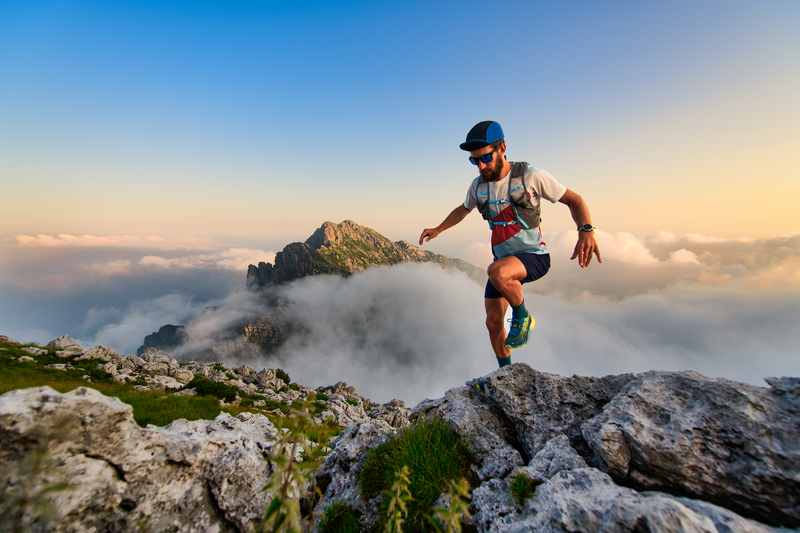
95% of researchers rate our articles as excellent or good
Learn more about the work of our research integrity team to safeguard the quality of each article we publish.
Find out more
REVIEW article
Front. Environ. Sci. , 19 January 2023
Sec. Toxicology, Pollution and the Environment
Volume 11 - 2023 | https://doi.org/10.3389/fenvs.2023.1101100
Rapid industrial development and extensive use of chemicals have resulted in elevated concentrations of emerging contaminants worldwide, posing a substantial threat to the ecological environment and human health. Per- and polyfluoroalkyl substances (PFASs) have been recognized as emerging pollutants that are widely distributed and accumulated in the environment and they have drawn the attention of scholars for several decades. The variety, long-term use, and long-distance transmission of PFASs have resulted in the ubiquitous contamination of global ecosystems, especially in aquatic environments. Ever since perfluorooctane sulfonate (PFOS) and perfluorooctanoic acid (PFOA) were added to the Stockholm Convention on Persistent Organic Pollutants (POPs), they have become the most typical, eye-catching, and frequently investigated PFASs. Owing to the high stability and bioaccumulation of PFASs, as well as the adverse impact on the endocrine, immune, and nervous systems, investigating their contamination levels, risk of transfer along the food chain, and ecotoxicity should be prioritized. In addition to the important evolutionary significance as primitive vertebrates and the main consumers of aquatic environment, fishes generally exist in various aquatic food chains from the bottom to the top and occupy a critical position in terms of aquatic ecology protection; while amphibians, as the key link from aquatic to terrestrial organisms, are highly sensitive to different environmental pollutants. This review is a comprehensive summary of the toxic effects and toxicity-related factors of PFASs on aquatic vertebrates, mainly Pisces and Amphilla organisms, the characteristics of different aquatic vertebrates in toxicity investigations, and the evaluation of the feasibility of PFASs substitute applications.
Per- and polyfluoroalkyl substances (PFASs), first produced by 3M, have been manufactured for more than 60 years as organic compounds of anthropogenic origin (Domingo and Nadal, 2019). Over 4000 PFAS have been used to manufacture paints, pesticide extenders, furniture, cosmetics, paper products, adhesives, waxes, firefighting foams, metal coatings, coal combustion, electronics, lubricants, non-stick cookware, polishes, fabrics, and carpets, as well as in caulks and grease-proof coatings for food packaging, such as microwaveable hamburger wrappers and popcorn bags (Birkholz et al., 2014; Jasrotia et al., 2021). Due to their global presence in various environmental matrices, biotas, and human populations, persistence, bioaccumulation potential, and possible adverse effects on living organisms, PFASs have drawn increasing attention as emerging pollutants in recent years (Giesy and Kannan, 2001; Silva et al., 2020; Zhao et al., 2022). According to numerous worldwide studies, in both rural and urban sites, a significant concentration of PFASs has been identified, even at great distances from the main possible sources (Teymourian et al., 2021).
Owing to the high demand for production and widespread use, perfluorooctanoic acid (PFOA) and perfluorooctane sulfonate (PFOS) have become the most typical and ubiquitous PFASs in the environment. PFASs, among other compounds, are emerging environmental endocrine disruptors raising growing global health concerns (Zakaria et al., 2022). In 2009, PFOS and related compounds were listed in the Stockholm Convention on Persistent Organic Pollutants (POPs) Annex B, an international environmental treaty, signed in 2001, and effective from May 2004, aimed at eliminating or restricting the production and use of POPs. In 2017, PFOA was listed in Annex A for global regulation (Ahrens and Bundschuh, 2014; Zhao et al., 2022). In October 2019, the 15th meeting of the POPs Review Committee, in Italy, recommended the inclusion of perfluorohexyl sulfonate (PFHxS) in the list. Polyfluoroalkyl and perfluoroalkyl substances are commonly divided into three classes: perfluoroalkyl substances (PerFASs), polyfluoroalkyl substances (PolyFASs), and fluorinated polymers, which are further divided into three subclasses: fluoropolymers, perfluoropolyethers, and side-chain fluorinated polymers. These complex substances have unique physicochemical properties that vary depending on the chain length and functional groups (Buck et al., 2011) hence, the adverse impacts of most PFAS compounds on human health or ecology need to be thoroughly evaluated, and fully understood.
On one hand, aquatic ecosystems act as the main sink for PFAs because of their continuing emissions and their persistence, due to the carbon-fluorine bond (Dai et al., 2022), it is imperative to have a more complete and updated knowledge of the adverse effects of PFASs. On the other hand, short-chain analogs, like F-53B [chlorinated polyfluoroalkyl ether sulfonic acids (Cl-PFESAs)], OBS (sodium p-perfluorous non-enoxybenzenesulfonate), GenX [2,3,3,3-tetrafluoro-2-(1,1,2,2,3,3,3-heptafluoropropoxy)-propanoic acid (HFPO-DA)] have been widely used as alternatives and have gradually attracted global attention with the worldwide regulation of PFOS and PFOA (Li et al., 2020), to assess the application security of the common substitutes is also a critical mission of the present review. On account of the critical ecology position of fishes and amphibians in aquatic environment, the toxicity effects of PFASs and their substitutes to them should be systematically summed up aiming at accurately understanding the environmental risks of PFASs and their substitutes so as to provide reliable reference resources for the subsequent application and the development of environmental management policies for the government. Moreover, the comparison between different test organisms is conducive to screening more accurate biological testing methods and objects for clarifying the potential toxicity of aquatic pollutants more quickly, accurately and comprehensively.
Compared to other aquatic organism groups, fish are the top community of the food web and play an important role in the regulation of the existence and abundance of other communities. Fish groups are diverse and can produce various adaptive changes according to the influence of environmental factors; therefore, even if they have been used in experimental research for only a few decades, their advantages are irreplaceable compared with other experimental animals.
The zebrafish (Latin name Brachydanio rerio var, binomial nomenclature Danio rerio), the second most used animal model in medical and life sciences research, is an excellent vertebrate model for estimating the toxicity of chemicals with remarkable advantages (Shen and Zuo, 2020). More importantly, as a workable alternative to mammalian models, zebrafish genes with human similarities as high as 87% have made outstanding contributions to human developmental biology and molecular developmental genetics. Moreover, it is widely used in toxicology tests owing to its high sensitivity and strong repeatability, and it is convenient to use zebrafish for further study of the pathogenic mechanism of pollutants. Besides the most focused PFOS and PFOA, the toxic effects of other PFASs and PFAS substitutes have been extensively investigated.
Due to their environmental persistence, bioaccumulation through the food chain, and potential toxicity in the aquatic environment (Kannan et al., 2005), PFOS have been the focus of the earliest attention from international organizations, the most frequently investigated, and the most commonly detected PFASs.
Lethal effects of PFOS on zebrafish have been listed in Table 1. PFOS can reduce embryo and larval survivorship in zebrafish (D. rerio) (Shi et al., 2008; Du et al., 2009; Hu, 2009; Ye et al., 2009; Kim et al., 2011; Wang et al., 2011; Zheng et al., 2012; Xia et al., 2013; Li et al., 2015; Zhao et al., 2016). The 48-h, 96-h, and 120-h LC50 of PFOS to zebrafish embryos were 31.27–107 mg/L (Ye et al., 2009; Li et al., 2015), 10.89–71 mg/L (Ye et al., 2009; Li et al., 2015) and 2.2–28.21 mg/L (Huang et al., 2010; Hagenaars et al., 2011), respectively; while 72-h, 96-h and 120-h LC50 to larvae were 68 mg/L (Zheng et al., 2012), 31.03 mg/L (Annunziato et al., 2020) and 18.091 mg/L (Tong, 2021). Classified as a medium toxicant, PFOS shows, however, more severe toxicity at different levels than other PFASs (Hu, 2009).
PFOS exposure causes reproductive toxicity including fertilization rate abnormal (Wang et al., 2011), cloacal defects and other reproductive system damage in embryos and larvae (Ye, 2008), inhibition of the gonads growth in the female zebrafish (Du et al., 2009), decreased germ cell density and activity and male gonad development impairment (Wang et al., 2011) (Supplementary Table S1).
PFOS exposure causes hatching delays (Zhao et al., 2016), a decrease in hatching rates (Ye, 2008), as well as larval malformation (Du et al., 2009). Gross developmental malformations of embryos can be induced by PFOS exposure, including spinal column malformation (Li et al., 2015), tail malformation (Zhao et al., 2016), ventral and dorsal fin malformations (Ye et al., 2009), swim bladder malformation, inflation failure (Chen et al., 2014) and damage in the ultrastructure of organelles related to material transport based e.g. deformation of mitochondrial cristae on histological and ultrastructural examination (Cui, 2012). Circulation system defects, edema of the yolk sac and tail (Xia et al., 2013), pericardial edema (Shi et al., 2008; Zheng et al., 2012; Zhao et al., 2016) and organ development-related biological processes (Dong et al., 2021) can be observed due to PFOS exposure.
For adult zebrafish, PFOS exposure retards growth and development (Ye, 2008; Xia et al., 2013; Chen et al., 2014), reduces body length (Chen et al., 2014), body weight (Du et al., 2009), mouth width: body length ratio, and mouth width: head length ratio (Xia et al., 2013), reducing or speeding up the heart rate (Xia et al., 2013; Zhao et al., 2016), and affected the process of myocardial contraction, which has a potentially toxic effect on the zebrafish heart (Yao, 2018). Disruption of organ development is the most significant biological process for PFOS exposure (Dong et al., 2021). PFOS exposure resulted in microstructure damage of the gills (Hu et al., 2009), swim bladder and gut organogenesis and development defects (Chen et al., 2014), arrangement of inner microvilli in intracellular canaliculus irregularity in the liver and intestines (Cui et al., 2016) and irreversible histopathological alterations in the liver, such as cytoplasmic vacuoles and pycnotic nuclei (Ye, 2008). PFOS causes visceral and muscle damage in zebrafish by disturbing the malondialdehyde (MDA) and alkaline phosphatase (ALP) activity (Yuan et al., 2015), causes kidney damage, as reflected by an increase in creatinine in the muscles (Yao, 2018). Adult zebrafish males were more affected by PFOS, presumably because females released some of the PFOS in their bodies through spawning. Growth suppression in weight and length in F1 embryos could also be observed (Du et al., 2009).
PFOS enhanced the production of reactive oxygen species (ROS), downregulated expression of peroxisome proliferator-activated receptor (PPAR) (Sant et al., 2021) and induced the activity of antioxidative enzymes in the visceral tissue, muscle, and liver, including superoxide dismutase (SOD), catalase (CAT), glutathione peroxidase (GPx), malondialdehyde (MDA) content (Shi and Zhou, 2010; Yuan, 2015; Yao, 2018), glutamic pyruvate transaminase (GPT), glutathione reductase (GR), and superoxide anion (Hu, 2009). Embryonic oxidative stress (Zhao et al., 2016) can also be revealed by the deregulation of cyclin-dependent kinase 5 (CDK5) and peroxiredoxin 2 (PRX2) (Zhang et al., 2011), altered expression of representative genes in the CYP450 oxidative stress enzyme system (Hu, 2009) and oxidative stress-related nuclear factor erythroid 2–related factor 2 (Nrf2) and heme oxygenase-1 (HO-1) in zebrafish larvae (Shi and Zhou, 2010). The upregulated expression of liver X receptor alpha (nr1h3), retinoic acid receptor alpha (rara), retinoid X receptor gamma b (rxrgb), and pregnane X receptor (nr1l2) in the liver of males (Cheng et al., 2016) reflects liver oxygenated stress damage (Guo et al., 2019). Lipid alterations in cell membrane properties (Hu et al., 2003), severe apoptosis in embryo cells (Zhao et al., 2016), and p38 gene expression in zebrafish larvae can be induced (Shi and Zhou, 2010). PFOS has a significant effect on cell composition and molecular function pathways, which are closely related to muscle composition and physiological function (Yao, 2018). PFOS exposure mis-expresses 1278 transcripts (Chen et al., 2014) and induces changes in miRNA expression profiles, indicating developmental toxicity, cytotoxicity, metabolic toxicity, and oncogenicity in zebrafish embryos (Zhang et al., 2010).
PFOS exposure elevates the point mutation rate in the p53 gene in zebrafish embryos (Chen et al., 2009), alternated 211 genes by at least two-fold resulted in swim bladder and gut organogenesis and development defects (Chen et al., 2014), upregulated cell apoptosis-related c-Jun NH (2)-terminal kinase (JNK) (Shi and Zhou, 2010) and induced the expression of taurocholic acid (Chen, 2021).
For adults, PFOS significantly affects lipid metabolism (Yao, 2018), especially observed from liver fat accumulation in males (Cui, 2012). PFOS reduces low/very low-density lipoprotein (LDL/VLDL) in the serum of males (Cheng et al., 2016), primarily with lipid droplet accumulation in the male liver (Du et al., 2009), fatty liver degeneration (hepatic steatosis) in adult males (Cheng et al., 2016; Cui et al., 2016), alterations in the expression of fabp10a genes associated with fatty acid metabolism in the liver (Khazaee et al., 2020). Metabolic damage including alternate expression of genes associated with fatty acid metabolism, such as peroxisomal acyl-CoA oxidase 1 (acox1), acyl-CoA dehydrogenase (acadm), carnitine palmitoyltransferase-1A (cpt1a) (Cheng et al., 2016), fatty acid metabolism-associated genes fabp1a in the liver and intestine, and fabp10a in the liver, intestine, heart, and ovaries (Khazaee et al., 2020), alteration expression of ALP, lactate dehydrogenase (LDH) in the head and ALP in the muscle and viscera of zebrafish are caused by PFOS exposure (Yuan, 2015). It also triggers the metabolic pathways of taurine and hypotaurine, glycolysis or glucose production, amino acid, pyruvate, and purine metabolisms in the liver (Yao et al., 2018) that result in altered metabolites in the liver (Yao, 2018). Taurine, glucose, and oleic acid can be used as potential markers of PFOS-induced liver metabolism abnormalities (Yao et al., 2018). Substance transport or metabolism-related mitochondria and endoplasmic reticulum variation, downregulation of solute carrier organic anion transporting polypeptide 2b1 (slco2b1) (Jantzen et al., 2016a); persisting effects in larvae and juvenile fish, including induction of adiposity and saturated fatty acid concentration increase (Sant et al., 2021) and interference with the AMPK signaling pathway related to energy metabolism (Yao, 2018) could also be the effects of PFOS exposure. PFOS reduces liver ATP content (Cheng et al., 2016), induces deoxycholic acid, coenzyme Q increase, primary bile acid synthesis, bile secretion, and retrograde endocannabinoid signaling (Chen, 2021).
Glucose and lipid metabolism disorder could be observed in both females and males of the F1 generation (Cui, 2012), including uneven lipid droplets in cells, and decreased liver glycogen, suggesting abnormal lipid transport. Expression of genes related to lipid and glucose metabolism, such as dgatlb, hb9, kissl, and leptin in both F0 and F1 offspring, and xdh in F1 offspring indicated that PFOS disturbed lipid and glucose metabolism (Cui, 2012; Cui et al., 2016).
PFOS exposure affects the accumulated distance of swimming and mobility state frequency (MSF) of zebrafish larvae, promotes acceleration and activity under light-dark transition (Tong, 2021) and tapping stimulus at F0 adult swimming speed (Wang et al., 2011; Chen et al., 2012). PFOS causes damage to the central nervous system by affecting the activity of acetylcholinesterase (AChE) in the head (Yuan, 2015), expression of LDH (Yuan et al., 2015), toxicity to motor neurons by alternating the expression of alpha-tubulin and proliferating cell nuclear antigen (PCNA) (Zhang et al., 2011) and on locomotor performance (Xia et al., 2013), and hyperactivity in zebrafish larvae (D. rerio) at non-teratogenic concentrations (Zheng et al., 2012; Annunziato et al., 2020; Gaballah et al., 2020). The expression alteration of neural functions associated genes, ChAT, AChE, and hdac6 (Khazaee et al., 2020; Dong et al., 2021), reduced aggressive behavior and upregulation of brain-derived neurotrophic factor (bdnf) are also detected (Jantzen et al., 2016a). PFOS in F0 eggs could induce severe body burden and hyperactivity of the basal swimming speed of offspring (Wang et al., 2011). Chronic PFOS exposure different swimming speeds in light and dark periods in F1 larvae (Wang et al., 2011; Chen et al., 2012).
PFOS shows persisting effects in larvae and juvenile fish, including the induction of aberrant islet morphologies and principal islet areas (Sant et al., 2021). The disruption mechanisms of PFOS to thyroid status are reflected by the significant promotion of triiodothyronine (T3) levels, altered gene expression in the hypothalamic-pituitary-thyroid (HPT) axis, especially downregulated expression of the critical genes in the synthesis, regulation, and action of thyroid hormones including thyroid-stimulating hormone (TSH), thyroglobulin (TG), transthyretin (TTR), and thyroid receptor (TRβ) (Shi et al., 2009) except for a decrease in thyroxine (T4) levels, downregulation of thyroid function-related thyroid hormone receptor-alpha (THRα), thyroid hormone receptor-beta (THRβ), hematopoietically expressed homeobox (hhex), and paired box gene 8 (pax8) and were also observed (Kim et al., 2011). The endocrine-disrupting effect of PFOS caused increased vitellogenin (VTG) levels in the blood plasma, whole body homogenate, head, and tail homogenate, and in male livers (VTG1 and VTG3) of zebrafish (Cheng et al., 2012a; b; Du et al., 2009). Induced expression of regucalcin in the liver and the estrogen-like effects revealed by the cytotoxicity of MCF-7, HeLa, and HepG2 cells in adult males also deserve attention (Hu, 2009).
The 48-h, 96-h, and 120-h LC50 of PFOA to zebrafish embryos were 1005 mg/L (Ye et al., 2009), 371–661.695 mg/L (Ding et al., 2013; Pan et al., 2020) and 200–224.61 mg/L (Ye et al., 2009; Wang, 2021), respectively, while the 120-h LC50 for larvae was 40.052 mg/L (Tong, 2021) (Table 1). For sub-lethal effects, PFOS had the most harmful and evident adverse effects, whereas PFOA affected zebrafish in the most lasting way (Jantzen et al., 2016b) although without severe reproductive toxic effects. PFOA exposure induces teratological effects in embryos, including crooked spine malformation, abnormal ventroflexion of the tail, ventral and dorsal fins (Ye, 2008). PFOA can cause growth suppression in juvenile, branchial epithelium of gills, which was not observed in PFOS-exposed fish (Ye, 2008) and liver abnormalities, affecting liver development in embryos and adults (Wang, 2021). PFOA causes cell apoptosis in the eye, head, heart, and tail regions (Pan et al., 2020), induces DNA damage (Cui et al., 2012) and causes inflammatory response in zebrafish embryos and adults e.g. altering expression of some immune-related amino acids (Wang, 2021). PFOA causes higher expression of esr1, hhex, and pax, altering the expression of major steroidogenic genes and the key steroidogenic gene regulator steroidogenic factor 1 (SF-1) in zebrafish embryos (Du et al., 2013), interferes with the amino acid metabolism in larvae, affects the metabolic functions of the adults (Wang, 2021) and causes energy metabolism disorder (Pan et al., 2020). PFOA can cause nervous system damage, including neural tube defects (Ye, 2008), spontaneous movement alteration, behavioral pattern changes under light stimulation in larvae (e.g. treated females prefer the dark section of the tank) (Cui et al., 2012; Jantzen et al., 2016a) and other neurotoxicity in different tissues (Pan et al., 2020). PFOA interferes with the hormone receptors ER and TR, as well as the production of estradiol (E2) and testosterone (T) in H295R cells (Du et al., 2013).
Perfluorododecanoic acid (PFDoA), an artificial C10 perfluorochemical, is widely distributed in different environments. Long-chain PFDoA inhibited the growth and development of larval zebrafish by reducing the hatching rate, heart rate, and body length, increasing the deformity and death rates, and causing spinal curvature, pericardial edema, and other teratogenic phenomena (Zhang, 2018) (Supplementary Table S2). Histopathological liver damage including swollen hepatocytes, vacuolar degeneration, nuclear pyknosis, and oxidative stress responses are involved in PFDoA-induced hepatotoxicity in female zebrafish (Liu et al., 2008). It also affected fatty acid oxidation by altering the expression of medium-chain fatty acid dehydrogenase (MCAD) and liver fatty acid binding protein (L-FABP) (Liu et al., 2008). PFDoA has the potential for developmental neurotoxicity and can induce the downregulation of acetylcholine (ACh) content and acetylcholinesterase (AChE) activity, as well as upregulation of dopamine levels in zebrafish embryos and the gene mesencephalic astrocyte-derived neurotrophic factor (manf) in larvae (Guo et al., 2018), which causes thyroid dysfunction by regulating the expression of important genes such as crh, trh, and tshβ along the hypothalamic-pituitary-thyroid (HPT) axis and interferes with hormone production by altering the content of T3 and T4 (Zhang, 2018).
PFOSA was the only structure that was bioactive in embryonic photomotor response (EPR), larval photomotor response (LPR), and altered morphology assays (Truong et al., 2022). Compared with PFOA and other commonly seen PFASs, perfluorooctane sulfonamide (PFOSA) was a more potent developmental toxicant because the pathways related to hepatotoxicity and lipid transport were disrupted in embryos, there was a decrease in the liver area and an increase in yolk sac neutral lipids (0.75–128 h post-fertilization/hpf) (Dasgupta et al., 2020).
Perfluorononanoic acid (PFNA) induces expression of UDP-glucuronosyltransferases in the liver of male zebrafish (Liu et al., 2011), reduction in total distance traveled and time of immobility, an increase in thigmotaxis behavior, aggressive attacks, preference for a bright environment, alteration in the expression of slco2b1, gfb1a, and bdnf (Jantzen et al., 2016a) and significant trans-generational elevates plasma T3 levels, thyroid-disrupting effects as well as histological changes in the thyroid follicles (Liu et al., 2011).
Besides lethal effects, short-chain PFHxA inhibited the growth and development of zebrafish larvae by reducing the hatching rate, heart rate, and body length; increasing deformity rates; causing spinal curvature, pericardial edema, and other teratogenic phenomena; causing thyroid dysfunction by regulating the expression of important genes such as crh, trh, and tshβ along the hypothalamic-pituitary-thyroid (HPT) axis; and interfering with hormone production by altering the content of T3 and T4 (Zhang, 2018).
6:2 saturated fluorotelomer carboxylic acids (FTCAs), affects the degradation of fluorotelomer alcohols (FTOHs), decreases the erythrocyte number, and disrupts erythroid differentiation during zebrafish embryonic development, due to differential transcriptional patterns of gata1 and gata2 (Shi et al., 2017a). In addition, perfluorodecanoic acid (PFDA) is the most potent teratogen (Truong et al., 2022).
Polyfluorinated alkylated phosphate ester surfactants (including FC807) are the precursors of PFOS and PFOA. PFOS, perfluorohexane sulfonate (PFHS), and PFBS lead to increased mortality of juvenile fish, bladder inflation defects, and toxicity in the following order: PFOS, PFHS, and PFBS (Yang 2014). PFHxS, PFHpS, or PFOS shared toxicity phenotype characterized by body axis and swim bladder defects (Gaballah et al., 2020). PFOS, FC807, PFNA, PFHS, and PFBS cause spinal curvature in the early stages of the development of zebrafish (Zheng et al., 2012; Yang 2014). PFOS, FC807, PFNA, and PFOA all induced pericardial edema (Zheng et al., 2012). The derivatives of 1-iodoperfluorooctane (PFOI), widely used as an intermediate in the chemical industry, can cause embryonic developmental delay, and hatching rate reduction (Yang et al., 2020). Octafluoro-1,4-diiodobutane (PFBDI), hexadecafluoro-1,8-diiodooctane (PFODI), and dodecafluoro-1,6-diiodohexane (PFHxDI) induced developmental toxicity in zebrafish embryos, with PFHxDI showing the highest developmental toxicity (Lu et al., 2019). PFOS, PFNA, and PFOA alter the morphology of the total body length and affect the gene expression endpoints of muscle development (tfc3a) and protein transport (ap1s) (Jantzen et al., 2016b). In exposed zebrafish embryos, significant differences were found in the total swimming distance (perfluoroheptanoic acid/PFHpA, PFOA, PFNA, PFHxS, PFOS, 6:2 fluorotelomer sulfonic acids/FTSA, PFAS mixture), burst activity (PFOA, PFNA, PFHxS, PFOS, PFAS mixture), and startle response (PFNA, PFHxS, PFOS, PFAS mixture) (Menger et al., 2020). PFOI upregulated the MALAT-1 gene in the brains of zebrafish larvae (Yang et al., 2020). Exposure to perfluorohexanoic acid (PFHxA), PFHxS, PFHpS, or PFOS results in a unique hyperactivity signature (Gaballah et al., 2020). PFBDI, PFODI or PFHxDI upregulated the expression of estrogen receptor (esr)1 and cytochrome P450 (CYP) 19b (CYP19b) (Lu et al., 2019).
Cl-PFESAs, whose commercial name is F-35B, are alternatives to PFOS, that have been used mainly as a mist suppressant in Chinese electroplating industries for over 30 years. F-53B is a moderately toxic substance, and the sensitivity of larvae to acute lethality is higher than that of embryos (Shi et al., 2017b; Deng, 2018; Lai, 2021). F-53B in F0 gonads could transfer via maternal eggs (Shi et al., 2018a). Its reproductive toxicity has been identified since it decreased gonadosomatic index and egg production/female, changed the histological structure of the gonads, increased serum testosterone levels, and disrupted spermatogenesis (Shi et al., 2018a). F-53B showed developmental toxicity, including hatching delay, survival reduction, impairment of the embryonic development of offspring (Shi et al., 2018a), growth inhibition, malformation (pericardial edemas and yolk sac abnormalities, abnormal spines, bent tails, and uninflated swim bladders), cardiac development disruption (Shi et al., 2017b; Tu et al., 2019) and affected tissue function of adult zebrafish (Deng, 2018) (Supplementary Table S3). The F-53B in adult zebrafish will have adverse effects on the zebrafish antioxidant system (Deng, 2018) in altering the expression of specific genes (superoxide dismutase/SOD, catalase/CAT) or proteins (Nrf2, Akt/p-Akt) related to oxidative stress in zebrafish (Wu et al., 2019a; b). Besides immune-toxicity in zebrafish embryos and larvae, exposure to either F-53B or PFOS increased energy expenditure, the expression of genes involved in metabolic pathways at the transcriptional and translational levels as well as reduced feed intake (Tu et al., 2019). F-53B in juvenile fish increased serum testosterone levels (Shi et al., 2018a) and has high thyroid hormone receptor (TR) agonist activity (increase in T4), altered protein expression, including downregulated levels of thyroglobulin (TG), upregulated thyroid transporter (TTR), and interference with the expression of most genes related to the hypothalamic-pituitary-thyroid axis (HPT axis) (downregulation of CRH, TSHβ, Trβ, Dio1, DiO2, NIS, NKX2.1, and PAX8), which cannot be recovered during the elimination phase (Deng, 2018).
PS-MPS greatly promoted the adsorption of F-53B, reduced the biological enrichment of F-53B in various tissues (liver, gill, intestine, and brain) of zebrafish larvae, weakened the degree of oxidative stress response and inflammatory responses of zebrafish when combined, changed the composition of intestinal microflora in zebrafish at the phylum level, and reduced the diversity of intestinal microflora in zebrafish (Lai, 2021). PFOS and its substitutes (F-53B and OBS) affect rate-limiting lipogenic (fasn), β-oxidation (cpt1a) transcripts, the hypothalamus-pituitary-somatotropic axis, and gh and igf2 (Tu et al., 2019).
The OBS fluorine protein foam extinguishing agent was highly toxic to zebrafish and the 48 h-LC50 concentration was 6.829% (Bi et al., 2017). Both OBS and PFOS exposure can cause acute injury to zebrafish embryos and affect their growth and development, including abnormal autonomous movement, inhibition of hatching rate, increased mortality, accelerated heart rate, and morphological changes, such as yolk sac edema, pericardial edema, tail curvature, and spinal curvature (Zou et al., 2020). OBS induces liver oxidative stress in zebrafish larvae and adult fish by regulating the expression of Nrf2 and SOD proteins (Zou et al., 2020; Zou, 2021); downregulates expression of the Bacl-2 gene; and upregulates expression of Bax, Caspase-3, and Caspase-9 in juvenile zebrafish to varying degrees, which increases the level of apoptosis in juvenile zebrafish cells and inhibits anti-apoptotic factors, thus leading to apoptosis. OBS reduces embryo oxygen consumption rate (Tu et al., 2019). Long-term exposure to OBS and PFOS disturbed the intestinal microbiota balance in adult zebrafish, in which Proteobacteria, Fusobacteria, and Actinobacteria were dominant, and Plesiomonas, Shewanella, and Aeromonas were the dominant genera (Zou, 2021).
The 6:2 fluorotelomer sulfonamide alkylbetaine (6:2 FTAB) is a major component of Forafac®1157, a novel perfluorooctane sulfonate (PFOS) alternative used globally in the aqueous film-forming foams (AFFFs). 6:2 FTAB was less toxic than PFOS to zebrafish embryos, although it decreased the survival percentage, increased the malformation percentage, resulting in rough-edged skin/fins. 6:2 FTAB exposure can cause oxidative stress by altering enzyme activities of catalase and glutathione peroxidase; induce cell apoptosis in the tail region by increasing the transcriptional levels of p53, bax, and apaf1 and activities of caspase-3, -8, and -9; and cause immunotoxicity by altering the transcriptional levels of CCL1, IL-1Β, IL-8, TNFΑ, IFN, and CXCL-C1C, which play important roles in the innate immune system (Shi et al., 2018a).
6:2 FTS (1,1,2,2,-perfluorooctyl sulfonic acid) has lower toxicity than PFOS, causing lesions in the heart, spleen, and intestine in adult; causing embryo developmental toxic effects, including increasing the yolk sac and the length of the pharyngeal pouch, leading to pericardial edema and inhibiting heart beating; induces vascular hyperplasia; inhibits atrial development and reduces blood flow velocity in juveniles; enhances the activity of CAT and GSH-Px, which are involved in calcium signal transduction and myocardial contraction; and affects the MAPK, FoxO, and p53 signaling pathways. On metabolic level, it decreases the level of phosphatidyls, lysophosphatidylls, and carnitines, increased levels of TAG and DAG influencing the phosphatidylinositol signaling system, inositol phosphate metabolism, and glycerolopid metabolism, induced the proliferation of HepG2 and T24 cells, and changed the migration of T24 cells could also be observed (Chen, 2021).
Perfluoropolyether carboxylic acids (PFECAs) are alternatives to PFOA but may not be safer. The EC50 order for zebrafish was PFO5DoDA > PFO4DA > PFOA > PFO3OA. They cause the malformation of uninflated posterior swim bladders, lowered levels of T3 and T4 in the whole body of larvae following disrupted thyroid hormone metabolism, and increased in the transcription of the UDP glucuronosyltransferase 1 family a, b (ugt1ab), a gene related to thyroid hormone metabolism (Wang et al., 2020).
Perfluorobutyric acid (PFBA) is less acutely toxic than PFOA as the second-generation counterpart (Godfrey et al., 2017a). A smaller posterior swim bladder and the expression alteration of genes encoding two swim bladder surfactant proteins, sp-a and sp-c and a thyroid disruption effect, including increased expression of the gene encoding thyroid peroxidase, tpo, and the genes encoding two swim bladder surfactant proteins, sp-a and sp-c could be observed when exposed to PFBA (Godfrey et al., 2017b). Besides toxic effects of perfluorinated butanesulfonic acid (PFBS) on growth, as substitutes for long-chain PFASs, endocrine toxicity of PFBA and PFBS could be observed from the increased content of VTG and inhibited the level of thyroxine T3 in zebrafish (Huang, 2022).
The unique chemical properties of PFOS change the permeability of cell membranes, making it easier for small particle size macromolecular pollutants, Nano-ZnO, to enter the membrane, leading to an increase in the Nano-ZnO residue in cells, and finally inducing the enhancement of embryonic developmental toxicity (Du, 2016). PFOS interacts with Nano-ZnO to enter the cell membrane, affected the generation of mature cells in sperms and ovaries, reduced the rate of egg fertilization, reduced the amount of eggs laid (Du et al., 2014), slowed the maturation of the animal’s sex organs (testicle and ovary) to adult zebrafish (Du et al., 2014), the development of accessory sexual characteristics, the maintenance of sexual function, and then affected the balance of some important enzyme activities and hormone secretion in vivo (Du et al., 2014; Du, 2016). Combined exposure increased the mortality of parental and offspring zebrafish and malformation rates (Du et al., 2014), suppressed embryonic hatchability, heart rate (Du et al., 2015a), development of adult body weight and length, and the content of egg protein in embryos (Du et al., 2014), and induced histological damage to the liver and intestinal organs by affecting the secretion of sex hormones in the body. The combination of pollutant exposure caused more serious damage than a single exposure, including downregulation of the expression of oxidative stress-related genes such as SOD1, Cat, and GPx1a, upregulation of apoptosis-related genes such as p53, Bax, Bcl-2, caspase-3, and caspase-9, and downregulation of the anti-apoptotic gene Bcl-2 in juvenile fish (Du et al., 2015a; Du, 2016). Combination of two pollutants causes more serious DNA damage to peripheral blood cells (Du et al., 2014), interferes with the function of the thyroid axis (an increase of T3 content and inhibition of T4 content), affects the absorption, synthesis, transport of iodine, and the binding of thyroid hormone nuclear receptor, destroys the HPT axis action site, and destroys the balance of thyroid hormone in the body by upregulating the expression of TRβ, Deio1, Deio2, NIS, CRH, corticotropin-releasing factor (CRF), and sodium/iodide symporter (NIS) genes and downregulating the expression of thyroid-stimulating hormone (TSHβ) and TRα genes, thyroglobulin (TG), and TTR genes and proteins, and development resulted in enhanced thyroid toxicity in juvenile fish as combined exposure of PFOS and Nano-ZnO (Du et al., 2015b; Du, 2016). The expression of the Vtg1 gene in zebrafish liver tissue was inhibited, the content of vitelloprotein in offspring embryos was affected, and the content of T in male fish was decreased, but the content of E2 in female fish was inhibited. (Du et al., 2014; Du, 2016).
With increasing molar ratios of PFOS in the mixture with PFOA, the combined toxicity to zebrafish embryos increased, even if complex interactive effects from additive to a synergistic effect, then to antagonistic effect, and finally, turnover to the synergic effect were observed (Ding et al., 2013). At a 1:10 concentration ratio of PFOS and PFOA, the combined effect was an additive; at 1:6 and 1:1, it had a synergistic effect, and at 1:3, it had an antagonistic effect on the lethality of embryos (Chen, 2012). The combined exposure of zebrafish to PFOS and PFOA caused significantly more oxidative damage than the single exposure, and the combined exposure triggered the oxidative stress response in zebrafish earlier (Pan, 2019).
The composition of a legacy 3% AFFF formulation containing over 100 PFAS, including approximately 80% PFOS, causes an apparent reduction in zebrafish larval growth (Annunziato et al., 2020). Hepatocellular vacuolization was induced in F1 and F2 males in both single PFOS and combined situations with BPA (Keiter et al., 2012). PFOS-induced reduction of the immune response potential, as well as VTG level inhibition in both F1 and F2 generations, but combined with BPA, suppressed VTG levels in F1 males (Keiter et al., 2012).
PFOS, PFNA, PFOA and PFBS mixture (1:1:1:1) declined the gonadosomatic index (GSI) of Japanese medaka (Oryzias latipes) (Lee et al., 2017). PFOA and PFOS significantly reduced fecundity, but they had different modes of action in reproductive effects for each sex in O. latipes (Kang et al., 2019). PFTriDA (perfluorotridecanoate acid) stimulated the growth of the gonads of female O. latipes and inhibited egg amount (Yu, 2011). Both PFOS and PFOA exposure caused mortality and histopathological changes in F1 O. latipes, even if not directly exposed, and continuous exposure from the F0 to F1 generations increased the extent of adverse effects (Ji et al., 2008). PFOS exposure induces the earlier upregulation of hatching enzymes HCE and LCE and promotion of enzymatic activity, leading to the precocious hatching of Oryzias melastigma embryos, including shortened hatching time and increased hatching rate, before inducing a decrease in subsequent larval survival (Wu et al., 2012) (Supplementary Table S4). The 96-h LC50 of the acid form of C6HF11O3.H3N in O. latipes was >100 mg/L (Hoke et al., 2016) (Table 2). PFOS inhibited body width, impaired the liver structure (Fang et al., 2013), enlarged the sinus venosus-bulbus arteriosus distance, changed the heart rate (Huang et al., 2011), altered the expression of heart-development-related genes, for example, GATA4, NKX2.5, COX-2, FGF8, and ATPase of O. melastigma embryos (Huang et al., 2011). Increased salinity leads to lower hatching rates, and lower expression of mRNA transcripts of PPARα, PPARβ, cyclooxygenase-2 (COX-2), and SMYD1 genes in O. melastigma (Huang et al., 2013). Inhibition of hatchability, and alteration of the F1 generation sex ratio in O. latipes proved the potential of multi-generational endocrine disruptors of the PFAA (PFOS, PFNA, PFOA, PFBS 1:1:1:1) mixture (Lee et al., 2017). Long-term PFTriDA exposure stimulated the growth of the liver of female O. latipes, and malformation in F1 embryos, caused more oxidative damage in male fish than in females and increased the expression of P53, which leads to apoptosis and embryo malformation (Yu, 2011). PFOS causes O. latipes differentially expressed genes in the liver, high cellular toxicity by suppressing the genes encoding transferrin and alcohol dehydrogenase class VI than PFOA (Oh et al., 2012).
PFOS upregulated inflammatory responses and immune-related genes, and led to functional dysfunction or weakness of the immune system in marine medaka (O. melastigma) larvae (Fang et al., 2013). PFOS exposure also causes differential expression of genes related to mitochondrial dysfunction (downregulation of ATP synthase and upregulation of uncoupling protein 2), metabolism of proteins and fats in O. melastigma (Huang et al., 2012) and differential expression of genes related to neurobehavioral defects (Huang et al., 2012). At the early developmental stage of the marine medaka O. melastigma embryo, estrogenic marker genes were downregulated and expression of ARNT and cyp1a was upregulated by PFOS exposure, but at the late developmental stage, ERs and related marker genes were upregulated. The expression of cyp19a and cyp19b is regulated by PFOS in a stage-specific manner, whereas PPARα and PPARβ are first inhibited and subsequently induced (Fang et al., 2012). PFTriDA has an inhibitory effect on the gonadal axis, including inhibition of StAR, Cyp11a, HSD 17β, and Cyp19 to O. latipes (Yu, 2011). PFOI upregulated the expression levels of estrogenic genes, including estrogenic receptor-α (ER-α), ER-β, VTG1, and VTG2 in the livers of male O. latipes (Wang et al., 2011). For PFOS, PFNA, PFOA and PFBS mixture (1:1:1:1) induced of VTG expression in F2 of Japanese medaka (O. latipes) (Lee et al., 2017). PFOA and PFOS caused different transcriptional regulation of vitellogenin (VTG1 and VTG2) and choriogenin (chgh, chghm, and chgl) in males O. latipes (Kang et al., 2019).
The rare minnow (latine name Gobiocypris rarus Ye et Fu, 1983), a warm-water fish known as rare minnow or raregudgeon, is more adaptable to temperature and other living conditions than zebrafish and other tropical fish. As a unique small fish in China, it is very sensitive to heavy metals, pesticides, and other chemicals as an ideal material for chemical and water sample toxicity testing. The Chinese Research Academy of Environmental Sciences has listed it as a subject organism in the National Environmental Protection Agency’s Guidelines for Qualified Laboratories (1996) and the water and wastewater monitoring and analysis methods (fourth edition). Whole-gene sequencing of G. rarus has been completed, and closed populations in genetic resources can be used as provenances for ecotoxicological tests.
As a potential alternative to PFOS, textile finishing agents have a 96-h LC50 of 14.79 mg/L (Table 2) and LOEC of 1.5 mg/L to G. rarus (Wang et al., 2015). development of oocytes in male testes, and degeneration of female ovaries in a freshwater rare minnow (G. rarus) could also be induced by PFOA (Wei et al., 2007). The gonadosomatic index of female G. rarus fish decreased significantly when exposed to Cl-PFESA (F-53B) after the water recovery experiment, but histopathological results showed that female fish had irreversible damage to gonadal development, and the proportion of immature oocytes increased significantly with increasing exposure concentration. KEGG pathway enrichment analysis revealed that the pathways related to ovarian development (ovarian steroidogenesis, oocyte meiosis, estrogen signaling pathway, and cholesterol metabolism) were affected. Cl-PFESA stimulated body weight and body length of rare minnow (Yang, 2020). PFOA can induce histopathological changes in the liver (Wei et al., 2008a; Li, 2018), while PFOA and PFOS mixture (1:1) increased the expression of gene clusters related to oxidative phosphorylation and diminished the expression of gene clusters involved in oxidative stress in G. rarus (Wei et al., 2009). Single PFOA induced oxidative stress and cell cycle alteration in the liver (Wei et al., 2008b). Exposure to equal ratios of PFOA and PFOS increased the expression of gene clusters related to immune response, fatty acid biosynthesis and blood system function and diminished the expression of gene clusters involved in metabolism of carbohydrates, and fatty acids, and translation in G. rarus (Wei et al., 2009). PFOA differentially expressed proteins related to intracellular fatty acid transport, macromolecule catabolism, maintenance of intracellular Ca2+ homeostasis, and mitochondrial function at the protein level in the liver (Wei et al., 2008b) as alterations in the expression of genes involved in fatty acid biosynthesis and transport, intracellular trafficking of cholesterol, mitochondrial fatty acid β-oxidation, and thyroid hormone biosynthesis in rare minnows (G. rarus) of both sexes (Wei et al., 2008a). F-53B induced transcriptome sequencing results showed that the differentially expressed genes were involved in biological processes such as cholesterol and lipid metabolism of G. rarus (Li, 2018).
PFOA induced synthesis of VTG and expression of the estrogen receptor (ER) in the livers of both mature males and females (Wei et al., 2007). Chronic exposure to Cl-PFESA during juvenile development has long-term effects on the regulation of thyroid and sex hormones in fish, but the interference effect of thyroid hormones and PFOS on fish may have different modes of action, and the interference effect of Cl-PFESA on the regulation of sex hormones is similar to that of PFOS (Yang, 2020). Unlike PFOS, interference of F-53B with thyroid hormones may cause toxic effects similar to hyperthyroidism by inhibiting the expression of thyroid hormone metabolism and transport genes, including increased concentrations of thyroid hormones T3 and T3 in juvenile fish, downregulation of thyroid hormone synthesis-related genes (CRH, THβ, NIS, TG, and TPO), transport (TTR), deiodinase (Dio1, Dio2), and receptors (TRα and TRβ); downregulation of thyroid hormone metabolism-related genes (UGT1A), the disorder of expression of genes related to the HPT axis could not be recovered even after several months. Downregulation of key genes regulating sex hormones (GnRH3 and LH-β) and estrogen rate-limiting enzyme genes (CYP19a), and upregulation of hormone receptor genes (ERα and AR), hypothalamic-pituitary-gonadal axis related genes in female and male fish, and VTG gene expression levels in the liver and gonads of male fish indicate that Cl-PFESA has a potential estrogen effect on rare minnow (Yang, 2020).
PFOA exposure causes obvious poisoning symptoms to Cynoglossus semilaevis, mainly intestinal, liver, gallbladder, visceral mass tissue damage, bone hyperplasia, and cerebral hemorrhage in juvenile fish (Zhang, 2020). PFOS-K showed a worse oxidative damage effect than PFOS on the gill than on the liver tissue of C. semilaevis by inducing activity of CAT, GST, and EROD (Hao, 2019). The toxic characteristics of PFOS and PFOA cause liver cells nuclear malformation, mitochondria and endoplasmic reticulum swelling, mitochondria and endoplasmic reticulum blurring, and severe destruction of the cell inner membrane system (Dang, 2015). PFOA exposure leads to the development of diseases such as tumors, cancers as well as the differential expression of genes related to immune deficiency, and damage to the immune systems (Zhang, 2020). Upregulation expression of hsp70 in the gill, intestine, and muscle tissues, especially in the liver, and alteration of the other two immune-related genes, c-type lectin domain and cytochrome c oxidase (cox), proved the immunotoxicity of PFOS in C. semilaevis (Wang et al., 2020). Organelles of C. semilaevis relevant to metabolism, such as intracellular mitochondria and endoplasmic reticulum, could swell and/or be damaged by PFOS (Dang et al., 2015). PFOA could also damage the nervous system of C. semilaevis (Zhang, 2020). Changes in the expression of hormone receptor-related genes, such as AR, ERα, ERβ, AHR, and ARNT, could be observed under the stress of different concentrations of PFOS and PFOS-K (Hao, 2019). PFOS or PFOA exposure activated the defense system of cell repair and tumor inhibition-related genes such as P53, HSP90, NADH, and TNF, causing oxidative stress in cells and damage to the mitochondria and endoplasmic reticulum by apoptosis, triggering apoptosis or carcinogenesis, which is reflected by an increase in intracellular ROS content, while the REDOX balance could be maintained by activating the SOD defense mechanism (Dang, 2015). The enhanced combined toxicity of PFOS, PFOA, and Cd2+ may be due to the increased degree of oxidative damage, decreased cell activity, increased organelle damage, and decreased OH and MT content, leading to decreased metal detoxification ability and cell death (Gao, 2016).
PFOA increased oxidative stress in the lipid peroxidation (LPO) level in primary cultured tilapia (Oreochromis niloticus) hepatocytes (Liu et al., 2007b; Abdel-Gawad et al., 2016). Exposure to either PFOS or PFOA could cause differences in 7-ethoxy-resorufin-o-deethylase (EROD) activity and liver glycogen content in tilapia (O. niloticus) (Han et al., 2012), induce ROS and apoptosis in primary cultured tilapia (Oreochromis niloticus) hepatocytes by activating caspase-3, -8, and-9 activities (Liu et al., 2007b), and inhibition of hepatocyte viability (Han et al., 2012). PFOA increased DNA damage in Nile tilapia (O. niloticus) (Abdel-Gawad et al., 2016). Exposure to either PFOS or PFOA could also cause an increase in immune cells, including leukocytes, B Cells, granulocytes, macrophages, and erythropoietin levels (Han et al., 2012). PFOA increased protein alteration, especially a significant increase in transaminase, blood urea nitrogen, and creatinine in O. niloticus (Abdel-Gawad et al., 2016). PFHxA inhibited the activity of β-N-acetyl-D-glucosaminidase (NAGase) in the liver of Nile tilapia (Chen et al., 2019). VTG induction in primary cultured hepatocytes of freshwater male tilapia (O. niloticus) after treatment with PFOS, PFOA, or FTOH (4:2, 6:2, and 8:2) and the anti-estrogenic effects of 17β-estradiol (E2) combined with PFCs caused inhibition of VTG accumulation, which might be mediated by the estrogen receptor pathway (Liu et al., 2007a).
In addition to lipid peroxidation caused by oxidative stress in cells, apoptosis rate elevation of lymphocytes in the head kidney of Cyprinidae crucian carp (Carassius auratus) indicated damage caused by PFOA to the immune system (Wang et al., 2015). Effects on hatching and success rate were found in PFOA-exposed embryos but heart rates were affected after exposure to PFOS, PFOA, and PFBS (Shi et al., 2020). PFOS levels observed in the tissues of wildlife populations could disrupt hepatocyte membrane integrity and interfere with the homeostasis of DNA metabolism in common carp (Cyprinus carpio) (Hoff et al., 2003). The hepatic PFOS concentration in carp (C. carpio) from natural surface water affected the serum alanine aminotransferase activity, serum protein content, and serum electrolyte concentrations (Hoff et al., 2005) and the expression of genes in liver tissue on energy metabolism, reproduction, and stress response were also affected (Hagenaars et al., 2008).
Swordtails (Xiphophorus helleri Heckel) are ovoviviparous, with small body size, short reproductive cycle, and strong fecundity, and have been used as an object of aquatic toxicology in recent decades (Huang et al., 2000). Histopathological changes in both sexes, the gonadosomatic index (GSI) in female juveniles as well as elevation of the hepatosomatic index (HSI) in males were observed following PFOS exposure (Han and Fang, 2010). Another major route for the toxic effect of PFOS is the induced oxidative damage in the liver of swordtail, which promotes MDA content and alteration activities of superoxide dismutase (SOD), catalase (CAT), and glutathione peroxidase (GSH-PX) (Zhang and Fang, 2013), At the genetic level, it upregulates the expression of sensitive biomarkers, such as GST mRNA, which is related to the Nrf2 signal transduction pathways and HSP70-2 mRNA, which can protect the body from oxidative damage by increasing SOD levels (Zhang et al., 2014a). PFOS can cause VTG mRNA expression in the liver of male swordtail (Han and Fang, 2010).
PFOS or PFOA exposure causes changes in the mRNA expression of peroxisome proliferator-activated receptors (PPARs), acyl-CoA oxidase (ACOX1), oxidative stress responses, and lipid peroxidation (TBARS), and higher severity in the kidney than in Salmo salar liver (Arukwe and Mortensen, 2011). Increases in kidney CYP3A, CYP1A1, and GST expression could be observed in both PFOS and PFOA treatments (Mortensen et al., 2011). PFOS exposure enhanced cellular stress in hepatocytes of Atlantic salmon (S. salar) (Krøvel et al., 2008). Impact on the gonadal hormone testosterone in the blood could be caused by PFOA (Mortensen et al., 2011).
For red sea bream (Pagrosomus major), 96 h-LC50 of PFOS was 22.56 mg/L (Wang et al., 2013). Lesions of gill tissue included edema of gill filament apex, shedding of gill filament epithelium, the fusion of gill filament, severe damage of whole gill filaments, and liver tissue lesions, including cytoplasmic vacuoles, fat drop vacuoles, and tissue vacuoles could be observed by PFOS exposure (Wang et al., 2012). Toxic effects were detected in SOD, CAT, and POD activities and MDA content in the gills (Wang et al., 2013), GSH content, and GST activity in the gill and liver (Wang et al., 2012), whereas at the gene expression level, CYP1A1, AHR2, GSTA1, GSTA2, and GSTR1 genes in the liver showed non-recoverable differences in pure water, indicating that PFOS toxicity on gene expression was more severe than that of antioxidant enzymes in P. major (Wang, 2012).
Fathead minnow (Pimephales promelas) was sensitive to PFOS with a 96 h-LC50 of 4.7 mg/L (Liu et al., 2013). PFOS/L (1 mg) is lethal to sexually mature adult fathead minnow (P. promelas) within two weeks (Ankley et al., 2005). PFOS exposure can reduce growth in F1 fish (Suski et al., 2020), cause a decrease aromatase activity in males (Ankley et al., 2005), while PFOA altered fatty acyl-CoA oxidase (FAO) activity (Oakes et al., 2004). PFOS exposure can reduce the gonadosomatic index in adult males, cause histopathological alterations in the ovaries of adult females (Ankley et al., 2005), and reduce fecundity in females (Suski et al., 2020). PFOS exposure can upregulate plasma testosterone in males (Ankley et al., 2005; Oakes, et al., 2005), 11-ketotestosterone in both sexes, and 17β-estradiol titers in females (Oakes, et al., 2005), and occasionally alter VTG and egg yolk precursor protein (Oakes, et al., 2005). However, except for 17β-estradiol in females, PFOA exposure can alter 11-ketotestosterone in males and testosterone in both sexes (Oakes et al., 2004).
The 96-h LC50 of C6HF11O3.H3N for rainbow trout (Oncorhynchus mykiss) was greater than 96.9 mg/L, and the overall NOEC was 8.89 mg/L (Hoke et al., 2016). In rainbow trout exposed to PFOS (O. mykiss), upregulation of plasma testosterone, 11-ketotestosterone, and 17β-estradiol titers, and occasionally altered VTG, the egg yolk precursor protein, could be observed (Oakes, et al., 2005). Ammonium perfluorooctanoate (APFO, the ammonium salt of PFOA) has a low potential hazard of chronic toxicity in rainbow trout (O. mykiss) (Colombo et al., 2008).
Acute EC50 values for PFOS and PFOA were 0.11 mg/L and 111.9 mg/L for Psetta maxima; 6.9 mg/L and 15.5 mg/L for Siriella armata (Mhadhbi et al., 2012). The peroxides generated from PFOA exposure can cause irreversible damage to vital organs of Murray River rainbowfish (Melanotaenia fluviatilis), such as gills and liver tissues (Miranda et al., 2020). Combined exposure to PFOS, PFOA, and Cu impairs antioxidant defenses and enhances lipid peroxidation in the liver of Carassius auratus (Feng et al., 2015). Short-term PFOS exposure altered the activity of mitochondrial citrate synthase (CS) and cytochrome c oxidase (CCO), and the expression of 20 proteins involved in the general stress response, ubiquitin-proteasome system, energy metabolism, and actin cytoskeleton in the gills of the European bullhead Cottus gobio (Dorts et al., 2011). For the European eel (Anguilla L.) species, an increase in hematocrit level, serum alanine aminotransferase activity, and reduced serum protein content have been observed under PFOS exposure (Hoff et al., 2005; Geeraerts and Belpaire, 2009). Short-term PFOS exposure of juvenile goldfish (Carassius auratus) leads to much higher energy consumption but lower maintenance of swimming capability (Xia et al., 2013). PFOS exposure increased the net energetic cost of transport of Qingbo Spinibarbus sinensis and had an important influence on routine metabolic rate, spontaneous swimming behavior, social interactions, and critical swimming speed; many of these effects also changed concerning temperature (Xia et al., 2015). PFOA altered T3/T4 ratios and the presence of vitellogenin in the plasma of male M. fluviatilis (Miranda et al., 2020).
Certain amphibian species could experience sublethal effects at sites where the surface waters are highly affected by PFAS because the negative impact on their body condition and development is more easily triggered than in fish (Flynn et al., 2022). LC50, EC50, and minimum concentration to inhibit growth (MCIG) of Xenopus laevis for PFOS were 51.46, 108.20, and 35 mg/L, respectively (Wei et al., 2012) (Table 3). As PFOS substitutes, the acute toxicity of textile finishing agents to X. laevis tadpoles is higher than that of PFOS (Su et al., 2012). PFBS and PFHS showed a higher LC50 and lower acute embryo toxicity than PFOS substitutes (Wei et al., 2012). PFOS induces a variety of malformations in the gonads, for example, damage of the tissue structure and ultrastructure, including segmented, tapered and elongated, atrophic and asymmetrical testes, the amalgamation of two or more follicles, and feminization of testis histology (Liu Q. et al., 2008). PFOS can inhibit the abnormal development of Xenopus tadpoles, including embryo malformation, growth inhibition, alteration of body weight, snout to vent length, hind limb length, postponement of the development stage, and sex ratio change (Liu et al., 2008; Liu, 2009; San-Segundo et al., 2016) (Supplementary Table S5). PFOS can cause structural damage to thyroid tissue, for example, thyroid follicle cell hyperplasia, depletion of colloids, and vacuolation and decrease the follicle number (Liu et al., 2008; Liu, 2009). C4 and C6 finishing agents (C4 and C6) synthesized by the electrolysis fluorination method as substitutes for PFOS showed so much lower toxicity than PFOS, while embryotoxicity of textile finishing agents and 50% cationic surfactants were higher than PFOS to X. laevis (Ren et al., 2012). X. laevis exposure to PFOS also causes alterations in oxidative stress, apoptosis, and differentiation related to hsp70, hsp47, ppard, tp53, and Bax genes (San-Segundo et al., 2016). Both thyroid and sex hormone disruptors can identify PFOS as environmental endocrine disruptors (Liu, 2009). The toxicity of PFOS exposure was still higher than that of PFOA in amphibians (Flynn et al., 2020; Tornabene et al., 2021), especially in disrupting the thyroid system of X. laevis tadpoles regarding forelimb emergence changes and regulation alternation of thyroid hormone-regulated genes (Cheng et al., 2011). PFBS caused potential degeneration of hepato-histology and sexual development by upregulating the expression of ER and androgen receptor (AR), sexual dimorphism, and secondary sex characteristics in X. laevis (Lou et al., 2013).
PFOS exposure interferes with growth, such as body weight and snout-vent length of Xenopus tropicalis (Silurana tropicalis) before metamorphic completion, and increases the proportion of phenotypic males and abnormal overlay development characterized by size asymmetry, necrosis, and formation of excessive fibrous connective tissue during juvenile development (Fort et al., 2018).
The acute toxicity of textile finishing agents to Rana nigromaculata tadpoles was higher than that of PFOS (Su et al., 2012). A PFOS concentration of 10 mg/L imperils the survival of the northern leopard frog (Rana pipiens) (Ankley et al., 2004). The 96-h acute toxicity of PFOS was 10-fold higher than that of PFOA to American bullfrogs (Rana catesbeiana) (Flynn et al., 2019). PFOA can cause an imbalance in ion homeostasis in the testis by decreasing sperm number and motility, increasing the sperm malformation rate, changing the microstructure of sperm, including vacuoles of spermatogenic Sertoli cells, cell dispersion and incomplete cell structure, changes in organelles in spermatogenic Sertoli cells, vacuoles, and tail deformity in sperm microstructure (Wang, 2017). In addition to sperm malformation of Rana nigromaculata at very low environmental concentrations (0.001 mg/L), the reproductive toxicity of PFOA in male amphibians could be reflected by interfering with sex hormone synthesis, for example, a decrease in testosterone and increase in estradiol in serum by upregulating expression of P450 aromatase and SF-1 gene (Lu and Chen, 2012). PFOS and PFOA slowed the development of northern leopard frog (Rana pipiens) larvae, such as metamorphosis delay and growth reduction (Ankley et al., 2004). PFOS drives the addictive combined toxicity on tadpole mass, developmental stage, and snout-vent length of R. catesbeiana (Flynn et al., 2019). PFOA exposure also induces oxidative stress by causing lipid peroxidation damage, destroying the homeostasis of the antioxidant system in the sperm, and causing apoptosis through the mitochondrial pathway/ER apoptosis pathway by upregulating the expression of Chop protein, Bax, and caspase 3, 8, and 9, and downregulation of Bcl-2 protein expression in Rana nigromaculata (Wang, 2017).
The eight-carbon backbone of PFOS and PFOA are two of the most widely used PFASs, and their ecotoxicity in the aquatic environment has been the focus of many studies (Flynn et al., 2022). In the general categorization of toxicity data, PFOS is moderately-acutely toxic and slightly chronically toxic to aquatic organisms (Hu, 2009), whereas PFOA is both acutely and chronically practically non-toxic (Van Rijn et al., 1995; Hekster et al., 2003; Shi et al., 2017b). For other fish types, PFOS also causes high cellular toxicity than PFOA (Oh et al., 2012) even if their reproductive toxicity modes vary with each other (Kang et al., 2019) or in different sexes (e.g., P. promelas) (Oakes et al., 2004; Ankley et al., 2005; Oakes, et al., 2005). PFOA causes a more obvious increase in LPO in tilapia (Liu et al., 2007b), and affects the gonadal hormone testosterone in the blood of S. salar than does PFOS (Mortensen et al., 2011). The toxicity of PFOS exposure was still higher than that of PFOA in amphibians (Flynn et al., 2020; Tornabene et al., 2021), even with 10-fold higher 96-h acute toxicity to American bullfrogs (Rana catesbeiana) (Flynn et al., 2019).
For all PFAS substances, the fate and toxicity of pollutants are influenced by their molecular properties and behavior (e.g., migration and accumulation) in the ecological environment; however, the distribution of PFASs can be better predicted by functionality compared with carbon chain length (Shi et al., 2018). PFASs can accumulate in organisms by binding to proteins or fatty acids, and their binding affinities generally increase with chain length (Wen et al., 2017; Shi et al., 2018; 2020). The median tissue/blood ratio, relative body burden, and bioaccumulation factor patterns were most consistent with protein-binding mechanisms, although partitioning to phospholipids may contribute to the accumulation of long-chain PFASs in specific tissues (Shi et al., 2018). PFAS accumulation is tissue-specific and is more commonly detected in protein-rich tissues, such as the blood, liver, muscle, and gonads (Dai et al., 2022). PFOS accumulated easily in F0 eggs of zebrafish (Wang et al., 2011), more in tissue of O. melastigma than PFOA (Oh et al., 2012) and more in increased salinity embryos (Huang et al., 2013). PFTriDA accumulation in male fish was higher, which might be due to the high mother-child transfer coefficient in the order of gonads, eggs, liver, and body, and the higher PFTriDA exposure concentration resulted in a downward accumulation trend (Yu, 2011). The accumulation level in both F1 and F2 generations of Japanese medaka (O. latipes) following the order PFOS > PFNA > PFOA > PFBS for equally mixed mixture exposure. Dietary exposure in juvenile S. salar caused a higher detection of PFOS than PFOA in the blood and recoverable accumulation in the liver and kidney (Arukwe and Mortensen, 2011). Both PFOS and PFHxS accumulate in the gonads and liver of adult fathead minnow (P. promelas) (Suski et al., 2020), while PFOS accumulation in females was higher than that in males, following the order of blood, liver, and gonads (Ankley et al., 2005). Toxicity based on mortality in a concentration-dependent manner and malformations followed the order PFOS > FC807 > PFNA > PFOA > PFBS > PFBA (Hagenaars et al., 2011; Zheng et al., 2012). PFAS with longer chain lengths (e.g., C8) tend to be more toxic than those with shorter chain lengths (e.g., C4) (Hagenaars et al., 2011; Wen et al., 2017; Menger et al., 2020). The presence of high-energy carbon-fluorine bonds in perfluoro compounds lends their environmental persistence with a distinct relationship between hydrophobicity and toxicity. Owing to the increased hydrophobicity resulting in a higher bioaccumulation potential for chemicals with longer carbon chains and the differences in functional groups, PFCs with a sulfonate group have a larger toxic potential than those with a carboxyl group (Ulhaq et al., 2013a; b; Shi et al., 2020). Among sulfonic acid aliphatic PFAS, chemical potencies were correlated with increasing carbon chain length for developmental neurotoxicity, but not developmental toxicity, which identified relationships between chemical structures and in vivo phenotypes that may arise from the shared mechanisms of PFAS toxicity (Gaballah et al., 2020). Low PFAS volatility is associated with developmental toxicity (Truong et al., 2022). BCFs are also related to perfluorocarbon chain length and functional groups (Menger et al., 2020). PFAS with averages BCF of approximately 4000 (PFOS), 200 (PFHxS), 50 (PFOA), and 0.8 (PFBA) L/kg dry weight, suggesting that both the alkyl chain length and the functional group influence the toxicokinetics e.g. lethal, teratogenic effects and locomotor behavior (i.e. for Trifluoroacetic acid/TFAA, PFBA, PFOA, PFNA, PFDA, PFBS, and PFOS) in D. rerio embryo (Vogs et al., 2019) and the toxicity of sulfonic acid compounds was significantly higher than that of carboxyl compounds (Yang 2014). For example, PFASs may penetrate the phospholipid bilayer membrane and enhance permeability, which will help the penetration of other pollutants into the small intestine epithelium and enter the circulatory system of zebrafish (Dai et al., 2022). Although long-chain PFCs are highly bio-accumulative in aquatic environments (Sun et al., 2016), research on the environmental accumulation and adverse effects of alternative PFCs has received more attention.
Although the toxicity degree and main effects of each PFAS compound are differentiated to some extent, for advanced consumers of aquatic ecosystems, the general mechanism of toxicity of PFASs after entering organisms could be similar, especially for fish (Figure 1). Firstly, they induced a series of stress reactions in biological cells including the response of various antioxidant enzymes, thus producing excess ROS (Gao, 2015), the response of lipid peroxidation, then, causing further destruction to membranes, damaged organs morphology and internal cell structure (Dong et al., 2021), regulated alterations of gene expression involved in various life activities (Chen et al., 2014), interferes with various vital metabolism functions and continued to exert toxic effects on the offspring as they accumulate in the parents (Chen et al., 2012; Cui et al., 2016). Variations in water salinity and pH conditions also have a great impact on the toxic effects of PFAS pollutants; therefore, it is necessary to conduct a toxicity risk assessment according to actual water conditions (Huang et al., 2013; Vogs et al., 2019). A point that should not be overlooked is, PFOS and PFOA slowed the development of northern leopard frog (R. pipiens) larvae which alarmed laboratory studies that may have underestimated the effects of aquatic environmental PFAS exposure (Flynn et al., 2020).
The toxic effects of the target PFASs show dependence on several factors. For PFOS, it causes dose-dependent male gonad development impairment (Wang et al., 2011), alteration of the sex ratio (with female dominance) (Wang et al., 2011), and response of oxidative stress (Ye et al., 2009), especially promoted ROS content (Shi and Zhou, 2010) on zebrafish, inhibition of body width in O. melastigma (Fang et al., 2013), hepatocyte apoptosis, expression of stress responsive genes, P450 and phase II enzymes, lipid metabolism and ion regulation related genes of Atlantic salmon (S. salar) (Krøvel et al., 2008) and structural damage of thyroid tissue in X. laevis (Liu et al., 2008; Liu, 2009); it also induces sex-dependent reduction of aggressive behavior, promotion of bdnf and upregulation of transforming growth factor beta 1a (tgfb1a) in male zebrafish (Jantzen et al., 2016a) and time-dependent increases in kidney P450 enzymes and GST as well as in PFOA treatments on juvenile S. salar (Mortensen et al., 2011). PFOA exposure induces a dose-dependent sperm malformation of R. nigromaculata (Lu and Chen, 2012), decrease in the heart rate in zebrafish embryos (Cui et al., 2012), LPO levels in primary cultured tilapia (O. niloticus) hepatocytes (Liu et al., 2007b) and embryonic developmental toxicity when combined with Nano-ZnO (Du, 2016). PFOS, PFOA, and FTOH (6:2) caused dose-dependent VTG induction in primary cultured hepatocytes of male O. niloticus (Liu et al., 2007a). OBS induces liver oxidative stress in zebrafish larvae and adult fish in a dose-dependent manner (Zou et al., 2020; Zou, 2021). F-53B and PFOS increased energy expenditure and reduced feed intake in zebrafish in a concentration-dependent manner (Tu et al., 2019). PFOI upregulated the expression of estrogenic genes in hepatocytes of male medaka in a dose-dependent manner and VTG proteins in a time-dependent manner (Wang et al., 2011). PFECAs dose-dependently increased the transcription of thyroid hormone metabolism-related genes (Wang et al., 2020).
On account of two items as the correlation factors, PFOS caused sex-related (male) and dose-dependent lesion of gill, intestine, liver, and gonad (Hu, 2009; Wang et al., 2011) and neural functions associated gene expression in zebrafish (Khazaee et al., 2020; Dong et al., 2021); dose and time-dependent lesions of gill tissue in P. major (Wang et al., 2012); organ-differentiated and time-dependent changes the mRNA expression profile, similar with PFOA (Arukwe and Mortensen, 2011). PFOA caused zebrafish oxidative stress reaction in a time- and dose-dependent manner (Ye et al., 2009; Yang 2014; Wang, 2021) and dose-dependent and sex-dependent germ cell damage of male amphibian (Lu and Chen, 2012). PFOI upregulated the expression levels of estrogenic genes in the livers of male medaka in a dose-dependent manner, and VTG proteins in a time-dependent manner (Wang et al., 2011). Toxic effects associated with multiple factors include the accumulation of F-53B in male zebrafish blood and in the female ovary, which is concentration-dependent, sex-dependent, and protein-dependent (Shi et al., 2018a; Wu et al., 2019a; b).
Non-linear changes in toxicity and pollutant concentrations could possibly exist. For example, the toxic characteristics of PFOS or PFOA include low-concentration promotion and high-concentration inhibition in the liver cells of C. semilaevis (Dang, 2015).
The sensitivity of an experimental organism is very important for the reliability of experimental results. For zebrafish, the most sensitive indicator of PFOS exposure is spine column malformation (EC50 value of 9.14 mg/L) (Ye et al., 2009); and adult male zebrafish are more sensitive to PFOS in histopathological changes (Hu, 2009), while PFOA the most sensitive indicator of incubation suppression (96-h EC50 of 328.0 mg/L) (Ye et al., 2009; Yang 2014). According to the 96 h LC50 to PFOS, which is 22.56 mg/L for P. major (Wang, 2012), it is more sensitive than C. semilaevis with a 24 h EC50 for liver cell proliferation inhibition of 161.2 mg/L (Gao, 2016). Although the 96-h LC50 of PFOS to zebrafish embryos were 10.89–71 mg/L (Ye et al., 2009; Li et al., 2015), while 96-h LC50 of textile finishing agent to G. rarus larvae was 14.79 mg/L (Wang et al., 2015), it still needs more comparable experments to prove the sensitivity of these two fish types.
When it comes to amphibians, salamanders in the genus Ambystoma were generally more sensitive to PFOS than anurans (frogs and a toad); small-mouthed salamanders and gray tree frogs were more sensitive to PFOA than Jefferson salamanders, American bullfrogs, green frogs, and wood frogs, and were more sensitive to PFHxS than American bullfrogs (Tornabene et al., 2021). Among different amphibians, gray tree frogs were more sensitive at later developmental stages, and small-mouthed salamanders were more sensitive at earlier developmental stages (Tornabene et al., 2021). Species with prolonged larval development (frogs and salamanders) are more sensitive to PFAS than more rapidly developing species (toads) (Flynn et al., 2022). However, amphibians are heterotetraploids with long life cycles, making it difficult to conduct genetic studies, especially genetic mutation experiments.
Between fishes and amphibians, the former group has the advantages of small size, short life cycles, optical transparent embryos, rapid embryonic development, low maintenance cost, high fecundity, controllability to functional genomics techniques (Shen and Zuo, 2020), and easily cultured under experimental conditions. Based on the general conclusion of sensitivity selection and the cost-effective rules, both zebrafish and G. rarus are suitable for toxicity detection of common emerging pollutants, in which G. rarus has more strong points such as living in wider water temperature range.
In terms of the overall research frequency, PFOS and PFOA appear in most (Supplementary Tables S1–S5) of the total toxicity investigations, which not only indicates serious pollution but also shows the significance of screening substitutes for them. F-53B and OBS are the two most frequently applied substitutes for PFOS (Tu et al., 2019) in addition to perfluorinated butyl organic ammonium salt cationic surfactants, textile finishing agents, C4 finishing agents, C6 finishing agents (Wang et al., 2015), PFHS, 6:2 FTAB, PFBSK, etc. (Wei et al., 2012; Liu et al., 2013; Shi et al., 2018b). PFECAs, including PFO3OA, PFO4OA, and PFO5OA, are alternatives to PFOA (Wang et al., 2020). PFBA, PFBS, 6:2 FTS, FC-98 (PFECHS), GenX (HFPO-DA), F-53B, and so on have been used as alternatives to PFOS and PFOA (Li et al., 2020; Chen, 2021).
F-53B is highly accumulated in zebrafish embryos and adults with continuous exposure (Shi et al., 2017b; Deng, 2018) in addition to accumulation in F0 gonads and transfer via maternal eggs (Shi et al., 2018a). F-53B accumulates in a concentration-dependent manner in zebrafish larvae (Wu et al., 2019a; b), protein-dependent, with higher accumulation levels in male zebrafish blood and female ovaries (Shi et al., 2018a), sex-specific and tissue-specific accumulation differences, accumulating more in males (liver > gill > gonad > brain) than in females (gonad > liver > gill > brain) (Deng, 2018). OBS has emerged as a novel substitute for PFOS with lower bioconcentration potential in the Chinese market (Tu et al., 2019), and it accumulates in the protein content of zebrafish in the order of blood > liver > intestine > gill > sperm > brain > muscle (Zou, 2021). F-53B has strong bioaccumulation in rare minnows (G. rarus). C10 Cl-PFESA was more easily enriched in rare minnow than C8 Cl-PFESA was (Li, 2018). In wild crucian carp (C. carassius), the distribution and bioaccumulation of OBS followed the pattern of PFOS, mostly in the blood, gonads, and muscle, but showed lower bioaccumulation than PFOS (Shi et al., 2018). The BCF of the acid form of ammonium 2,3,3,3-tetrafluoro-2-(heptafluoropropoxy)-propanoate (C6HF11O3.H3N) in common carp (C. carpio) over time during the 28-day exposure phase indicates that the substance is unlikely to be bioconcentrated in aquatic organisms (Hoke et al., 2016). C4 and C6 finishing agents (C4 and C6) synthesized by the electrolysis fluorination method could be used as substitutes for PFOS, while the higher embryotoxicity of textile finishing agents and 50% cationic surfactants than PFOS to X. laevis makes it questionable for further application (Ren et al., 2012).
The order of accumulation was F-53B > PFOS > OBS (Li, 2018); and the order of toxicity was GenX > F-53B > FC-98 (Li et al., 2020), PFOS > PFBSK (Liu et al., 2013), PFOS >6:2 FTS (Chen, 2021), PFOS >6:2 FTAB (Shi et al., 2018b), PFO5DoDA > PFO4DA > PFOA > PFO3OA (Wang et al., 2020), PFOS > PFOA > PFBS > PFBA (Hagenaars et al., 2011; Zheng et al., 2012), PFOS > PFHS > PFBS (Yang 2014), and textile finishing agent > perfluorinated butyl organic ammonium salt surfactant > PFOS > C4 finishing agent > C6 finishing agent (Wang et al., 2015). Most of the toxicity experiments have been utilized for no more than four test organisms so there might be limitations to the definition of the toxicity sequence of PFASs substitutes. For example, based on the results of perfluorinated butyl organic ammonium salt cationic surfactants on the target organism, Soirodela polyrhiza, Scenedesmus brananda, Daphnia pulex, and G. rarus, almost no toxicity has been observed (Wang et al., 2015). However, in teratogenicity experiments on X. laevis embryos and development experiments on Rana nigromaculata embryos, its toxicity was much higher than that of PFOS (Ren et al., 2012). Therefore, to ensure the reliability of toxicity conclusions, the toxicity of aquatic organisms should be systematically designed and carried out at multiple trophic levels to confirm that the classification of toxicity levels is not determined only by selecting the experimental results of a single model organism. However, on the basis of the current research results, we can generally conclude that the application of F-53B, GenX, FC-98, PFO5DoDA, PFO4DA, textile finishing agent, and perfluorinated butyl organic ammonium salt surfactant as alternatives is still questionable.
Conceptualization, TM, PW, and QL; Formal analysis, TM, PW, LW, and XL; Funding acquisition, TM; Investigation, PW, QL, and TM; Methodology, TM and QL; Project administration, YL; Resources, PW, LW, QL, and XL; Software, PW; Visualization, YL; Writing–original draft, TM; Writing–review and editing, YL. All authors have approved the submitted version of the manuscript.
We gratefully acknowledge the funding from the National Natural Science Foundation of China (42077143 and 41877127), The Young Top-notch Talent Cultivation Program of Hubei Province, Outstanding Young and Middle-aged Science and Technology Innovation Team Project of the Hubei Provincial Department of Education (HPDE) (T2020016), and the Training Fund Program for Scientific Research of Hubei University of Arts and Science (2020KYPYTD005).
We thank the qualified native English-speaking editors at Language Editing Services from Elsevier (Date: 01-Nov-2022; Serial number: LE-252025-97E92B614065) for editing the manuscript in correct English language usage, grammar, punctuation and spelling.
PW was employed by Jiangsu Rainfine Environmental Science and Technology Co., Ltd.
The remaining authors declare that the research was conducted in the absence of any commercial or financial relationships that could be construed as a potential conflict of interest.
All claims expressed in this article are solely those of the authors and do not necessarily represent those of their affiliated organizations, or those of the publisher, the editors and the reviewers. Any product that may be evaluated in this article, or claim that may be made by its manufacturer, is not guaranteed or endorsed by the publisher.
The Supplementary Material for this article can be found online at: https://www.frontiersin.org/articles/10.3389/fenvs.2023.1101100/full#supplementary-material
Abdel-Gawad, F. K., Khalil, W. K. B., El-Kady, A. A., Waly, A. I., and Abdel-Wahhab, M. A. (2016). Carboxymethyl chitosan modulates the genotoxic risk and oxidative stress of perfluorooctanoic acid in Nile tilapia. (Oreochromis niloticus. J. Saudi Soc. Agric. Sci. 15, 57–66. doi:10.1016/j.jssas.2014.04.005
Ahrens, L., and Bundschuh, M. (2014). Fate and effects of poly- and perfluoroalkyl substances in the aquatic environment: A review. Environ. Toxicol. Chem. 33, 1921–1929. doi:10.1002/etc.2663
Ankley, G. T., Kuehl, D. W., Kahl, M. D., Jensen, K. M., Butterworth, B. C., and Nichols, J. W. (2004). Partial life-cycle toxicity and bioconcentration modeling of perfluorooctanesulfonate in the northern leopard frog (Rana pipiens). Environ. Toxicol. Chem. 23, 2745–2755. doi:10.1897/03-667
Ankley, G. T., Kuehl, D. W., Kahl, M. D., Jensen, K. M., Linnum, A., Leino, R. L., et al. (2005). Reproductive and developmental toxicity and bioconcentration of perfluorooctanesulfonate in a partial life-cycle test with the fathead minnow (Pimephales Promelas). Environ. Toxicol. Chem. 24, 2316–2324. doi:10.1897/04-634r.1
Annunziato, K. M., Doherty, J., Lee, J., Clark, J. M., Liang, W., Clark, C. W., et al. (2020). Chemical characterization of a legacy aqueous film-forming foam sample and developmental toxicity in zebrafish (Danio rerio). Environ. Health Perspect. 128, 097006. doi:10.1289/ehp6470
Arukwe, A., and Mortensen, A. S. (2011). Lipid peroxidation and oxidative stress responses of salmon fed a diet containing perfluorooctane sulfonic- or perfluorooctane carboxylic acids. Comp. Biochem. Physiol. Part C. Toxicol. Pharmacol. 154, 288–295. doi:10.1016/j.cbpc.2011.06.012
Birkholz, D. A., Stilson, S. M., and Elliott, H. S. (2014). Analysis of emerging contaminants in drinking water – A review. Compr. Water Qual. Purif., 212–229. doi:10.1016/b978-0-12-382182-9.00035-9
Buck, R. C., Franklin, J., Berger, U., Conder, J. M., Cousins, I. T., de Voogt, P., et al. (2011). Perfluoroalkyl and polyfluoroalkyl substances in the environment: Terminology, classification, and origins. Integr. Environ. Assess. Manag. 7, 513–541. doi:10.1002/IEAM.258
Chen, J., Das, S. R., La Du, J., Corvi, M. M., Bai, C., Chen, Y., et al. (2012). Chronic PFOS exposures induce life stage-specific behavioral deficits in adult zebrafish and produce malformation and behavioral deficits in F1 offspring. Environ. Toxicol. Chem. 32, 201–206. doi:10.1002/etc.2031
Chen, J., Tanguay, R. L., Tal, T. L., Gai, Z., Ma, X., Bai, C., et al. (2014). Early life perfluorooctanesulphonic acid (PFOS) exposure impairs zebrafish organogenesis. Aquat. Toxicol. 150, 124–132. doi:10.1016/j.aquatox.2014.03.005
Chen, S. (2021). Toxicological effects of 1H,1H,2H,2H perfluorooctane sulfonic acid (6:2 FTS) in zebrafish and cell. Wuhan: Master's Degree Dissertation of Jianghan University.
Chen, W. F., Zhao, Q. S., and Yin, D. Q. (2009). Treatment with PFOS causes point mutations in zebrafish embryos genome. Environ. Chem. 28, 215–219.
Chen, X., Zhang, W., Hu, K., Sun, L., and Huang, X. (2019). Inhibition kinetics of perfluorohexic acid on NAGase from liver of tilapia. J. Fujian Agric. For. Univ. (Nat. Sci. Ed.) 48, 97–102. doi:10.13323/j.cnki.j.fafu(nat.sci.)
Chen, Y. H. (2012). Study on the toxicity of PFOS and PFOA to zebrafish embryos. Dalian: Master’s Degree Dissertation of Dalian Maritime University.
Cheng, J., Lv, S., Nie, S., Liu, J., Tong, S., Kang, N., et al. (2016). Chronic perfluorooctane sulfonate (PFOS) exposure induces hepatic steatosis in zebrafish. Aquat. Toxicol. 176, 45–52. doi:10.1016/j.aquatox.2016.04.013
Cheng, Y., Cui, Y., Chen, H., and Xie, W. (2011). Thyroid disruption effects of environmental level perfluorooctane sulfonates (PFOS) in Xenopus laevis. Ecotoxicol 20, 2069–2078. doi:10.1007/s10646-011-0749-3
Cheng, Y., Cui, Y., Dang, Z. C., Xie, W. P., Li, H. S., Yin, H. H., et al. (2012a). Effects of perfluorooctane sulfonate (PFOS) exposure on vitellogenin mRNA level in zebrafish (Brachydanio rerio). Environ. Sci. 33, 1865–1870. doi:10.13227/j.hjkx.2012.06.005
Cheng, Y., Cui, Y., He, P., Dang, Z., Xie, W., Yin, H., et al. (2012b). Effect of perfluorooctane sulfonate (PFOS) on vitellogenin levels in blood plasma and tissue homogenate of zebrafish (Brachydanio rerio). Asian J. Ecotoxicol. 7, 65–70.
Colombo, I., Wolf, W. de, Thompson, R. S., Farrar, D. G., Hoke, R. A., and L’Haridon, J. (2008). Acute and chronic aquatic toxicity of ammonium perfluorooctanoate (APFO) to freshwater organisms. Ecotoxicol. Environm. Saf. 71, 749–756. doi:10.1016/j.ecoenv.2008.04.002
Cui, Y. (2012). Altered lipid metabolism and glucose metabolism in zebrafish exposed to perfluorooctanesuiphonic acid. Master’s Degree Dissertation of Wenzhou Medical College.
Cui, Y., Bai, C., Xu, T., Wang, X., Chen, Y., and Jin, D. (2012). PFOA-induced developmental toxicity, behavior change and DNA damage in zebrafish embryos. Asian J. Ecotoxicol. 7, 241–250.
Cui, Y., Lv, S., Liu, J., Noe, S., Chen, J., Dong, Q., et al. (2016). Chronic perfluorooctanesulfonic acid exposure disrupts lipid metabolism in zebrafish. Hum. Exp. Toxicol. 36, 207–217. doi:10.1177/0960327116646615
Dai, Y., Zhao, J., Sun, C., Li, D., Liu, X., Wang, Z., et al. (2022). Interaction and combined toxicity of microplastics and per- and polyfluoroalkyl substances in aquatic environment. Front. Environ. Sci. Eng. 16, 136. doi:10.1007/s11783-022-1571-2
Dang, H. L. (2015). The toxicity and mechanisms of perfluorinated compounds on Cynoglossus semilaevis liver cells. Dalian: Master’s Degree Dissertation of Dalian Polytechnic University.
Dang, H., Na, G., Gao, H., Li, R., Gao, Y., Yao, Z., et al. (2015). Toxicity effects of perfluorooctane sulfonate (PFOS) on liver cells of Cynoglossus semilaevis. Asian J. Ecotoxicol. 10, 162–169. doi:10.7524/AJE.1673-5897.20141111002
Dasgupta, S., Reddam, A., Liu, Z., Liu, J., and Volz, D. C. (2020). High-content screening in zebrafish identifies perfluorooctanesulfonamide as a potent developmental toxicant. Environ. Pollut. 113550, 113550. doi:10.1016/j.envpol.2019.113550
Deng, M. (2018). Effects of F-53B on thyroid function and antioxidant status and its mechanism in zebrafish. Nanchang: Doctor’s Degree Dissertation of Nanchang University.
Ding, G., Zhang, J., Chen, Y., Wang, L., Wang, M., Xiong, D., et al. (2013). Combined effects of PFOS and PFOA on zebrafish (Danio rerio) embryos. Arch. Environ. Contam. Toxicol. 64, 668–675. doi:10.1007/s00244-012-9864-2
Domingo, J. L., and Nadal, M. (2019). Human exposure to per- and polyfluoroalkyl substances (PFAS) through drinking water: A review of the recent scientific literature. Environ. Res. 177, 108648. doi:10.1016/j.envres.2019.108648
Dong, G., Zhang, R., Huang, H., Lu, C., Xia, Y., Wang, X., et al. (2021). Exploration of the developmental toxicity of TCS and PFOS to zebrafish embryos by whole-genome gene expression analyses. Environ. Sci. Pollut. Res. 28, 56032–56042. doi:10.1007/s11356-021-14527-9
Dorts, J., Kestemont, P., Marchand, P. -A., D’Hollander, W., Thézenas, M. -L., Raes, M., et al. (2011). Ecotoxicoproteomics in gills of the sentinel fish species, Cottus gobio, exposed to perfluorooctane sulfonate (PFOS). Aquat. Toxicol. 103, 1–8. doi:10.1016/j.aquatox.2011.01.015
Du, G., Huang, H., Hu, J., Qin, Y., Wu, D., Song, L., et al. (2013). Endocrine-related effects of perfluorooctanoic acid (PFOA) in zebrafish, H295R steroidogenesis and receptor reporter gene assays. Chemosphere 91, 1099–1106. doi:10.1016/j.chemosphere.2013.01.012
Du, J. (2016). Joint toxicity effects of PFOS and nano-Zno on zebrafish. Harbin: Doctor’s Degree Dissertation of Harbin Institute of Technology.
Du, J., Wang, S. T., Liu, Z., and You, H. (2015a). PFOS and ZnO nanoparticles induced oxidative Stress and apoptosis in zebrafish (Danio rerio). Asian J. Ecotoxicol. 10, 238–247. doi:10.7524/AJE.1673-5897-20141121001
Du, J., Wang, S. T., and You, H. (2015b). Co-exposure to PFOS and nano-ZnO disrupted the hypothalamus-pituitary-thyroid axis in zebrafish larvae. Asian J. Ecotoxicol. 10, 144–153. doi:10.7524/AJE.1673-5897.20150528004
Du, J., Wang, S., You, H., Jiang, R., Zhuang, C., and Zhang, X. (2014). Developmental toxicity and DNA damage to zebrafish induced by perfluorooctane sulfonate in the presence of ZnO nanoparticles. Environ. Toxicol. 31, 360–371. doi:10.1002/tox.22050
Du, Y., Shi, X., Liu, C., Yu, K., and Zhou, B. (2009). Chronic effects of water-borne PFOS exposure on growth, survival and hepatotoxicity in zebrafish: A partial life-cycle test. Chemosphere 74, 723–729. doi:10.1016/j.chemosphere.2008.09.075
Fang, C., Huang, Q., Ye, T., Chen, Y., Liu, L., Kang, M., et al. (2013). Embryonic exposure to PFOS induces immunosuppression in the fish larvae of marine medaka. Ecotoxicol. Environ. Saf. 92, 104–111. doi:10.1016/j.ecoenv.2013.03.005
Fang, C., Wu, X., Huang, Q., Liao, Y., Liu, L., Qiu, L., et al. (2012). PFOS elicits transcriptional responses of the ER, AHR and PPAR pathways in Oryzias melastigma in a stage-specific manner. Aquat. Toxicol. 106-107, 9–19. doi:10.1016/j.aquatox.2011.10.009
Feng, M., He, Q., Meng, L., Zhang, X., Sun, P., and Wang, Z. (2015). Evaluation of single and joint toxicity of perfluorooctane sulfonate, perfluorooctanoic acid, and copper to Carassius auratus using oxidative stress biomarkers. Aquat. Toxicol. 161, 108–116. doi:10.1016/j.aquatox.2015.01.025
Flynn, R. W., Chislock, M. F., Gannon, M. E., Bauer, S. J., Tornabene, B. J., Hoverman, J. T., et al. (2019). Acute and chronic effects of perfluoroalkyl substance mixtures on larval American bullfrogs (Rana catesbeiana). Chemosphere 236, 124350. doi:10.1016/j.chemosphere.2019.124350
Flynn, R. W., Hoover, G., Iacchetta, M., Guffey, S., Perre, C. D., Huerta, B., et al. (2022). Comparative toxicity of aquatic per- and polyfluoroalkyl substance exposure in three species of amphibians. Environ.Toxicol. Chem. 41, 1407–1415. doi:10.1002/etc.5319
Flynn, R. W., Iacchetta, M., Perre, C., Lee, L., Sepúlveda, M. S., and Hoverman, J. T. (2020). Chronic PFAS-exposure under environmentally relevant conditions delays development in northern leopard frog (Rana pipiens) larvae. Environ. Toxicol. Chem. 40, 711–716. doi:10.1002/etc.4690
Fort, D. J., Mathis, M. B., Guiney, P. D., and Weeks, J. A. (2018). Evaluation of the developmental toxicity of perfluorooctanesulfonate in the Anuran, Silurana tropicalis. J. Appl. Toxicol. 1, 365–374. doi:10.1002/jat.3727
Gaballah, S., Swank, A., Sobus, J. R., Howey, X. M., Schmid, J., Catron, T., et al. (2020). Evaluation of developmental toxicity, developmental neurotoxicity, and tissue dose in zebrafish exposed to GenX and other PFAS. Environ. Health Perspect. 128, 047005. doi:10.1289/ehp5843
Gao, Y. F. (2016). Complex toxicity of metal and PFOS or PFOA on Cynoglossus semilaevis liver cells. Dalian: Master’s Degree Dissertation of Dalian Polytechnic University.
Geeraerts, C., and Belpaire, C. (2009). The effects of contaminants in European eel: A review. Ecotoxicol 19, 239–266. doi:10.1007/s10646-009-0424-0
Giesy, J. P., and Kannan, K. (2001). Global distribution of perfluorooctane sulfonate in wildlife. Environ. Sci. Technol. 35, 1339–1342. doi:10.1021/es001834k
Godfrey, A., Abdel-moneim, A., and Sepúlveda, M. S. (2017a). Acute mixture toxicity of halogenated chemicals and their next generation counterparts on zebrafish embryos. Chemosphere 181, 710–712. doi:10.1016/j.chemosphere.2017.04.146
Godfrey, A., Hooser, B., Abdelmoneim, A., Horzmann, K. A., Freemanc, J. L., and Sepúlveda, M. S. (2017b). Thyroid disrupting effects of halogenated and next generation chemicals on the swim bladder development of zebrafish. Aquat. Toxicol. 193, 228–235. doi:10.1016/j.aquatox.2017.10.024
Guo, J., Wu, P., Cao, J., Luo, Y., Chen, J., Wang, G., et al. (2019). The PFOS disturbed immunomodulatory functions via nuclear factor-kappa B signaling in liver of zebrafish (Danio rerio). Fish. Shellfish Immunol. 91, 87–98. doi:10.1016/j.fsi.2019.05.018
Guo, X., Zhang, S., Lu, S., Zheng, B., Xie, P., Chen, J., et al. (2018). Perfluorododecanoic acid exposure induced developmental neurotoxicity in zebrafish embryos. Environ. Pollut. 241, 1018–1026. doi:10.1016/j.envpol.2018.06.013
Hagenaars, A., Knapen, D., Meyer, I. J., van der Ven, K., Hoff, P., and De Coen, W. (2008). Toxicity evaluation of perfluorooctane sulfonate (PFOS) in the liver of common carp (Cyprinus carpio). Aquat. Toxicol. 88, 155–163. doi:10.1016/j.aquatox.2008.04.002
Hagenaars, A., Vergauwen, L., De Coen, W., and Knapen, D. (2011). Structureactivity relationship assessment of four perfluorinated chemicals using a prolonged zebrafish early life stage test. Chemosphere 82, 764–772. doi:10.1016/j.chemosphere.2010.10.076
Han, J., and Fang, Z. (2010). Estrogenic effects, reproductive impairment and developmental toxicity in ovoviparous swordtail fish (Xiphophorus helleri) exposed to perfluorooctane sulfonate (PFOS). Aquat. Toxicol. 99, 281–290. doi:10.1016/j.aquatox.2010.05.010
Han, Z., Liu, Y., Wu, D., Zhu, Z., and Lü, C. (2012). Immunotoxicity and hepatotoxicity of PFOS and PFOA in tilapia (Oreochromis niloticus). Chin. J. Geochem. 31, 424–430. doi:10.1007/s11631-012-0593-z
Hao, R. B. (2019). Effects of PFOS and PFOS-K on the activities of antioxidant enzymes and expression of hormone receptors related genes in Cynoglossus semilaevis. Tianjin: Master’s Degree Dissertation of Tianjin Agricultural University.
Hekster, F. M., Laane, R. W. P. M., and de Voogt, P. (2003). Environmental and toxicity effects of perfluoroalkylated substances. Rev. Environ. Contam. Toxicol. 179, 99–121. doi:10.1007/0-387-21731-2_4
Hoff, P. T., Van Campenhout, K., Van de Vijver, K., Covaci, A., Bervoets, L., Moens, L., et al. (2005). Perfluorooctane sulfonic acid and organohalogen pollutants in liver of three freshwater fish species in flanders (Belgium): Relationships with biochemical and organismal effects. Environ. Pollut. 137, 324–333. doi:10.1016/j.envpol.2005.01.008
Hoff, P. T., Van Dongen, W., Esmans, E. L., Blust, R., and De Coen, W. M. (2003). Evaluation of the toxicological effects of perfluorooctane sulfonic acid in the common carp (Cyprinus carpio). Aquat. Toxicol. 62, 349–359. doi:10.1016/s0166-445x(02)00145-5
Hoke, R. A., Ferrell, B. D., Sloman, T. L., Buck, R. C., and Buxton, L. W. (2016). Aquatic hazard, bioaccumulation and screening risk assessment for ammonium 2, 3, 3, 3-tetrafluoro-2-(heptafluoropropoxy)-propanoate. Chemosphere 149, 336–342. doi:10.1016/j.chemosphere.2016.01.009
Hu, Q. (2009). Embryo developmental and maternal toxicity of perfluorooctane sulfonate (PFOS) in the zebrafish Zhong. Master's Degree Dissertation of Huazhong. Wuhan: Agricultural University.
Hu, Q., Zhou, Z., Zhou, Q., Zhang, J., and Liang, Y. (2009). Acute effects of perfluorooctane sulfonate on microstructure of the gill of zebrafish (Danio rerio). Asian J. Ecotoxicol. 4, 530–536. http://159.226.240.226/handle/311016/5388.
Hu, W. Y., Jones, P. D., DeCoen, W., King, L., Fraker, P., Newsted, J., et al. (2003). Alterations in cell membrane properties caused by perfluorinated compounds. Comp. Biochem. Physiol. C 135, 77–88. doi:10.1016/s1532-0456(03)00043-7
Huang, H., Huang, C., Wang, L., Ye, X., Bai, C., Simonich, M. T., et al. (2010). Toxicity, uptake kinetics and behavior assessment in zebrafish embryos following exposure to perfluorooctanesulphonicacid (PFOS). Aquat. Toxicol. 98, 139–147. doi:10.1016/j.aquatox.2010.02.003
Huang, Q., Dong, S., Fang, C., Wu, X., Ye, T., and Lin, Y. (2012). Deep sequencing-based transcriptome profiling analysis of Oryzias melastigma exposed to PFOS. Aquat. Toxicol. 120-121, 54–58. doi:10.1016/j.aquatox.2012.04.013
Huang, Q., Fang, C., Wu, X., Fan, J., and Dong, S. (2011). Perfluorooctane sulfonate impairs the cardiac development of a marine medaka (Oryzias melastigma). Aquat. Toxicol. 105, 71–77. doi:10.1016/j.aquatox.2011.05.012
Huang, Q. S., Chen, Y. J., Fang, C., Kang, M., and Dong, S. J. (2013). The effects of salinity on the toxicology of PFOS to marine medaka (Oryzias melastigma). Chi. Sci. Bull. Chin. Ver.) 58, 151–157. doi:10.1360/972012-538
Huang, Z. B., Wu, S. Q., Shi, C. B., Pan, H. J., and Li, K. B. (2000). Study on biology characteristics of swordtails. Xiphophorus Helleri. J. Fish. Sci. China 7, 107–109.
Huang, Z. Y. (2022). Water quality criteria and risk assessment of typical short-chain perfluorinated substitutes. Hefei: Master’s Degree Dissertation of Anhui Jianzhu University.
Jantzen, C. E., Annunziato, K. A., Bugel, S. M., and Cooper, K. R. (2016b). PFOS, PFNA, and PFOA sub-lethal exposure to embryonic zebrafish have different toxicity profiles in terms of morphometrics, behavior and gene expression. Aquat. Toxicol. 175, 160–170. doi:10.1016/j.aquatox.2016.03.026
Jantzen, C. E., Annunziato, K. M., and Cooper, K. R. (2016a). Behavioral, morphometric, and gene expression effects in adult zebrafish (Danio rerio) embryonically exposed to PFOA, PFOS, and PFNA. Aquat. Toxicol. 180, 123–130. doi:10.1016/j.aquatox.2016.09.011
Jasrotia, R., Langer, S., and Dhar, M. (2021). Endocrine disrupting chemicals in aquatic ecosystem: An emerging threat to wildlife and human health. Proc. Zool. Soc. 74, 634–647. doi:10.1007/s12595-021-00410-5
Ji, K., Kim, Y., Oh, S., Ahn, B., Jo, H., and Choi, K. (2008). Toxicity of perfluorooctane sulfonic acid and perfluorooctanoic acid on freshwater macroinvertebrates (Daphnia Magna and Moina Macrocopa) and fish (Oryzias Latipes). Environ. Toxicol. Chem. 27, 2159–2168. doi:10.1897/07-523.1
Kang, J. S., Ahn, T. G., and Park, J. W. (2019). Perfluorooctanoic acid (PFOA) and perfluooctane sulfonate (PFOS) induce different modes of action in reproduction to Japanese medaka (Oryzias latipes). J. Hazard. Mat. 368, 97–103. doi:10.1016/j.jhazmat.2019.01.034
Kannan, K., Tao, L., Sinclair, E., Pastva, S. D., Jude, D. J., and Giesy, J. P. (2005). Perfluorinated compounds in aquatic organisms at various trophic levels in a Great Lakes food chain. Arch. Environ. Contam. Toxicol. 48, 559–566. doi:10.1007/s00244-004-0133-x
Keiter, S., Baumann, L., Färber, H., Holbech, H., Skutlarek, D., Engwall, M., et al. (2012). Long-term effects of a binary mixture of perfluorooctane sulfonate (PFOS) and bisphenol A (BPA) in zebrafish (Danio rerio). Aquat. Toxicol. 118-119, 116–129. doi:10.1016/j.aquatox.2012.04.003
Khazaee, M., Guardian, M. G. E., Aga, D. S., and Ng, C. A. (2020). Impacts of sex and exposure duration on gene expression in zebrafish following perfluorooctane sulfonate exposure. Environ. Toxicol. Chem. 39, 437–449. doi:10.1002/etc.4628
Kim, S., Ji, K., Lee, S., Lee, J., Kim, J., Kim, S., et al. (2011). Perfluorooctane sulfonic acid exposure increases cadmium toxicity in early life stage of zebrafish, Danio rerio. Environ. Toxicol. Chem. 30, 870–877. doi:10.1002/etc.443
Krøvel, A. V., Søfteland, L., Torstensen, B., and Olsvik, P. A. (2008). Transcriptional effects of PFOS in isolated hepatocytes from Atlantic salmon Salmo salar L. Comp. Biochem. Physiol. Part C. Toxicol. Pharmacol. 148, 14–22. doi:10.1016/j.cbpc.2008.03.001
Lai, H. (2021). Impacts of polystyrene microplastics and F-53B on zebrafish immune system and intestinal microbial diversity. Nanchang: Master’s Degree Dissertation of Jiangxi Normal University.
Lee, J. W., Lee, J.-W., Shin, Y.-J., Kim, J.-E., Ryu, T.-K., Ryu, J., et al. (2017). Multi-generational xenoestrogenic effects of Perfluoroalkyl acids (PFAAs) mixture on Oryzias latipes using a flow-through exposure system. Chemosphere 169, 212–223. doi:10.1016/j.chemosphere.2016.11.035
Li, J., He, J., Niu, Z., and Zhang, Y. (2020). Legacy per- and polyfluoroalkyl substances (PFASs) and alternatives (short-chain analogues, F-53B, GenX and FC-98) in residential soils of China: Present implications of replacing legacy PFASs. Environ. Int. 135, 105419. doi:10.1016/j.envint.2019.105419
Li, J. W. (2018). Effects and mechanisms of chlorinated polyfluorinated ether sulfonate on green algae and rare minnow. Dalian: Master’s Degree Dissertation of Dalian University of Technology.
Li, Y., Han, Z., Zheng, X., Ma, Z., Liu, H., Giesy, J. P., et al. (2015). Comparison of waterborne and in ovo nanoinjection exposures to assess effects of PFOS on zebrafish embryos. Environ. Sci. Pollut. Res. 22, 2303–2310. doi:10.1007/s11356-014-3527-y
Liu, C., Du, Y., and Zhou, B. (2007a). Evaluation of estrogenic activities and mechanism of action of perfluorinated chemicals determined by vitellogenin induction in primary cultured tilapia hepatocytes. Aquat. Toxicol. 85, 267–277. doi:10.1016/j.aquatox.2007.09.009
Liu, C., Yu, K., Shi, X., Wang, J., Lam, P., Wu, R., et al. (2007b). Induction of oxidative stress and apoptosis by PFOS and PFOA in primary cultured hepatocytes of freshwater tilapia (Oreochromis niloticus). Aquat. Toxicol. 82, 135–143. doi:10.1016/j.aquatox.2007.02.006
Liu, M., Yin, H. W., Chen, X. Q., Li, K., Yang, J., Zhang, J. J., et al. (2013). Aquatic toxicities of potassium perfluorobutane sulfonate as potential alternative of perfluorooctane sulfonate to multi - species of different trophic levels. Asian J. Ecotoxicol. 8, 714–721. doi:10.7524/AJE.1673-5897.20120710003
Liu, Q. P. (2009). Screening thyroid hormone and sex hormone disrupting effects of perfluorooctanesulfonate (PFOS) using Xenopus. Shanghai: Master’s Degree Dissertation of East China Normal University.
Liu, Q, Q., Qian, L., Guo, S., and Shi, H. (2008). Effects of Perfluorooctanesulfonate on the growth, metamorphosis, thyroid and gonadal histology of Xenopus laevis. Asian J. Ecotoxicol. 3, 464–472.
Liu, Y., Wang, J., Fang, X., Zhang, H., and Dai, J. (2011). The thyroid-disrupting effects of long-term perfluorononanoate exposure on zebrafish (Danio rerio). (Danio rerio. Ecotoxicol. 20, 47–55. doi:10.1007/s10646-010-0555-3
Liu, Y, Y., Wang, J., Wei, Y., Zhang, H., Xu, M., and Dai, J. (2008). Induction of time-dependent oxidative stress and related transcriptional effects of perfluorododecanoic acid in zebrafish liver. Aquat. Toxicol. 89, 242–250. doi:10.1016/j.aquatox.2008.07.009
Lou, Q.-Q., Zhang, Y.-F., Zhou, Z., Shi, Y.-L., Ge, Y.-N., Ren, D.-K., et al. (2013). Effects of perfluorooctanesulfonate and perfluorobutanesulfonate on the growth and sexual development of Xenopus laevis. Ecotoxicol 22, 1133–1144. doi:10.1007/s10646-013-1100-y
Lu, L., Chang, J., Chang, Y., and Ma, J. (2019). Fluorinated diiodine alkanes exert developmental toxicity on embryo-larval stages of zebrafish strain AB via regulating the expression of the specific endocrine-related genes. J. Appl. Toxicol. 39, 1691–1700. doi:10.1002/jat.3893
Lu, X. M., and Chen, P. P. (2012). Reproductive toxic effects and mechanisms of the male frog Rana nigromaculata induced by low dose PFOA. Acta Sci. Circumstantiae 32, 1497–1502. doi:10.13671/j.hjkxxb.2012.06.008
Menger, F., Pohl, J., Ahrens, L., Carlsson, G., and Örn, S. (2020). Behavioural effects and bioconcentration of per- and polyfluoroalkyl substances (PFASs) in zebrafish (Danio rerio) embryos. Chemosphere 245, 125573. doi:10.1016/j.chemosphere.2019.125573
Mhadhbi, L., Rial, D., Pérez, S., and Beiras, R. (2012). Ecological risk assessment of perfluorooctanoic acid (PFOA) and perfluorooctanesulfonic acid (PFOS) in marine environment using Isochrysis galbana, Paracentrotus lividus, Siriella armata and. Psetta maxima. J. Environ. Monit. 14, 1375. doi:10.1039/c2em30037k
Miranda, A. F., Trestrail, C., Lekamge, S., and Nugegoda, D. (2020). Effects of perfluorooctanoic acid (PFOA) on the thyroid status, vitellogenin, and oxidant–antioxidant balance in the Murray River rainbowfish. Ecotoxicol 29, 163–174. doi:10.1007/s10646-020-02161-z
Mortensen, A. S., Letcher, R. J., Cangialosi, M. V., Chu, S., and Arukwe, A. (2011). Tissue bioaccumulation patterns, xenobiotic biotransformation and steroid hormone levels in Atlantic salmon (Salmo salar) fed a diet containing perfluoroactane sulfonic or perfluorooctane carboxylic acids. Chemosphere 83, 1035–1044. doi:10.1016/j.chemosphere.2011.01.067
Oakes, K. D., Sibley, P. K., Martin, J. W., MacLean, D. D., Solomon, K. R., Mabury, S. A., et al. (2005). Short-term exposures of fish to perfluorooctane sulfonate: Acute effects on fatty acyl–coa oxidase activity, oxidative stress, and circulating sex steroids. Environ. Toxicol. Chem. 24, 1172–1181. doi:10.1897/04-419.1
Oakes, K. D., Sibley, P. K., Solomon, K. R., Mabury, S. A., and Van Der Kraak, G. J. (2004). Impact of perfluorooctanoic acid on Fathead Minnow (Pimephales Promelas) fatty acyl-coa oxidase activity, circulating steroids, and reproduction in outdoor microcosms. Environ. Toxicol. Chem. 23, 1912–1919. doi:10.1897/03-190
Oh, J. H., Moon, H.-B., and Choe, E. S. (2012). Alterations in differentially expressed genes after repeated exposure to perfluorooctanoate and perfluorooctanesulfonate in liver of Oryzias latipes. Arch. Environ. Contam. Toxicol. 64, 475–483. doi:10.1007/s00244-012-9840-x
Pan, W., Han, J., and Shen, H. (2020). Effect of PFOA on the biologic toxicity in zebrafish. Appl. Chem. Ind. 49, 2442–2446. doi:10.16581/j.cnki.issn1671-3206.20200724.020
Pan, W. Y. (2019). Biotoxicological effects and risk assessment of zebrafish by PFOS and PFOA. Shijiazhuang: Master’s Degree Dissertation of Hebei University of Science and Technology.
Ren, D., Su, H., Liu, P., Wei, R., and Qin, Z. (2012). Developmental toxicity of perfluorooctane sulfonate (PFOS) and its substitutes to amphibian embryos. Asian J. Ecotoxicol. 7, 561–564.
San-Segundo, L., Guimarães, L., Fernández Torija, C., Beltrán, E. M., Guilhermino, L., and Pablos, M. V. (2016). Alterations in gene expression levels provide early indicators of chemical stress during Xenopus laevis embryo development: A case study with perfluorooctane sulfonate (PFOS). Ecotoxicol. Environ. Saf. 127, 51–60. doi:10.1016/j.ecoenv.2016.01.005
Sant, K. E., Annunziato, K., Conlin, S., Teicher, G., Chen, P., Venezia, O., et al. (2021). Developmental exposures to perfluorooctanesulfonic acid (PFOS) impact embryonic nutrition, pancreatic morphology, and adiposity in the zebrafish, Danio rerio. Environ. Pollut. 275, 116644. doi:10.1016/j.envpol.2021.116644
Shen, C., and Zuo, Z. (2020). Zebrafish (Danio rerio) as an excellent vertebrate model for the development, reproductive, cardiovascular, and neural and ocular development toxicity study of hazardous chemicals. Environ. Sci. Pollut. Res. 27, 43599–43614. doi:10.1007/s11356-020-10800-5
Shi, G., Cui, Q., Pan, Y., Sheng, N., Guo, Y., and Dai, J. (2017a). 6:2 fluorotelomer carboxylic acid (6:2 FTCA) exposure induces developmental toxicity and inhibits the formation of erythrocytes during zebrafish embryogenesis. Aquat. Toxicol. 190, 53–61. doi:10.1016/j.aquatox.2017.06.023
Shi, G., Cui, Q., Pan, Y., Sheng, N., Sun, S., Guo, Y., et al. (2017b). 6:2 Chlorinated polyfluorinated ether sulfonate, a PFOS alternative, induces embryotoxicity and disrupts cardiac development in zebrafish embryos. Aquat. Toxicol. 185, 67–75. doi:10.1016/j.aquatox.2017.02.002
Shi, G., Guo, H., Sheng, N., Cui, Q., Pan, Y., Wang, J., et al. (2018a). Two-generational reproductive toxicity assessment of 6:2 chlorinated polyfluorinated ether sulfonate (F-53B, a novel alternative to perfluorooctane sulfonate) in zebrafish. Environ. Pollut. 243, 1517–1527. doi:10.1016/j.envpol.2018.09.120
Shi, G., Xie, Y., Guo, Y., and Dai, J. (2018b). 6:2 fluorotelomer sulfonamide alkylbetaine (6:2 FTAB), a novel perfluorooctane sulfonate alternative, induced developmental toxicity in zebrafish embryos. Aquat. Toxicol. 195, 24–32. doi:10.1016/j.aquatox.2017.12.002
Shi, X., Du, Y., Lam, P. K. S., Wu, R. S. S., and Zhou, B. (2008). Developmental toxicity and alteration of gene expression in zebrafish embryos exposed to PFOS. Toxicol. Appl. Pharmacol. 230, 23–32. doi:10.1016/j.taap.2008.01.043
Shi, X., Liu, C., Wu, G., and Zhou, B. (2009). Waterborne exposure to PFOS causes disruption of the hypothalamus–pituitary–thyroid axis in zebrafish larvae. Chemosphere 77, 1010–1018. doi:10.1016/j.chemosphere.2009.07.074
Shi, X., and Zhou, B. (2010). The role of Nrf2 and MAPK pathways in pfos-induced oxidative stress in zebrafish embryos. Toxicol. Sci. 115, 391–400. doi:10.1093/toxsci/kfq066
Shi, Y., Song, X., Jin, Q., Li, W., He, S., and Cai, Y. (2020). Tissue distribution and bioaccumulation of a novel polyfluoroalkyl benzenesulfonate in crucian carp. Environ. Int. 135, 105418. doi:10.1016/j.envint.2019.105418
Shi, Y., Vestergren, R., Nost, T. H., Zhou, Z., and Cai, Y. (2018c). Probing the differential tissue distribution and bioaccumulation behavior of per- and polyfluoroalkyl substances of varying chain-lengths, isomeric structures and functional groups in Crucian Carp. Environ. Sci. Technol. 52, 4592–4600. doi:10.1021/acs.est.7b06128
Silva, A. V., Ringblom, J., Lindh, C., Scott, K., Jakobsson, K., and Öberg, M. (2020). A probabilistic approach to evaluate the risk of decreased total triiodothyronine hormone levels following chronic exposure to PFOS and PFHxS via contaminated drinking water. Environ. Health Perspect. 128, 076001. doi:10.1289/ehp6654
Su, H., Ren, D., Cao, S., and Qin, Z. (2012). Acute toxicity of perfluorooctane sulfonate (PFOS) and its substitutes to Amphibian Tadpoles. Asian J. Ecotoxicol. 7, 521–524.
Sun, M., Arevalo, E., Strynar, M., Lindstrom, A., Richardson, M., Kearns, B., et al. (2016). Legacy and emerging perfluoroalkyl substances are important drinking water contaminants in the Cape Fear River Watershed of North Carolina. Environ. Sci. Technol. Lett. 3, 415–419. doi:10.1021/acs.estlett.6b00398
Suski, J. G., Salice, C. J., Chanov, M. K., Ayers, J., Rewarts, J., and Field, J. (2020). Sensitivity and accumulation of perfluorooctanesulfonate (PFOS) and perfluorohexanesulfonic acid (PFHxS) in Fathead Minnows (Pimephales promelas) exposed over critical life-stages of reproduction and development. Environ. Toxicol. Chem. 40, 811–819. doi:10.1002/etc.4936
Teymourian, T., Teymoorian, T., Kowsari, E., and Ramakrishna, S. (2021). A review of emerging pfas contaminants: Sources, fate, health risks, and a comprehensive assortment of recent sorbents for PFAS treatment by evaluating their mechanism. Res. Chem. Int. 47, 4879–4914. doi:10.1007/s11164-021-04603-7
Tong, W. C. (2021). Study on the effects of typical environmental pollutants on swimming behavior of zebrafish larvae. Wuhan: Master’s Degree Dissertation of Huazhong Agricultural University.
Tornabene, B. J., Chislock, M. F., Gannon, M. E., Sepúlveda, M. S., and Hoverman, J. T. (2021). Relative acute toxicity of three per- and polyfluoroalkyl substances on nine species of larval amphibians. Integr. Environ. Assess. Manage. 17, 684–690. doi:10.1002/ieam.4391
Truong, L., Rericha, Y., Thunga, P., Marvel, S., Wallis, D., Simonich, M. T., et al. (2022). Systematic developmental toxicity assessment of a structurally diverse library of PFAS in zebrafish. J. Hazard. Mat. 431, 128615. doi:10.1016/j.jhazmat.2022.128615
Tu, W., Martínez, R., Navarro-Martin, L., Kostyniuk, D. J., Hum, C., Huang, J., et al. (2019). Bioconcentration and metabolic effects of emerging PFOS alternatives in developing zebrafish. Environ. Sci. Technol. 53, 13427–13439. doi:10.1021/acs.est.9b03820
Ulhaq, M., Carlsson, G., Örn, S., and Norrgren, L. (2013a). Comparison of developmental toxicity of seven perfluoroalkyl acids to zebrafish embryos. Environ.Toxicol. Pharmacol. 36, 423–426. doi:10.1016/j.etap.2013.05.004
Ulhaq, M., Örn, S., Carlsson, G., Morrison, D. A., and Norrgren, L. (2013b). Locomotor behavior in zebrafish (Danio rerio) larvae exposed to perfluoroalkyl acids. Aquat. Toxicol. 144-145, 332–340. doi:10.1016/j.aquatox.2013.10.021
Van Rijn, J. P., Van Straalen, N. M., and Willems, J. (1995). Handboek bestrijdingsmiddelen, gebruik en milieu-effecten. Amsterdam, Netherlands: VU Uitgeverij.
Vogs, C., Johanson, G., Näslund, M., Wulff, S., Sjödin, M., Hellstrandh, M., et al. (2019). Toxicokinetics of perfluorinated alkyl acids influences their toxic potency in the zebrafish embryo (Danio rerio). Environ. Sci. Technol. 53, 3898–3907. doi:10.1021/acs.est.8b07188
Wang, B. N. (2021). Toxic effects of three typical water pollutants on zebrafish embryos. Beijing: Master’s Degree Dissertation Chin. Acad. Agric. Sci.
Wang, D. D., Qian, Y. S., Zhang, H. J., and Ni, W. M. (2015). Toxic effects of persistent organic pollutants PFOA on immune cells of crucian carp. Academic Annual Meeting of Chinese Society for Environmental SciencesEnvironmental Medicine and Health, 4821–4826. Chapter 8.
Wang, H., Ma, S., Zhang, Z., Chen, H., Huang, Z., Gong, X., et al. (2013). Effects of perfluorooctanesulfonate (PFOS) on antioxidant enzyme in the gill of Pagrosomus major and damage to its tissues. J. Ecol. Rural. Environ. 29, 98–105. doi:10.3969/j.issn.1673-4831.2013.01.017
Wang, H. W. (2012). Effects of perfluorooctanesulfonate on antioxidant system, tissues injury and genes expression of Perna viridis and Pagrosomus major. Shanghai: Master’s Degree Dissertation of Shanghai Ocean University.
Wang, H., Zhang, Z., Ma, S., Chen, H., Huang, Z., and Gong, X. (2012). Effect of perfluorooctane sulfonate potassium on glutathione content and glutathione S-transferase activity of red sea bream. South China Fish. Sci. 8, 23–28. doi:10.3969/j.issn.2095-0780.2012.04.004
Wang, J, J., Shi, G., Yao, J., Sheng, N., Cui, R., Su, Z., et al. (2020). Perfluoropolyether carboxylic acids (novel alternatives to PFOA) impair zebrafish posterior swim bladder development via thyroid hormone disruption. Environ. Int. 134, 105317. doi:10.1016/j.envint.2019.105317
Wang. M, M., Chen, H. H., Zha, J. M., and Wang, Z. J. (2015). Ecotoxicity of alternatives of typical perfluorooctane sulfonate (PFOS) to four native aquatic organisms. Asian J. Ecotoxicol. 10, 230–235. doi:10.7524/AJE.1673.589720141022001
Wang, M., Chen, J., Lin, K., Chen, Y., Hu, W., Tanguay, R. L., et al. (2011a). Chronic zebrafish PFOS exposure alters sex ratio and maternal related effects in F1 offspring. Environ. Toxicol. Chem. 30, 2073–2080. doi:10.1002/etc.594
Wang, S. Y. (2017). Reproductive toxic effects and mechanisms of PFOA on male Rana Nigromaculata. Hangzhou: Master’s Degree Dissertation of Hangzhou Normal University.
Wang, X. Y, X. Y., Dai, Y. Y., Jia, L., Wang, Q. S., and Chen, C. X. (2020). Effects of perfluorooctane sulfonate (PFOS) on immune-related gene expression in half-smooth tongue sole (Cynoglossus semilaevis). Asian J. Ecotoxicol. 15, 71–80. doi:10.7524/AJE.1673-5897.20190710001
Wang, Y., Zhou, Q., Wang, C., Yin, N., Li, Z., Liu, J., et al. (2011b). Estrogen-like response of perfluorooctyl iodide in male medaka (Oryzias latipes) based on hepatic vitellogenin induction. Environ. Toxicol. 28, 571–578. doi:10.1002/tox.20751
Wei, R., Su, H., and Qin, Z. (2012). Developmental toxicity of perfluorooctane sulfonate, perfluorohexane sulfonate and perfluorobutane sulfonate to Xenopus embryos. Asian J. Ecotoxicol. 7, 542–548. http://ir.rcees.ac.cn/handle/311016/8363.
Wei, Y., Chan, L. L., Wang, D., Zhang, H., Wang, J., and Dai, J. (2008a). Proteomic analysis of hepatic protein profiles in rare minnow (Gobiocypris rarus) exposed to perfluorooctanoic acid. J. Proteome Res. 7, 1729–1739. doi:10.1021/pr7008059
Wei, Y., Dai, J., Liu, M., Wang, J., Xu, M., Zha, J., et al. (2007). Estrogen-like properties of perfluorooctanoic acid as revealed by expressing hepatic estrogen-responsive genes in rare minnows (Gobiocypris Rarus). Environ. Toxicol. Chem. 26, 2440–2447. doi:10.1897/07-008r1.1
Wei, Y., Liu, Y., Wang, J., Tao, Y., and Dai, J. (2008b). Toxicogenomic analysis of the hepatic effects of perfluorooctanoic acid on rare minnows (Gobiocypris rarus). Toxicol. Appl. Pharmacol. 226, 285–297. doi:10.1016/j.taap.2007.09.023
Wei, Y., Shi, X., Zhang, H., Wang, J., Zhou, B., and Dai, J. (2009). Combined effects of polyfluorinated and perfluorinated compounds on primary cultured hepatocytes from rare minnow (Gobiocypris rarus) using toxicogenomic analysis. Aquat. Toxicol. 95, 27–36. doi:10.1016/j.aquatox.2009.07.020
Wen, W., Xia, X., Hu, D., Zhou, D., Wang, H., Zhai, Y., et al. (2017). Long-chain perfluoroalkyl acids (PFAAs) affect the bioconcentration and tissue distribution of short-chain PFAAs in zebrafish (Danio rerio). Environ. Sci. Technol. 51, 12358–12368. doi:10.1021/acs.est.7b03647
Wu, X., Huang, Q., Fang, C., Ye, T., Qiu, L., and Dong, S. (2012). PFOS induced precocious hatching of Oryzias melastigma – from molecular level to individual level. Chemosphere 87, 703–708. doi:10.1016/j.chemosphere.2011.12.060
Wu, Y., Deng, M., Jin, Y., Mu, X., He, X., Luu, N.-T., et al. (2019b). Uptake and elimination of emerging polyfluoroalkyl substance F-53B in zebrafish larvae: Response of oxidative stress biomarkers. Chemosphere 215, 182–188. doi:10.1016/j.chemosphere.2018.10.025
Wu, Y., Huang, J., Deng, M., Jin, Y., Yang, H., Liu, Y., et al. (2019a). Acute exposure to environmentally relevant concentrations of Chinese PFOS alternative F-53B induces oxidative stress in early developing zebrafish. Chemosphere 235, 945–951. doi:10.1016/j.chemosphere.2019.07.016
Xia, J. -G., Nie, L. -J., Mi, X. -M., Wang, W. -Z., Ma, Y. -J., Cao, Z. -D., et al. (2015). Behavior, metabolism and swimming physiology in juvenile Spinibarbus sinensis exposed to PFOS under different temperatures. Fish. Physiol. Biochem. 41, 1293–1304. doi:10.1007/s10695-015-0086-1
Xia, J, J., Fu, S., Cao, Z., Peng, J., Peng, J., Dai, T., et al. (2013). Ecotoxicological effects of waterborne PFOS exposure on swimming performance and energy expenditure in juvenile goldfish (Carassius auratus). J. Environ. Sci. 25, 1672–1679. doi:10.1016/s1001-0742(12)60219-8
Xia. J. G, J. G., Niu, C. J., and Sun, L. Y. (2013). Ecotoxicological effects of exposure to PFOS on embryo and larva of zebrafish Danio rerio. Acta Ecol. Sin. 33, 7408–7416. doi:10.5846/stxb201207291073
Yang, J. (2020). Endocrine disrupting effects and mechanisms of chlorinated polyfluorinated ether sulfonate on Rare Minnows. Dalian: Master’s Degree Dissertation of Dalian University of Technology.
Yang, J. P. (2014a). The preliminary study for toxicity effects of toxicology of PFCs on Daphnia Magna and Zebrafish Embryo. Shijiazhuang: Master’s Degree Dissertation of Hebei University of Science and Technology.
Yang, X., Song, F., Peng, L., Hu, Y. L., Cheng, B., and Ding, Q. (2020). The toxic effects of 1-iodoperfluorooctane and its regulation of long-chain non-coding RNA MALAT-1. Asian J. Ecotoxicol. 15, 149–157. doi:10.7524/AJE.1673-5897.20190103002
Yang, X. X. (2014b). Toxicity of bisphenol AF and perfluorinated chemicals on zebrafish. Wuhan: Master’s Degree Dissertation of Huazhong Agriculture University.
Yao, D. (2018). Effects of perfluorooctane sulfonate (PFOS) on liver and muscle of Danio rerio. Shanghai: Master’s Degree Dissertation of Shanghai Ocean University.
Yao, D., Wu, H., and Jiang, M. (2018). Effects of perfluorooctane sulfonate on the liver metabolism of Danio rerio. Asian J. Ecotoxicol. 13, 97–106. doi:10.7524/AJE.1673-5897.20180305002
Ye, L. (2008). Study on the aquatic toxicology effects of two typic perfluorochemical to zebrafish embryos. Shanghai: Master’s Degree Dissertation of Tongji University.
Ye, L.‚ W, L. L., Jiang, Y. X., Zhang, C. J., and Chen, L. (2009). Toxicological study of PFOS/PFOA to zebrafish (Danio rerio) embryos. Environ. Sci. 30, 1727–1732. doi:10.13227/.hjkx.2009.06.004
Yu, Y. Y. (2011). The chronic toxic effects of perfluorotridecanoate acid (PFTriDA) on Medaka. Jinan: Master’s Degree Diserrtation of Shandong Normal University.
Yuan, L., Pan, R., and Jiang, M. (2015). Effects of PFOS on the physiological and biochemical indices of. Danio rerio J. Shanghai Ocean. Univ. 24, 869–879.
Yuan, L. Y. (2015). Effects of PFOS on physiological and biochemical indices of Scenedesmus obliquus and Danio rerio. Shanghai: Master's Degree Dissertation of Shanghai Ocean University.
Zakaria, A. F., Yahaya, N., Raznisyafiq, M., Loh, S. H., and Kamaruzaman, S. (2022). Recent advances in applications of hybrid natural polymers as adsorbent for perfluorinated compounds removal – review paper. J. Polym. Res. 29, 21. doi:10.1007/s10965-021-02820-7
Zhang, J., and Fang, Z. (2013). Effects of perfluorooctane sulfonate on hepatic antioxidant enzyme activities in swordtails (Xiphophorus helleri). Acta labo. Anim. Sci. Sin. 21, 61–67. doi:10.3969/j.issn.1005-4847.2013.04.014
Zhang, J., Liang, Y., and Fang, Z. (2014a). The effects of perfluorooctane sulfonate on Cu/Zn-SOD and stress-related gene expression in swordtails (Xiphophorus helleri). J. Fish. China 38, 482–490. doi:10.3724/SP.J.1231.2014.49002
Zhang, L., Li, Y., Chen, T., Xia, W., Zhou, Y., Wan, Y., et al. (2011). Abnormal development of motor neurons in perfluorooctane sulphonate exposed zebrafish embryos. Ecotoxicol 20, 643–652. doi:10.1007/s10646-011-0604-6
Zhang, L., Li, Y., Zeng, H., Wei, J., Wan, Y., Chen, J., et al. (2010). MicroRNA expression changes during zebrafish development induced by perfluorooctane sulfonate. J. Appl. Toxicol. 31, 210–222. doi:10.1002/jat.1583
Zhang, S. N. (2018). The thyroid-disrupting effects of PFDoA and PFHxA on zebrafish larvae. Taiyuan: Master’s Degree Dissertation of Shanxi University.
Zhang, X. (2020). Neurotoxicity and immunotoxicity of perfluorooctanoic acid on juvenile Cynoglossus semilaevis through transcriptome analysis. Qingdao: Master’s Degree Dissertation of Qingdao University.
Zhao, X., Ren, X., Duan, X., Luo, Z., and Zhu, R. (2016). On the effect of PFOS on developmental toxicity and oxidative stress in zebrafish embryos. J. Tangshan Univ. 29, 12–16. doi:10.16160/j.cnki.tsxyxb.2016.06.004
Zhao, Z., Li, J., Zhang, X., Wang, L., Wang, J., and Lin, T. (2022). Perfluoroalkyl and polyfluoroalkyl substances (PFASs) in groundwater: Current understandings and challenges to overcome. Environ. Sci. Pollut. Res. 29, 49513–49533. doi:10.1007/s11356-022-20755-4
Zheng, X.-M., Liu, H.-L., Shi, W., Wei, S., Giesy, J. P., and Yu, H.-X. (2012). Effects of perfluorinated compounds on development of zebrafish embryos. Environ. Sci. Pollut. Res. 19, 2498–2505. doi:10.1007/s11356-012-0977-y
Zou, W. L. (2021). The toxicokinetics and toxic mechanism of PFOS alternative OBS in zebrafish (Danio rerio). Nanchang: Doctor’s Degree Dissertation of Nanchang University.
Keywords: aquatic toxicity, perfluorooctane surlfonate (PFOS), perflurooctanoic acid (PFOA), fish, amphibian
Citation: Ma T, Wu P, Wang L, Li Q, Li X and Luo Y (2023) Toxicity of per- and polyfluoroalkyl substances to aquatic vertebrates. Front. Environ. Sci. 11:1101100. doi: 10.3389/fenvs.2023.1101100
Received: 17 November 2022; Accepted: 09 January 2023;
Published: 19 January 2023.
Edited by:
Cheng Zhang, Jiangnan University, ChinaReviewed by:
Yuhe He, City University of Hong Kong, Hong Kong SAR, ChinaCopyright © 2023 Ma, Wu, Wang, Li, Li and Luo. This is an open-access article distributed under the terms of the Creative Commons Attribution License (CC BY). The use, distribution or reproduction in other forums is permitted, provided the original author(s) and the copyright owner(s) are credited and that the original publication in this journal is cited, in accordance with accepted academic practice. No use, distribution or reproduction is permitted which does not comply with these terms.
*Correspondence: Quanguo Li, bHFnQGhidWFzLmVkdS5jbg==
Disclaimer: All claims expressed in this article are solely those of the authors and do not necessarily represent those of their affiliated organizations, or those of the publisher, the editors and the reviewers. Any product that may be evaluated in this article or claim that may be made by its manufacturer is not guaranteed or endorsed by the publisher.
Research integrity at Frontiers
Learn more about the work of our research integrity team to safeguard the quality of each article we publish.