- 1Shiley School of Engineering, University of Portland, Portland, OR, United States
- 2Department of Civil and Environmental Engineering, Colorado School of Mines, Golden, CO, United States
- 3Southern California Coastal Water Research Project, Costa Mesa, CA, United States
Contaminants of emerging concern such as pharmaceuticals, personal care products, per- and polyfluoroalkyl substances, and plasticizers, are ubiquitous in effluent-dominated rivers and have potential adverse effects on humans and aquatic life. Demands on water supply have prompted conservation and water reuse measures, impacting the discharge in these rivers, yet the effects of these management decisions on water quality are largely intuited and not quantified. This research examines how changes in water reuse practices will impact concentrations of contaminants of emerging concern, specifically carbamazepine, diclofenac, galaxolide, gemfibrozil, 4-nonylphenol, and perfluorooctane sulfonic acid (PFOS), in the effluent-dominated Los Angeles River (Los Angeles County, California). A water quality module was added to a calibrated hydrologic model of the system and parametrized with observed water quality monitoring data in EPA SWMM. Results indicate that water reuse (i.e., reduced effluent flow) will consistently improve in-stream water quality for all compounds studied except PFOS. However, the improvements are often not substantial enough to mitigate high concentrations directly downstream of treated effluent discharge points. Concentrations of these pharmaceuticals are substantially reduced through attenuation as dilution and degradation occur downstream, though the rate of this attenuation is variable and based on the contaminant. In contrast, concentrations of PFOS increase under some wastewater reuse scenarios and decrease under others but remain below the recommended environmental screening levels. Our work also highlights that management decisions regarding water quantity should integrate water quality modeling to help identify priority monitoring locations and constituents.
1 Introduction
Rivers are used as both sources of drinking water (and other beneficial uses) as well as places to discharge treated wastewater. Many rivers near highly populated areas are effluent-dominated, where most river discharge is due to treated wastewater effluent, particularly during dry weather (Brooks et al., 2006). The water quality of this effluent can then dictate the water quality in the river (Wolfand et al., 2022a). Treated wastewater effluent can be of greater water quality than surface waters, as it is typically low in solids and metals (Wolfand et al., 2022a). However, it can also be a source of other pollutants such as nitrogen and contaminants of emerging concern (CECs), such as pharmaceuticals, personal care products, and perfluorinated alkylated substances (Brooks et al., 2006).
CECs, also called trace organic contaminants or micropollutants, are often not regulated yet pose a major risk to ecosystems, aquatic organisms, and human health (Brooks et al., 2006; Patel et al., 2019). For example, at the parts per trillion level, endocrine-disrupting pharmaceuticals cause adverse effects to fish (Corcoran et al., 2010), and perfluorinated alkylated substances, such as perfluorooctane sulfonic acid (PFOS), are toxic to humans (U.S. Environmental Protection Agency, 2022). Compared to conventional pollutants, CECs pose a unique risk to aquatic life and human health because they are broad in chemical characteristics, difficult and expensive to monitor, and may pose increased toxicity in mixture (Schwarzenbach et al., 2006; Geissen et al., 2015; Mutzner et al., 2022). Both monitoring and modeling have been used, often in conjunction, to develop risk assessments related to CEC exposure or predict the impacts of management decisions (Johnson et al., 2008). Due to the complexity, there is no standard for modeling CECs in river systems (Keller, 2015). Various process-based and empirical water quality models such as EPA SWMM (Park et al., 2007; Jackson et al., 2011; Dittmer et al., 2020), SWAT (Wang et al., 2019), QUAL2K (Zhi et al., 2022), GREAT-ER, (Koormann et al., 2006; Sumpter et al., 2006; Lämmchen et al., 2021a; Lämmchen et al., 2021b), and WASP (Agustin et al., 2023), have been modified or developed to simulate the fate and transport of CECs (Sharma and Kansal, 2013). Others, more recently, have applied various machine learning approaches to simulate concentrations and loads of CECs in rivers (Yun et al., 2021).
Treated wastewater is a pathway for CECs to enter surface waters (Roberts and Thomas, 2006) as many of these pollutants are not fully removed during the conventional wastewater treatment process (Kim et al., 2003). Monitoring studies have shown that these contaminants are present in effluent-dominated rivers (Mandaric et al., 2018), though they are often transformed and attenuated downstream (Gross et al., 2004; Wiegel et al., 2004; Moldovan, 2006). However, the impact of water reuse practices on CEC concentrations in-river is generally unknown.
There is increasing motivation (from the public and regulators) for enhanced water efficiency at the local level. One approach to improving water efficiency is encouraging water reuse and water conservation, especially for outdoor uses. Water reuse practices are increasing globally, especially in the United States, but also in Australia, Belgium, and Singapore (WateReuse Association, 2023), where wastewater is treated centrally for reuse for either irrigation or public drinking water supply (Council, 2012; Ghernaout et al., 2019; Luthy et al., 2020; Olivieri et al., 2020; Radcliffe et al., 2020; Jeffrey et al., 2022). Domestic, commercial, and industrial water conservation practices reduce the amount of raw wastewater flows to wastewater treatment plants (Chappelle et al., 2019). Both conservation measures and water reuse will likely decrease the contribution of treated effluent to flows in urban rivers.
As water conservation and reuse become increasingly efficient, chemical characteristics and quantity of treated wastewater discharges may be drastically altered, impacting both the flow and the water quality in receiving water bodies. The purpose of this study is to quantify the effects of wastewater reuse on in-river concentrations of CECs, using the Los Angeles River as a case study. We use a calibrated hydrologic model created in EPA SWMM to investigate how proposed reductions in treated wastewater flow may impact concentrations of pollutants within the river.
2 Methods
2.1 Study site
The Los Angeles River is an effluent-dominated river located in Los Angeles County, California (Figure 1). The contributing watershed is highly developed, with approximately 32% impervious cover. The primary land use is residential, though there are pockets of industrial and commercial use surrounding the downstream reaches, as well as undeveloped open space surrounding upstream tributaries (Wolfand et al., 2022b). There are eight major flood control dams located along tributaries to the Los Angeles River mainstem. Most of the Los Angeles River mainstem and many of its tributaries are armored with concrete for flood control purposes (Los Angeles County and Los Angeles County Public Works, 2020), but the river also provides recreation and habitat, particularly in the soft-bottom portions of the river such as Sepulveda Basin (Figure 1; approx. from LA20 to LA20_2) and Glendale Narrows (Figure 1; approx. from Glendale WRP to F57C). (Los Angeles County and Los Angeles County Public Works, 2020)
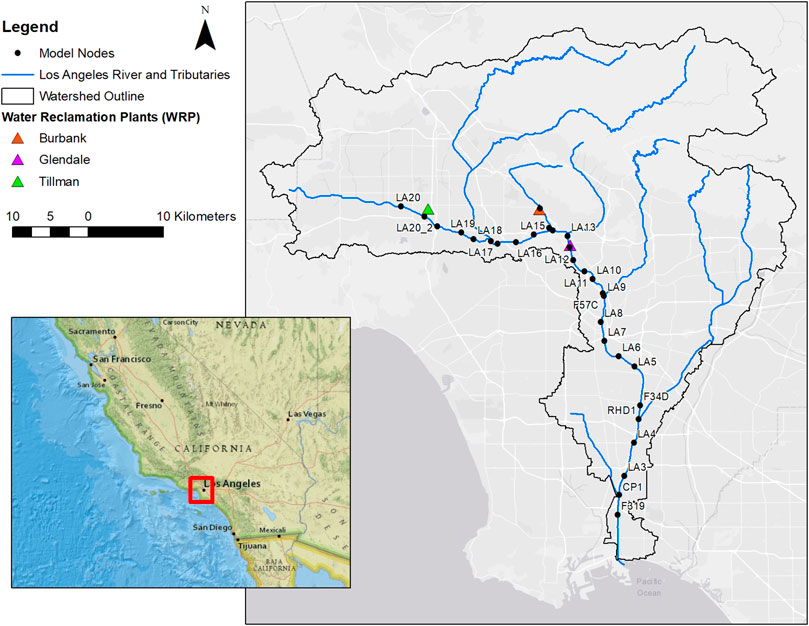
FIGURE 1. Map of the Los Angeles River watershed and model nodes. The model was calibrated at node LA12, immediately downstream of all three water reclamation plants.
Three water reclamation plants (WRPs) are located within the Los Angeles River watershed: Donald C. Tillman (Tillman) and Glendale, which are located on the mainstem, and Burbank, which discharges to the tributary Burbank Channel. The climate is semi-arid Mediterranean with spatially variable rainfall of 200–460 mm (8–18 in.) annually (Wolfand et al., 2022b). The drinking water supply is primarily from imported water and local groundwater supplies (Los Angeles Department of Water and Power, 2020). The availability and reliability of these sources are expected to be strained by climate change and continuing population growth in the region, so there are regional plans to diversify water supply portfolios, especially by increasing recycled water (Los Angeles Department of Water and Power, 2020). On average, Tillman, Glendale, and Burbank WRPs typically discharge approximately 46 MGD (2.0 m3/s) of treated wastewater to the river (for water years 2011–2017), but they have plans to collectively reuse approximately 20% (California State Water Resources Control Board, 2017b; California State Water Resources Control Board, 2017a; City of Los Angeles Bureau of Sanitation, 2021). The flow, ecology, water quality, and temperature implications of these changes have been predicted by various other studies (Abdi et al., 2021; Stein et al., 2021a; Stein et al., 2021b; Abdi et al., 2022; Wolfand et al., 2022a; Wolfand et al., 2022b). Notably, previous work has found that indicator species may experience habitat degradation due to just a 4% decrease in wastewater discharge during the dry season (Wolfand et al., 2022b).
CECs have been previously monitored in treated effluent, freshwater, and sediments in the Los Angeles River basin (City of Los Angeles Bureau of Sanitation, 2014; Southern California Coastal Water Research Project, 2017). An expert panel in the state established monitoring trigger levels (MTLs) for CECs that pose the greatest risk to aquatic systems (Anderson et al., 2012). Six emerging CECs were chosen based on historical monitoring data: carbamazepine, diclofenac, galaxolide, gemfibrozil, 4-nonylphenol, and perfluorooctane sulfonic acid (PFOS) (Table 1). Diclofenac, galaxolide, and 4-nonylphenol are consistently present at higher concentrations at stations downstream of WRPs in the Los Angeles River Basin. Carbamazepine, gemfibrozil, and PFOS are also observed in the treated effluent (City of Los Angeles Bureau of Sanitation, 2014). The observation of these six pollutants in the Los Angeles River is consistent with other studies that show that many of these compounds are not fully removed in current wastewater removal processes (Kim et al., 2018).
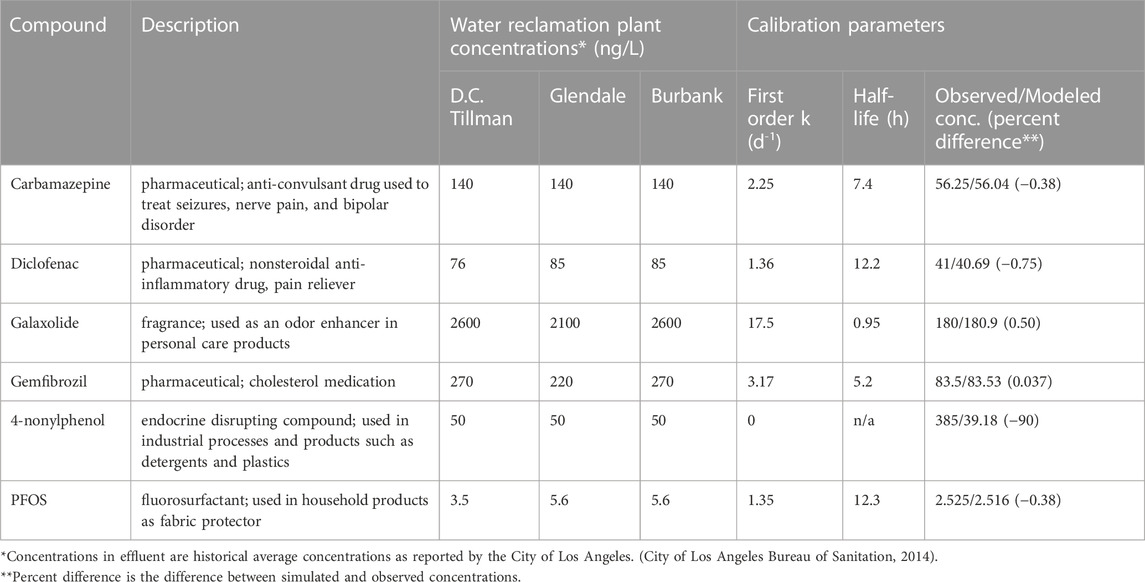
TABLE 1. Compounds of interest, average concentrations in wastewater effluent, and calibration parameters.
2.2 Water quality model
2.2.1 Model design
An hourly hydrologic model was created in EPA’s Storm Water Management Model (SWMM), for the Los Angeles River. Detailed documentation on the hydrologic model development, calibration, and validation is provided in Wolfand et al., 2022b. Briefly, the basin was discretized into 77 catchments, and precipitation data was retrieved from 72 gages in Los Angeles County and spatially interpolated for each catchment using kriging methods. The model was run for water years 2011–2017. This time range was chosen due to 1) the availability of high-resolution hydrologic and climate data, and 2) relatively constant wastewater discharge during this period before major water reuse practices were implemented. Observed time series data, such as WRP discharges, dam discharges, and dry weather baseflows were included in the model. The model was calibrated and validated at 7 observed gage stations (4 on the mainstem, 3 on tributaries), with Nash Sutcliffe Efficiency (NSE) between 0.67 and 0.94 and percent bias between −20% and 17.3%.
For this study, channel geometry within the SWMM model was updated based on a previously developed HEC-RAS hydraulic model of the system (Stein et al., 2021b). The model was then parameterized to include CECs of interest: carbamazepine, diclofenac, galaxolide, gemfibrozil, 4-nonylphenol, and PFOS. There are three primary inputs of CECs in the river: stormwater runoff, dry-weather runoff from stormdrains (from activities such as car-washing and irrigation return flows), and WRP effluent. The analysis focused on flows during May and June, so that contribution of stormwater runoff to river discharge was negligible; during these months, there is typically no precipitation due to seasonal rainfall patterns.
Dry-weather runoff from stormdrains, which contributes approximately 20%–50% of the river discharge (Wolfand et al., 2022b), was not explicitly modeled due to lack of available data; instead, the contribution of this source to CEC concentrations was captured by parameterizing river water concentrations (baseflows). River water concentrations of the selected pollutants were included in the model upstream of the three WRPs at LA20 based on historical monitoring data. However, only PFOS was detected at an initial concentration of 6.85 ng/L.
WRP effluent concentrations for each CEC were included in the model based on monitoring grab samples taken in June 2014 (Table 1) (City of Los Angeles Bureau of Sanitation, 2014). 4-nonylphenol was not detected in WRP effluent, due to challenges in low-level quantification, so it was assumed concentrations were equal to the method detection limit (MDL), 50 ng/L. No effluent monitoring data was provided for Burbank WRP, so it was assumed that concentrations were the highest average value of those calculated for Tillman or Glendale WRPs.
2.2.2 Calibration
First-order decay was used to approximate the natural decay of these compounds due to photolysis, biodegradation, sorption, and other environmental conditions (Lin et al., 2006). The first-order decay constant for each compound was manually calibrated to minimize the percent difference between simulated and observed concentrations downstream of the last wastewater discharge point, node LA12 (Figure 1; Table 1). Simulated concentrations were the monthly median concentrations from the model for May and June, while observed concentrations were grab samples taken within the river below Glendale WRP in May and June 2018 (Maruya et al., 2022).
2.2.3 Scenarios
Seven WRP reuse scenarios were assessed: 0%, 10%, 25%, 50%, 75%, 90%, and 100% reuse, where 100% reuse means all effluent is treated and directed to other uses and is not directly discharged to the river. Flows and concentrations were evaluated at each node along the river mainstem. Outputs are reported as the median value for May and June. To distinguish between the effects of dilution and degradation, an additional scenario of 0% reuse (baseline conditions) that did not include chemical degradation was simulated. Note baseline conditions are defined by the simulation period (WY 2011–2017), during which time wastewater reuse was minimal. Simulated concentrations were compared to monitoring trigger levels for diclofenac (100 ng/L) and galaxolide (700 ng/L) determined by a Science Advisory Panel convened by the California State Water Resources Control Board (Anderson et al., 2012). Regulatory standards for PFOS are developing globally. The U.S. EPA released draft aquatic life ambient water quality criteria for PFOS in April 2022 of 3.0 mg/L for acute exposure (1-h average) and 0.0084 mg/L for chronic exposure (4-day average) in freshwater (EPA, 2022). As of 2020, California has an Interim Final Environmental Screening Level of 0. 56 μg/L (560 ng/L) for groundwater for direct exposure to freshwater aquatic species (California Water Boards, 2023). EPA’s non-enforceable drinking water advisory level for PFOS is 70 ng/L. (U.S. Environmental Protection Agency, 2022)
3 Results
3.1 Calibration
The percent bias of simulated river concentrations was minimized to <1% for all compounds except for 4-nonylphenol (Table 1). Concentrations for 4-nonylphenol are much higher in the river than can be explained by discharge from WRP effluent alone, and thus calibration was unsuccessful (percent bias: 90%; Table 1). We have several hypotheses for why calibration may have been unsuccessful: 1) there is limited observational data, which may not have fully captured river concentrations; 2) there are permitted industrial dischargers to the river, which were not captured by the model; and 3) the presence of this pollutant in bed sediment could be an additional flux to freshwater; 4-nonylphenol has been observed in estuarine sediments collected in other parts of California (Anderson et al., 2012). Model results for 4-nonylphenol were therefore not reported. Calibrated half-lives for other compounds are within the observed ranges of experimental half-lives. For example, the calibrated half-life of carbamazepine was 7.4 h, which is similar to the half-life range of 8–12 h found in solar simulator experiments in river water (Matamoros et al., 2009). The calibrated half-life of diclofenac was 12.2 h, which falls within the reported range of 10–21 h found in field observations from lakes in Sweden (Bu et al., 2016).
3.2 Scenario analysis
3.2.1 Existing conditions
Existing flows are substantially augmented by WRP discharge, particularly in May and June when precipitation and stormwater runoff are limited (Supplementary Figure S1). (Wolfand et al., 2022b) Under baseline conditions (0% wastewater reuse), concentrations of CECs typically increase immediately downstream of the wastewater discharge point. For example, galaxolide concentrations increase from approximately 0 ng/L to 1,994 ng/L in the river after the last discharge point from Tillman (Figure 2). Note that Tillman WRP has multiple discharge points between node LA20 and Sepulveda Dam (USGS gage # 11092450; Supplementary Figure S9). The same pattern holds for pharmaceuticals carbamazepine, diclofenac, and gemfibrozil (Supplementary Figures S2–S4). However, concentrations of PFOS in the river decrease downstream of WRPs, due to the relatively low concentration of PFOS in WRP effluent compared to background concentrations. Because of this, WRP discharge serves to dilute PFOS concentrations immediately downstream of all three WRPs.
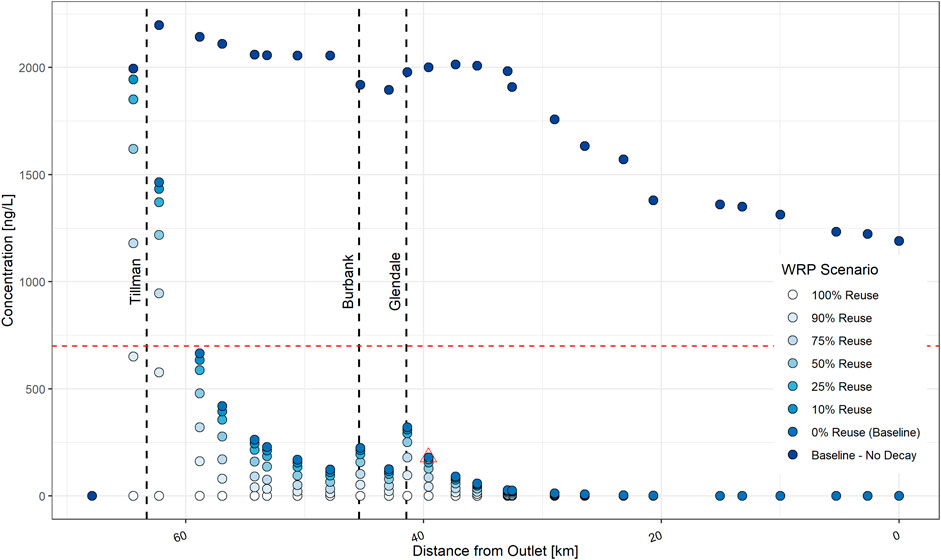
FIGURE 2. Simulated galaxolide concentrations (median across May and June) in the Los Angeles River under various water reclamation plant (WRP) scenarios. The locations of the three WRPs along the river are marked with vertical dashed lines. The horizontal dashed line indicates the monitoring trigger level for galaxolide of 700 ng/L. The red triangle indicates observed monitoring data.
Simulated concentrations of CECs are attenuated along the river as flows increase. The rate of decrease is due to both dilution and degradation of the compounds, described by first-order decay in the model. Under existing conditions, at the outlet of the watershed (node F319), simulated concentrations of carbamazepine are reduced to 6.1 ng/L, diclofenac is reduced to 8.4 ng/L, and gemfibrozil is reduced to 4.8 ng/L (Supplementary Figures S2–S4). The simulated concentration of PFOS is 0.5 ng/L and galaxolide is 0.02 ng/L at the watershed outlet (Figures 2, 3). A monitoring study in the nearby Santa Ana River also found that CECs were substantially attenuated downstream of wastewater discharge points (Gross et al., 2004).
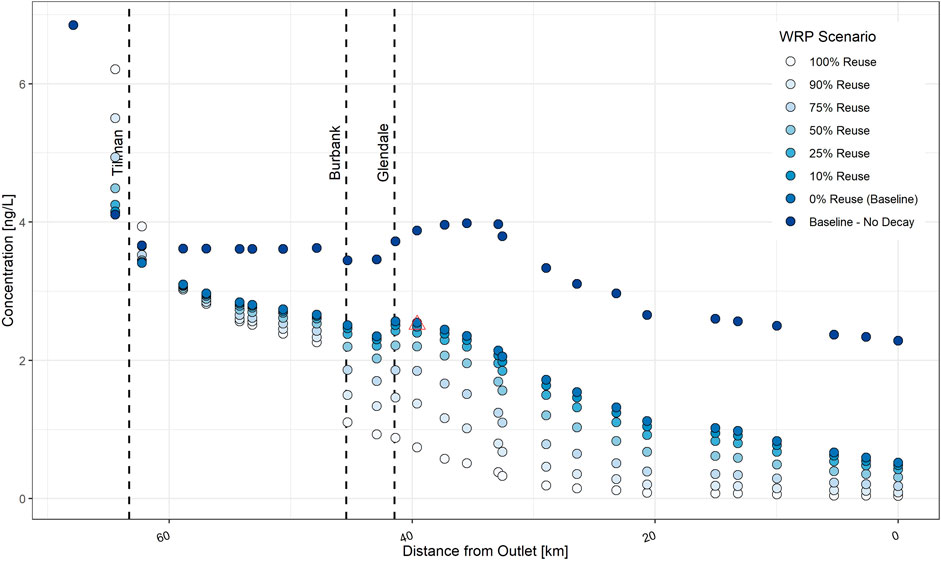
FIGURE 3. Simulated PFOS concentrations (median across May and June) in the Los Angeles River under various water reclamation plant (WRP) scenarios. The locations of the three WRPs along the river are marked with vertical dashed lines. The red triangle indicates observed monitoring data. The U.S. EPA drinking water advisory level for PFOS is 70 ng/L (beyond the y-axis scale).
3.2.2 Water reuse scenarios
Simulated concentrations of CECs in the Los Angeles River decrease with increased water reuse for all compounds except PFOS. WRP discharge contains most of these CECs, so increased reuse results in lower concentrations in the river, due to dilution by dry-weather baseflow (primarily from dry-weather runoff). For example, concentrations of galaxolide downstream of Tillman WRP at node LA20_2 decreases from 1,994 ng/L to 1,620 ng/L with 50% reuse (Figure 2). The same is true for pharmaceuticals carbamazepine, diclofenac, and gemfibrozil (Supplementary Figures S2–S4).
In the case of PFOS, the concentration in treated effluent from Tillman WRP (3.5 ng/L) is less than the background levels of PFOS in the river, so the treated effluent helps dilute the concentration in the river. Simulated WRP discharge from Burbank and Glendale have higher concentrations of PFOS (5.6 ng/L) than the Los Angeles River water, so when chemical degradation is not considered, these WRPs increase concentrations of PFOS in the river (Figure 3; “Baseline–No Decay”). When both dilution and chemical degradation are considered, PFOS concentrations either increase or decrease depending on the WRP reuse scenario and the location in the river (Figure 3). All simulated concentrations of PFOS fall well below the environmental screening level of 560 ng/L and the EPA drinking water advisory level of 70 ng/L, with a maximum simulated in-river concentration of less than 7 ng/L across all scenarios (Figure 3). However, simulated concentrations likely underestimate PFOS because only background in-river concentrations and WRP effluent were parametrized as sources of PFOS in the model. PFOS has been observed in wet weather runoff (stormwater) (Houtz and Sedlak, 2012; Xiao et al., 2012), and dry weather runoff likely contains PFOS, though there was no monitoring data for this potential source. Dry weather runoff should be targeted for future monitoring efforts.
The rate of attenuation is important when considering the potential impacts of these compounds on aquatic species. Under existing conditions, the recommended monitoring trigger level of 700 ng/L for galaxolide is exceeded at some locations directly downstream of the Tillman WRP and Burbank WRP (Figure 2; Supplementary Figure S9). Simulated median concentrations fall below the monitoring trigger level at approximately 3.5 km downstream of Tillman (Figure 2) and at the confluence of Burbank Channel with the mainstem of the Los Angeles River (Supplementary Figure S9). Wastewater reuse will decrease in-stream concentrations of galaxolide, below the monitoring trigger levels, especially when reuse is upwards of 90%. However, as mentioned previously, the proposed WRP reuse is likely only about 20%, at which point concentrations are diminished but only minimally (Figure 2). This suggests that there may be localized impacts of the WRP, regarding galaxolide, associated with currently planned wastewater reuse.
According to simulation results, the monitoring trigger level for diclofenac is not exceeded at any point along the river under baseline conditions or wastewater reuse scenarios (Supplementary Figures S3, S7). Concentrations of PFOS are also below EPA’s draft chronic aquatic exposure criteria (0.0084 mg/L) and drinking water advisory level (70 ng/L; Figure 3). Aquatic life criteria have not been established for the three other compounds (carbamazepine, gemfibrozil, and 4-nonylphenol).
4 Discussion
4.1 Significance and limitations
Water quality modeling of CECs can be especially powerful because it is often difficult to draw river-scale or watershed-scale conclusions with the limited availability of CEC monitoring data. This work provides a framework for future studies to estimate the impacts of management scenarios on downstream conditions without intensive monitoring. The developed approach uses industry-standard software (EPA SWMM) to predict concentrations of CECs across management scenarios. Few studies have applied EPA SWMM in this way (Park et al., 2007; Jackson et al., 2011; Dittmer et al., 2020). The model can be readily applied to multiple compounds and can be used as a screening tool to determine which compounds and/or locations should be prioritized for monitoring and/or intervention.
However, the limited availability of monitoring data results in models that are inherently limited in their scope and applicability. In this study, no data was available on concentrations of the pollutants of interest in sources other than wastewater such as industrial discharges, or dry weather runoff. Because the study was limited to the dry season (summer months), impacts from stormwater runoff are negligible. However, dry weather runoff, from activities such as irrigation, car washing, and industrial discharges, may contain the pollutants of interest, particularly PFOS, which is a household fluorosurfactant. The pharmaceuticals diclofenac, carbamazepine, and gemfibrozil are likely not present in dry weather runoff, except in the case of illicit discharges, which were not captured by the model. A study of the South Platte River (Denver, CO) reported monitoring data that showed that wastewater treatment plant discharge was not the primary factor controlling water quality, instead, industrial discharges and stormwater runoff contributed (Schliemann et al., 2021), both of which were not included in our model due to limited data. Even just one or two more monitoring grab samples, either spatially or temporally, could improve model calibration, and therefore the accuracy, of predictions. In addition, a more complete dataset would allow for the validation of the model.
An additional limitation of this study includes assuming a first-order decay of these compounds as a proxy for combined degradation processes in the environment. Photodegradation is likely a primary degradation pathway for these compounds in the shallow and wide Los Angeles River, but other processes are occurring including hydrolysis, oxidation, sorption, and biodegradation. The degradation rates were assumed to be constant, but degradation is variable based on factors such as sunlight, temperature, and pH.
4.2 Management implications
Los Angeles plans to recycle approximately 20% of existing wastewater, which will result in modest reductions in the CEC concentrations simulated in this study, except for PFOS, which may increase in concentration immediately downstream of Tillman WRP but remain below environmental screening levels. Assuming 20% wastewater reuse, galaxolide concentrations will still be above recommended monitoring thresholds, for kilometers downstream of Tillman WRP. Therefore, there are still potential adverse effects from CECs, particularly near WRP discharge points. This may be of particular concern because areas downstream of the WRP discharges are often the locations where wetland and riparian habitat, recreational boating, fishing, and wading occur due to the persistence of flows. Therefore, while the adverse effects may be concentrated in specific areas, the risk to humans and wildlife could be intensified, emphasizing the need for prioritized monitoring of these locations in the future.
In this work, we found that as water reuse increases, CEC concentrations typically decrease (except for PFOS). This contrasts with our previous work that shows concentrations of conventional pollutants total suspended solids, total dissolved solids, copper, and lead increase with increased water reuse (Wolfand et al., 2022a). Treated WRP effluent is an input of CECs to the river, whereas it serves as a mechanism for dilution for conventional pollutants such as solids and metals. Therefore, the impact on water quality in the Los Angeles basin as a result of water reuse is expected to vary depending on the specific pollutant, leading to both improvements and potential deterioration. In addition, water reuse will reduce flows in the river, also potentially having a negative impact on wetland and riparian habitats (Wolfand et al., 2022b). The implications of water reuse for river flow and water quality should be weighed against the benefits of increased local water supply.
While we only evaluated a subset of CECs, we know that there are an indeterminate number of unmonitored compounds and degradation products. Some degradation products are equally if not more toxic to aquatic life in the environment (Li et al., 2016). Our analysis shows that CEC concentrations generally improve with increased reuse, but the true ecological impacts of this are much more complex as toxicity can be compounded by CECs in mixture. As water reuse increases, particularly for potable reuse, the urban water cycle approaches a closed-loop system, particularly within one region or city. Advanced water purification processes can help ensure that CECs are fully removed from the urban water cycle.
Data availability statement
The original contributions presented in the study are included in the article/Supplementary Material, further inquiries can be directed to the corresponding author.
Author contributions
JW, ES, and TH designed the study. JW completed the water quality modeling and analysis. KT-Q and AS provided input on the model development and data analysis. All authors contributed to the article and approved the submitted version.
Funding
Primary funding was provided by the City of Los Angeles Bureau of Sanitation (BoS) and the Los Angeles Department of Water and Power (LADWP). Additional funding was provided by the Los Angeles County Department of Public Works (LADPW), Los Angeles County Sanitation Districts (LACSD), the Watershed Conservation Authority (WCA), a joint powers authority between the Rivers and Mountains Conservancy (RMC) and the Los Angeles County Flood Control District, and the Mountains Recreation and Conservation Authority (MRCA), a joint power of the Santa Monica Mountains Conservancy, the Conejo Recreation and Park District, and the Ranch Simi Recreation and Park District.
Acknowledgments
Thank you to Alvina Mehinto at the Southern California Coastal Water Research Project (SCCWRP) who assisted with the model conceptualization and provided monitoring data. This project was conducted through a collaboration with SCCWRP, the State Water Resources Control Board, the Los Angeles Regional Water Quality Control Board, and local municipalities and stakeholders. We thank all members of the Stakeholder Workgroup and the Technical Advisory Group who provided critical input, advice, and review throughout this project. Additional project information is available at https://www.waterboards.ca.gov/water_issues/programs/larflows.html and https://www.sccwrp.org/about/research-areas/ecohydrology/los-angeles-river-flows-project.
Conflict of interest
The authors declare that the research was conducted in the absence of any commercial or financial relationships that could be construed as a potential conflict of interest.
Publisher’s note
All claims expressed in this article are solely those of the authors and do not necessarily represent those of their affiliated organizations, or those of the publisher, the editors and the reviewers. Any product that may be evaluated in this article, or claim that may be made by its manufacturer, is not guaranteed or endorsed by the publisher.
Supplementary material
The Supplementary Material for this article can be found online at: https://www.frontiersin.org/articles/10.3389/fenvs.2023.1091229/full#supplementary-material
References
Abdi, R., Rogers, J. B., Rust, A., Wolfand, J. M., Philippus, D., Taniguchi-Quan, K., et al. (2021). Simulating the thermal impact of substrate temperature on ecological restoration in shallow urban rivers. J. Environ. Manage. 289 (112560), 112560–112610. doi:10.1016/j.jenvman.2021.112560
Abdi, R., Rust, A., Wolfand, J. M., Taniguchi-Quan, K., Irving, K., Philippus, D., et al. (2022). Thermal suitability of the Los Angeles river for Cold water resident and migrating fish under physical restoration alternatives. Front. Environ. Sci. 9, 1–14. doi:10.3389/fenvs.2021.749085
Agustin, R. G., Rollon, A. P., Kwan, C., and Ballesteros, F. C. (2023). Occurrence, attenuation and dynamic modeling of pesticides transport along pampanga river basins. Eur. Chem. Bull. 12 (4), 4477–4488. doi:10.48047/ecb/2023.12.si4.403
Anderson, P., Denslow, N., Olivieri, A., Schlenk, D., and Scott, G. I. (2012). Monitoring strategies for chemicals of emerging concern (CECs) in California’s aquatic ecosystems: final report and recommendations of a science advisory panel. Available at: http://www.waterboards.ca.gov/water_issues/programs/water_recycling_policy/docs/cec_ecosystems_rpt.pdf.
Brooks, B. W., Riley, T. M., and Taylor, R. D. (2006). Water quality of effluent-dominated ecosystems: ecotoxicological, hydrological, and management considerations. Hydrobiologia 556 (1), 365–379. doi:10.1007/s10750-004-0189-7
Bu, Q., Shi, X., Yu, G., Huang, J., and Wang, B. (2016). Assessing the persistence of pharmaceuticals in the aquatic environment: challenges and needs. Emerg. Contam. 2 (3), 145–147. doi:10.1016/j.emcon.2016.05.003
California Water Boards (2023). Transmittal of Interim final environmental screening levels (ESLs) for two per- and polyfluoroalkyl substances (PFAS): perfluorooctane sulfonate (PFOS) and perfluorooctanoate (PFOA). San Francisco: Regional Water Quality Board. Available at: https://www.waterboards.ca.gov/sanfranciscobay/water_issues/programs/esl.html.
California State Water Resources Control Board (2017a). Notice of wastewater change petition WW0091 and petition for change in place of use for order approving wastewater change petition WW0019. Sacramento: CA.
California State Water Resources Control Board (2017b). Notice of wastewater change petition WW0097. Sacramento: CA.
Chappelle, C., Mccann, H., Jassby, D., Schwabe, K., and Szeptycki, L. Managing wastewater in a changing climate; 2019.
City of Los Angeles Bureau of Sanitation (2014). D. C. Tillman water reclamation plant 2014 annual reporting of constituents of emerging concern. Los Angeles: CA.
City of Los Angeles Sanitation and Environment (2021). D. C. Tillman water reclamation plant: Japanese garden discharge reuse initial study/negative declaration. Los Angeles: CA.
Corcoran, J., Winter, M. J., and Tyler, C. R. (2010). Pharmaceuticals in the aquatic environment: a critical review of the evidence for health effects in fish. Crit. Rev. Toxicol. 40 (4), 287–304. doi:10.3109/10408440903373590
Council, N. R. (2012). “Current state of water reuse,” in Water reuse: potential for expanding the nation’s water supply through reuse of municipal wastewater (The National Academies Press).
Dittmer, U., Bachmann-Machnik, A., and Launay, M. A. (2020). Impact of combined sewer systems on the quality of urban streams: frequency and duration of elevated micropollutant concentrations. WaterSwitzerl. 12 (3), 850. doi:10.3390/w12030850
EPA (2022). Draft aquatic life ambient water quality criteria for perfluorooctane sulfonate (PFOS). Washington: D.C. Available at: https://www.epa.gov/wqc/aquatic-life-criteria-perfluorooctane-sulfonate-pfos.
Geissen, V., Mol, H., Klumpp, E., Umlauf, G., Nadal, M., Ploeg, M., et al. (2015). Emerging pollutants in the environment: a challenge for water resource management. Int. Soil Water Conserv. Res. 3 (1), 57–65. doi:10.1016/j.iswcr.2015.03.002
Ghernaout, D., Elboughdiri, N., and Alghamdi, A. (2019). Direct potable reuse: the Singapore NEWater project as a role model. Open Access Libr. J. 06 (5980), 1–10. doi:10.4236/oalib.1105980
Gross, B., Montgomery-Brown, J., Naumann, A., and Reinhard, M. (2004). Occurrence and fate of pharmaceuticals and alkylphenol ethoxylate metabolites in an effluent-dominated river and wetland. Environ. Toxicol. Chem. 23 (9), 2074–2083. doi:10.1897/03-606
Houtz, E. F., and Sedlak, D. L. (2012). Oxidative conversion as a means of detecting precursors to perfluoroalkyl acids in urban runoff. Environ. Sci. Technol. 46 (17), 9342–9349. doi:10.1021/es302274g
Jackson, S. H., and Winchell, M. (2011). “Use of the EPA Storm water management model (SWMM) for diagnosis of pesticide off-target movement from residential areas,” in Pesticide mitigation strategies for surface water quality. Editors K. S. Goh, B. L. Bret, T. L. Potter, and J. Gan, 309–327.
Jeffrey, P., Yang, Z., and Judd, S. J. (2022). The status of potable water reuse implementation. Water Res. 214, 118198. doi:10.1016/j.watres.2022.118198
Johnson, A. C., Ternes, T., Williams, R. J., and Sumpter, J. P. (2008). Assessing the concentrations of polar organic microcontaminants from point sources in the aquatic environment: measure or model? Environ. Sci. Technol. 42 (15), 5390–5399. doi:10.1021/es703091r
Keller, V. (2015). Risk assessment of “down-the-drain” chemicals: search for a suitable model. Sci. Total Environ. 360 (2006), 305–318. doi:10.1016/j.scitotenv.2005.08.042
Kim, H., Seagren, E. A., and Davis, A. P. (2003). Engineered bioretention for removal of nitrate from stormwater runoff. Water Environ. Res. 75 (4), 355–367. doi:10.2175/106143003X141169
Kim, S., Chu, K. H., Al-Hamadani, Y. A. J., Park, C. M., Jang, M., Kim, D. H., et al. (2018). Removal of contaminants of emerging concern by membranes in water and wastewater: a review. Chem. Eng. J. 335 (2017), 896–914. doi:10.1016/j.cej.2017.11.044
Koormann, F., Rominger, J., Schowanek, D., Wagner, J. O., Schröder, R., Wind, T., et al. (2006). Modeling the fate of down-the-drain chemicals in rivers: an improved software for GREAT-ER. Environ. Model. Softw. 21 (7), 925–936. doi:10.1016/j.envsoft.2005.04.009
Lämmchen, V., Klasmeier, J., Hernandez-Leal, L., and Berlekamp, J. (2021a). Spatial modelling of micro-pollutants in a strongly regulated cross-border lowland catchment. Environ. Process. 8 (3), 973–992. doi:10.1007/s40710-021-00530-2
Lämmchen, V., Niebaum, G., Berlekamp, J., and Klasmeier, J. (2021b). Geo-referenced simulation of pharmaceuticals in whole watersheds: application of GREAT-ER 4.1 in Germany. Environ. Sci. Pollut. Res. 28 (17), 21926–21935. doi:10.1007/s11356-020-12189-7
Li, Z., Sobek, A., and Radke, M. (2016). Fate of pharmaceuticals and their transformation products in four small European rivers receiving treated wastewater. Environ. Sci. Technol. 50 (11), 5614–5621. doi:10.1021/acs.est.5b06327
Lin, A. Y. C., Plumlee, M. H., and Reinhard, M. (2006). Natural attenuation of pharmaceuticals and alkylphenol polyethoxylate metabolites during river transport: photochemical and biological transformation. Environ. Toxicol. Chem. 25 (6), 1458–1464. doi:10.1897/05-412R.1
Los Angeles County and Los Angeles County Public Works (2020). LA River master plan. Los Angeles: CA.
Luthy, R. G., Wolfand, J. M., and Bradshaw, J. L. (2020). Urban water revolution: sustainable water futures for California cities. J. Environ. Eng. 146 (7), 146. doi:10.1061/(asce)ee.1943-7870.0001715
Mandaric, L., Mor, J. R., Sabater, S., and Petrovic, M. (2018). Impact of urban chemical pollution on water quality in small, rural and effluent-dominated mediterranean streams and rivers. Sci. Total Environ. 613–614, 763–772. doi:10.1016/j.scitotenv.2017.09.128
Maruya, K. A., Lao, W., Vandervort, D. R., Fadness, R., Lyons, M., and Mehinto, A. C. (2022). Bioanalytical and chemical-specific screening of contaminants of concern in three California (USA) watersheds. Heliyon 8 (5), e09534. doi:10.1016/j.heliyon.2022.e09534
Matamoros, V., Duhec, A., Albaigés, J., and Bayona, J. M. (2009). Photodegradation of carbamazepine, ibuprofen, ketoprofen and 17α-ethinylestradiol in fresh and seawater. Water. Air. Soil Pollut. 196 (1–4), 161–168. doi:10.1007/s11270-008-9765-1
Moldovan, Z. (2006). Occurrences of pharmaceutical and personal care products as micropollutants in rivers from Romania. Chemosphere 64 (11), 1808–1817. doi:10.1016/j.chemosphere.2006.02.003
Mutzner, L., Furrer, V., Castebrunet, H., Dittmer, U., Fuchs, S., Gernjak, W., et al. (2022). A decade of monitoring micropollutants in urban wet-weather flows: what did we learn? Water Res. 223, 118968. doi:10.1016/j.watres.2022.118968
Olivieri, A. W., Pescon, B., Crook, J., and Hultquist, R. (2020). California water reuse--past, present and future perspectives. Adv. Chem. Pollut. Environ. Manag. Prot. 5, 65–111. doi:10.1016/bs.apmp.2020.07.002
Park, J., Kim, M.-H., Choi, K., Kim, Y., and Kim, M.-Y. (2007). Environmental risk assessment of pharmaceuticals: model application for estimating pharmaceutical exposures in the han River Basin: Korea Environment Institute.
Patel, M., Kumar, R., Kishor, K., Mlsna, T., Pittman, C. U., and Mohan, D. (2019). Pharmaceuticals of emerging concern in aquatic systems: chemistry, occurrence, effects, and removal methods. Chem. Rev. 119 (6), 3510–3673. doi:10.1021/acs.chemrev.8b00299
Radcliffe, J. C., and Page, D. (2020). Water reuse and recycling in Australia — history, current situation and future perspectives. Water Cycle 1, 19–40. doi:10.1016/j.watcyc.2020.05.005
Roberts, P. H., and Thomas, K. V. (2006). The occurrence of selected pharmaceuticals in wastewater effluent and surface waters of the lower tyne catchment. Sci. Total Environ. 356 (1–3), 143–153. doi:10.1016/j.scitotenv.2005.04.031
Schliemann, S. A., Grevstad, N., and Brazeau, R. H. (2021). Water quality and spatio-temporal hot spots in an effluent-dominated urban river. Hydrol. Process. 35 (1). doi:10.1002/hyp.14001
Schwarzenbach, R. P., Escher, B. I., Fenner, K., Hofstetter, T. B., Johnson, C. A., Gunten, U. V., et al. (2006). The challenge of micropollutants in aquatic systems. Science 313 (5790), 1072–1077. doi:10.1126/science.1127291
Sharma, D., and Kansal, A. (2013). Assessment of river quality models: a review. Rev. Environ. Sci. Biotechnol. 12 (3), 285–311. doi:10.1007/s11157-012-9285-8
Southern California Coastal Water Research Project (2017). Screening study for constituents of emerging concern (CECs) in selected freshwater rivers in the Los Angeles region – phase 3 – in vitro screening and targeted analysis of CECs in water and sediment. Costa Mesa: CA.
Stein, E. D., Taniguchi-Quan, K., Wolfand, J., Gallo, E., Irving, K., Philippus, D., et al. (2021a). Process and decision support tools for evaluating flow management targets to support aquatic life and recreational beneficial uses of the Los. Angeles River: Los Angeles River Environmental Flows Project.
Stein, E. D., Wolfand, J., Abdi, R., Irving, K., Hennon, V., Taniguchi-Quan, K., et al. (2021b). Assessment of aquatic life use needs for the Los Angeles river. Southern California Coastal Water Research Project.
Sumpter, J. P., Johnson, A. C., Williams, R. J., Kortenkamp, A., and Scholze, M. (2006). Modeling effects of mixtures of endocrine disrupting chemicals at the river catchment scale. Environ. Sci. Technol. 40 (17), 5478–5489. doi:10.1021/es052554d
U.S. Environmental Protection Agency (2022). Fact sheet: PFOA and PFOS drinking water health advisories. https://www.epa.gov/sdwa/drinking-water-health-advisories-pfoa-and-pfos.
Wang, R., Yuan, Y., Yen, H., Grieneisen, M., Arnold, J., Wang, D., et al. (2019). A review of pesticide fate and transport simulation at watershed level using SWAT: current status and research concerns. Sci. Total Environ. 669, 512–526. doi:10.1016/j.scitotenv.2019.03.141
WateReuse Association (2023). Global connections map. Available at: https://water360.com.au/map/.
Wiegel, S., Aulinger, A., Brockmeyer, R., Harms, H., Löffler, J., Reincke, H., et al. (2004). Pharmaceuticals in the river elbe and its tributaries. Chemosphere 57 (2), 107–126. doi:10.1016/j.chemosphere.2004.05.017
Wolfand, J. M., Sytsma, A., Hennon, V. L., Stein, E. D., and Hogue, T. (2022a). Dilution and pollution: assessing the impacts of water reuse and flow reduction on water quality in the Los Angeles River Basin. Environ. Sci. Technol. Water 2, 1309–1319. doi:10.1021/acsestwater.2c00005
Wolfand, J. M., Taniguchi-Quan, K. T., Abdi, R., Gallo, E., Irving, K., Philippus, D., et al. (2022b). Balancing water reuse and ecological support goals in an effluent dominated river. J. Hydrol. X 15, 100124. doi:10.1016/j.hydroa.2022.100124
Xiao, F., Simcik, M. F., and Gulliver, J. S. (2012). Perfluoroalkyl acids in urban stormwater runoff: influence of land use. Water Res. 46 (20), 6601–6608. doi:10.1016/j.watres.2011.11.029
Yun, D., Abbas, A., Jeon, J., Ligaray, M., Baek, S., and Cho, K. H. (2021). Developing a deep learning model for the simulation of micro- pollutants in a watershed. J. Clean. Prod. 300, 126858. doi:10.1016/j.jclepro.2021.126858
Keywords: wastewater reuse, water quality, contaminants of emerging concern, los angeles, urban water management, PFOS, EPA SWMM
Citation: Wolfand JM, Sytsma A, Taniguchi-Quan KT, Stein ED and Hogue TS (2023) Impact of wastewater reuse on contaminants of emerging concern in an effluent-dominated river. Front. Environ. Sci. 11:1091229. doi: 10.3389/fenvs.2023.1091229
Received: 06 November 2022; Accepted: 17 October 2023;
Published: 06 November 2023.
Edited by:
Michael Nones, Polish Academy of Sciences, PolandReviewed by:
Gianluigi Buttiglieri, Catalan Institute for Water Research, SpainGabrielle David, Cold Regions Research and Engineering Laboratory, United States
Copyright © 2023 Wolfand, Sytsma, Taniguchi-Quan, Stein and Hogue. This is an open-access article distributed under the terms of the Creative Commons Attribution License (CC BY). The use, distribution or reproduction in other forums is permitted, provided the original author(s) and the copyright owner(s) are credited and that the original publication in this journal is cited, in accordance with accepted academic practice. No use, distribution or reproduction is permitted which does not comply with these terms.
*Correspondence: Jordyn M. Wolfand, d29sZmFuZEB1cC5lZHU=