- 1MARE—Marine and Environmental Sciences Centre, ARNET—Aquatic Research Network Associate Laboratory, NOVA School of Science and Technology, NOVA University Lisbon, Caparica, Portugal
- 2GeoBioTec—NOVA School of Science and Technology, NOVA University Lisbon, Caparica, Portugal
- 3GeoBioTec—Geosciences Department, University of Aveiro, Aveiro, Portugal
Understanding local accumulation patterns of microplastics in subtidal sediments is crucial to assess how available such particles are for ingestion by benthic feeders and to identify the potential pollution sources in the region upon which is urgent to act. The coastal urban centers of Setúbal and Sesimbra (Portugal) and the multiple activities taking place at the contiguous Sado estuary and in the sheltered waters of Professor Luiz Saldanha Marine Park make this a relevant case study about MPs pollution in the seabed. Here, a short-term investigation assessed the spatiotemporal distribution, abundance, and composition of MPs on the nearshore seabed. Sediment samples were monthly collected from summer 2018 to winter 2019, in six stations. Despite the differences observed in rainfall between campaigns, no distinct patterns were detected in the accumulation of MPs throughout the sampled months. Yet, strong variations occurred among stations. The abundance of MPs in the Sado estuary (1042.8 ± 430.8 items kg−1) was higher in comparison to all the stations located along the marine park (52.9 ± 31.9 items kg−1). Fragments comprised 70% of particles found in estuarine sediments, while fibers were the predominant type in marine sediments. The majority of MPs collected in the estuary shared the same size class as the best represented grain size fraction: 0.250–0.500 mm. On average, the ratio between the abundance of MPs and the abundance of meiofauna organisms was higher in the estuary, suggesting more encounter rates, by both meiofauna and their predators, with MPs. The distribution of MPs throughout the study area was moderately correlated with sediment sorting and organic matter content. Also, the distinct mineralogical content of each station indicates a reduced sediment transit between stations and consequently a weak exportation of MPs from the estuary. The majority of the polymers identified by Fourier Transform Infrared Spectroscopy was denser than seawater. Polyethylene terephthalate represented 41% of the items analyzed and was mostly assigned to fibers and fiber bundles. Unveiling the distribution patterns of MPs along this segment of the Portuguese west coast enabled to identify a high-risk area where the implementation of preventive measures is urgent.
Introduction
Marine sediments are long-term sinks for microplastics (MPs) (Cozar et al., 2014; Zhang, 2017; Pohl et al., 2020; Coppock et al., 2021). Though firstly demonstrated by Thompson et al. (2004), such evidence was actually in accordance with several previous studies reporting marine sediments as the ultimate fate of larger plastic debris (Bingel et al., 1987; Galgani et al., 1995a, 1995b, 2000; Kanehiro et al., 1995). However, despite this perception, plastic pollution research has been mostly focused on seawater surface, as argued by Lusher et al. (2014), van Sebille et al. (2015), Porter et al. (2018) and Yao et al. (2019). The shift on scientific interest towards sedimentary matrices was triggered with the growing understanding about the 100-fold discrepancy between the estimates of all the plastic waste input in the oceans and the lower estimates of the global load of floating debris being reported (Cozar et al., 2014; Eriksen et al., 2014; Lindeque et al., 2020).
The lack of methodological standardization in pioneer studies about MPs in the marine substrate compelled the improvement and development of new extraction protocols (Rochman et al., 2017) that could prevent, for instance, the underestimation of high-density polymers (Imhof et al., 2012; Claessens et al., 2013; Nuelle et al., 2014; Coppock et al., 2017; Frias et al., 2018; Pagter et al., 2018). The baseline data being subsequently acquired confirmed that, besides the particles exceeding seawater density (>1.02 g cm−3), the low-density MPs would also end up reaching the seafloor (Frias et al., 2016; Martin et al., 2017; Coppock et al., 2021) as earlier reported for larger items (Holmström, 1975; Kanehiro et al., 1995; Hess et al., 1999; Stefatos et al., 1999). Deposition of low density particles into benthic substrates will depend on biofouling processes (Holmström, 1975; Pegram and Andrady, 1989; Ye and Andrady, 1991; Andrady, 2011), incorporation into marine snow (Van Cauwenberghe et al., 2013; Woodall et al., 2014; Porter et al., 2018) or into fecal pellets (Cole et al., 2016; Coppock et al., 2019).
Regardless of their density, MPs in the marine environment may have multiple origins. Besides those resulting from the fragmentation of larger plastic (Thompson et al., 2004; Ryan et al., 2009), which strongly occurs at shorelines due to the higher mechanical abrasion, temperatures, and exposure to UV radiation (Pegram and Andrady, 1989; Gregory and Andrady, 2005; Barnes et al., 2009; Andrady, 2011); others come from land, namely through wastewater treatment plants (WWTP) effluents (Gregory, 1996; Fendall and Sewell, 2009; Browne et al., 2011; Murphy et al., 2016), sewage discharges, and urban (stormwater) runoff (Piñon-Colin et al., 2020; Werbowski et al., 2021); other pathways of plastic transport from land include wind, rivers and tides (McCormick et al., 2014; Jambeck et al., 2015). Additionaly, several sea-based activities may also contribute to MPs pollution, such as fishing, aquaculture, maritime traffic, offshore platforms and recreational (Andrady, 2011; Jambeck et al., 2015; UNEP, 2016).
The high potential for MPs to accumulate in coastal sediments is therefore related with both the proximity to the multiple pollution sources and the propensity of particles to sink, independently of their polymeric composition. As a consequence, the interactions of MPs with bottom-dweller organisms (Graham and Thompson, 2009; Murray and Cowie, 2011; Van Cauwenberghe et al., 2015; Bour et al., 2018), as meiofauna (Gusmão et al., 2016) and/or their predators (Lusher et al., 2013; Bellas et al., 2016), end up occuring more frequently at coastal areas. Furthermore, as both estuarine and coastal marine sediments are known to accumulate high concentrations of organic and inorganic pollutants (Castro and Vale, 1995; Lacorte et al., 2003; Vieira et al., 2021; Bellanova et al., 2022), the MPs settling in these areas are expected to be highly associated to such contaminants (Bakir et al., 2014).
Despite the growing concern regarding the ecotoxicological risk faced by sediment biota upon MPs ingestion (including commercial species) and the increase of research about this topic, the identification of the adverse effects is yet to be fully accomplished and far from being consensually accepted. In fact, as argued by several researchers (Rochman and Boxallz, 2014; Lenz et al., 2016; Phuong et al., 2016; Burns and Boxall, 2018), detrimental impacts being reported in experimental studies have frequently resulted testing conditions which greatly exceed those considered as environmentally relevant. Thus, assessing realistic levels of exposure to MPs faced by biota associated to subtidal sediments (defined as those permanently submerged and extending from the low tide mark to about 200 m depth) is critical, particularly in Portugal, a coastal nation, where studies on this topic are scarce. So far, the available data about MPs pollution on the seafloor at Portuguese coastal waters relies on the research conducted by Frias et al. (2016), about sediments collected in Algarve, from depths lower than 25 m.
Hence, here we aim to provide baseline data about MPs accumulation in subtidal sediments from the Portuguese west coast, namely from the Professor Luiz Saldanha Marine Park and the Sado river estuary, where conservation measures with more than 2 decades aim to protect habitats and species. In particular, besides investigating if temporal and/or spatial patterns occur in MPs abundance, distribution, and composition in this region, we also estimated (to the authors knowledge, for the first time) the MPs to meiofauna ratio in each sample. By comparing their abundances we intended to identify the areas where meiofauna organisms would face higher risk of exposure to MPs. In addition, we aimed to assess potential relationships between MPs abundance and rainfall, sediment organic matter and granulometric parameters, which might be usefull in further monitoring studies. Lastly, the analysis of the mineral content of sediment samples was also conducted to determine, together with polymers identification, possible links to potential pollution sources in this region.
Materials and methods
Study area
The study area, comprised by the Sado estuary and the Professor Luiz Saldanha Marine Park (Figure 1), is subject to multiple anthropogenic pressures. These have been described in a preceding study focused on MPs occurring at these surface waters (Rodrigues et al., 2020), which shares the same fieldwork period and location as this study. Sediment samples were thus collected in six sampling campaigns, as described in the previously mentioned study (between August 2018 and February 2019), at the same six nearshore sampling stations (located at the 5 m isobaths). The six sampling campaigns occurred every 30 days (approximately) whenever the weather conditions allowed. As we had to ensure a Beaufort wind scale ≤3 to properly collect the floating MPs with neuston trawls, the scheduling of sampling campaigns required adjustments which prevented an entirely consecutive sequence of months.
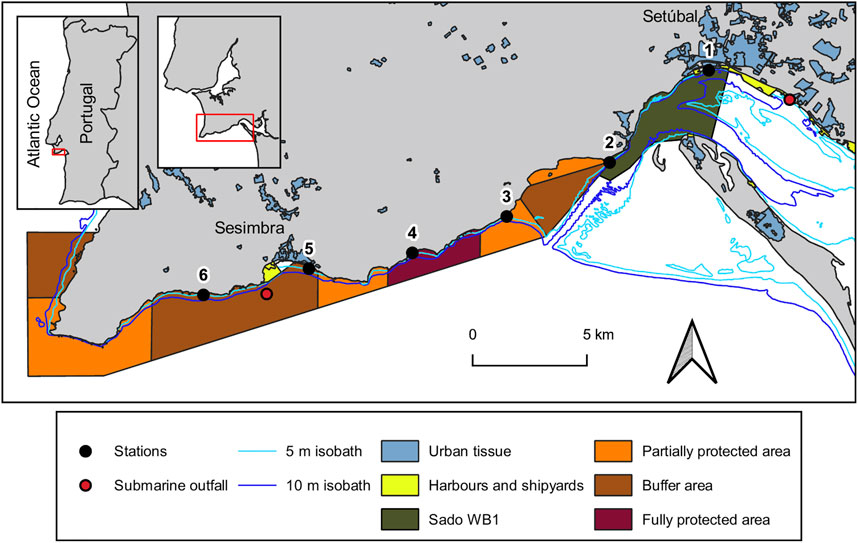
FIGURE 1. Map of the study area (developed in QGIS) with the location of the six sampling stations (black dots) distributed along the coastal area of Setúbal and Sesimbra, on the Portuguese west coast. The complete information about the map layers as well as the list with the GPS coordinates of each station are provided at the map source: Rodrigues et al. (2020).
Sampling methods
Two replicate sediment samples (R1 and R2) were collected at each station with a Wildco® Petite Ponar benthic grab. This grab collects sediments from the seabed superficial layer and has a sample area of ca 15 cm × 15 cm. After retrieving the grab from the water, its load was laid directly into a stainless-steel tray (34 cm × 24 cm) and the excess water was discarded. Sediment was then transferred with a wooden spoon to a 500 ml glass jar, up to its maximum capacity. Separately, a small aliquot was collected from one of the replicates for meiofauna analysis, stored in a 250 ml glass jar and fixed in 70% ethanol. Sediment samples (72 in total) were transported in ice coolers and kept frozen at −20°C until analysis.
Laboratory procedures
MPs extraction and characterization
MPs extraction was carried out at the laboratory, after thawing samples at room temperature. Approximately 250 g (wet weight) of each replicate was transferred into Ø150 mm glass Petri dishes, manually homogenized and placed in the oven at 60°C for 48 h. The content left in the jars was frozen again until further analysis.
Three sub-replicates of 50 g each (dry sediment) were directly weighed in 1 L beakers. In order to remove the organic matter content, 150 ml of 10% hydrogen peroxide (Frias et al., 2018) was added to each beaker. The content was mixed with a glass rod for 1 min and left for 24 h in a fume hood, at room temperature. Each sub-replicate was subsequently poured into a 63 μm sieve and rinsed with distilled water. Then, it was transferred into a Sediment-Microplastic Isolation device (SMI-unit; designed by Coppock et al., 2017) where a magnetic stir bar (45 × 8 mm) was previously added. The SMI-unit was topped up with ZnCl2 solution (1.5 g cm−3; APC Pure®) until a volume of 700 ml was achieved, and the sediment was mixed as described in the protocol of Coppock et al. (2017). All samples were left to settle for 2 h, except for those collected from st1 (at the estuary) which, due to higher silt/clay fractions, needed a longer period (20 h).
When the settling period was over, the valve was closed and the headspace content was vacuum filtered through a glass microfiber filter placed on the stainless-steel screen support of the glass filtration base (filter: MFV2 FILTER-LAB 47 mm Ø with 1 μm pore; filtration base: XX1014732, Millipore). To recover the MPs eventually left in the internal surface of the SMI-unit, the top part was rinsed thoroughly with ZnCl2 and filtered a second time. Finally, the ZnCl2 solution at the bottom part was also filtered, ensuring the solution reuse in subsequent samples. Since the solution density could slightly decline with the continuous use, two batches of ZnCl2 solution were prepared to ensure a similar MPs extraction efficiency among all samples (5 L each; one for R1 samples and another for R2 samples). The extraction of MPs from R2 replicates was only performed after procedures for R1 group were completed.
Filters from each sub-replicate, were stored individually in glass Petri dishes and observed under a stereomicroscope (Leica® S8APO) equipped with a camera (Motic® MOTICAM 10+). Particles were classified according to color and type, counted, and measured with the Motic® Images Plus 3.0 software. Only MPs belonging to the 0.063–5 mm size range were considered, being all categorized to one of the following size classes: 0.063–0.125 mm, 0.125–0.250 mm, 0.250–0.500 mm, 0.500–1 mm, and 1–5 mm; these size classes were selected to match the grain size fractions considered in granulometric analysis. Also, each microplastic was assigned to one of seven types: fragment, film, fiber, fiber bundles, filament, glitter, and bead, as described in Table 1 (adapted from Lusher et al. (2017), Rochman et al. (2019) and Rodrigues et al. (2020)). All particles similar to shavings (Total = 621) were excluded from analysis because they were considered to result from the degradation of the SMI valve made of Polyvinyl chloride (Nel et al., 2019). Particles selected for polymer identification were isolated in covered concave slides. The abundance of MPs per sample consisted of the average of counts from the six sub-replicates of 50 g (3 from R1 and three from R2), which were then normalized to a constant weight and reported as items per kg of dry sediment (items kg−1).
Polymer identification
About 8% (186 items; Table 2) of the total of particles was selected for polymer identification. The selection was conducted after discarding fibers considered airborne contamination and was based on the best expert judgment according to similarity, texture, thickness, and shine. All particles with a 1–5 mm size range, except for fibers, were analyzed by Fourier Transform Infrared Spectroscopy in attenuated total reflectance (FTIR-ATR), using a Perkin Elmer® Spectrum Two spectrometer. For smaller particles (0.063–1 mm) and fibers, analyses were carried out on a µ-FTIR spectrometer (Perkin Elmer® Spotlight 200i Microscope System), with microscope aperture 100 μm × 100 μm, using a strong Norton-Beer apodization. All spectra were acquired at room temperature under reflectance mode with a resolution of 4 cm−1 and 1 cm−1 wavenumber intervals, within 4,000–500 cm−1. The analysis was performed on the sample surface, sometimes in more than one point, when results were dubious. A background scan was performed before any analysis series. Polymer identification relied on a match over 80% (Pequeno et al., 2021) between the sample and a referenced database (Primpke et al., 2018). The assignments were confirmed with the analysis of the polymers characteristic bands (Hummel, 2002; Xiao et al., 2002; Marković et al., 2009; Arshad et al., 2011; Kausar, 2015; Jung et al., 2018).
Quality assurance and quality control
To assess airborne contamination, control filters (blanks) were exposed to the air, both during sampling (inside a hanging open glass jar at the boat deck, one blank per sampling campaign) and throughout lab work (in Petri dishes, one per replicate). Thus, all the fibers extracted from samples which were similar to those found in respective blanks were excluded from results. Other contamination sources were minimized, both during field and laboratory work, by using glass, stainless-steel and wooden materials. At the laboratory, samples were kept covered at all times, a cotton lab coat and nitrile gloves were always worn and working surfaces were rinsed before use with Milli-Q water and ethanol. Moreover, all prepared solutions and rinsing liquids were filtered before use.
MPs to meiofauna ratios
After staining sediment aliquots with Rose Bengal for 1 h, the content of each jar was transferred into a 38 μm sieve, in order to discard the ethanol. Next, from the sediment retrieved on the sieve, six sub-aliquots of 5 ml were collected with a measuring spoon. Whereas the average abundance of meiofauna was quantified in three out of the six sub-aliquots, the other three allowed the conversion of the 5 ml volume into dry weight. The former group of sub-aliquots was placed separately into 1 L beakers and the other group was pre-weighed in Ø100 mm glass Petri dishes. The meiofauna extraction protocol, adapted from Somerfield and Warwick (2013), consisted of adding 200 ml of filtered tap water to each beaker, being their content stirred and decantated onto a 63 μm sieve. This was repeated 4 times, except for st1 samples due to the higher silt/clay fraction (6 times). The meiofauna (size range: 63–500 μm; (Giere, 2009)) retained by the sieve was washed back into a Bogorov counting chamber and counted under the stereomicroscope with the support of a hand tally counter. In what concerns the sub-aliquots kept in Petri dishes, these were placed uncovered in the oven at 60°C to dry (for ca 12 h) and then weighed; the mean of the three weight measurements was calculated. Finally, the abundance of meiofauna in sub-aliquots was extrapolated to a standard (dry) sediment weight (kg) and expressed as individuals per kg of dry sediment (ind kg−1), to match the reported units used for MPs (items kg−1) and to enable the calculation of the MPs to meiofauna ratio.
Sediment characterization
One replicate per sample was randomly selected to run granulometry and loss-on-ignition procedures. After defrosting, approximately 100 g (wet weight) of sediment, from each sample, was transferred to a Ø150 mm glass Petri dish, manually homogenized and oven-dried at 105°C for 24 h.
For granulometric analyses (protocol adapted from Pagter et al. (2018)), 50 g of each sample (dry sediment), was weighed in 1 L beakers, to the nearest 0.01 g. Then, to remove the organic matter, 200 ml of H2O2 (6%) was added to each beaker. After manually stirring, samples were left to digest until there was no sign of reaction (2 days on average; 50 ml of H2O2 were added per each extra day). Samples were subsequently poured onto a 63 μm sieve, rinsed with distilled water, and washed back into the 1 L glass beaker till a volume of 400 ml was obtained. To cause dispersion and to disaggregate fine-grained particles, 225 ml of a 4.2% Calgon solution (35 g of sodium hexametaphosphate and 7 g of sodium carbonate in 1 L of distilled water (Kaur and Fanourakis, 2016)) was added to each beaker, stirred and left overnight. Then, samples were poured again into a 63 μm sieve and rinsed with distilled water. After that, they were transferred into a Ø150 mm Petri dish and oven-dried at 105°C for 24 h. After cooling, each sample was weighed and dry sieved in an automated column shaker (RETSCH AS 200 basic) through a series of graduated sieves (2 mm, 1 mm, 0.500 mm, 0.250 mm, 0.125 mm, and 0.063 mm) for 10 min. The weight of the sediment retained in each sieve was registered. To determine the weight of the <63 μm fraction (clay and silt fractions combined), the sum of all weighed fractions was subtracted to the initial weight of sediment. Finally, the sediment grain size distribution, mean grain size and sorting, according to Folk and Ward (1957) geometric graphical measures, was determined from weight of the six fractions using the freeware Gradistat® (Version 9.1). Grain size fractions were classified according to the Udden-Wentworth grade scale (Udden, 1914; Wentworth, 1922).
To determine the organic matter content of sediment (Cambardella et al., 2001), three replicates of 1 g per sample (dry sediment) were weighed to the nearest 0.0001 g and transferred to labelled and pre-weighed crucibles. These were placed in a muffle furnace at 450°C for 4 h and, at the end of this period, moved into a desiccator for 1 h and weighed again. The organic matter content, expressed as percentage, was calculated from weight loss on ignition i.e., from the difference between the sediment weight before and after ignition.
Lastly, approximately 2 g per sample (dry sediment) were transferred into individual plastic bottles and taken to the Geobiotec/Aveiro University laboratory facilities to identify the mineral content of sediment samples. Qualitative and semi-quantitative mineralogical analyses were carried out by X-ray diffraction (XRD) using a Philips®/Panalytical X’Pert-Pro MPD, Kα Cu (λ = 1,5405 Å) radiation. All samples (total sample) were ground in an agate ring mill to obtain a finer granulometry and were analyzed on random-oriented powders, X-ray scanned in the 2° to 40° 2θ interval at 1°2θ/min goniometer speed. The identification of the different mineral phases followed the criteria recommended by Schultz (1964), Thorez (1976), Mellinger (1979), Brindley and Brown (1980) and Pevear and Mumpton (1989).
Rainfall
Measurements from four meteorological stations (obtained from SNIRH1) located near the sampling site were considered: Comporta (23E/01C), Vila Nogueira de Azeitão (22C/02UG), Águas de Moura (22E/01UG) and Montevil (23F/01UG). Except for the first sampling campaign (Aug18), the mean rainfall (mm) assigned to each campaign was based on daily measurements collected from the four stations, registered uninterruptedly from the day after the last sampling until the day before sampling (about 30 days in total). In what concerns the August 18 campaign, the mean rainfall calculation has only considered measurements from three stations, since data from Comporta was completely unavailable. In addition, the data obtained from those three meteorological stations was exclusively comprised by measurements registered during the 10 days which preceded the August sampling (because it was the only data available). Still, since the August month of 2018 was extremely dry (according to The Portuguese Institute for Sea and Atmosphere; IPMA; (IPMA, 2018)), we may assume that the missing data would not significantly change the mean rainfall calculation assigned to this specific campaign.
Statistical analysis
Data was analyzed through non-parametric tests whenever parametric assumptions (normality by Shapiro-Wilk test and homogeneity of variances by Levene test) were not met. Spearman correlations assessed if rainfall, sediment organic matter, mean grain size and sorting could interfere with MPs abundances. To evaluate if and how the previous variables (except for sediment organic matter) changed temporally (along 6 months) and/or spatially (among the six stations), Kruskal–Wallis tests were performed, being post-hoc multiple comparisons followed with Dunn’s test. The same analysis was conducted with the MPs to meiofauna ratio and with the Carbonate/Siliciclastic index. The variance of sediment organic matter (log (x+1) transformed), among stations and campaigns, was analyzed by a two-way ANOVA followed by Tukey post-hoc test for pairwise comparisons. The same test procedure was applied to assess the variance of meiofauna abundances (log (x+1) transformed). To analyze if fragments (log (x+1) transformed) were spatially distributed according to their mean size, a one-way ANOVA was conducted; the same test was performed with fibers (though with raw data). All the previously mentioned tests were performed with TIBCO Statistica™ 14.0.0 software and the level of significance was set at a p-value ≤ 0.05.
A univariate permutational analysis of variance (PERMANOVA), with 999 permutations, was performed to detect significant differences in MPs abundances, between stations and campaigns (fixed factors; with six levels each). Data were square-root transformed and the resemblance matrix between samples was calculated based on Bray-Curtis similarities. When differences were statistically significant, pair-wise comparisons among levels were analyzed and non-metric Multi-Dimensional Scaling (nMDS) plots were created. Additionally, whereas a multivariate PERMANOVA tested the effect of campaigns and stations in the abundance of each type of MPs in sediments, another focused on the response of MPs abundance according to size classes. Subsequently, to determine which type or size class most contributed to explain dissimilarities, the similarity percentages routine (SIMPER; with a cut-off percentage of 90% for low contributions) was conducted. Similarly, a multivariate PERMANOVA was applied to assess temporal or spatial patterns in the granulometric fractions of the sediment samples. All PERMANOVA analyses were developed in the Primer six software with the Permanova + add-on (Clarke and Gorley, 2006; Anderson et al., 2021).
Results
Presence and abundance of MPs
From the total amount of particles extracted from sediment samples (4,060), 1,603 (40%) fibers were discarded for being considered airborne contamination during field and lab work. Also, 104 particles (belonging to several types) were excluded due to one of the following FTIR results: non-plastic particle, inconclusive match, or match under 80%. Therefore, the assessment of temporal and spatial distribution patterns of MPs in sediments was based on a total of 2353 particles (0.063–5 mm size range). Although 80% of this amount were MPs extracted from estuarine sediments (st1), all samples contained MPs (Figure 2A). The abundance of MPs in sediments collected at st1 was 1042.8 ± 430.8 items kg−1 (mean ± SD), whereas in sediments from st2 to st6 was 52.9 ± 31.9 items kg−1 (Figure 2B). The lowest MPs abundance (23.3 ± 29.3 items kg−1) was observed in Sesimbra bay (st5) in Feb19 (winter), while the highest (2170 ± 1157.1 items kg−1) was found in Oct18 (autumn), at Setúbal closest station (st1).
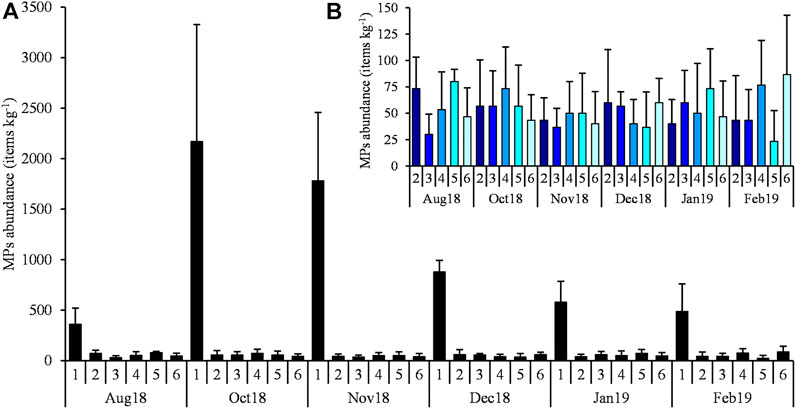
FIGURE 2. MPs abundance (items kg−1; mean ± SD) per sample. Whereas all samples (n = 36) are depicted in (A), only samples from stations two to 6 (n = 30) are represented in (B).
Fourier transformed infrared spectroscopy (FTIR) analysis
Among the 82 particles confirmed as plastic by FTIR analysis, a total of 11 polymers, including the Copolymer PP/PE, were identified (Table 3; Figure 3). Despite the high diversity of polymers, the majority of particles were identified as PET (41%), mostly assigned to fibers or fiber bundles (ca 65%; Figure 4).
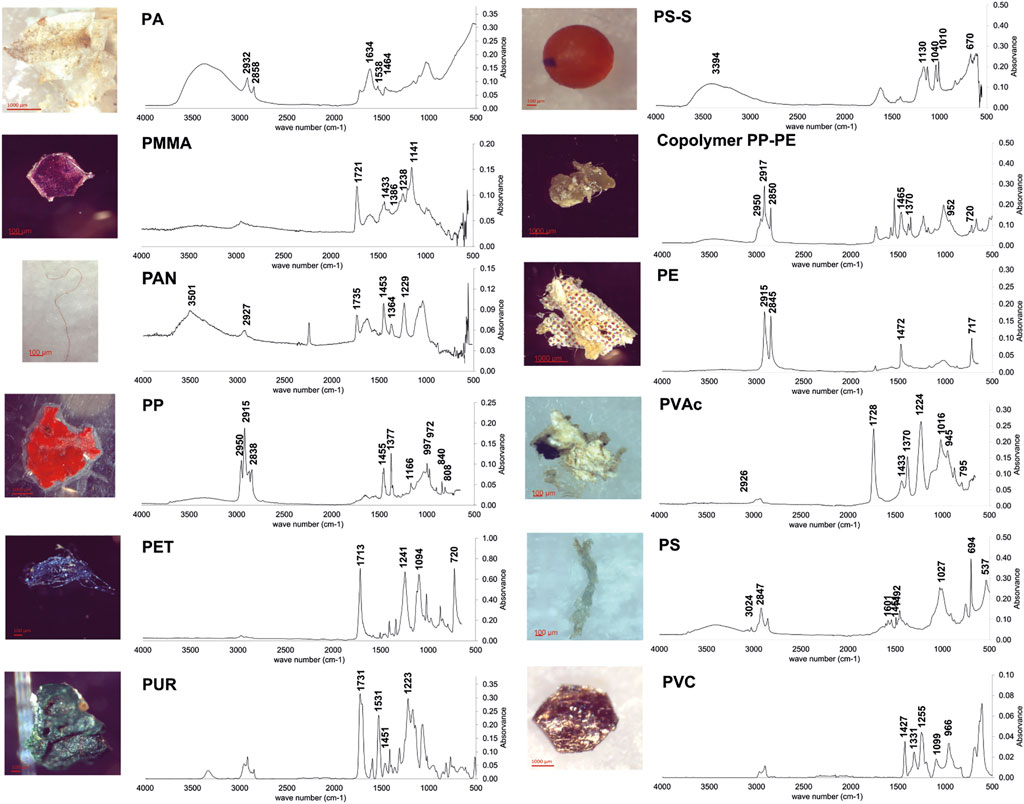
FIGURE 3. Representative infrared spectra of the 11 identified polymers, with Polyacrylates represented by two items: PMMA and PAN. The image assigned to each spectrum corresponds to the MPs analyzed. The 12 MPs depicted in this figure belong to the following types: PA and PE - film; PS-S - bead; PMMA and PVC - glitter; Copolymer PP-PE, PP, PVAc and PUR - fragment; PAN - fiber; PS–filament; PET–fiber bundle.
Meiofauna abundance and MPs to meiofauna ratios
Fluctuations in meiofauna abundance were only significant between stations (F(5,25) = 5.941, p = 0.0009; Figure 5B), being undoubtedly higher in the estuary (23444.1 ± 16614.9 individuals kg−1; mean ± SD) than in all the other stations (p < 0.05). The lowest meiofauna abundance (3411.5 ± 2014.5 individuals kg−1; mean ± SD) was registered at st6 (the furthest station from the estuary). The ratio between MPs and meiofauna differed significantly between stations (H (5) = 13.95, p = .02; Figure 5B), namely between st1 and st3, but not between campaigns (H (5) = 4.64, p = .46; Figure 5A). The highest ratio observed in st1 (estuarine sediments) was 0.065, or one microplastic to 15.3 meiofauna organisms, where both MPs and meiofauna abundances reached maximum levels; the average ratio in st1 was 0.047 ± 0.013 (mean ± SD). The lowest ratio among all samples was registered in st3 (0.003 or one microplastic to 383.1 meiofauna organisms).
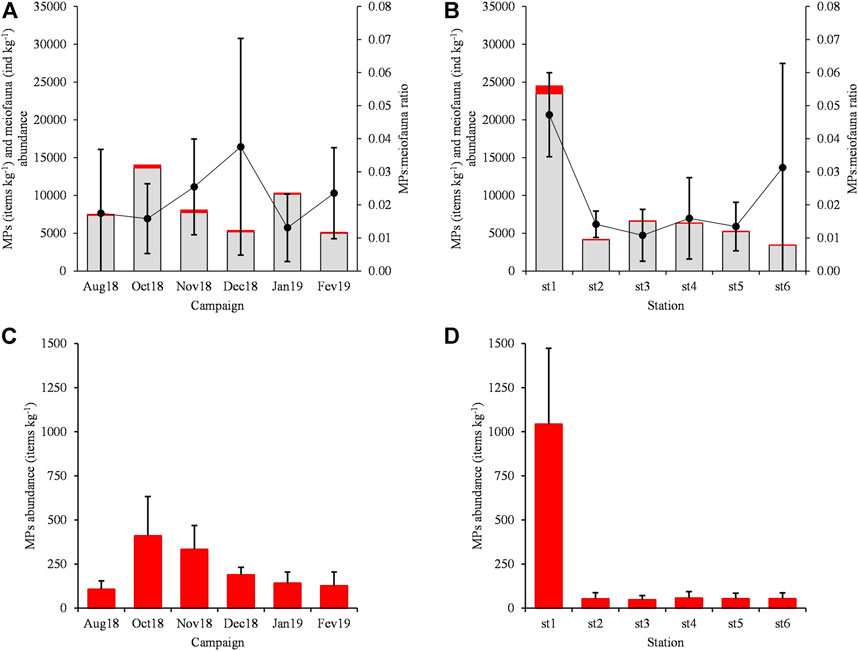
FIGURE 5. MPs abundance (items kg−1) (red bar), meiofauna abundance (individuals kg−1) (grey bar) and MPs to meiofauna ratio (black dots), per campaign (A) and per station (B). MPs abundance (items kg−1; mean ± SD; n = 6) per campaign (C) and per station (D).
Temporal and spatial distribution patterns of MPs
In contrast to the absence of significant temporal variations in MPs abundances among the monthly campaigns (Pseudo-F = 0.61, P (perm) = 0.823; Figure 5C), a significant spatial distribution pattern was observed (Pseudo-F = 31.22, P (perm) = 0.001; Figure 5D), consisting of a higher abundance of MPs in estuarine sediments (st1), in comparison with all the other stations (Figures 6A–D). SIMPER results show that this dissimilarity (between st1 and all the other stations) mainly relies on the predominance and particular accumulation of fragments in the estuary (43–45% contribution for the differences; Figure 6C). Furthermore, all the other types, except for fibers, were also mostly available in the estuary, though considerably less represented than fragments (Figure 6E). Conversely, fibers residually contributed (max 8%, according to SIMPER results) for the mentioned dissimilarity, as their abundances were similar throughout the study area (Figure 6C). While fragments predominated in estuary sediments (st1), fibers were the prevalent type found in marine sediments (i.e., at all the other five stations; Figure 6E). Moreover, dissimilarities between stations were also based on particle size (Figures 6B,D), particularly on MPs belonging to the 0.250–0.500 mm size class (with 26–27% contribution to the differences found; Figure 6D). While MPs accumulated in the estuarine station were mostly assigned to the 0.250–0.500 mm, followed by the 0.500–1 mm size classes, at the ocean exposed stations, the majority of MPs belonged to 0.500–1 mm and 1–2 mm size classes (Figure 6F).
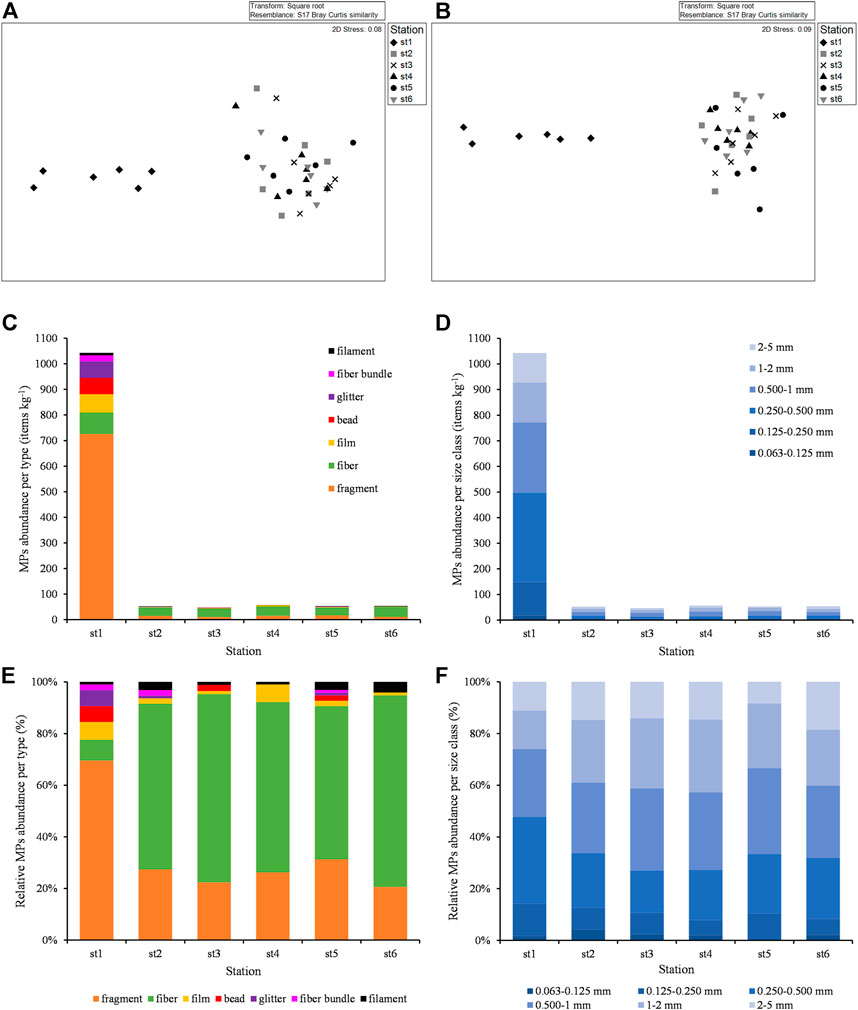
FIGURE 6. Multidimensional scaling plot based on the Bray-Curtis distance between samples of the different stations according to type of MPs (A) and to MPs size class (B). Spatial variation of mean MPs abundance (items kg−1) per particle type (C) and per size class (D). Relative proportion of particle types, per station (E). Relative distribution of particles size class, per station (F).
Among fragments collected from the estuary (st1; representing 70% of MPs in this station), the color green (34%) was the prevalent one, followed by blue (20%) and white (13%) (Figure 7A). Regarding fibers (similarly distributed among the six stations), black was the most frequent color (17%), followed by transparent (15%) and red (14%) (Figure 7B).
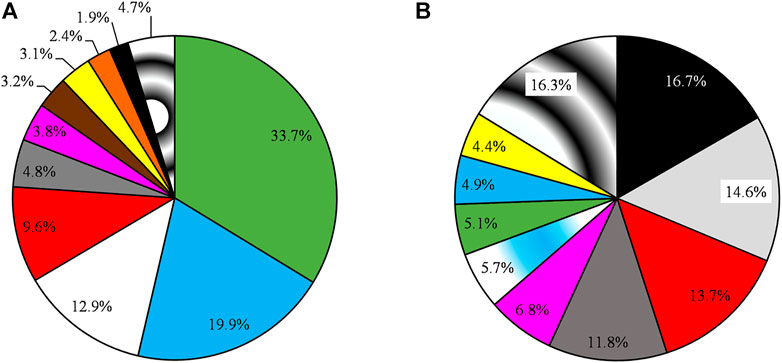
FIGURE 7. Color composition of fragments collected at st1 (A) and of fibers collected from the six stations (B). The black and white slice corresponds to a pool of other colors. The light grey slice in (B) represents transparent fibers.
Neither fragments (F(5,30) = 2.18, p = 0.08; Figure 8A) nor fibers (F(5,30) = 1.05, p = 0.41; Figure 8B) showed significant differences in their mean size among the six stations. Mean size of fragments (486.8 ± 247.4) was 2.6 times smaller than fibers (1244.1 ± 243.6).
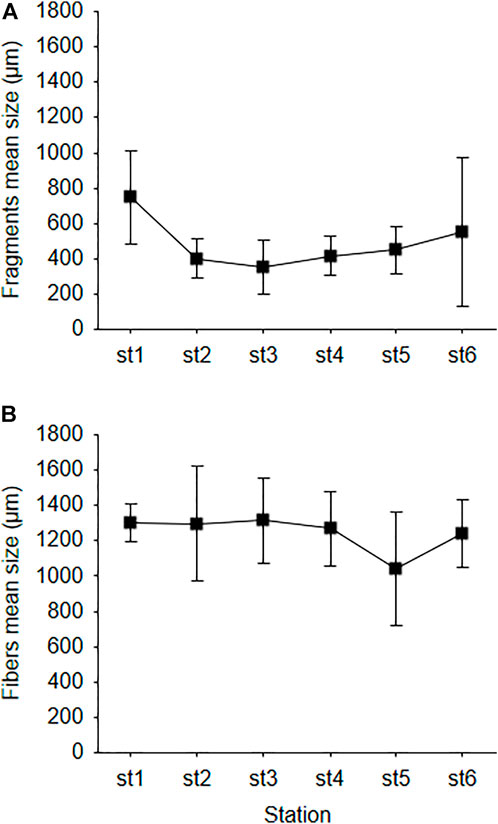
FIGURE 8. Spatial variation of fragments (µm; mean ± SD) (A) and fibers (µm; mean ± SD) (B) mean size.
Granulometric parameters and organic matter content
In what concerns sediment granulometry, significant differences were observed between stations (Pseudo-F = 13.44, P (perm) = 0.001; Figure 9A), but not among sampling campaigns (Pseudo-F = 0.33, P (perm) = 0.95). The prevalence of the 0.125–0.250 mm (fine sand) and 0.250–0.500 mm (medium sand) grain size fractions in stations 1, 4 and 5 largely contributed (with a 35 to 51 cumulative percentage range) to distinguish them from the other three stations. Yet, st4 (located at the fully protected area of the marine park) significantly differed from st5 (at Sesimbra bay) due to the lower representation of the 0.500–1 mm (coarse sand) size fraction. Conversely, although coarse sand was the predominant grain size fraction (Figure 9B) in stations 2, 3 and 6, the sediments collected from the mouth of the estuary (st2) and from the closest station to Portinho da Arrábida (st3) were considered distinct due to the significantly higher content of the 1–2 mm size fraction (very coarse sand) in st2. Lastly, despite the generally low representation of the fine grain fraction (silt/clay) in all stations, it was slightly higher in st1. Gradistat® categorized sediments from stations 1, 4 and 5 as medium sand, and the other three stations as coarse sand (following the Udden-Wentworth classification).
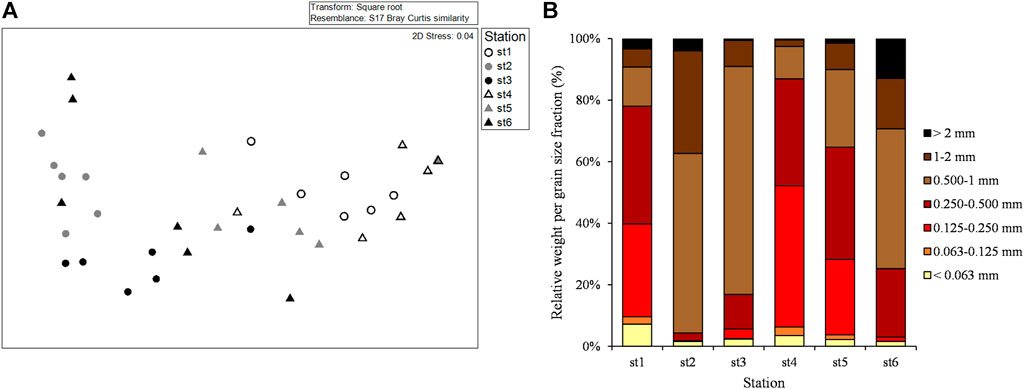
FIGURE 9. Multidimensional scaling plot based on the Bray-Curtis distance between samples of the different stations according to grain size fractions (A) and relative distribution of grain size fractions per station (B).
Mean grain size (H (5) = 25.40, p < .001), sediment sorting (H (5) = 25.78, p < .001) and organic matter content (F(5,25) = 5.42 p < 0.05) significantly differed between stations, but not between campaigns. Whereas mean grain size of both st1 (335.3 ± 131.4 µm; mean ± SD) and st4 (277.3 ± 99.7 µm) was considered distinct (p < 0.05) from st2 (918.7 ± 84.9 µm), only st4 differed significantly from st6 (863.2 ± 384.4 µm) (Figure 10A). The poorly sorted sediments in st1 (2.49 ± 0.28 µm) were significantly different from the moderately well sorted sediments from station 2 (1.60 ± 0.07 µm) and 3 (1.51 ± 0.19 µm) (p < 0.05; Figure 10B); also, sediment sorting at st3 was significantly distinct from st5 (1.96 ± 0.21 µm). The organic matter content of sediments at st1 (1.84 ± 0.88%) was significantly higher than st2 and st5 (p < 0.05; Figure 10C). Conversely, sediments of st2 had significantly lower organic matter content (0.57 ± 0.25%) than all the other stations (p < 0.05), except from st5. Significant correlations (positive, though moderate) were detected between MPs abundances and both organic matter content (rs (34) = .37, p = .028) and sorting (rs (34) = .43, p = .009), but no correlation was detected with mean grain size (rs (34) = -.32, p = .055).
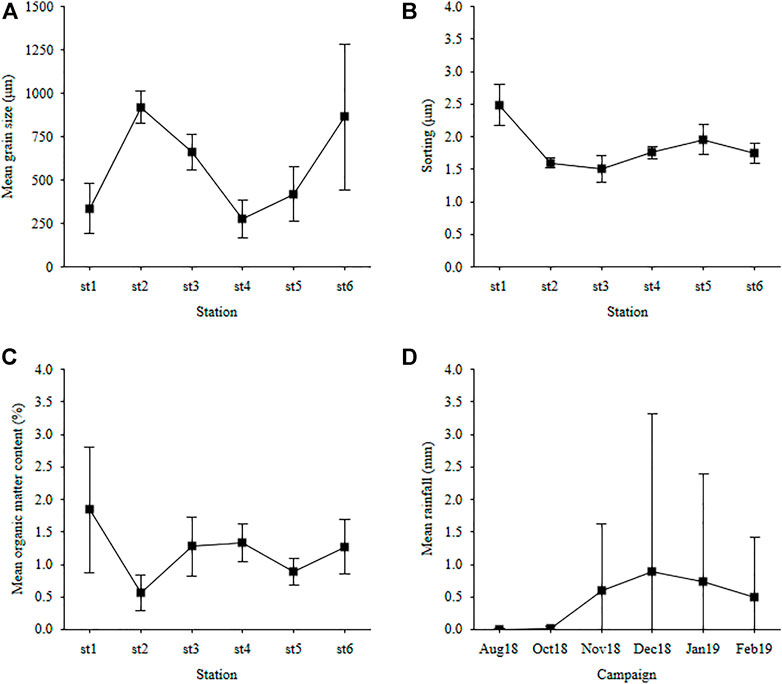
FIGURE 10. Spatial variation of (A) mean grain size (B) sediment sorting and (C) organic matter content. Temporal variation of rainfall (D). Data is provided as mean ± SD.
Rainfall
No relationship was observed between mean MPs abundance and rainfall (rs (4) = .26, p = .623), despite the significant differences found in rainfall among campaigns (H (5) = 102.50, p < .001; Figure 10D). Rainfall registered in August 18 and October 18 was significantly lower than that registered during all the other sampling campaigns (p < 0.001).
Mineralogical content
The mineral composition of sediment samples mainly consisted of quartz, calcite, and dolomite. Proportions of aragonite (assumed to have origin in seashells), potassium feldspars and halite were residual and relatively random, being therefore not considered for the calculation of the Carbonate/Siliciclastic index. Mineral proportions changed significantly among stations (H (5) = 28.11, p = .00; Table 4), but not between campaigns (H (5) = 0.67, p = .98). The index calculated for st2 (the lowest, due to the absence of carbonates) was significantly different from the index obtained for st4 and st6, where the carbonate proportions were considerably higher.
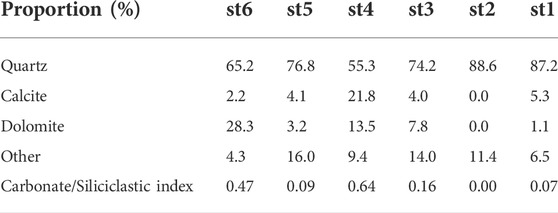
TABLE 4. Average proportion of each mineral and Carbonate/Siliciclastic index displayed per station. Stations were ordered from West to East.
Discussion
Abundance of MPs and comparison with other studies
Evident patterns were identified in the spatial distribution of MPs in subtidal sediments of the Portuguese west coast. While the Sado estuarine sediments presented extremely high abundances of MPs (1042.8 ± 430.8 items kg−1), marine sediments from the Arrábida Marine Park, in comparison, accumulated 20-fold less MPs (52.9 ± 31.9 items kg−1). This conspicuous difference, observed throughout the six monthly campaigns (as shown in Figure 2A), suggests a pronounced contribution from (i) the Sado river, which is expected to transport MPs resulting from the diverse industrial and artisanal fishing activities taking place in the Setúbal municipality (ca 123,000 inhabitants; according to INE Statistics Portugal, 20212), mostly at Sado north margin; (ii) the sewage and stormwater discharges into the Livramento stream, which joins the estuary at the st1 location; and (iii) the effluent of Setúbal WWTP (submarine outfall) located east of st1. Contrarily to the station located inside the estuary, all the others–though located at a sheltered coastline from the prevailing north and north-west winds by the Arrábida mountain chain (Henriques, 1999)–are ocean exposed. Such marine sediments are thus expected to face higher turbulence levels which prevent the accumulation of higher abundances of MPs, as observed inside the estuary. Our findings corroborate the classification of estuaries and urban coastal areas as MPs hotspots (Wright et al., 2013; Maes et al., 2017) and show how urgent is to implement local preventive measures that engage the different society sectors into the decrease of MPs inputs in this coastal environment.
Comparatively with other estuaries, the abundance of MPs found in Sado sediments was only lower than levels reported for Durban Bay in South Africa (111,933 ± 29,189 items kg−1; Preston-Whyte et al., 2021). However, it was higher than Densu delta in Ghana (4.0 ± 0.82 items per 10 g; Blankson et al., 2022), Warnow in Germany (379 ± 28 items kg−1 at the S10 station; Enders et al., 2019), Miri in Borneo Island (456.2 ± 33.6 items kg−1 in S5; Liong et al., 2021), Sebou in Morocco (187 items kg−1 in E1; Haddout et al., 2021) and both Dalio (ca 400 items kg−1; Xu et al., 2020) and Changjiang (where the higher abundance was ca 150 items kg−1; Peng et al., 2017) in China. Conversely, the mean abundance of MPs in marine sediments of the Arrábida coast is at an intermediate position, considering what has been reported from other marine coastal areas. While slightly lower values were found in the Polish zone of the Southern Baltic Sea (range: 0–27 particles kg−1; Graca et al., 2017), Algarve coast (10 ± 1 items kg−1; Frias et al., 2016) and Gdansk Bay (34 ± 10 items kg−1; Zobkov and Esiukova, 2017), there are reports of higher MPs pollution levels in marine sediments at the Galway Bay (73 items kg−1; Pagter et al., 2020), Park of Telaščica bay in Croacia (range: 32.3 ± 20.2 and 377.8 ± 18.8 items kg−1; Blašković et al., 2017), Belgian coast (91.9 ± 21.9 items kg−1 in BCS Coast (S1–S3); Claessens et al., 2011), Aeolian Archipelago, Italy (range: 151.0 ± 34.0 and 678.7 ± 345.8 items kg−1; Fastelli et al., 2016) and Southern North Sea (421 items kg−1; Maes et al., 2017). Even though it is assumed that local pollution sources and hydrodynamic conditions differ between the mentioned regions, such comparisons (only possible due to the harmonized reporting units) are important to provide the big picture of the addressed topic.
Distribution patterns according to types and sizes of MPs
Regarding the representativeness of MPs types, fragments were by far the most abundant one in the estuarine sediments (st1), in agreement with findings from other studies (Vianello et al., 2013; Talley et al., 2020; Haddout et al., 2021; Liong et al., 2021). Conversely, fibers were less abundant, but consistently spread throughout the six stations. Here it is important to highlight the potential for the fiber type to be commonly underestimated (Rummel et al., 2016) and therefore conceal serious scenarios, especially in sediments where this type of MPs is usually better represented (Martin et al., 2017; Marques Mendes et al., 2021). The considerable number of fibers discarded in this study (1603) for resembling those found in airborne contamination controls, may explain the reduced representation of this type of MPs in our study area. Even though consisting of a critical precaution step conducted before data analysis, this discarding process may wrongly exclude fibers which, despite being coincidently similar to those from controls, did not result from airborne contamination. Nevertheless, running this conservative step is preferable than to absolutely exclude all fibers from reports/studies. An additional explanation for the reduced number of fibers in this study was the potential loss of fibers that may have occurred onboard, during sampling, when the water excess of each sediment sample was discarded (mentioned in the Sampling methods section). This step was carried out because we could not assure this water source: if it was sediment pore water, bottom seawater adjacent to the sediment surface (“fluff layer”; Queirós et al., 2019)) or from the water column. Yet, despite such constraints, fibers were the prevalent type occurring in marine sediments, i.e. from st2 to st6, as reported elsewhere (Claessens et al., 2011; Frias et al., 2016; Graca et al., 2017; Martin et al., 2017; Zobkov and Esiukova, 2017; Pagter et al., 2020). This type of MPs can thus be considered the most available one for ingestion by marine benthic foragers occurring in this study area. Lastly, concerning all the other types of MPs (beads, glitter, films, filaments, fiber bundles), they were mainly found inside the estuary, similar to the accumulation pattern described for fragments.
Possibly, the occurrence of such distribution according to the type of MPs could be related with the higher surface area to volume ratio of fibers, in comparison to fragments and other irregular or voluminous types (Shin and Koch, 2005; Khatmullina and Isachenko, 2017; Pohl et al., 2020). As a result, due to a slower sinking process and easy resuspension from seabed, even with weak currents (Herzke et al., 2021), fibers end up being transported/deposited further away from their potential source - sewage and/or WWTP discharges. Although an inefficient retention of fibers was reported to occur in WWTP (Browne et al., 2011), recent studies have shown that wastewater treatment processes are indeed highly efficient (Mason et al., 2016; Murphy et al., 2016; Mintenig et al., 2017; Conley et al., 2019). However, the authors argue that the reduced amount of MPs being released per liter in the effluent is still substantial and, thus, should be considered as a significant source. In what concerns our study area, besides the sewage discharge located close to st1, there are two WWTP discharge points, one at each extremity (depicted in Figure 1), which may be contributing for fibers ubiquity among all stations. Regarding the marginally higher amount of fibers observed in st1, it may possibly result from the weak hydrodynamic conditions occurring at this location, comparatively with the ocean exposure at the Sesimbra WWTP outfall (located between st5 and st6), where MPs should easily disperse. Lastly, such evident spatial distribution patterns, influenced by MPs type, may be also determining the distribution of MPs according to their size. This interpretation is not only based on the absence of significant changes in the mean size of both fragments and fibers among stations, but also on the distinct size displayed by these two types of MPs. Fibers mean length was almost 3 times higher than mean fragments size (strongly related with fibers elongated shape). We therefore assume that the prevalence of smaller MPs in st1 (between 0.250 and 1 mm) was due to fragments high abundance in this station, while the prevalence of bigger MPs (between 0.500 and 2 mm) in all the other stations, resulted from fibers predominance.
Granulometric parameters and organic matter content
According to the granulometric profile of sediments, whereas stations 1, 4, and 5 could be considered as depositional areas (relatively protected, low energy environments) due to the predominance of smaller grain size fractions, the conversely larger grain size fractions prevailing in stations 2, 3 and 6, indicate higher energy environments (Kersten and Smedes, 2002). However, despite the potential for MPs to accumulate in stations 4 and 5, this was only verified in st1. Such strong retention of MPs inside the estuary may be greatly attributed to its reported slow flow rate (Vale et al., 1993; Cunha et al., 2007; Biguino et al., 2021) and to flocculation (Andersen et al., 2021; Laursen et al., 2022). The typical aggregation of suspended particulate matter in the estuary water column (Meade, 1972; Eisma, 1986; Manning and Dyer, 1999) enhanced by the mixture between freshwater and seawater, is suggested to transport MPs from the water surface into the estuarine sediments due to their incorporation in such flocs (Andersen et al., 2021; Laursen et al., 2022). Conversely, the abrupt decrease of MPs abundances at st2 may be eventually explained by the higher hydrodynamism occurring at the mouth of the Sado estuary (the interface with the Atlantic Ocean), preventing MPs entrapment in the sediment. Likewise, MPs at stations 3–6, may be under permanent resuspension into the water column, which consequently decrease their availability on the seabed (Näkki et al., 2019; Shamskhany et al., 2021). An additional explanation is that, eventually, MPs might get buried at the submerged ebb-tide delta (Costas et al., 2015) located at the estuary mouth south margin, preventing the exportation of higher MPs abundances to the Arrábida coast. However, as sampling in this study was only conducted at the north margin, further studies would be necessary to clarify this possibility.
Nonetheless, if plastic inputs in the ocean continue to increase as estimates predict (Jambeck et al., 2015; Geyer et al., 2017; Isobe et al., 2019), it is plausible to expect an increase of MPs accumulation in areas with potential for deposition, as described for st4 in particular. Therefore, and considering this station specific location, which is inside the fully protected area of the Arrábida Marine Park, we suggest its integration in a monitoring plan of MPs pollution, along with st1, as a preventive measure. Regarding st5, despite its deposition potential and the extreme proximity to Sesimbra town (even though it consists of a smaller municipality; ca. 52,000 inhabitants; according to INE Statistics Portugal, 20213), MPs accumulation was unexpectedly low. Here we hypothesize that st5 was not close enough to the Sesimbra submarine outfall in order to capture more realistic data about MPs inputs in this area. As mentioned before, this outfall, contrarily to the one inside the estuary (Setúbal WWTP), is located far from the shore, being exposed to higher hydrodynamic conditions (turbulence) that prevent the deposition of potentially emitted MPs. Nevertheless, the influence of Sesimbra WWTP effluent is not completely inexistent, considering the higher diversity of MPs types in st5, in comparison to st4 and st6 (Figure 6E), and the before mentioned absence of significant differences in fibers abundance among the six stations.
Despite the absence of a correlation between MPs abundances and sediment grain size, similarly to what was reported by Alomar et al. (2016), Fastelli et al. (2016), Blašković et al. (2017) and Coppock et al. (2021), it should be highlighted that in st1, both the majority of MPs and the dominant grain size fraction belonged to the 0.250–0.500 mm range. Further studies are thus needed to confirm such relationship, which could hence support the use of sediment grain size as a proxy for MPs size characterization inside the estuary. In what concerns grain sorting, and contrarily to what is reported by other studies (Zobkov and Esiukova, 2017), our findings suggest that this sediment feature may contribute to infer and identify areas with potential for MPs accumulation. In fact, sediments at st1 were both the most poorly sorted (i.e., the less calibrated sediments) and a MPs hotspot. Moreover, though only to some extent, the higher accumulation/entrapment of MPs observed in st1 (in comparison to all the other stations) may have possibly resulted from its subtly larger fine grain fraction (silt/clay; Figure 9B) and higher organic matter content (significantly higher than st2 and st5). These variables were expected to interfere with MPs accumulation due to the potential of fine grain fraction and organic matter to provide sediment cohesion (Shrestha and Blumberg, 2005) and to enhance particle aggregation (Maes et al., 2017). However, owing to the lack of stronger relations with these sediment characteristics, our data suggests that proximity to MPs sources and the local hydrodynamic conditions (slow flow rate in the estuary and ocean wave turbulence) are the main variables affecting MPs accumulation in sediments at our study area.
Meiofauna abundance and MPs to meiofauna ratios
Despite the unadvised use of grabs as a sampling method for meiofauna studies due to the bow-wave effects that disturb the sediment surface prior to sampling (Somerfield and Warwick, 2013), it should be highlighted that the selection of this method - Petite Ponar grab - was primarily based on MPs as the main target. Also, as such disturbance could be assumed to similarly interfere with the calculation of both MPs and meiofauna abundances, we may consider our MPs to meiofauna ratio patterns robust. As expected, meiofauna abundance was higher in st1 than in the other stations. This could be linked to the higher content of organic matter found inside Sado estuary (Sandulli et al., 2010), where hydrodynamic conditions are weak (inferred by the smaller mean grain size and more poorly sorted sediments). Also, since about 48% of MPs accumulated in the estuary (Figure 6F) overlap the size range of meiofauna organisms (between 63 and 500 μm; Giere, 2009), there is a high potential for MPs to be ingested by meiofauna predators in st1, either accidentally or intentionally. In what concerns the MPs to meiofauna ratio, a clear contrast was noticed between st1 and all the other stations, especially with st3. It reinforces the higher exposure of benthic feeders in the estuary, an ecosystem known to provide important habitats and nursery grounds (Beck et al., 2001; Sheaves et al., 2015) but also known to accumulate high concentrations of pollutants in sediments, as reported for Sado (Carvalho et al., 2009; Nunes et al., 2014; Ribeiro et al., 2016). The conversely lower ratios occurring between st2 and st6, suggesting fewer interactions, is explained by their low abundances of both MPs and meiofauna (at st6 in particular), which is potentially caused by the exposure of these stations to the predominant swell direction (NW; Mota and Pinto, 2014).
Rainfall
Regarding the assessment of temporal patterns in MPs abundance, although there was a slight decrease from October 2018 to February 2019, no significant changes were noticed. In fact, despite the significantly reduced rainfall observed during the two first sampling campaigns, no correlation was detected between rainfall and the monthly abundances of MPs here reported. This is contrary to the patterns reported by Rodrigues et al. (2020) regarding surface water samples collected in the same sampling stations, suggesting that rainfall (or stormwater runoff) mainly interferes with MPs abundances on the sea surface, or has at least causes a more immediate effect at this marine compartment. In fact, when low-density particles (known to ultimately contribute for the pool of MPs found in sediments) enter in the marine environment, they will not sink for several weeks, due to the lack of biofouling (Ye and Andrady, 1991; Lobelle and Cunliffe, 2011; Kaiser et al., 2017). Moreover, the lack of such relation in this study may be also related with an increase of the current velocity at the Sado estuary from late summer to February (Vale et al., 1993; Martins et al., 2002; Biguino et al., 2021) preventing a faster settling of the new MPs inputs in the system.
Polymer diversity
As observed in a preceding study focused on the MPs floating at the surface waters from this coastal area, the polymer diversity here determined was also high (11 different polymers), mirroring the multiple activities taking place, both on land (domestic, commercial, industrial and tourism) and at the sea/estuary (fishing, maritime recreational activities and intense traffic to shipyards). In fact, 6 of the 11 polymers here identified (PE, PS, PP, PUR, Copolymer PP/PE and PET) were also detected in the seawater surface samples, reflecting the widespread of several polymers in the water column. In addition to the potential links already suggested by Rodrigues et al. (2020), here others may be established between the identified polymers and the local sources/activities. For example, the PVAc may have the shipyard as a source, as this polymer is used in shipbuilding (Graca et al., 2017). Others may be released via wastewater as a result of laundry (Polyacrylates; textile fibers; Browne et al., 2011; Napper and Thompson, 2016), use of cosmetic and art/craft products (Polyacrylates and PVC; glitters; Yurtsever, 2019), use of pharmaceutical products for the treatment of hyperkalemia (PS-S; Wong et al., 2020; Rahman and Marathi, 2022) or use of water softening products (PS-S; Saleh, 2009). It should be highlighted that out of the 11 polymers, seven are denser than seawater (>1.02 g cm−3), namely PET, Polyacrylates, PVAc, PVC, PUR, PA and PS-S. Also, as reported elsewhere about other coastal sediments (Graca et al., 2017; Zheng et al., 2019), the predominant polymer collected in this study was PET, being mostly assigned to fibers and fiber bundles. Besides being widely are known to result from textile laundry (Browne et al., 2011; Napper and Thompson, 2016), they might also result from the degradation of fishing gear and maritime equipment (Murray and Cowie, 2011; Cole, 2016; OSPAR, 2020). The second most abundant polymer in our sediments was PE, which is in accordance with other studies (Zheng et al., 2019; Coppock et al., 2021) and is explained by its multiple applicability, high demand (PlasticsEurope, 2021) and fast discard (mainly packaging of consumer goods and single-use items). As mentioned before, despite these items tendency to float, biofouling processes will ultimately cause them to sink.
Mineralogical content
Lastly, in addition to the different organic matter contents and granulometric parameters found in sediments of each station, they also present distinct mineral composition which strongly mirrors the sediment sources occurring in the nearby shore. We could assume a weak transport of sediments along the shoreline or, at least, a strong influence of local sediment sources in the samples’ mineral composition. In fact, the higher proportion of carbonates in st4 and st6 coincided with their proximity to the Arrábida carbonated cliffs, while the higher siliciclastic (quartz) proportions found in the other stations are suggested to come from the adjacent sandy beaches (Figueirinha, st2, Portinho da Arrábida, st3, and Califórnia, st5) or from the Sado river (st1). The reduced transit of sediments between stations (throughout the six sampling campaigns) agrees with the reduced exportation of MPs from the estuary to the western marine coast, which contributes to the accumulation of MPs inside the estuary, i.e., in the vicinity of their emission sources.
Conclusion
The temporal fluctuation of MPs abundance in subtidal sediments at the Portuguese west coast, between late summer and winter (August 18 and February 19), was not significant and revealed no relationship with rainfall. However, a clear spatial distribution pattern was observed: MPs accumulation was high in the estuary (hotspot; mostly comprised by fragments) and low in all the other five stations (mostly represented by fibers). The high abundance of MPs in the estuary was moderately correlated with its poorly sorted sediments and with the high organic matter content. Also, besides possessing the higher silt/clay fraction, the size range of the majority of MPs inside the estuary was coincident with sediments most abundant grain size fraction: 0.250–0.500 mm.
The residual exportation of MP from the estuary is supported by the distinct mineralogical content of each station, which indicates a reduced transit of sediment along the coast. Conversely, the similar abundance of fibers among the six stations is potentially linked to the higher surface area to volume ratio of this type of MPs and to the location a WWTP outfall at each extremity of the study area. The MPs to meiofauna ratio was particularly higher in the estuary (1:15.3) suggesting a higher exposure level faced by biota. As expected, most polymers found in sediment were denser than seawater and may be linked to local activities.
Understanding patterns and identifying environmental factors capable of interfering with MPs accumulation in sediments are critical for the establishment of effective measures that aim to reduce and prevent plastic inputs to the marine environment. In marine protected areas, such information is especially important not only to evaluate their effectiveness in what concerns the protection of species and habitats from MPs pollution (Fastelli et al., 2016; Blašković et al., 2017), but also to adjust their management and/or monitoring plans accordingly. Therefore, continued research and local dissemination at awareness campaigns are necessary to engage citizens and stakeholders to tackle MPs pollution in this Portuguese coastal region.
Data availability statement
The raw data supporting the conclusions of this article will be made available by the authors, without undue reservation.
Author contributions
DR conducted fieldwork sampling, laboratory procedures (MPs extraction and characterization, sediment characterization), statistical analysis, and wrote the manuscript. JA performed FTIR analysis, collaborated in the discussion and selection of the best method for MPs extraction from sediment samples, provided assistance with laboratory procedures and at reporting FTIR analysis. JPa performed micro - FTIR analysis, assisted in the interpretation of spectra, and at reporting FTIR analysis and results. JPe compiled the rainfall data and gave important contributions to improve the manuscript. PSC provided assistance with sedimentological laboratory procedures and gave important contributions to improve the manuscript, namely about granulometry analysis, mineralogical content interpretations and XRD technique. FR conducted the XRD technique and assisted with mineralogical content interpretations. PS coordinated the study, discussed results, gave important contributions to the writing and to the English review of the text. MHC reviewed and made important contributions to the text.
Funding
This research was partially funded by National Geographic Society through an Early Career Grant (EC-397R-18), by Clear Reef Social Fund and by Fundação para a Ciência e a Tecnologia (FCT, Portugal) through the research project BASEMAN (JPIOCEANS/0001/2015), the strategic projects UIDB/04292/2020 granted to MARE and UIDB/04035/2020 granted to GeoBioTec and the project LA/P/0069/2020 granted to the Associate Laboratory ARNET. DR was supported by a Ph.D. grant from FCT (SFRH/BD/130652/2017).
Acknowledgments
The authors thank ICNF collaboration, in particular to M. Henriques, for providing patrol vessels as research platforms for sampling campaigns. We also thank park rangers C. Silva and A. Silveira, and several volunteers, especially M. D'Ambrosio, for all the assistance during fieldwork. We are also grateful to CCMAR Scientific Dive Centre for lending their boat for the August sampling campaign. We acknowledge Instituto Hidrográfico for granting access to µ-FTIR equipment and for providing the isobaths data presented in the map of Figure 1 which was developed with R. Cândido assistance. Our gratitude also goes to Eduardo Jobling, for assembling the SMI units, and to the student A. Dyussenbayeva for collaboration on the MPs extraction procedures.
Conflict of interest
The authors declare that the research was conducted in the absence of any commercial or financial relationships that could be construed as a potential conflict of interest.
Publisher’s note
All claims expressed in this article are solely those of the authors and do not necessarily represent those of their affiliated organizations, or those of the publisher, the editors and the reviewers. Any product that may be evaluated in this article, or claim that may be made by its manufacturer, is not guaranteed or endorsed by the publisher.
Footnotes
1https://snirh.apambiente.pt/index.php?idMain=
2https://www.ine.pt/scripts/db_censos_2021.html
3https://www.ine.pt/scripts/db_censos_2021.html
References
Alomar, C., Estarellas, F., and Deudero, S. (2016). Microplastics in the Mediterranean Sea: Deposition in coastal shallow sediments, spatial variation and preferential grain size. Mar. Environ. Res. 115, 1–10. doi:10.1016/j.marenvres.2016.01.005
Andersen, T. J., Rominikan, S., Olsen, I. S., Skinnebach, K. H., and Fruergaard, M. (2021). Flocculation of PVC microplastic and fine-grained cohesive sediment at environmentally realistic concentrations. Biol. Bull. 240, 42–51. doi:10.1086/712929
Andrady, A. L. (2011). Microplastics in the marine environment. Mar. Pollut. Bull. 62, 1596–1605. doi:10.1016/j.marpolbul.2011.05.030
Arshad, S. N., Naraghi, M., and Chasiotis, I. (2011). Strong carbon nanofibers from electrospun polyacrylonitrile. Carbon N. Y. 49, 1710–1719. doi:10.1016/j.carbon.2010.12.056
Bakir, A., Rowland, S. J., and Thompson, R. C. (2014). Transport of persistent organic pollutants by microplastics in estuarine conditions. Estuar. Coast. Shelf Sci. 140, 14–21. doi:10.1016/j.ecss.2014.01.004
Barnes, D. K. A., Galgani, F., Thompson, R. C., and Barlaz, M. (2009). Accumulation and fragmentation of plastic debris in global environments. Phil. Trans. R. Soc. B 364, 1985–1998. doi:10.1098/rstb.2008.0205
Beck, M. W., Heck, K. L., Able, K. W., Childers, D. L., Eggleston, D. B., Gillanders, B. M., et al. (2001). The identification, conservation, and management of estuarine and marine nurseries for fish and invertebrates, Bioscience 51, 6332–6641. doi:10.1641/0006-3568(2001)051
Bellanova, P., Feist, L., Costa, P. J. M., Orywol, S., Reicherter, K., Lehmkuhl, F., et al. (2022). Contemporary pollution of surface sediments from the Algarve shelf, Portugal. Mar. Pollut. Bull. 176, 113410. doi:10.1016/j.marpolbul.2022.113410
Bellas, J., Martínez-Armental, J., Martínez-Cámara, A., Besada, V., and Martínez-Gómez, C. (2016). Ingestion of microplastics by demersal fish from the Spanish Atlantic and Mediterranean coasts. Mar. Pollut. Bull. 109, 55–60. doi:10.1016/j.marpolbul.2016.06.026
Biguino, B., Sousa, F., and Brito, A. C. (2021). Variability of currents and water column structure in a temperate estuarine system (Sado estuary, Portugal). Water 13, 187. doi:10.3390/w13020187
Bingel, F., Avsar, D., and Ünsal, M. (1987). A note on plastic materials in trawl catches in the North-Eastern Mediterranean. Meeresforsch. - Rep. Mar. Res. 31, 227
Blankson, E. R., Tetteh, P. N., Oppong, P., and Gbogbo, F. (2022). Microplastics prevalence in water, sediment and two economically important species of fish in an urban riverine system in Ghana. PLoS One 17, e0263196. doi:10.1371/journal.pone.0263196
Blašković, A., Fastelli, P., Čižmek, H., Guerranti, C., and Renzi, M. (2017). Plastic litter in sediments from the Croatian marine protected area of the natural park of Telaščica bay (Adriatic Sea). Mar. Pollut. Bull. 114, 583–586. doi:10.1016/j.marpolbul.2016.09.018
Bour, A., Avio, C. G., Gorbi, S., Regoli, F., and Hylland, K. (2018). Presence of microplastics in benthic and epibenthic organisms: Influence of habitat, feeding mode and trophic level. Environ. Pollut. 243, 1217–1225. doi:10.1016/j.envpol.2018.09.115
Brindley, G. W., and Brown, G. (1980). Crystal structures of clay minerals and their X. London: Ray Identification.
Browne, M. A., Crump, P., Niven, S. J., Teuten, E., Tonkin, A., Galloway, T., et al. (2011). Accumulation of microplastic on shorelines woldwide: Sources and sinks. Environ. Sci. Technol. 45, 9175–9179. doi:10.1021/es201811s
Burns, E. E., and Boxall, A. B. A. (2018). Microplastics in the aquatic environment: Evidence for or against adverse impacts and major knowledge gaps. Environ. Toxicol. Chem. 37, 2776–2796. doi:10.1002/etc.4268
Cambardella, C. A., Gajda, A. M., Doran, J. W., Wienhold, B. J., and Kettler, T. (2001). “Estimation of particulate and total organic matter by weight loss-on-ignition,” in Assessment methods for soil carbon. Editors R. Lal, J. M. Kimble, R. F. Follett, and B. A. Stewart (Boca Raton: CRC Press), 349
Carvalho, P. N., Rodrigues, P. N. R., Basto, M. C. P., and Vasconcelos, M. T. S. D. (2009). Organochlorine pesticides levels in Portuguese coastal areas. Chemosphere 75, 595–600. doi:10.1016/j.chemosphere.2009.01.060
Castro, O. G., and Vale, C. (1995). Total PCB-organic matter correlation in sediments from three estuarine areas of Portugal. Neth. J. Aquatic Ecol. 29, 297–302. doi:10.1007/BF02084228
Claessens, M., Meester, S., and Janssen, C. R. (2011). Occurrence and distribution of microplastics in marine sediments along the Belgian coast. Mar. Pollut. Bull. 62, 2199–2204. doi:10.1016/j.marpolbul.2011.06.030
Claessens, M., Van Cauwenberghe, L., Vandegehuchte, M. B., and Janssen, C. R. (2013). New techniques for the detection of microplastics in sediments and field collected organisms. Mar. Pollut. Bull. 70, 227–233. doi:10.1016/j.marpolbul.2013.03.009
Clarke, K. R., and Gorley, R. N. (2006). PRIMER v6: User manual/tutorial. R. N. Clarke, and K. R. Gorley (Plymouth, United Kingdom: PRIMER-E Ltd.).
Cole, M. (2016). A novel method for preparing microplastic fibers. Sci. Rep. 6, 34519. doi:10.1038/srep34519
Cole, M., Lindeque, P. K., Fileman, E., Clark, J., Lewis, C., Halsband, C., et al. (2016). Microplastics alter the properties and sinking rates of zooplankton faecal pellets. Environ. Sci. Technol. 50, 3239–3246. doi:10.1021/acs.est.5b05905
Conley, K., Clum, A., Deepe, J., Lane, H., and Beckingham, B. (2019). Wastewater treatment plants as a source of microplastics to an urban estuary: Removal efficiencies and loading per capita over one year. Water Res. X 3, 100030. doi:10.1016/j.wroa.2019.100030
Coppock, R. L., Cole, M., Lindeque, P. K., Queirós, A. M., and Galloway, T. S. (2017). A small-scale, portable method for extracting microplastics from marine sediments. Environ. Pollut. 230, 829–837. doi:10.1016/j.envpol.2017.07.017
Coppock, R. L., Galloway, T. S., Cole, M., Fileman, E. S., Queirós, A. M., and Lindeque, P. K. (2019). Microplastics alter feeding selectivity and faecal density in the copepod, Calanus helgolandicus. Sci. Total Environ. 687, 780–789. doi:10.1016/j.scitotenv.2019.06.009
Coppock, R. L., Lindeque, P. K., Cole, M., Galloway, T. S., Näkki, P., Birgani, H., et al. (2021). Benthic fauna contribute to microplastic sequestration in coastal sediments. J. Hazard. Mat. 415, 125583. doi:10.1016/j.jhazmat.2021.125583
Costas, S., Rebêlo, L., Brito, P., Burbidge, C. I., and PrudêncioMaria Isabel FitzGerald, D. (2015). “The joint history of Tr?oia peninsula and Sado ebb-delta,” in Sand and gravel spits. Editors C. J. G. Randazzo, and D. Jackson (Cham: Springer), 79–102. doi:10.1007/978-3-319-13716-2
Cozar, A., Echevarria, F., Gonzalez-Gordillo, J. I., Irigoien, X., Ubeda, B., Hernandez-Leon, S., et al. (2014). Plastic debris in the open ocean. Proc. Natl. Acad. Sci. U. S. A. 111, 10239–10244. doi:10.1073/pnas.1314705111
Cunha, I., Neuparth, T., Caeiro, S., Costa, M. H., and Guilhermino, L. (2007). Toxicity ranking of estuarine sediments on the basis of sparus aurata biomarkers. Environ. Toxicol. Chem. 26, 444–453. doi:10.1897/06-119R.1
Eisma, D. (1986). Flocculation and de-flocculation of suspended matter in estuaries. Neth. J. Sea Res. 20, 183–199. doi:10.1016/0077-7579(86)90041-4
Enders, K., Käppler, A., Biniasch, O., Feldens, P., Stollberg, N., Lange, X., et al. (2019). Tracing microplastics in aquatic environments based on sediment analogies. Sci. Rep. 9, 15207–15215. doi:10.1038/s41598-019-50508-2
Eriksen, M., Lebreton, L. C. M., Carson, H. S., Thiel, M., Moore, C. J., Borerro, J. C., et al. (2014). Plastic pollution in the world’s oceans: More than 5 trillion plastic pieces weighing over 250, 000 tons afloat at sea. PLoS One 9, e111913–15. doi:10.1371/journal.pone.0111913
Fastelli, P., Blašković, A., Bernardi, G., Romeo, T., Čižmek, H., Andaloro, F., et al. (2016). Plastic litter in sediments from a marine area likely to become protected (Aeolian Archipelago’s islands, Tyrrhenian sea). Mar. Pollut. Bull. 113, 526–529. doi:10.1016/j.marpolbul.2016.08.054
Fendall, L. S., and Sewell, M. A. (2009). Contributing to marine pollution by washing your face: Microplastics in facial cleansers. Mar. Pollut. Bull. 58, 1225–1228. doi:10.1016/j.marpolbul.2009.04.025
Folk, R. L., and Ward, W. C. (1957). Brazos river bar: A study in the significance of grain size parameters. J. Sediment. Res. 27, 3. doi:10.1306/74D70646-2B21-11D7-8648000102C1865D
Frias, J., Pagter, E., Nash, R., O’Connor, I., Carretero, O., Filgueiras, A., et al. (2018). Standardised protocol for monitoring microplastics in sediments. doi:10.13140/RG.2.2.36256.89601/1
Frias, J. P. G. L., Gago, J., Otero, V., and Sobral, P. (2016). Microplastics in coastal sediments from Southern Portuguese shelf waters. Mar. Environ. Res. 114, 24–30. doi:10.1016/j.marenvres.2015.12.006
Galgani, F., Burgeot, T., Bocquéné, G., Vincent, F., Leauté, J. P., Labastie, J., et al. (1995a). Distribution and abundance of debris on the continental shelf of the bay of biscay and in seine bay. Mar. Pollut. Bull. 30, 58–62. doi:10.1016/0025-326X(94)00101-E
Galgani, F., Jaunet, S., Campillo, A., Guenegen, X., and His, E. (1995b). Distribution and abundance of debris on the continental shelf of the north-western Mediterranean Sea. Mar. Pollut. Bull. 30, 713–717. doi:10.1016/0025-326X(95)00055-R
Galgani, F., Leaute, J. ., Moguedet, P., Souplet, A., Verin, Y., Carpentier, A., et al. (2000). Litter on the sea floor along European coasts. Mar. Pollut. Bull. 40, 516–527. doi:10.1016/S0025-326X(99)00234-9
Geyer, R., Jambeck, J. R., and Law, K. L. (2017). Production, use, and fate of all plastics ever made. Sci. Adv. 3, e1700782. doi:10.1126/sciadv.1700782
Giere, O. (2009). Meiobenthology. The microscopic motile fauna of aquatic sediments. Germanny: Springer Available at: http://www.tandfonline.com/doi/abs/10.1080/11250000903476616.
Graca, B., Szewc, K., Zakrzewska, D., Dołęga, A., and Szczerbowska-Boruchowska, M. (2017). Sources and fate of microplastics in marine and beach sediments of the southern Baltic Sea—A preliminary study. Environ. Sci. Pollut. Res. 24, 7650–7661. doi:10.1007/s11356-017-8419-5
Graham, E. R., and Thompson, J. T. (2009). Deposit- and suspension-feeding sea cucumbers (Echinodermata) ingest plastic fragments. J. Exp. Mar. Biol. Ecol. 368, 22–29. doi:10.1016/j.jembe.2008.09.007
Gregory, M. R., and Andrady, A. L. (2005). “Plastics in the marine environment,” in Plastics and the environment. Editor A. L. Andrady (New Jersey, United States: John Wiley & Sons), 379–401. doi:10.1002/0471721557.ch10
Gregory, M. R. (1996). Plastic ‘scrubbers’ in hand cleansers: A further (and minor) source for marine pollution identified. Mar. Pollut. Bull. 32, 867–871. doi:10.1016/S0025-326X(96)00047-1
Gusmão, F., DomenicoDiAmaral, M. A. C. Z., Martínez, A., Gonzalez, B. C., Worsaae, K., et al. (2016). In situ ingestion of microfibres by meiofauna from sandy beaches. Environ. Pollut. 216, 584–590. doi:10.1016/j.envpol.2016.06.015
Haddout, S., Gimiliani, G. T., Priya, K. L., Hoguane, A. M., Casila, J. C. C., and Ljubenkov, I. (2021). Microplastics in surface waters and sediments in the Sebou estuary and atlantic coast, Morocco. Anal. Lett. 0, 256–268. doi:10.1080/00032719.2021.1924767
Herzke, D., Ghaffari, P., Sundet, J. H., Tranang, C. A., and Halsband, C. (2021). Microplastic fiber emissions from wastewater effluents: Abundance, transport behavior and exposure risk for biota in an arctic fjord. Front. Environ. Sci. 9, 1–14. doi:10.3389/fenvs.2021.662168
Hess, N. A., Ribic, C. A., and Vining, I. (1999). Benthic marine debris, with an emphasis on fishery-related items, surrounding kodiak Island, Alaska, 1994–1996. Mar. Pollut. Bull. 38, 885–890. doi:10.1016/S0025-326X(99)00087-9
Holmström, A. (1975). Plastic films on the bottom of the Skagerack. Nature 255, 622–623. doi:10.1038/255622a0
Hummel, D. O. (2002). Atlas of plastics additives: Analysis by spectrometric methods. Berlin: Springer-Verlag Berlin Heidelberg. doi:10.1007/978-3-642-56211-2
Imhof, H. K., Schmid, J., Niessner, R., Ivleva, N. P., and Laforsch, C. (2012). A novel, highly efficient method for the separation and quantification of plastic particles in sediments of aquatic environments. Limnol. Oceanogr. Methods 10, 524–537. doi:10.4319/lom.2012.10.524
Isobe, A., Iwasaki, S., Uchida, K., and Tokai, T. (2019). Abundance of non-conservative microplastics in the upper ocean from 1957 to 2066. Nat. Commun. 10, 417–513. doi:10.1038/s41467-019-08316-9
Jambeck, J. R., Geyer, R., Wilcox, C., Siegler, T. R., Perryman, M., Andrady, A., et al. (2015). Plastic waste inputs from land into the ocean. Science 347, 768–771. doi:10.1126/science.1260352
Jung, M. R., Horgen, F. D., Orski, S. V., Rodriguez, C., V., Beers, K. L., Balazs, G. H., et al. (2018). Validation of ATR FT-IR to identify polymers of plastic marine debris, including those ingested by marine organisms. Mar. Pollut. Bull. 127, 704–716. doi:10.1016/j.marpolbul.2017.12.061
Kaiser, D., Kowalski, N., and Waniek, J. J. (2017). Effects of biofouling on the sinking behavior of microplastics. Environ. Res. Lett. 12, 124003. doi:10.1088/1748-9326/aa8e8b
Kanehiro, H., Tokai, T., and Matuda, K. (1995). Marine litter composition and distribution on the sea-bed of tokyo bay. Fish. Eng. 31, 195–199. doi:10.18903/fisheng.31.3_195
Kaur, A., and Fanourakis, G. C. (2016). The effect of type, concentration and volume of dispersing agent on the magnitude of the clay content determined by the hydrometer analysis. J. S. Afr. Inst. Civ. Eng. 58, 48–54. doi:10.17159/2309-8775/2016/v58n4a5
Kausar, A. (2015). Fabrication and characteristics of poly(benzimidazole/fluoro/ether/siloxane/amide)/sulfonated polystyrene/silica nanoparticle-based proton exchange membranes doped with phosphoric acid. Int. J. Polym. Mater. Polym. Biomaterials 64, 184–191. doi:10.1080/00914037.2014.936589
Kersten, M., and Smedes, F. (2002). Normalization procedures for sediment contaminants in spatial and temporal trend monitoring. J. Environ. Monit. 4, 109–115. doi:10.1039/b108102k
Khatmullina, L., and Isachenko, I. (2017). Settling velocity of microplastic particles of regular shapes. Mar. Pollut. Bull. 114, 871–880. doi:10.1016/j.marpolbul.2016.11.024
Lacorte, S., Guillamón, M., Martínez, E., Viana, P., and Barceló, D. (2003). Occurrence and specific congener profile of 40 polybrominated diphenyl ethers in river and coastal sediments from Portugal. Environ. Sci. Technol. 37, 892–898. doi:10.1021/es020839+
Laursen, S. N., Fruergaard, M., and Andersen, T. J. (2022). Rapid flocculation and settling of positively buoyant microplastic and fine-grained sediment in natural seawater. Mar. Pollut. Bull. 178, 113619. doi:10.1016/j.marpolbul.2022.113619
Lenz, R., Enders, K., and Nielsen, T. G. (2016). Microplastic exposure studies should be environmentally realistic. Proc. Natl. Acad. Sci. U. S. A. 113, E4121–E4122. doi:10.1073/pnas.1606615113
Lindeque, P. K., Cole, M., Coppock, R. L., Lewis, C. N., Miller, R. Z., Watts, A. J. R., et al. (2020). Are we underestimating microplastic abundance in the marine environment? A comparison of microplastic capture with nets of different mesh-size. Environ. Pollut. 265, 114721. doi:10.1016/j.envpol.2020.114721
Liong, R. M. Y., Hadibarata, T., Yuniarto, A., Tang, K. H. D., and Khamidun, M. H. (2021). Microplastic occurrence in the water and sediment of Miri river estuary, Borneo Island. Water. Air. Water Air Soil Pollut. 232, 342. doi:10.1007/s11270-021-05297-8
Lobelle, D., and Cunliffe, M. (2011). Early microbial biofilm formation on marine plastic debris. Mar. Pollut. Bull. 62, 197–200. doi:10.1016/j.marpolbul.2010.10.013
Lusher, A. L., Burke, A., O’Connor, I., and Officer, R. (2014). Microplastic pollution in the northeast Atlantic Ocean: Validated and opportunistic sampling. Mar. Pollut. Bull. 88, 325–333. doi:10.1016/j.marpolbul.2014.08.023
Lusher, A. L., McHugh, M., and Thompson, R. C. (2013). Occurrence of microplastics in the gastrointestinal tract of pelagic and demersal fish from the English Channel. Mar. Pollut. Bull. 67, 94–99. doi:10.1016/j.marpolbul.2012.11.028
Lusher, A. L., Welden, N. A., Sobral, P., and Cole, M. (2017). Sampling, isolating and identifying microplastics ingested by fish and invertebrates. Anal. Methods 9, 1346–1360. doi:10.1039/C6AY02415G
Maes, T., Van der Meulen, M. D., Devriese, L. I., Leslie, H. A., Huvet, A., Frère, L., et al. (2017). Microplastics baseline surveys at the water surface and in sediments of the north-east atlantic. Front. Mar. Sci. 4, 1–13. doi:10.3389/fmars.2017.00135
Manning, A. J., and Dyer, K. R. (1999). A laboratory examination of floc characteristics with regard to turbulent shearing. Mar. Geol. 160, 147–170. doi:10.1016/S0025-3227(99)00013-4
Marković, G., Marinović-Cincović, M., Vodnik, V., Radovanović, B., Budinski-Simendić, J., and Veljković, O. (2009). Thermal stability of acrylonitrile/chlorosulphonated polyethylene rubber blend. J. Therm. Anal. Calorim. 97, 999–1006. doi:10.1007/s10973-009-0162-9
Marques Mendes, A., Golden, N., Bermejo, R., and Morrison, L. (2021). Distribution and abundance of microplastics in coastal sediments depends on grain size and distance from sources. Mar. Pollut. Bull. 172, 112802. doi:10.1016/j.marpolbul.2021.112802
Martin, J., Lusher, A., Thompson, R. C., and Morley, A. (2017). The deposition and accumulation of microplastics in marine sediments and bottom water from the Irish continental shelf. Sci. Rep. 7, 10772. doi:10.1038/s41598-017-11079-2
Martins, F., Leitão, P., and Neves, R. (2002). Simulating vertical water mixing in homogeneous estuaries: The Sado Estuary case. Hydrobiologia 475/476, 221–227. doi:10.1023/A:1020369431924
Mason, S. A., Garneau, D., Sutton, R., Chu, Y., Ehmann, K., Barnes, J., et al. (2016). Microplastic pollution is widely detected in US municipal wastewater treatment plant effluent. Environ. Pollut. 218, 1045–1054. doi:10.1016/j.envpol.2016.08.056
McCormick, A., Hoellein, T. J., Mason, S. A., Schluep, J., and Kelly, J. J. (2014). Microplastic is an abundant and distinct microbial habitat in an urban river. Environ. Sci. Technol. 48, 11863–11871. doi:10.1021/es503610r
Meade, R. H. (1972). “Transport and deposition of sediments in estuaries,” in Environmental framework of coastal plain estuaries. Editor B. W. Nelson (USA: The Geological Cociety of America), 91–120. doi:10.1130/MEM133-p91
Mellinger, R. M. (1979). Quantitative X-ray diffraction analysis of clay minerals. Eval. SRC Rep. G 79, 1
Mintenig, S. M., Int-Veen, I., Löder, M. G. J., Primpke, S., and Gerdts, G. (2017). Identification of microplastic in effluents of waste water treatment plants using focal plane array-based micro-Fourier-transform infrared imaging. Water Res. 108, 365–372. doi:10.1016/j.watres.2016.11.015
Mota, P., and Pinto, J. P. (2014). Wave energy potential along the Western Portuguese coast. Renew. Energy 71, 8–17. doi:10.1016/j.renene.2014.02.039
Murphy, F., Ewins, C., Carbonnier, F., and Quinn, B. (2016). Wastewater treatment works (WwTW) as a source of microplastics in the aquatic environment. Environ. Sci. Technol. 50, 5800–5808. doi:10.1021/acs.est.5b05416
Murray, F., and Cowie, P. R. (2011). Plastic contamination in the decapod crustacean Nephrops norvegicus (Linnaeus, 1758). Mar. Pollut. Bull. 62, 1207–1217. doi:10.1016/j.marpolbul.2011.03.032
Näkki, P., Setälä, O., and Lehtiniemi, M. (2019). Seafloor sediments as microplastic sinks in the northern Baltic Sea – negligible upward transport of buried microplastics by bioturbation. Environ. Pollut. 249, 74–81. doi:10.1016/j.envpol.2019.02.099
Napper, I. E., and Thompson, R. C. (2016). Release of synthetic microplastic plastic fibres from domestic washing machines: Effects of fabric type and washing conditions. Mar. Pollut. Bull. 112, 39–45. doi:10.1016/j.marpolbul.2016.09.025
Nel, H., Krause, S., Sambrook Smith, G. H., and Lynch, I. (2019). Simple yet effective modifications to the operation of the Sediment Isolation Microplastic unit to avoid polyvinyl chloride (PVC) contamination. MethodsX 6, 2656–2661. doi:10.1016/j.mex.2019.11.007
Nuelle, M.-T., Dekiff, J. H., Remy, D., and Fries, E. (2014). A new analytical approach for monitoring microplastics in marine sediments. Environ. Pollut. 184, 161–169. doi:10.1016/j.envpol.2013.07.027
Nunes, M., Vernisseau, A., Marchand, P., Le Bizec, B., Ramos, F., and Pardal, M. A. (2014). Occurrence of PCDD/Fs and dioxin-like PCBs in superficial sediment of Portuguese estuaries. Environ. Sci. Pollut. Res. 21, 9396–9407. doi:10.1007/s11356-014-2891-y
OSPAR (2020). OSPAR scoping study on best practices for the design and recycling of fishing gear as a means to reduce quantities of fishing gear found as marine litter in the North-East Atlantic. London, United Kingdom.
Pagter, E., Frias, J., Kavanagh, F., and Nash, R. (2020). Varying levels of microplastics in benthic sediments within a shallow coastal embayment. Estuar. Coast. Shelf Sci. 243, 106915. doi:10.1016/j.ecss.2020.106915
Pagter, E., Frias, J., and Nash, R. (2018). Microplastics in Galway bay: A comparison of sampling and separation methods. Mar. Pollut. Bull. 135, 932–940. doi:10.1016/j.marpolbul.2018.08.013
Pegram, J. E., and Andrady, A. L. (1989). Outdoor weathering of selected polymeric materials under marine exposure conditions. Polym. Degrad. Stab. 26, 333–345. doi:10.1016/0141-3910(89)90112-2
Peng, G., Zhu, B., Yang, D., Su, L., Shi, H., and Li, D. (2017). Microplastics in sediments of the Changjiang Estuary, China. Environ. Pollut. 225, 283–290. doi:10.1016/j.envpol.2016.12.064
Pequeno, J., Antunes, J., Dhimmer, V., Bessa, F., and Sobral, P. (2021). Microplastics in marine and estuarine species from the coast of Portugal. Front. Environ. Sci. 9, 1–12. doi:10.3389/fenvs.2021.579127
Pevear, D. R., and Mumpton, D. (1989). Quantitative mineral analysis of clays. Editors D. R. Pevear, and D. Mumpton (USA: The Clay Minerals Society).
Phuong, N. N., Zalouk-Vergnoux, A., Poirier, L., Kamari, A., Châtel, A., Mouneyrac, C., et al. (2016). Is there any consistency between the microplastics found in the field and those used in laboratory experiments? Environ. Pollut. 211, 111–123. doi:10.1016/j.envpol.2015.12.035
Piñon-Colin, T. de J., Rodriguez-Jimenez, R., Rogel-Hernandez, E., Alvarez-Andrade, A., and Wakida, F. T. (2020). Microplastics in stormwater runoff in a semiarid region, Tijuana, Mexico. Sci. Total Environ. 704, 135411. doi:10.1016/j.scitotenv.2019.135411
PlasticsEurope (2021). Plastics - the Facts 2021. An analysis of European plastics production, demand and waste data.
Pohl, F., Eggenhuisen, J. T., Kane, I. A., and Clare, M. A. (2020). Transport and burial of microplastics in deep-marine sediments by turbidity currents. Environ. Sci. Technol. 54, 4180–4189. doi:10.1021/acs.est.9b07527
Porter, A., Lyons, B. P., Galloway, T. S., and Lewis, C. (2018). Role of marine snows in microplastic fate and bioavailability. Environ. Sci. Technol. 52, 7111–7119. doi:10.1021/acs.est.8b01000
Preston-Whyte, F., Silburn, B., Meakins, B., Bakir, A., Pillay, K., Worship, M., et al. (2021). Meso- and microplastics monitoring in harbour environments: A case study for the port of durban, south Africa. Mar. Pollut. Bull. 163, 111948. doi:10.1016/j.marpolbul.2020.111948
Primpke, S., Wirth, M., Lorenz, C., and Gerdts, G. (2018). Reference database design for the automated analysis of microplastic samples based on Fourier transform infrared (FTIR) spectroscopy. Anal. Bioanal. Chem. 410, 5131–5141. doi:10.1007/s00216-018-1156-x
Queirós, A. M., Stephens, N., Widdicombe, S., Tait, K., McCoy, S. J., Ingels, J., et al. (2019). Connected macroalgal‐sediment systems: Blue carbon and food webs in the deep coastal ocean. Ecol. Monogr. 89, 1–21. doi:10.1002/ecm.1366
Rahman, S., and Marathi, R. (2022). Sodium polystyrene sulfonate. StatPearls [Internet] Treasure Isl. StatPearls Publ. Available at: https://www.ncbi.nlm.nih.gov/books/NBK559206/(Accessed August 8, 2022).
Ribeiro, C., Ribeiro, A. R., and Tiritan, M. E. (2016). Occurrence of persistent organic pollutants in sediments and biota from Portugal versus European incidence: A critical overview. J. Environ. Sci. Health Part B 51, 143–153. doi:10.1080/03601234.2015.1108793
Rochman, C. M., and Boxallz, A. B. (2014). Environmental relevance: A necessary component of experimental design to answer the question, “so what?”. Integr. Environ. Assess. Manag. 10, 311–312. doi:10.1002/ieam.1515
Rochman, C. M., Brookson, C., Bikker, J., Djuric, N., Earn, A., Bucci, K., et al. (2019). Rethinking microplastics as a diverse contaminant suite. Environ. Toxicol. Chem. 38, 703–711. doi:10.1002/etc.4371
Rochman, C. M., Regan, F., and Thompson, R. C. (2017). On the harmonization of methods for measuring the occurrence, fate and effects of microplastics. Anal. Methods 9, 1324–1325. doi:10.1039/C7AY90014G
Rodrigues, D., Antunes, J., Otero, V., Sobral, P., and Costa, M. H. (2020). Distribution patterns of microplastics in seawater surface at a Portuguese estuary and marine park. Front. Environ. Sci. 8, 1–15. doi:10.3389/fenvs.2020.582217
Rummel, C. D., Löder, M. G. J., Fricke, N. F., Lang, T., Griebeler, E. M., Janke, M., et al. (2016). Plastic ingestion by pelagic and demersal fish from the north Sea and Baltic Sea. Mar. Pollut. Bull. 102, 134–141. doi:10.1016/j.marpolbul.2015.11.043
Ryan, P. G., Moore, C. J., van Franeker, J. A., and Moloney, C. L. (2009). Monitoring the abundance of plastic debris in the marine environment. Phil. Trans. R. Soc. B 364, 1999. doi:10.1098/rstb.2008.0207
Saleh, M. M. (2009). Water softening using packed bed of polypyrrole from flowing solutions. Desalination 235, 319–329. doi:10.1016/j.desal.2008.02.011
Sandulli, R., De Leonardis, C., and Vanaverbeke, J. (2010). Meiobenthic communities in the shallow subtidal of three Italian Marine Protected Areas. Ital. J. Zool. (Modena). 77, 186–196. doi:10.1080/11250000903476616
Schultz, L. G. (1964). Quantitative interpretation of mineralogical composition from X-ray and chemical data for the Pierre Shale. U. S. Geol. Surv. Prof. Pap. C, 1
Shamskhany, A., Li, Z., Patel, P., and Karimpour, S. (2021). Evidence of microplastic size impact on mobility and transport in the marine environment: A review and synthesis of recent research. Front. Mar. Sci. 8, 760649. doi:10.3389/fmars.2021.760649
Sheaves, M., Baker, R., Nagelkerken, I., and Connolly, R. M. (2015). True value of estuarine and coastal nurseries for fish: Incorporating complexity and dynamics. Estuaries Coasts 38, 401–414. doi:10.1007/s12237-014-9846-x
Shin, M., and Koch, D. L. (2005). Rotational and translational dispersion of fibres in isotropic turbulent flows. J. Fluid Mech. 540, 143–173. doi:10.1017/S0022112005005690
Shrestha, P. L., and Blumberg, A. F. (2005). “Cohesive sediment transport,” in Encyclopedia of coastal science. Encyclopedia of earth science series. (Dordrecht: Springer), 327–330. doi:10.1007/1-4020-3880-1_95
Somerfield, P. J., and Warwick, R. M. (2013). “Meiofauna techniques,” in Methods for the study of marine benthos. Editor A. Eleftheriou (John Wiley & Sons), 229–272. doi:10.1002/9780470995129.ch6
Stefatos, A., Charalampakis, M., Papatheodorou, G., and Ferentinos, G. (1999). Marine debris on the seafloor of the mediterranean sea: Examples from two enclosed gulfs in western Greece. Mar. Pollut. Bull. 36, 389–393. doi:10.1016/S0025-326X(98)00141-6
Talley, T. S., Venuti, N., and Whelan, R. (2020). Natural history matters: Plastics in estuarine fish and sediments at the mouth of an urban watershed. PLoS One 15, e0229777–19. doi:10.1371/journal.pone.0229777
Thompson, R. C., Olsen, Y., Mitchell, R. P., Davis, A., Rowland, S. J., John, A. W. G., et al. (2004). Lost at sea: Where is all the plastic? Science 304, 838. doi:10.1126/science.1094559
Thorez, J. (1976). “Practical identification of clay minerals,” A handbook for teachers and students in clay mineralogy. Editor Lelotte, G. (Belgium).
Udden, J. A. (1914). Mechanical composition of clastic sediments. Geol. Soc. Am. Bull. 25, 655–744. doi:10.1130/gsab-25-655
UNEP (2016). Marine plastic debris and microplastics – global lessons and research to inspire action and guide policy change. Available at: https://wedocs.unep.org/handle/20.500.11822/7720.
Vale, C., Cortesão, C., Castro, O., and Ferreira, A. M. (1993). Suspended-sediment response to pulses in river flow and semidiurnal and fortnightly tidal variations in a mesotidal estuary. Mar. Chem. 43, 21–31. doi:10.1016/0304-4203(93)90213-8
Van Cauwenberghe, L., Claessens, M., Vandegehuchte, M. B., and Janssen, C. R. (2015). Microplastics are taken up by mussels ( Mytilus edulis ) and lugworms (Arenicola marina) living in natural habitats. Environ. Pollut. 199, 10–17. doi:10.1016/j.envpol.2015.01.008
Van Cauwenberghe, L., Vanreusel, A., Mees, J., and Janssen, C. R. (2013). Microplastic pollution in deep-sea sediments. Environ. Pollut. 182, 495–499. doi:10.1016/j.envpol.2013.08.013
van Sebille, E., Wilcox, C., Lebreton, L., Maximenko, N., Hardesty, B. D., van Franeker, J. A., et al. (2015). A global inventory of small floating plastic debris. Environ. Res. Lett. 10, 124006. doi:10.1088/1748-9326/10/12/124006
Vianello, A., Boldrin, A., Guerriero, P., Moschino, V., Rella, R., Sturaro, A., et al. (2013). Microplastic particles in sediments of Lagoon of Venice, Italy: First observations on occurrence, spatial patterns and identification. Estuar. Coast. Shelf Sci. 130, 54–61. doi:10.1016/j.ecss.2013.03.022
Vieira, H. C., Bordalo, M. D., Figueroa, A. G., Soares, A. M. V. M., Morgado, F., Abreu, S. N., et al. (2021). Mercury distribution and enrichment in coastal sediments from different geographical areas in the North Atlantic Ocean. Mar. Pollut. Bull. 165, 112153. doi:10.1016/j.marpolbul.2021.112153
Wentworth, C. K. (1922). A scale of grade and class terms for clastic sediments. J. Geol. 30, 377–392. doi:10.1086/622910
Werbowski, L. M., Gilbreath, A. N., Munno, K., Zhu, X., Grbic, J., Wu, T., et al. (2021). Urban stormwater runoff: A major pathway for anthropogenic particles, black rubbery fragments, and other types of microplastics to urban receiving waters. ACS Est. Water 1, 1420–1428. doi:10.1021/acsestwater.1c00017
Wong, S. W. S., Zhang, G., Norman, P., Welihinda, H., and Wijeratne, D. T. (2020). Polysulfonate resins in hyperkalemia: A systematic review. Can. J. Kidney Health Dis. 7, 205435812096583–19. doi:10.1177/2054358120965838
Woodall, L. C., Sanchez-Vidal, A., Canals, M., Paterson, G. L. J., Coppock, R., Sleight, V., et al. (2014). The deep sea is a major sink for microplastic debris. R. Soc. Open Sci. 1, 140317. doi:10.1098/rsos.140317
Wright, S. L., Thompson, R. C., and Galloway, T. S. (2013). The physical impacts of microplastics on marine organisms: A review. Environ. Pollut. 178, 483–492. doi:10.1016/j.envpol.2013.02.031
Xiao, D., Chen, D., Zhou, Z., and Zhong, A. (2002). Three-group type mechanism in the curing behavior of polyacrylate and blocked toluene diisocyanate. J. Appl. Polym. Sci. 83, 112–120. doi:10.1002/app.2233
Xu, Q., Xing, R., Sun, M., Gao, Y., and An, L. (2020). Microplastics in sediments from an interconnected river-estuary region. Sci. Total Environ. 729, 139025. doi:10.1016/j.scitotenv.2020.139025
Yao, P., Zhou, B., Lu, Y., Yin, Y., Zong, Y., Chen, M.-T., et al. (2019). A review of microplastics in sediments: Spatial and temporal occurrences, biological effects, and analytic methods. Quat. Int. 519, 274–281. doi:10.1016/j.quaint.2019.03.028
Ye, S., and Andrady, A. L. (1991). Fouling of floating plastic debris under Biscayne Bay exposure conditions. Mar. Pollut. Bull. 22, 608–613. doi:10.1016/0025-326X(91)90249-R
Yurtsever, M. (2019). Glitters as a source of primary microplastics: An approach to environmental responsibility and ethics. J. Agric. Environ. Ethics 32, 459–478. doi:10.1007/s10806-019-09785-0
Zhang, H. (2017). Transport of microplastics in coastal seas. Estuar. Coast. Shelf Sci. 199, 74–86. doi:10.1016/j.ecss.2017.09.032
Zheng, Y., Li, J., Cao, W., Liu, X., Jiang, F., Ding, J., et al. (2019). Distribution characteristics of microplastics in the seawater and sediment: A case study in jiaozhou bay, China. Sci. Total Environ. 674, 27–35. doi:10.1016/j.scitotenv.2019.04.008
Keywords: pollution, accumulation, microplastics to meiofauna ratio, granulometry, organic matter content, rainfall, mineralogical content, sewage discharge
Citation: Rodrigues D, Antunes J, Pais J, Pequeno J, Caetano PS, Rocha F, Sobral P and Costa MH (2022) Distribution patterns of microplastics in subtidal sediments from the Sado river estuary and the Arrábida marine park, Portugal. Front. Environ. Sci. 10:998513. doi: 10.3389/fenvs.2022.998513
Received: 20 July 2022; Accepted: 29 August 2022;
Published: 20 September 2022.
Edited by:
Nsikak U. Benson, Covenant University, NigeriaReviewed by:
Omowunmi H. Fred-Ahmadu, Covenant University, NigeriaJoão P. G. L. Frias, Galway-Mayo Institute of Technology, Ireland
Copyright © 2022 Rodrigues, Antunes, Pais, Pequeno, Caetano, Rocha, Sobral and Costa. This is an open-access article distributed under the terms of the Creative Commons Attribution License (CC BY). The use, distribution or reproduction in other forums is permitted, provided the original author(s) and the copyright owner(s) are credited and that the original publication in this journal is cited, in accordance with accepted academic practice. No use, distribution or reproduction is permitted which does not comply with these terms.
*Correspondence: Diana Rodrigues, ZGR1LnJvZHJpZ3Vlc0BjYW1wdXMuZmN0LnVubC5wdA==