.2019.05.139
Zhang, Q., Duan, P., Gunina, A., Zhang, X., Yan, X., Kuzyakov, Y., et al. (2021). Mitigation of carbon dioxide by accelerated sequestration from long-term biochar amended paddy soil. Soil Tillage Res. 209, 104955. doi:10.1016/j.still.2021.104955
Zhang, W., Mo, J., Zhou, G., Gundersen, P., Fang, Y., Lu, X., et al. (2008). Methane uptake responses to nitrogen deposition in three tropical forests in southern China. J. Geophys. Research-Atmospheres 113, D11116. doi:10.1029/2007jd009195
Zhong, Y., Yan, W., and Shangguan, Z. (2016). The effects of nitrogen enrichment on soil CO2 fluxes depending on temperature and soil properties. Glob. Ecol. Biogeogr. 25, 475–488. doi:10.1111/geb.12430
Zhou, L., Zhou, X., Shao, J., Nie, Y., He, Y., Jiang, L., et al. (2016). Interactive effects of global change factors on soil respiration and its components: A meta-analysis. Glob. Chang. Biol. 22, 3157–3169. doi:10.1111/gcb.13253
Zhou, L., Zhou, X., Zhang, B., Meng, L., Bo, L., Liu, L., et al. (2013). Different responses of soil respiration and its components to nitrogen addition among biomes: A meta-analysis. Glob. Change Biol. 20, 2332–2343. doi:10.1111/gcb.12490
Keywords: biochar, nitrogen, interactive effect, greenhouse gas emissions, C and N cycle, crop yield
Citation: Jia X, Yan W, Ma H and Shangguan Z (2023) Antagonistic and synergistic interactions dominate GHGs fluxes, soil properties and yield responses to biochar and N addition. Front. Environ. Sci. 11:1123897. doi: 10.3389/fenvs.2023.1123897
Received: 14 December 2022; Accepted: 07 March 2023;
Published: 17 March 2023.
Edited by:
Jian Liu, Norwegian Institute of Bioeconomy Research (NIBIO), NorwayReviewed by:
Mengyang You, Northeast Institute of Geography and Agroecology (CAS), ChinaYangquanwei Zhong, Northwestern Polytechnical University, China
Copyright © 2023 Jia, Yan, Ma and Shangguan. This is an open-access article distributed under the terms of the Creative Commons Attribution License (CC BY). The use, distribution or reproduction in other forums is permitted, provided the original author(s) and the copyright owner(s) are credited and that the original publication in this journal is cited, in accordance with accepted academic practice. No use, distribution or reproduction is permitted which does not comply with these terms.
*Correspondence: Weiming Yan, eWFud2VpbWluZzAxMTBAMTI2LmNvbQ==; Zhouping Shangguan, c2hhbmdndWFuQG1zLmlzd2MuYWMuY24=