- Sino-Dutch R&D Centre for Future Wastewater Treatment Technologies, Key Laboratory of Urban Stormwater System and Water Environment, Beijing University of Civil Engineering and Architecture, Beijing, China
Mining and smelting result in vanadium (V) being released into the environment. Biologically removing V(V) with washing water of rice (WWR) was investigated in this study. Over a 7-d trial, the V(V) removal efficiency increased with dosing washing water of rice dosage up to 56.6%. The results demonstrated that washing water of rice could be used as carbon and microbial sources for biologically reducing V(V). Using domesticated sludge as the inoculum could enhance V(V) detoxification performance, and 95.5% of V(V) was removed in the inoculated system for 5 d. Soluble V(V) was transformed into insoluble V(IV) (VO2), which could be further removed with precipitation. In addition to ABC transporters, a two-component system was also involved in V(V) reduction. The study confirmed that washing water of rice could be utilized for V(V) bio-detoxification.
1 Introduction
Vanadium (V) pollution is a persistent problem in numerous parts of the world, such as the Persian Gulf (Pourang et al., 2005), California (Wright and Belitz, 2010), Colorado River Basin (United States) (Noël et al., 2017), and the area of Mount Fuji (Japan) (Ono et al., 2019). Although V is an essential element for normal cell growth, it becomes toxic to animal cells at concentrations higher than 1–10 nM (Yelton et al., 2013). Adding 182 μg of V2O5 three times a week for a month can lead to neurobehavioral and neurochemical damage (Ngwa et al., 2014). The limit of V for drinking water quality in China is 0.01 mg/L (GB26452-2022). Although V removal by biological, physical, or chemical methods are all effective, the main difference lies in the input of economic and environmental costs (Birgitta et al., 2007). The application of adsorbent and co-precipitation technology requires a large amount of the absorbent or chemical agent. Although efficient and cost-effective biological methods are considered appropriate elimination techniques, attracting more and more attention (Lai et al., 2018), heterotrophic microbes could reduce V(V) to V(IV) by using organics as electron donors (Yelton et al., 2013). However, organic matter in V(V) contaminated wastewater is generally stoichiometrically deficient; therefore, adding carbon substrates can improve stability and efficiency, such as glucose (Hao et al., 2015), acetate (Liu et al., 2016), and soluble starch (Hao et al., 2016). However, the remediation fee would correspondingly increase substantially in this way, as acetate (0.042–15.2 CNY/mL), lactate (0.082–0.088 CNY/mL), glucose (0.05 CNY/g), citrate (89.6–166 CNY/g), and soluble starch (0.074–8.39 CNY/g) (www.reagent.com.cn). Hence, it is vital to ascertain apposite economical carbon sources for actual remediation of V(V) contamination.
Waste products are considered a potentially economical and environmentally friendly source of carbon. Rice is the second fastest growing grain and also the main food source for more than two-thirds of humans all over the world (He et al., 2016b). The annual consumption of rice in China has recently been estimated at 120–130 million t (Zhong and Zhang, 2014). Cleaning first is required before use; thus, large amounts of washing water of rice (WWR) are produced (He et al., 2019). According to statistics, the annual per capita WWR emission is 2,573.2 L in China (Huang D. et al., 2019). Depending on its use for direct consumption, rice can also be used to make a wide variety of processed foods. A single winery (Xiangshan Winery, Quanzhou County, Guilin) could produce wastewater during the production process up to 20 t/d with main sewage from WWR (Wang et al., 2003). WWR-containing solid particles composed of starch, proteins, and vitamins would adversely affect nearby freshwater ecosystems if discharged directly (Watanabe et al., 2011; Zou et al., 2012). Separate treatment of the WWR would also require significant capital, manpower, and material resources. A sewage treatment station (design scale: 35 m3/d) adopts a UASB reactor and contact oxidation method to treat WWR, and the project investment is 95,000 CNY, with 0.96 CNY/m3 of daily power consumption (Jiang et al., 2004). For a winery producing WWR as the main wastewater (20 t/d), the total investment of the treatment station project is 180,000 CNY and 1.54 CNY/t of wastewater for the operating cost (electricity, labor, and maintenance) (Wang et al., 2003). From an economic view, the utilization of WWR could still further save economic costs and resources compared with commonly used liquid carbon sources. Moreover, WWR was already proven to be successful as both carbon and microbial sources for denitrification (He et al., 2016a; He et al., 2016b; He et al., 2019). WWR could be another vital alternative microbial and carbon source for V(V) remediation in terms of environmental protection and resource conservation. However, it has not been attempted before and needs further confirmation.
Furthermore, revealing bacterial community structures in the WWR-based V(V) bio-detoxification processes would be vital and can give insights into the microbial restoring mechanisms. Recently, dominant and functional bacteria in V(V) reduction systems using various kinds of carbon sources have been widely determined (Hao et al., 2021c; Shi et al., 2021; Chen et al., 2022). The highly toxic V(V) could be reduced to the less toxic V(IV) by many species in a polluted environment (V(V)-reducing-related bacteria, VRB) (Supplementary Table S1). VRB was the community that possessed a common V reduction function with a vital role in the related process (Wang et al., 2020). To the best of our knowledge, this was the first V(V) bio-detoxification system based on WWR to analyze V(V) reduction from the perspective of WWR utilization and microbial community.
This study has focused on the potential V(V) bio-detoxification based on WWR. The main objectives were to 1) investigate the feasibility of V(V) removal by using WWR as both microbial and carbon sources; 2) explore the relationship between V(V) removal and WWR utilization with its various dosages; 3) evaluate the enhanced removal of V(V) with inoculated sludge based on WWR; and 4) identify the core microbiome responsible for mediating community response to V(V) pollution and elucidate the core role of VRB with V(V) stress.
2 Materials and methods
2.1 Experimental program
2.1.1 WWR as both microbial and carbon sources
Rice was obtained from northeast China (Supplementary Figure S1A), and 200 g of rice was washed with deionized water (350 mL) three times for WWR collection (Supplementary Figure S1B) to investigate whether WWR could contribute to V(V) reduction as both microbial and carbon sources. TOC (total organic carbon) in the WWR was around 208.8 mg/L. To better simulate the actual application, 0, 20, 50, 100, and 150 mL WWR were added to serum bottles (250 mL), and correspondingly, deionized water was supplemented to 250 mL (Supplementary Figure S2A). Tests in serum bottles were repeated in duplicate. NaVO3 was added to the whole 250 mL liquid with the given 75 mg V(V)/L concentration. The whole experiment period was also set as 7 days and cultivated at 35°C in a constant temperature incubator (Nicolet iS5, Thermo Scientific, United States).
2.1.2 Enhanced V(V) reduction with WWR and supernumerary inocula
For the experiment, 200 g of rice was washed three times in a 1-L beaker with deionized water. The washed water was then collected and mixed as WWR. V(V) was added directly to above 800 mL WWR as synthetic V(V) pollution in the form of NaVO3 with the given concentration of 75 mg V(V)/L. Sludge was collected from the Gaobeidian wastewater treatment plant (Beijing, China), which was domesticated at room temperature for 3 months before inoculation. The culture solution was prepared by adding CH3COONa (1.6 g/L) and NaVO3 (0.17942 g/L) to tap water and replaced every 3 days. Acetate was selected as the carbon source in the domestication stage since it was widely used in previous studies, including actual V(V) remediation applications (Yelton et al., 2013).
Microcosm V(V) removal experiments were carried out in four 250-mL serum bottles containing 200 mL WWR with 75 mg/L V(V). The reactors were divided evenly into two groups, and the test was carried out simultaneously with and/or without the inoculated sludge (Supplementary Figure S2B): 1) indigenous experiments without inoculation in R1 and R2 (Group RC, the un-inoculated system); 2) 20 mL sludge centrifuged at 3,000 rpm (30 min) and the solid part was added to R3 and R4 (Group RS, the inoculated system). All reactors were purged by nitrogen gas for 5 min prior and then cultivated at 150 rpm and 35°C. The operation period was 5 days for both the inoculated and uninoculated systems.
2.1.3 Chemical analysis
The supernatant sample of 5 mL was periodically (1 day) collected from each reactor for analysis. Afterward, samples were filtered through 0.45-μm membrane filters. The V(V) concentration was measured using spectrophotometric methods at 601 nm (Cary 5000, Agilent Technologies, United States). ICP-OES (ICAP7200, Thermo Fisher Scientific, United States) determined the total V concentration in the solution. TOC of the liquid samples was measured (vario TOC cube, Elementar, Germany). XPS (K-Alpha, Thermo Scientific, United States) and XRD (Bruker D8 Advance, Bruker, Germany) was used to characterize the reaction products. Changes in WWR before and after utilization were compared by SEM (Zeiss Merlin Compact, Zeiss, Germany). An optical microscope was used to assess the changes in the morphology of samples (Axioscope, Carl Zeiss Microscopy GmbH, Germany).
2.1.4 Microbial analysis
Molecular biology analysis was performed to obtain the characteristics of the microbial population. Samples were collected in the reactors without (RC)/with (RS) inocula at the end of the operation and the inoculated sludge. Total genomic DNA was extracted, which was then pooled and amplified by PCR with primers 515FmodF (5′-GTGYCAGCMGCCGCGGTAA-3′) and 806RmodR (5′-GGACTACNVGGGTWTCTAAT-3′). The mixture of amplicons was used for high-throughput 16S rRNA gene pyrosequencing on MiSeq (Illumina, The United States) after being purified and quantified. Raw pyrosequencing data obtained from this study were deposited in the NCBI Sequence Read Archive database (No. PRJNA863753).
3 Results and discussion
3.1 WWR served as both microbial and carbon sources for V(V) removal
3.1.1 V(V) removal performance
V(V) concentrations varied with the dosage of WWR (Figure 1A). In the reactors with different WWR dosages, the removal trends of V(V) were also different. In group A, the control group, V(V) remained stable despite slight fluctuation. When WWR dosage was 20 mL (group B), V(V) was a minimum of 68.0 mg/L on day 3 and then fluctuated in a small range around 73.5 mg/L on day 7. Similar to group B, the concentration of V(V) in group C gradually reduced to 59.2 mg/L on day 2 and then slightly increased to 68.6 mg/L (day 6), and it was 58.4 mg/L on day 7, while the concentration of V(V) on day 2 had a little difference from that on day 7. V(V) in groups D and E decreased sharply in the first 2 days, decreasing to 34.8 and 29.1 mg/L on day 3, respectively. Subsequently, V(V) in group D fluctuated, and the reaction endpoint was 41.6 mg/L. V(V) in group E remained stable from 3 to 7 days, around 32.6 mg/L. Virtually all the groups (groups B, D, and E) achieved a relatively good removal performance on day 3. The solid particles in WWR might adsorb V(V) first, and then, they were hydrolyzed with the extension of time (Figures 2A, B). The adsorbed V(V) might be released back again into the liquid state (Watanabe, 2009; Huang Z. et al., 2019); meanwhile, microbes used organics contributing to V(V) reduction.
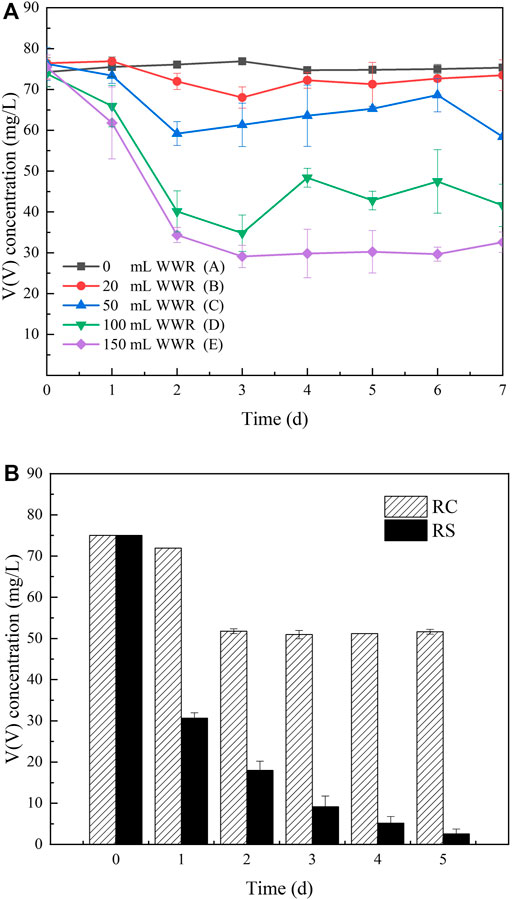
FIGURE 1. V(V) removal in various WWR dosage groups (A). V(V) changes in un-inoculated (RC) and inoculated (RS) systems (B).
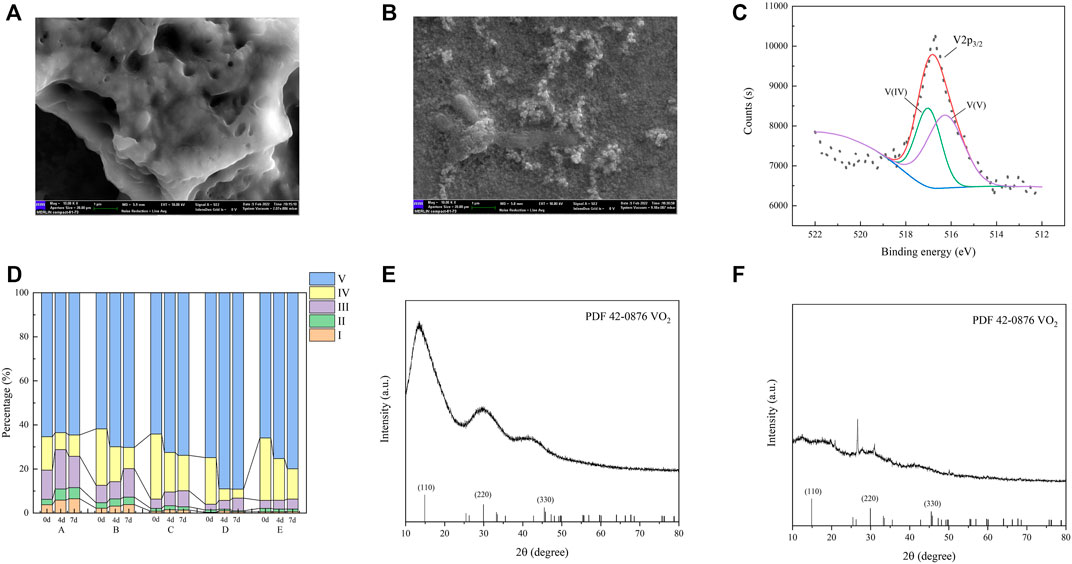
FIGURE 2. SEM images of WWR before (A) and after (B) use. XPS pattern of the precipitate in group D (100 mL WWR) (C). Characterization of the dissolved organic matter by fluorescence regional integration with various WWR dosages (D). XRD pattern of the precipitate in reactors without (E) and with (F) inoculated sludge.
As the dosage of WWR increased, V(V) removal increased with its removal efficiency around 2.0% (group B), 22.1% (group C), 44.5% (group D), and 56.6% (group E). Among them, the highest V(V) removal efficiency by unit WWR (group D) was up to 0.445% V(V)/mL WWR. The XPS (Figure 2C) pattern confirmed the presence of V(IV), which meant that V(V) was indeed reduced. Thus, the feasibility of microbially reducing V(V) with WWR was confirmed. WWR was also reported to be able to support the reduction of nitrate (He et al., 2016a; He et al., 2016b; He et al., 2019).
Moreover, the total V removal (Supplementary Figure S3A) has shown that it was almost consistent with V(V) with the final removal efficiency (7 d) as 14.1% (group B), 15.6% (group C), 21.7% (group D), and 33.0% (group E), while the maximum total V removal per unit WWR was obtained in group E (0.22% total V/mL WWR). Soluble V(IV) generated or a section of V(V) possibly adsorbed by solid particles of WWR was able to result in a higher removal performance of V(V) than total V (Noisuwan et al., 2011). However, it is obvious that V(V) removal needs to be further strengthened for better actual application, such as by using sludge inoculation and other appropriate methods.
3.1.2 WWR utilization
TOC results showed that the initial TOC in WWR was 208.8 mg/L, and the end-point TOC in group C (42.2 mg/L) was almost twice that in group B (21.2 mg/L). TOC concentrations in groups D and E were 74.6 and 110.3 mg/L, respectively (Supplementary Figure S3B). Results confirmed that WWR could provide both microbial and carbon sources for V(V) removal, while TOC concentration relations differed from the WWR dose ratio in the study. Solid particles in WWR might be continuously hydrolyzed into the solution as a supplement TOC (Nabayi et al., 2021).
Based on fluorescence spectra and the PARAFAC model, four kinds of fluorescence components in liquid samples were analyzed (Ex/Em = 340/440 nm for humic acid-like substances, Ex/Em = 270/340 nm for tryptophan, Ex/Em = 360 (270)/420, and Ex/Em = 375 (270)/485 nm for humic-like organics) (Baghoth et al., 2011; Hao et al., 2021a). In systems with various WWR dosages (groups A, B, C, D, and E), the proportion of organic matter in each zone changed along with the time (Supplementary Figure S4). As shown in Figure 2D, the results changed significantly in regions IV and V on days 0, 4, and 7. The humic acid-like substance area (region V) increased, and the soluble microbial products (region IV) decreased over time. The reduced proportion of soluble microbial products reflected the small number of microorganisms in the WWR, coupled with the lack of domestication prior to exploitation, which resulted in their inability to adapt to the system environment, thus reducing their activity and even dying. The polycarboxylate humic acid-like component (Ex/Em = 340/410 nm) was considered, produced by the dead organisms and the excrements of organisms (He et al., 2019).
The surface of the solid substance in WWR was rough and conducive to microbial adhesion growth but it changed after the utilization (Figures 2A, B) (Lee et al., 2017; Liu J. et al., 2021). The solid particles in the used WWR were significantly smaller, and the hole was significantly altered. It was speculated that functional microorganisms were attached to its surface.
3.2 Enhanced V(V) bio-detoxification by additional inocula
V(V) in the inoculated systems sharply decreased (Figure 1B), from 75.0 to 31.6 mg/L (1 d), 19.6 mg/L (2 d), and then steadily remained at around 3.4 mg V(V)/L until the end of tests (5 d). In contrast, V(V) in the un-inoculated systems barely decreased from 75.0 to 71.9 mg/L (1 d); afterward, it dramatically decreased to 51.8 mg/L (2 d) and then remained at a constant level of 51.6 mg/L (5 d). Finally, V(V) reduction efficiencies in the inoculated and un-inoculated systems were 95.5% and 31.2%, respectively. XRD (Figures 2E, F) analysis of solid samples after the reaction showed that VO2 was generated in both RC and RS. Therefore, enhanced V(V) removal could be effectively achieved by using domesticated sludge additionally as inocula based on WWR as a microbial and carbon source. Inoculation of acclimated sludge was necessary for better V(V) removal in the short term for actual remediation processes.
The V(V) removal rate obtained in the un-inoculated system (R1 and R2) was 9.6 mg V(V)/(L·d) lower than that in the inoculated system [14.3 mg V(V)/(L·d)]. In the present study, the WWR-based V(V) removal rate was higher than those obtained in previous studies using different carbon sources, such as 7.1 mg V(V)/(L·d) for glucose (Hao et al., 2021c), 7.82 mg V(V)/(L·d) for citrate (Wang et al., 2017), and 1.94 ± 0.04 mg V(V)/(L·d) for ethanol (Zhang et al., 2019a). The combined fermentation of peanut meal and WWR was used to produce volatile fatty acids as an additional carbon source (Huang D. et al., 2019), which also helped explain the faster V(V) removal rate by using WWR to some extent.
3.3 Evolution of microbial communities
The changes in the morphology of particles in WWR before use (Supplementary Figures S5A, B) and endpoint samples of un-inoculated (Supplementary Figures S5C, D) and inoculated systems (Supplementary Figures S5E, F) were observed by using an optical microscope. It seemed that the particles in the WWR were degraded and utilized in the microbial reduction process. Therefore, high-throughput analysis was used to reveal microbial evolution and function throughout the process.
Microbial communities were analyzed for samples S0 (the original inoculated sludge), RWW (the initial WWR), W1 (tests with sole WWR), and SW1 (tests with WWR and inocula). The coverage of all samples was above 99.6%, suggesting the results were able to represent a nearly overall microbial diversity and reflected the true state of the microbial community. Shannon and Ace indexes (Supplementary Table S2) in W1 were higher than those in the RWW sample, reflecting that WWR acted as an effective source of carbon, as did wheat straw (Hao et al., 2021a), altering the microbial diversity and richness in the system. Compared with S0, sample SW1 showed a declined richness and biodiversity. Compared with WWR as the sole microbial source, the inoculated sludge increased microbial richness and biodiversity in the RS group, probably contributing to the better V(V) removal performance.
Evolutions of communities were observed in all samples on the phylum level (Figures 3A, B). There were a total of 17 phyla detected in all samples, accounting for 63.6%, 50.0%, 18.9%, and 20.6% of the total phyla in the RWW, W1, S0, and SW1 samples, respectively (Figure 3A). Proteobacteria (RWW, W1, S0, and SW1), Firmicutes (W1, S0, SW1), Chloroflexi (S0, SW1), and Bacteroidetes (S0, SW1) dominated the communities (Figure 3B), identical to the main phyla detected in the V(V)-contaminated soil (Zhang et al., 2020). Firmicutes dominated W1, S0, and SW1 samples with abundances of 67.3%, 6.6%, and 13.7%, respectively. They could play an important role not only in plant growth promotion (bio-fertilizer) and plant pathogen biological control (biological control agent) but also in heavy metal remediation (Hashmi et al., 2020). Firmicutes were also considered highly culturable, with about 14% of the species in cultured specimens belonging to them (Hugenholtz, 2002). Proteobacteria was increased orderly in the S0 (16.5%), W1 (32.0%), and SW1 (62.3%) samples, taking the majority of abundance in SW1. In the marine environment, Proteobacteria participated in sulfur autotrophic sulfate reduction and denitrification (Zhou et al., 2020). Proteobacteria (38.5%) and Cyanobacteria (61.0%) were the majority in RWW, with the sum of them as high as 99.5%. Cyanobacteria can simultaneously fix nitrogen and release oxygen under aerobic and micro-aerobic conditions (Kulasooriya and Magana-Arachchi, 2016), which was one of the important contributors to paddy stability (Singh et al., 2018). Cyanobacteria, as photoautotrophs (Sánchez-Baracaldo and Cardona, 2020), also explained the sharp decrease of the proportions in W1, S0, and SW1. Results suggested that V(V) and WWR affected constituents of microbial communities.
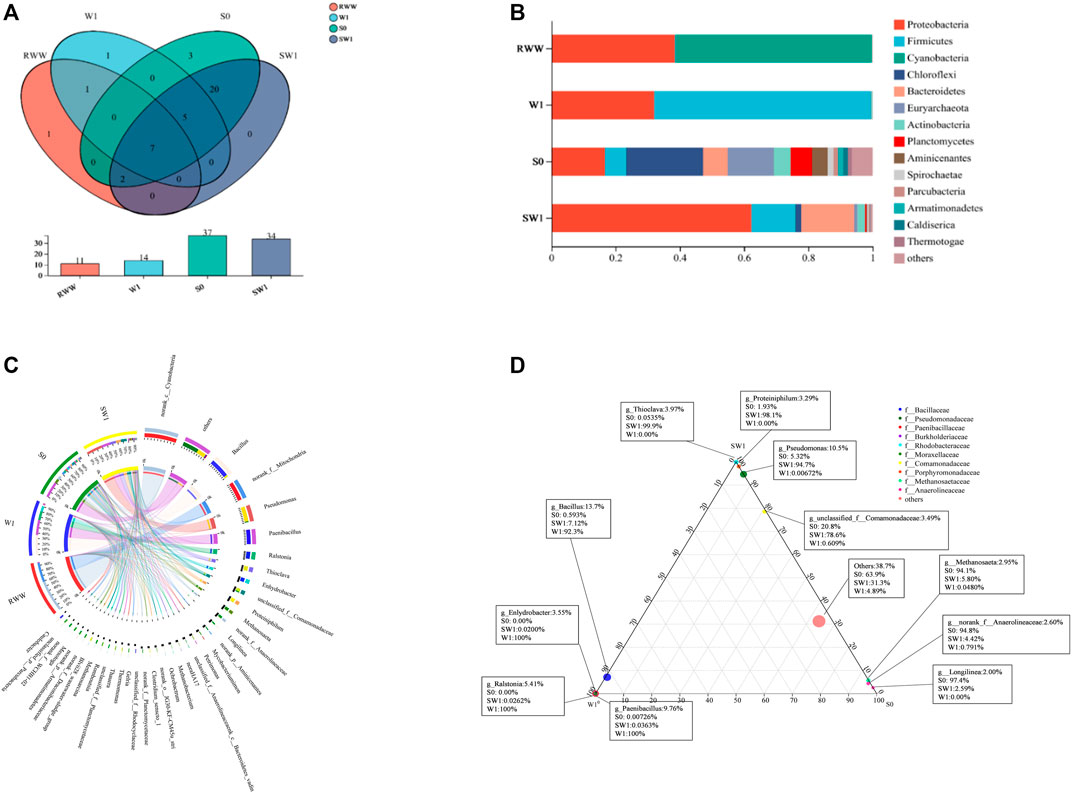
FIGURE 3. Venn diagram of samples (A) and percentage of community abundance (B) on the phylum level. Circos diagram (C) and ternary analysis (D) of microbial communities on the genus level.
3.4 Functional microbes and genes
Figure 3C suggests the various microbial communities in each group at the genus level. Unclassified_f_Comamonadaceae largely accounted for the communities in S0 (21.0%) and SW1 (78.0%), also reported to be the dominant bacterial community in the constructed wetland-microbial electrolytic cell coupling system (CW-MEC) (Xia et al., 2020). Patureau et al. (1996) found that Unclassified_f_Comamonadaceae can efficiently remove ammonia nitrogen and nitrite nitrogen. Bacillus was found in this study, and it accounted for 92.0% in W1, with a conspicuous decrease to 7.1% in SW1 (inoculated samples). Bacillus was one of the VRB, which was related to the V content (Wang et al., 2020). Two Bacillus sp., namely, F2.5 and F2.8, were able to produce hydrogen from organic components of municipal solid waste (Shah et al., 2016). Bacillus mycoides enhanced the redox of V ions by reducing the V(IV)/V(V) and V(II)/V(III) redox potentials at the porous lamellar carbon electrode used in the composite electrode (Deng et al., 2020). Moreover, some microbes in the communities might be able to use WWR to maintain activity and provide energy for V(V) bio-reduction. It was considered that the addition of WWR as the carbon source stimulated the enrichment of Pseudomonas in SW1 (0.20% in RWW, 5.3% in S0, and 94.0% in SW1). It was reported that carbon sources, like glucose, produced electron transfer intermediates such as NADPH and small organic acids in EPS, avoiding inhibition of reducibility under bacterial starvation (Dogan et al., 2011). Pseudomonas aeruginosa could use glucose rapidly as a nutrient to promote Cr(VI) reduction (Li et al., 2020). Pseudomonas stutzeri D6, selectively isolated from activated sludge, obtained more complete denitrification at a higher initial organic carbon concentration or a directly metabolic carbon type by increasing the oxygen consumption rate of substrate stimulation (Yang et al., 2012). Pseudomonas was isolated from the uranium mine (India), realizing Ni2+ (1,048 ng) or Cd2+ (700 ng) absorption for 1 mg dry weight, with preferential metal deposition happening in the cell envelope (Choudhary and Sar, 2009). Pseudomonas putida was reported to achieve 69% and 48% vanadate and uranyl ion removal, respectively, under alkaline conditions (Safonov et al., 2018).
In Figure 3D, the three corners represent three samples, the circles represent microbes at the genus level, and the size of the circles represents the average relative abundance of genera. Based on the ternary plot results, genera of g_Thioclava, g_Proteiniphilum, g_Pseudomonas, and g_unclassified_f_Comamonadaceae were mostly associated with the SW1 communities, whereas g_Bacillus was associated with microbial communities of sample W1, which were consistent with findings from the results in Figure 3C.
As shown in Figures 4A, B, the species of unclassified_g_Bacillus (38.1%) and Paenibacillus_riograndensis_SBR5 (24.9%) accounted for large proportions in communities of W1, while for SW1, Pseudomonas_stutzeri_g_Pseudomonas (29.3%) was the main species with a high abundance. Both Pseudomonas V-reductans strain T-1 (a plant-processing V-containing slags) and Pseudomonas V-reductans strain A-1 (an ascidian worm in the Bay of Kuril Islands, Pacific Ocean) separated from different places could reduce V(V) to V(IV) and V(III), respectively, with color changes (Lyalkova and Yurkova, 1992). Pseudomonas chlororaphis strain L19, isolated from stone coal soil, could perform bioleaching to release V ions from mineral ores (Peng et al., 2009). W1 and SW1 had a removal effect of V(V), and it was speculated that Bacillus belonged to VRB (Supplementary Table S1). Perhaps, due to the complexity of microbes in sludge, the high V(V) removal efficiency appeared in SW1 with a low abundance of Bacillus (2.9%). Wang et al. (2020) found that the existing Bacillus accounted for 6.61% ± 9.82% by means of the Panzhihua V smelter range of 10–4,000 m soil sampling inspection. Investigations have shown that V(V) was bio-reduced to less toxic by enriched Bacillus, and Thauera was proposed (Zhang et al., 2019b).
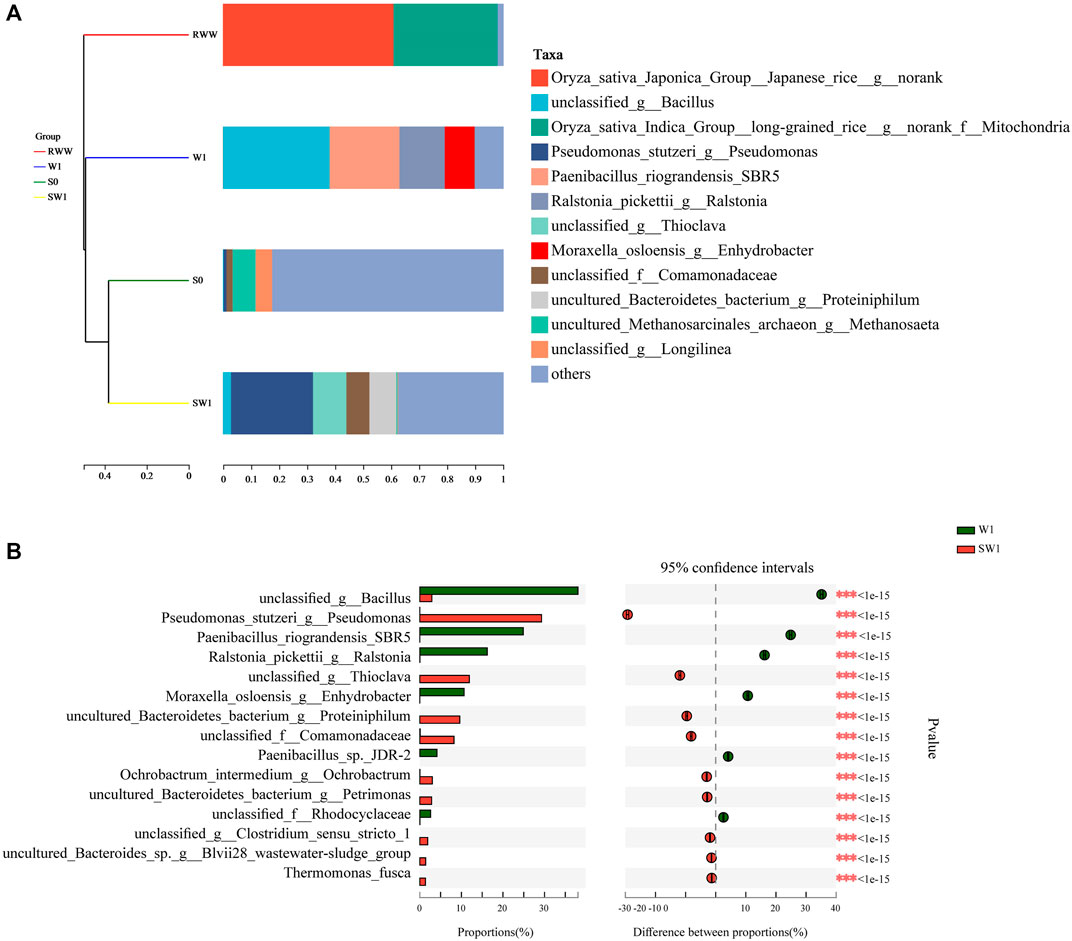
FIGURE 4. Hierarchical clustering tree (A) and Fisher’s exact test bar plot (B) on the species level.
Analyses with functional genes were summarized based on PICRUSt 2 to indicate the microbial variation (Figure 5). Genes involved in the biosynthesis of metabolic pathways, biosynthesis of secondary metabolites, microbial metabolism in diverse environments, biosynthesis of amino acids and pyruvate metabolism (metabolism), ABC transporters and two-component system (environmental information processing), and quorum sensing (cellular processes) in W1 and SW1 were enriched compared with RWW, while the functional enrichment of carbon metabolism in W1 reflected the presence of bacteria in RWW. Unexpectedly, the functional enrichment of ABC transporters in W1 was more obvious than in SW1. It was speculated that the complexity of inoculated sludge might support other ways for reducing V(V) in the system, such as sulfur metabolism and nitrogen metabolism in SW1. V(V) bio-reduction could be coupled with the biological oxidation of elemental sulfur [S(0)] and zero-valent iron [Fe(0)] to produce V(IV) (Zhang et al., 2018). Another reason for the lower ABC transporters and two-component system in SW1 was speculated to be competition. The A. vinelandii strain expressing the V-nitrogenase strain could reduce CO in vivo, and CO was reduced to hydrocarbons in the secondary metabolic pathway rather than being used as a carbon source for cell growth (Singh et al., 1993), which may compete for electrons with V(V) reduction. The two-component system was enriched in both W1 and SW1 (Figure 5A). It was reported that ABC transporters and two-component system were also the main function of the microbial community in mining areas and watersheds related to metal substances (As, Zn, and Pb) (Hou et al., 2019). ABC transporters and two-component system belonged to the top five enriched functional attributes in both active tailings (Liu Y. et al., 2021). Similarly, their enrichments were also found in biological V reduction by using wheat straw as the carbon source (Hao et al., 2021b).
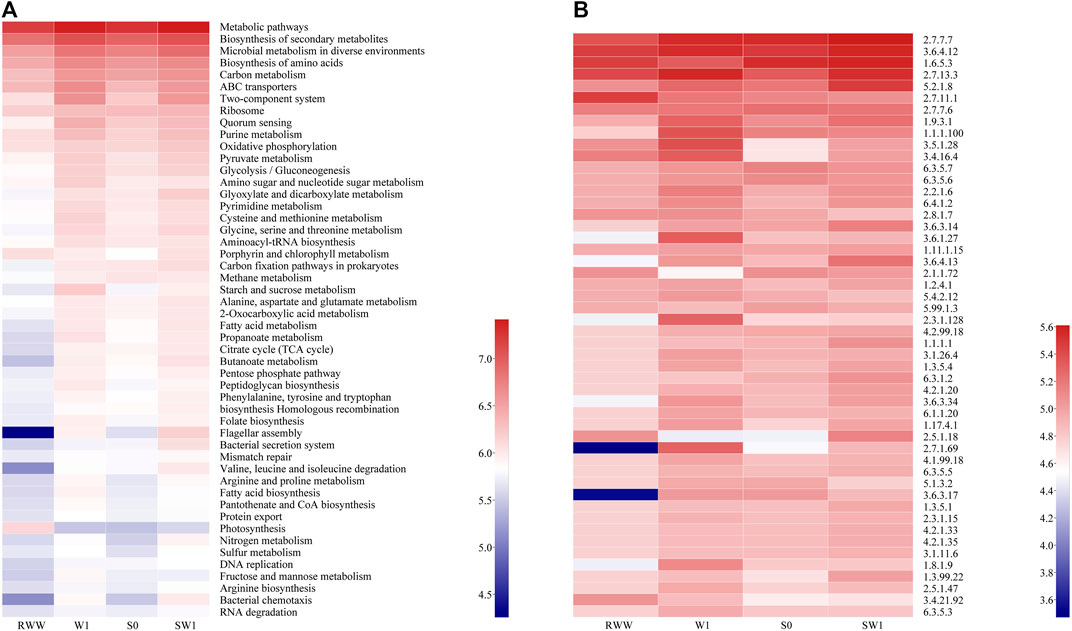
FIGURE 5. Heatmap of pathway level 3 (A) and enzyme (B) through PICRUSt 2 analysis based on the KEGG database.
The interconnectedness and differences of initial WWR, S0, W1, and SW1 could be explained by Figure 5A. The functional abundance of starch and sucrose metabolism in W1 was significantly stronger than that in RWW, suggesting V alone can stimulate microbial activity to some extent (Ortiz-Bernad et al., 2004). It was also reported that the stimulation of V could lead to the improvement of microbial enzyme activity (Fei et al., 2022). The relative abundances of starch and sucrose metabolism were also enriched in SW1, possibly as a result of the hydrolysis of starch and other substances by microbes in the WWR. Amino sugar and nucleotide sugar metabolism, starch and sucrose metabolism, and fructose and mannose metabolism were also reported in the simultaneous removal of V(V) and U(VI) reactors (Liu et al., 2022). The changes of alanine, aspartate, and glutamate metabolism; 2-oxocarboxylic acid metabolism; fatty acid metabolism; propanoate metabolism; citrate cycle (TCA cycle);butanoate metabolism; pentose phosphate pathway; peptidoglycan biosynthesis; phenylalanine, tyrosine and tryptophan biosynthesis; homologous recombination; folate biosynthesis; flagellar assembly; and bacterial secretion system (increasing in W1 and SW1 compared with that in RWW and S0) helped prove that V and WWR promoted the microbial activities.
Enrichments of enzymes in samples were also observed, presumably contributing to V(V) bio-reduction (Figure 5B). The abundance of 2.7.13.3 histidine kinase was enhanced in SW1 compared with S0. Enzyme analysis may also explain the inhibition of V(V) bio-reduction to a certain extent. The abundance of 1.9.3.1 cytochrome-c oxidase existed in W1 and SW1, with its abundance higher in W1. Mitochondria or purified cytochrome c oxidase could oxidize the positive vanadyl and negative vanadite ions (Frederick, 1975). It was speculated that the higher abundance of 1.9.3.1 cytochrome-c oxidase may have led to a lower V(V) removal performance in W1. Immobilized metal ion affinity chromatography (IMAC) analysis revealed that 2.5.1.18 glutathione transferase had a high affinity with V(IV), Fe(III), and Cu(II) and had high selectivity for V(IV); in other words, the metal-binding ability of the enzyme might play a role in metal homeostasis and detoxification (Yoshinaga et al., 2007), which created conditions for the stable existence of V(IV) (Figure 2F) by its high abundance in SW1 (Figure 5B).
To summarize, WWR could act as both microbial and carbon sources, contributing to V(V) bio-reduction. Results from this study could provide a theoretical reference for practical WWR and V(V) wastewater co-treatment. In the future, more advanced techniques can be used to analyze the mechanism of V(V) bio-detoxification in the system.
4 Conclusion
WWR was proved as a promising microbial and carbon source for V(V) bio-detoxification. Here, 0.445% V(V)/mL WWR was realized with 100 mL WWR dosage (group D). Solid particles in the system were utilized, and microbes were attached to the surface. In tests with inoculated sludge, V(V) removal efficiency was up to 95.5% within 5 days, confirming the enhanced V(V) bio-reduction performance. Functional genes based on the PICRUSt 2 analysis indicated ABC transporters and two-component system played vital roles in V(V) bio-reduction, while sulfur metabolism and nitrogen metabolism might also contribute to it. Further research on the co-treatment of V-contaminated wastewater with WWR can be on the agenda, providing the economical and environmentally friendly co-treatment bioremediation for WWR and V(V) polluted wastewater.
Data availability statement
The original contributions presented in the study are included in the article/Supplementary Material; further inquiries can be directed to the corresponding authors.
Author contributions
LH: conceptualization, methodology, formal analysis, investigation, writing—original draft, writing—review and editing, and funding acquisition. XW: formal analysis, investigation, data curation, writing—original draft, and writing—review and editing. JS: formal analysis, resources, and investigation. LL: validation and writing—original draft. XH: conceptualization, resources, writing—review and editing, supervision, and funding acquisition.
Funding
This research work was supported by the National Natural Science Foundation of China (NSFC) (No. 51908021), the R&D Program of Beijing Municipal Education Commission (No. KM202210016006), BUCEA Pyramid Talent Training Project (No. JDYC20220816), the BUCEA Scientific Research Ability Improvement Program for Young Teachers (No. X21017), and the BUCEA Post Graduate Innovation Project (No. PG2022068).
Conflict of interest
The authors declare that the research was conducted in the absence of any commercial or financial relationships that could be construed as a potential conflict of interest.
Publisher’s note
All claims expressed in this article are solely those of the authors and do not necessarily represent those of their affiliated organizations, or those of the publisher, the editors, and the reviewers. Any product that may be evaluated in this article, or claim that may be made by its manufacturer, is not guaranteed or endorsed by the publisher.
Supplementary material
The Supplementary Material for this article can be found online at: https://www.frontiersin.org/articles/10.3389/fenvs.2023.1096845/full#supplementary-material
References
Baghoth, S. A., Sharma, S. K., and Amy, G. L. (2011). Tracking natural organic matter (NOM) in a drinking water treatment plant using fluorescence excitation-emission matrices and PARAFAC. Water Res. 45, 797–809. doi:10.1016/j.watres.2010.09.005
Birgitta, B., Vesa, K., Timo, R., Samrit, L., and Virpi, K. (2007). Arsenic removal from groundwater and surface water-Field tests in the Pirkanmaa Region. Finland: RAMAS. Available at: http://projects.gtk.fi/export/sites/projects/ramas/reports/Remediationtests.pdf
Chen, J., Lu, J., Chen, S., Wang, J., and Zhang, B. (2022). Synchronous bio-reduction of uranium(VI) and vanadium(V) in aquifer: Performance and mechanisms. Chemosphere 288, 132539. doi:10.1016/j.chemosphere.2021.132539
Choudhary, S., and Sar, P. (2009). Characterization of a metal resistant Pseudomonas sp. isolated from uranium mine for its potential in heavy metal (Ni2+, Co2+, Cu2+, and Cd2+) sequestration. Bioresour. Technol. 100 (9), 2482–2492. doi:10.1016/j.biortech.2008.12.015
Deng, Q., Tian, Y., Ding, P., Yue, J., Zeng, X., Yin, Y., et al. (2020). Porous lamellar carbon assembled from Bacillus mycoides as high-performance electrode materials for vanadium redox flow batteries. J. Power Sources 450, 227633. doi:10.1016/j.jpowsour.2019.227633
Dogan, N. M., Kantar, C., Gulcan, S., Dodge, C. J., Yilmaz, B. C., and Mazmanci, M. A. (2011). Chromium(VI) bioremoval by Pseudomonas bacteria: Role of microbial exudates for natural attenuation and biotreatment of Cr(VI) contamination. Environ. Sci. Technol. 45, 2278–2285. doi:10.1021/es102095t
Fei, Y., Zhang, B., He, J., Chen, C., and Liu, H. (2022). Dynamics of vertical vanadium migration in soil and interactions with indigenous microorganisms adjacent to tailing reservoir. J. Hazard. Mater. J. 424, 127608. doi:10.1016/j.jhazmat.2021.127608
Frederick, C. (1975). Oxidation of vanadium IV by cytochrome c oxidase: Evidence for a terminal copper pathway. Biochem. Bioph. Res. Co. 63 (1), 355–361. doi:10.1016/S0006-291X(75)80051-9
Hao, L., He, Y., Shi, C., and Hao, X. (2021a). Biologically removing vanadium(V) from groundwater by agricultural biomass. J. Environ. Manage. 296, 113244. doi:10.1016/j.jenvman.2021.113244
Hao, L., He, Y., Shi, C., and Hao, X. (2021b). Performance and mechanisms for V(V) bio-reduction by straw: Key influencing factors. RSC Adv. 11, 27246–27256. doi:10.1039/d1ra03201a
Hao, L., Liu, Y., Chen, N., Hao, X., Zhang, B., and Feng, C. (2021c). Microbial removal of vanadium (V) from groundwater by sawdust used as a sole carbon source. Sci. Total Environ. 751, 142161. doi:10.1016/j.scitotenv.2020.142161
Hao, L., Zhang, B., Cheng, M., and Feng, C. (2016). Effects of various organic carbon sources on simultaneous V(V) reduction and bioelectricity generation in single chamber microbial fuel cells. Bioresour. Technol. 201, 105–110. doi:10.1016/j.biortech.2015.11.060
Hao, L., Zhang, B., Tian, C., Liu, Y., Shi, C., Cheng, M., et al. (2015). Enhanced microbial reduction of vanadium (V) in groundwater with bioelectricity from microbial fuel cells. J. Power Sources 287, 43–49. doi:10.1016/j.jpowsour.2015.04.045
Hashmi, I., Bindschedler, S., and Junier, P. (2020). Firmicutes. Benef. Microbes Agro-Ecology 18, 363–396. doi:10.1016/b978-0-12-823414-3.00018-6
He, Q., Feng, C., Chen, N., Zhang, D., Hou, T., Dai, J., et al. (2019). Characterizations of dissolved organic matter and bacterial community structures in rice washing drainage (RWD)-based synthetic groundwater denitrification. Chemosphere 215, 142–152. doi:10.1016/j.chemosphere.2018.10.026
He, Q., Feng, C., Hu, Q., Li, R., and Chen, N. (2016a). Biological denitrification using rice washing drainage (RWD) as carbon source for removing nitrate from groundwater. Desalin. Water Treat. 57, 21990–21999. doi:10.1080/19443994.2015.1127780
He, Q., Feng, C., Peng, T., Chen, N., Hu, Q., and Hao, C. (2016b). Denitrification of synthetic nitrate-contaminated groundwater combined with rice washing drainage treatment. Ecol. Eng. 95, 152–159. doi:10.1016/j.ecoleng.2016.06.043
Hou, D., Zhang, P., Zhang, J., Zhou, Y., Yang, Y., Mao, Q., et al. (2019). Spatial variation of sediment bacterial community in an acid mine drainage contaminated area and surrounding river basin. J. Environ. Manage. 251, 109542. doi:10.1016/j.jenvman.2019.109542
Huang, D., Fang, Q., Ji, S., Huang, Z., Zeng, Y., and Xiao, Y. (2019). Synergistic effects of acid production by combined fermentation of peanut meal and rice washing water. Chin. J. Environ. Eng. 13, 465–473. doi:10.12030/j.cjee.201808077
Huang, Z., Fang, Q., He, S., and Ji, S. (2019). Change regulation of quantitative relationship retween fermentation characteristic and VFAs production of rice wash. Industrial Saf. Environ. Prot. 45 (01), 5–11+54.
Hugenholtz, P. (2002). Exploring prokaryotic diversity in the genomic era. Genome Biol. 3, REVIEWS0003–8. doi:10.1186/gb-2002-3-2-reviews0003
Jiang, J., Ma, Z., and Zhang, H. (2004). Application of UASB reactor and contact oxidation method to the treatment of washing water of rice. Ind. Water Treat. 08, 75–77.
Kulasooriya, S. A., and Magana-Arachchi, D. N. (2016). Nitrogen fixing cyanobacteria: Their diversity, ecology and utilization with special reference to rice cultivation. J. Natl. Sci. Found. Sri Lanka 44, 111–128. doi:10.4038/jnsfsr.v44i2.7992
Lai, C., Dong, Q., Chen, J., Zhu, Q., Yang, X., Chen, W., et al. (2018). Role of extracellular polymeric substances in a methane based membrane biofilm reactor reducing vanadate. Environ. Sci. Technol. 52, 10680–10688. doi:10.1021/acs.est.8b02374
Lee, K. H., Park, S. J., Choi, S. J., Uh, Y., Park, J. Y., and Han, K. H. (2017). The influence of urinary catheter materials on forming biofilms of microorganisms. J. Bacteriol. Virol. 47, 32–40. doi:10.4167/jbv.2017.47.1.32
Li, Y., Wang, H., Wu, P., Yu, L., Rehman, S., Wang, J., et al. (2020). Bioreduction of hexavalent chromium on goethite in the presence of Pseudomonas aeruginosa. Environ. Pollut. 265, 114765. doi:10.1016/j.envpol.2020.114765
Liu, H., Chen, S., Lu, J., Li, Q., Li, J., and Zhang, B. (2022). Pentavalent vanadium and hexavalent uranium removal from groundwater by woodchip-sulfur based mixotrophic biotechnology. Chem. Eng. J. 437, 135313. doi:10.1016/j.cej.2022.135313
Liu, H., Zhang, B., Xing, Y., and Hao, L. (2016). Behavior of dissolved organic carbon sources on the microbial reduction and precipitation of vanadium(v) in groundwater. RSC Adv. 6, 97253–97258. doi:10.1039/c6ra19720e
Liu, J., Yao, J., Zhu, X., Zhou, D., Duran, R., Mihucz, V. G., et al. (2021). Metagenomic exploration of multi-resistance genes linked to microbial attributes in active nonferrous metal(loid) tailings. Environ. Pollut. 273, 115667. doi:10.1016/j.envpol.2020.115667
Liu Y., Y., Liu, S., Yang, Z., and Xiao, L. (2021). Synergetic effects of biochars and denitrifier on nitrate removal. Bioresour. Technol. 335, 125245. doi:10.1016/j.biortech.2021.125245
Lyalkova, N. N., and Yurkova, N. A. (1992). Role of microorganisms in vanadium concentration and dispersion. Geomicrobiol. J. 10, 15–26. doi:10.1080/01490459209377901
Nabayi, A., Sung, C. T. B., Zuan, A. T. K., and Paing, T. N. (2021). Fermentation of washed rice water increases beneficial plant bacterial population and nutrient concentrations. Sustain 13, 13437. doi:10.3390/su132313437
Ngwa, H. A., Kanthasamy, A., Jin, H., Anantharam, V., and Anumantha, G. (2014). Vanadium exposure induces olfactory dysfunction in an animal model of metal neurotoxicity. Neurotoxicology 43, 73–81. doi:10.1016/j.neuro.2013.12.004
Noël, V., Boye, K., Kukkadapu, R. K., Bone, S., Lezama Pacheco, J. S., Cardarelli, E., et al. (2017). Understanding controls on redox processes in floodplain sediments of the Upper Colorado River Basin. Sci. Total Environ. 603-604, 663–675. doi:10.1016/j.scitotenv.2017.01.109
Noisuwan, A., Hemar, Y., Wilkinson, B., and Bronlund, J. E. (2011). Adsorption of milk proteins onto rice starch granules. Carbohydr. Polym. 84, 247–254. doi:10.1016/j.carbpol.2010.11.029
Ono, M., Machida, I., Ikawa, R., Kamitani, T., Oyama, K., Muranaka, Y., et al. (2019). Regional groundwater flow system in a stratovolcano adjacent to a coastal area: A case study of Mt. Fuji and suruga Bay, Japan. Hydrogeol. J. 27, 717–730. doi:10.1007/s10040-018-1889-9
Ortiz-Bernad, I., Anderson, R. T., Vrionis, H. A., and Lovley, D. R. (2004). Vanadium respiration by Geobacter metallireducens: Novel strategy for in situ removal of vanadium from groundwater. Appl. Environ. Microbiol. 70, 3091–3095. doi:10.1128/AEM.70.5.3091-3095.2004
Patureau, D., Bernet, N., and Moletta, R. (1996). Effect of oxygen on denitrification in continuous chemostat culture with Comamonas sp SGLY2. J. Ind. Microbiol. Biotechnol. 16, 124–128. doi:10.1007/bf01570072
Peng, Q., Yi, L., Zhou, L., and Peng, Q. (2009). Draft genome sequence of the vanadium-leaching bacterium Pseudomonas chlororaphis Strain L19. Genome announc. 6 (1), 009666-17. doi:10.1128/genomeA.00966-17
Pourang, N., Nikouyan, A., and Dennis, J. H. (2005). Trace element concentrations in fish, surficial sediments and water from northern part of the Persian Gulf. Environ. Monit. Assess. 109, 293–316. doi:10.1007/s10661-005-6287-9
Safonov, A., Tregubova, V., Ilin, V., Boldyrev, K., Zinicovscaia, I., Frontasyeva, M., et al. (2018). Comparative study of lanthanum, vanadium, and uranium bioremoval using different types of microorganisms. Water. Air. Soil Pollut. 229 (3), 821–912. doi:10.1007/s11270-018-3740-2
Sánchez-Baracaldo, P., and Cardona, T. (2020). On the origin of oxygenic photosynthesis and Cyanobacteria. New Phytol. 225, 1440–1446. doi:10.1111/nph.16249
Shah, A. T., Favaro, L., Alibardi, L., Cagnin, L., Sandon, A., Cossu, R., et al. (2016). Bacillus sp. strains to produce bio-hydrogen from the organic fraction of municipal solid waste. Appl. Energy 176, 116–124. doi:10.1016/j.apenergy.2016.05.054
Shi, J., Li, Z., Zhang, B., Li, L., and Sun, W. (2021). Synergy between pyridine anaerobic mineralization and vanadium (V) oxyanion bio-reduction for aquifer remediation. J. Hazard. Mater. 418, 126339. doi:10.1016/j.jhazmat.2021.126339
Singh, A. K., Singh, P. P., Tripathi, V., Verma, H., Singh, S. K., Srivastava, A. K., et al. (2018). Distribution of cyanobacteria and their interactions with pesticides in paddy field: A comprehensive review. J. Environ. Manage. 224, 361–375. doi:10.1016/j.jenvman.2018.07.039
Singh, H. N., Chakravarty, D., Rao, K. S., and Singh, A. K. (1993). Vanadium requirement for growth on N2 or nitrate as nitrogen source in a tungsten-resistant mutant of the cyanobacterium Nostoc muscorum. J. Basic Microbiol. 33, 201–205. doi:10.1002/jobm.3620330312
Wang, D., Zhang, X., Xie, Q., and Yu, Z. (2003). Rice washing water treatment by hydrolytic acidification + SBR process. J. Guilin Univ. Technol. 01, 76–78.
Wang, G., Zhang, B., Li, S., Yang, M., and Yin, C. (2017). Simultaneous microbial reduction of vanadium (V) and chromium (VI) by Shewanella loihica PV-4. Bioresour. Technol. 227, 353–358. doi:10.1016/j.biortech.2016.12.070
Wang, S., Zhang, B., Li, T., Li, Z., and Fu, J. (2020). Soil vanadium(V)-reducing related bacteria drive community response to vanadium pollution from a smelting plant over multiple gradients. Environ. Int. 138, 105630. doi:10.1016/j.envint.2020.105630
Watanabe, M. (2009). Effect of enzymatic treatment for sedimentation and concentrate of solid particles in rice washing drainage and its electrostatic properties. J. Biosci. Bioeng. 108, S47. doi:10.1016/j.jbiosc.2009.08.135
Watanabe, M., Ichinose, K., Sasano, K., Ozaki, Y., Tsuiki, T., Hidaka, H., et al. (2011). Effect of enzymatic treatment on sedimentation and flocculation abilities of solid particles in rice washing drainage and its relationship with protein profiles. J. Biosci. Bioeng. 112, 67–70. doi:10.1016/j.jbiosc.2011.03.005
Wright, M. T., and Belitz, K. (2010). Factors controlling the regional distribution of vanadium in groundwater. Ground Water 48, 515–525. doi:10.1111/j.1745-6584.2009.00666.x
Xia, H., Wu, Y., Fu, C., and Hu, Q. (2020). Nitrogen removal characteristics of the coupling system of constructed wetland and microbial electrolysis cell. Chem. Industry Eng. Prog. 39 (11), 4677–4684. doi:10.16085/j.issn.1000-6613.2020-0071
Yang, X., Wang, S., and Zhou, L. (2012). Effect of carbon source, C/N ratio, nitrate and dissolved oxygen concentration on nitrite and ammonium production from denitrification process by Pseudomonas stutzeri D6. Bioresour. Technol. 104, 65–72. doi:10.1016/j.biortech.2011.10.026
Yelton, A. P., Williams, K. H., Fournelle, J., Wrighton, K. C., Handley, K. M., and Banfield, J. F. (2013). Vanadate and acetate biostimulation of contaminated sediments decreases diversity, selects for specific taxa, and decreases aqueous V5+ concentration. Environ. Sci. Technol. 47, 6500–6509. doi:10.1021/es4006674
Yoshinaga, M., Ueki, T., and Michibata, H. (2007). Metal binding ability of glutathione transferases conserved between two animal species, the vanadium-rich ascidian Ascidia sydneiensis samea and the schistosome Schistosoma japonicum. Biochim. Biophys. Acta - Gen. Subj. 1770, 1413–1418. doi:10.1016/j.bbagen.2007.05.007
Zhang, B., Cheng, Y., Shi, J., Xing, X., Zhu, Y., Xu, N., et al. (2019a). Insights into interactions between vanadium (V) bio-reduction and pentachlorophenol dechlorination in synthetic groundwater. Chem. Eng. J. 375, 121965. doi:10.1016/j.cej.2019.121965
Zhang, B., Qiu, R., Lu, L., Chen, X., He, C., Lu, J., et al. (2018). Autotrophic vanadium(V) bioreduction in groundwater by elemental sulfur and zerovalent iron. Environ. Sci. Technol. 52, 7434–7442. doi:10.1021/acs.est.8b01317
Zhang, B., Wang, S., Diao, M., Fu, J., Xie, M., Shi, J., et al. (2019b). Microbial community responses to vanadium distributions in mining geological environments and bioremediation assessment. J. Geophys. Res. Biogeosciences 124, 601–615. doi:10.1029/2018JG004670
Zhang, H., Zhang, B., Wang, S., Chen, J., Jiang, B., and Xing, Y. (2020). Spatiotemporal vanadium distribution in soils with microbial community dynamics at vanadium smelting site. Environ. Pollut. 265, 114782. doi:10.1016/j.envpol.2020.114782
Zhong, Y., and Zhang, H. (2014). Impact of China's grain import on grain security in recent years. Agric. outlook 10 (04), 59–65.
Zhou, Z., Tran, P. Q., Kieft, K., and Anantharaman, K. (2020). Genome diversification in globally distributed novel marine Proteobacteria is linked to environmental adaptation. ISME J. 14, 2060–2077. doi:10.1038/s41396-020-0669-4
Keywords: vanadium, washing water of rice, reduction, microbial evolution, mechanisms
Citation: Hao L, Wang X, Shi J, Li L and Hao X (2023) Vanadium (V) bio-detoxification based on washing water of rice as microbial and carbon sources. Front. Environ. Sci. 11:1096845. doi: 10.3389/fenvs.2023.1096845
Received: 14 November 2022; Accepted: 06 January 2023;
Published: 20 January 2023.
Edited by:
Hongguo Zhang, Guangzhou University, ChinaReviewed by:
Rongkui Su, Central South University Forestry and Technology, ChinaLei Huang, Guangzhou University, China
Copyright © 2023 Hao, Wang, Shi, Li and Hao. This is an open-access article distributed under the terms of the Creative Commons Attribution License (CC BY). The use, distribution or reproduction in other forums is permitted, provided the original author(s) and the copyright owner(s) are credited and that the original publication in this journal is cited, in accordance with accepted academic practice. No use, distribution or reproduction is permitted which does not comply with these terms.
*Correspondence: Liting Hao, aGFvbGl0aW5nQGJ1Y2VhLmVkdS5jbg==; Xiaodi Hao, eGRoYW9AaG90bWFpbC5jb20=