- 1State Key Laboratory of Environment Criteria and Risk Assessment, Chinese Research Academy of Environmental Sciences, Beijing, China
- 2School of Ecology and Environment, Inner Mongolia University, Hohhot, China
- 3School of Environment, Harbin Institute of Technology, Harbin, China
Reservoirs have an important impact on riverine material migration and transformation. Taking a drinking water reservoir located in the cold-temperature forest in the Xiaoxing’an mountains as an example, we comprehensively analyzed the fractions and interrelationships of phosphorus (P) and iron (Fe) from soils to riverine and reservoir sediments and discussed the coordinated migration and transformation process of regional P and Fe and the effects of dam interception. The results showed that iron-bound P (Fe-P) and aluminum-bound P (Al-P) were significantly correlated with adsorbed Fe(II), carbonate-bound Fe (Fecarb), low-activity silicate-bound Fe (Feprs), and total contents of Fe (TFe), which indicated that the geochemical cycling of these P fractions and Fe fractions is likely closely related and active in the soils and sediments. Calcium-bound P (Ca-P) was significantly correlated with magnate (Femag) and Feprs, which may be due to the correlation between their background values in this area. The contents of active P including loosely sorbed P (L-P), Al-P, and Fe-P in the reservoir’s sediments were significantly higher than those in the upstream soils and sediments (p < 0.05). However, Ca-P and residual P (Res-P) in soil and sediment samples showed no significant difference between the reservoir and its upstream (p = 0.309 > 0.05 and p = 0.748 > 0.05, respectively). Construction of reservoirs has played a certain role in intercepting P, especially bioavailable P, transferred from upstream soils and riverine sediments. The contents of highly active Fe fractions including easily reducible (amorphous) Fe oxide (Feox1), reducible (crystalline) Fe oxide (Feox2) and Fecarb (p < 0.01), and other Fe fractions (p < 0.05) in the sediments of the reservoir were significantly higher than those in the soil or sediment samples from upstream. This indicated that all Fe fractions migrated in the river were affected by the dam interception. The effects of dam interception would affect the bioavailability of P and Fe and enhance the cycling of nutrients in the region. Finally, this also effects the bioavailability and cycling of P and Fe in the downstream rivers and even the oceans.
Introduction
Phosphorus (P) and iron (Fe) play important roles in regulating aquatic environmental quality and biological productivity (Babu et al., 2000). Among them, P is an essential nutrient element required for life processes; however, excessive P input often leads to eutrophication of aquatic systems (Ni et al., 2019). P can form important inorganic anion ligands in water, interstitial water, and sediments, complex with metal cations such as Fe, aluminum (Al), and calcium (Ca), or undergo adsorption and chemical reactions with these metal oxides (Zhang and Huang, 2007; Skoog and Arias-Esquivel, 2009). Fe is most closely related to the migration and transformation of P in soils, rivers, lakes, and reservoirs (Jensen et al., 1992). Fe is a redox-sensitive element, which is an important element in the iron–manganese cycle (Stauffer and Armstrong, 1986). Migration and transformation of Fe are mainly achieved through the transition between valence states and the interaction with other surrounding substances, especially organic matter and P (Taillefert et al., 2000).
The physicochemical characteristics of sediments from lakes, reservoirs, and rivers control the exchange process of P at the sediment–water interface. Among them, Fe oxides are one of the main factors controlling adsorption of P in sediments, especially amorphous Fe oxides (Feox1) due to their large specific surface area for high adsorption capacity for P (Zhang and Huang, 2007). Fe would be reduced, dissolved, and released into the overlying water, also accompanied by the release of P when the sediment is in anoxic conditions (Wu et al., 2001). Therefore, total phosphorus (TP) and total iron (TFe) can usually be used as important indicators to evaluate the pollution degree of nutrients in water or sediments. However, only the indicators of TP and TFe are difficult to truly reflect their ecological characteristics (Fitzwater et al., 2000). P and Fe exist in different fractions in sediments, which would be varied in bioavailability and mobility and thus produce different environmental effects (Babu et al., 2000). For example, the ratio of Fe-bound P (Fe-P) to Feox1 [(Fe-P)/Feox1] can be used to predict the potential of P released from the sediments (Loeb et al., 2008). Therefore, it is of great significance to study the occurrence fractions of P and Fe in sediments and the relationships between them for a further understanding of the geochemical cycles of P and Fe.
A reservoir is an artificial lake formed by building a dam at the narrow mouth of a ravine or river, and sometimes, a natural lake is also called a reservoir (natural reservoir). Reservoirs can significantly alter the flow regime of rivers. In addition, the reservoir can retain suspended sediments and the nutrients they carry, thereby affecting the water quality of the reservoir and downstream rivers and even having an important impact on the regional ecological environment (Wei et al., 2011; Maavara et al., 2020). Reservoirs can play the role of reactors in the river and its drainage area by slowing down the flow rate of rivers, increasing the residence time of water, and hindering the flow of substances such as P and Fe. Nutrients such as P are stranded in the reservoir by biological effects such as adsorption, precipitation, or assimilation by primary productivity (Maavara et al., 2015a; Liu et al., 2015). For example, reservoirs on the Mekong River can trap dissolved P, which results in significantly higher concentrations of P in the upstream sediments of reservoirs than in downstreams after long-term retention of P (Liu et al., 2015). Numerous studies have shown that between 1970 and 2000, the amount of TP trapped in reservoirs worldwide nearly doubled, accounting for 12% of the load of TP in rivers (Maavara et al., 2015b). Moreover, with the increase of the number of reservoirs, it is predicted that by 2030, about 17% of the TP in the river will be intercepted by the reservoir to precipitate into sediments (Maavara et al., 2015b; Lehner et al., 2011; Maavara et al., 2020). Therefore, sediments from reservoirs play an important role in the biogeochemical cycle of nutrients through their ability to intercept and re-release nutrients (Wang and Liang 2016).
Reservoirs often deposit and intercept a large amount of sediment input from rivers, which results in pools of organic matter, P, and Fe. In particular, the sediments are characterized as a “sink” of iron in the reservoirs. However, with the increase in the water level in the reservoir, the anaerobic biological activities in the sediments would result in Fe (III) being reduced to Fe (II) under anaerobic conditions. In this process, P captured or combined by part of Fe (III) oxides and hydroxides such as ferrihydrite and goethite would be released synchronously and then diffuses into the interstitial water or overlying water (Kim et al., 2003). Compared with the reservoir, the river is usually in an oxidizing environment; the dissolved and particulate active Fe in the river generally exists in the form of Fe (III). The content and activity of Fe (III) oxides generally control the content and activity of Fe-P in the river (Poulton and Raiswell, 2005). In the sediments, different fractions of Fe oxides have obviously different chemical activities and adsorption affinities, which not only affect the diagenetic cycle of Fe itself but also affect some fractions and activity of P (Lalonde et al., 2012). Therefore, the study of the sediments in the drainage area can deepen the understanding of the retention of P and Fe in the reservoir. Reservoirs affect the distribution of P and Fe in the soils or sediment samples from upstream and downstream and even affect the geochemical cycle of regional nutrients. At present, from the knowledge of the authors, there is still little research on the geochemical cycling of P and Fe together in the soils and sediments in the watershed.
This study assumes that the reservoir intercepts P and Fe in the upstream and has an important impact on the coupled migration process of P and Fe, which in turn plays an important role in the downstream and regional P and Fe cycles. The Xiaoxing’an mountains are one of the northwest-southeast trending mountain ranges of the Xing’an mountains in northeastern Asia. The area is rich in water systems, and many rivers originate here. The Xishan Reservoir is located in the hinterland of the Xiaoxing’an mountains. It is formed by the three major water systems of the Dangshi River, Cuiluan River, and Yao River in the watershed, which finally merge into the Yichun River. The Yichun River flows into the Tangwang River from the east and finally joins the Sea of Okhotsk and the Pacific Ocean through the Tangwang River and Songhua River. As a result, this area has formed a complete river system from Xiaoxing’an mountains to the Xishan Reservoir to the Yichun River and finally into the Pacific Ocean. The migration and transformation processes of P and Fe in this drainage area would also have an important impact on the nutrient migration cycle in the Xiaoxing’an mountainous area and even in the downstream area. Therefore, the Xishan Reservoir and its drainage area in Northeast China were selected as the study site to analyze the fractions of P and Fe together in soils and sediments. The relationships between P and Fe characteristics and differences in P and Fe fractions in the soil sample upstream, riverine, and reservoir’s sediment samples were analyzed. The sources of P and Fe in the reservoir sediments and the interception effect of the reservoirs on various fractions of P and Fe from typical forest natural sources were discussed. Finally, the influence of dam construction on the geochemical cycle of P and Fe together and its effect on water quality were further analyzed.
Materials and methods
Study sites and sampling
Xiaoxing’an mountains are located in Yichun City, Heilongjiang Province, Northeastern China. The region has a large number of rivers and abundant water systems, particularly including the rivers in the Heilong River system, such as the Xun River (also named the Xunbiela River), the Zhan River, the Wuyun River, and the rivers in the Songhua River system, such as the Hulan River and Tangwang River. Xiaoxing’an mountains act as the watershed between the Heilong River system and the Songhua River system. There are many lakes, artificial reservoirs, and dams in this region, such as the Shankou Reservoir, Dadingzishan Reservoir, Guanmenzuizi Reservoir, Kuerbin Reservoir, and Jingpo Lake. The Xishan Reservoir is one of the large artificially constructed reservoirs on the Yichun River built in year 2009 (Figure 1). The Yichun River is the upper reaches of the Tangwang River and Songhua River. The Xishan Reservoir covers an area of 11.54 km2 and controls a drainage area of 1,613 km2. Within the drainage area of the Xishan Reservoir, the forest coverage rate is 97.5%, the proportion of the agricultural cultivated land is small, the population density is only one person per 4 km2, and the pollution induced by human activities is small. Thus, P and Fe in the drainage area mainly come from natural sources. Since the construction of the reservoir, the range of the TP concentration in the overlying water has varied from 0.01 to 0.15 mg L−1, and the range of the TFe concentration has varied from 0.05 to 1.30 mg L−1 (unpublished data from the Ecological Environment Monitoring Station of Yichun City, Heilongjiang Province). The background values of TFe and TP in the drainage area of the Xishan Reservoir are high, which may interact strongly and influence the biogeochemical cycling of P and Fe on each other. Therefore, the drainage area of the Xishan Reservoir was selected to study the effect of the dam on the geochemical cycling of P and Fe from natural sources in the Xiaoxing’an mountains.
At the end of May and beginning of June 2021 (flood season), sediment samples (about 0–10 cm) of the Xishan Reservoir and its upstream were collected with a mud grab, and surface soil samples (about 0–10 cm) of typical points of the upstream were collected with a shovel (Figure 1). The samples were briefly described as follows: sites S1 to S8 are the sediment samples from the reservoir; sites R1 to R14 are the sediment samples from the upstream; and sites R1-S to R14-S are the surface soil samples along the upstream. The sampling sediment and soil samples were transported to the laboratory in airtight plastic bags and placed in cold storage on dry ice. Then, samples were lyophilized, ground to powder, and stored at 0 to −20°C until analysis.
Chemical property and sequential extraction of P
The composition characteristics of elements and oxides in sediments and soils were analyzed by using an energy dispersive X-ray fluorescence spectrometer (model FDX-8100). The content of TP in sediment and soil samples is analyzed by the SMT method (Ruban et al., 2001), which is briefly described as follows: 0.20 g of the sediment sample was added and calcined in a muffle furnace at 450°C for 3 h. Then, 20 ml of 3.5 mol L−1 HCl was added to the treated samples and shaken for 16 h to extract TP. After centrifugation, the supernatant was taken, and the concentration of TP was analyzed by the use of the molybdate blue method (Murphy and Riley, 1962).
Phosphorus fractionation schemes are used, basically following Psenner (1988) and improved by Rydin (2000), which are widely used in the study of P fractions, migration, and transformation in soils and sediments (Fytianos and Kotzakioti, 2005; Zhu et al., 2013). The details are as follows: take an appropriate amount of sediment or soil samples, add 25 ml of 1 mol L−1 NH4Cl, extract for 2 h at pH 7, centrifuge and filter, take the filtrate, and measure the loosely sorbed P (L-P) in the samples; then, add 0.11 mol L−1 Na2S2O4/NaHCO3 to the residue of the previous step for 1 h, centrifuge and filter, and take the filtrate to measure the iron-bound phosphorus (Fe-P); then add 0.1 mol L−1 NaOH to the residue of the previous step for 16 h, centrifuge and filter, and take the filtrate to measure the aluminum-bound P (Al-P); then add 0.5 mol L−1 HCl to the residue of the previous step for 16 h, centrifuge and filter, and take the filtrate to measure calcium-bound P (Ca-P). The fractions of P in the extract were determined by the molybdate blue colorimetric method. Residual P (Res-P) can be calculated by subtracting the aforementioned extracted P from TP. The P fractions in each filtrate were measured three times, and the average value was reported.
Sequential extraction of Fe in sediment and soil samples
Weigh a certain amount of sediment samples, digest them with HCl and HNO3, and dilute them, and the content of TFe in soil and sediment samples should be analyzed by o-phenanthroline spectrophotometry (Zhong, 2010). The fractions of Fe in soil and sediment samples are analyzed by the method of a sequential extraction protocol reported by Poulton and Canfield (2005) and Yang et al. (2016). The sequential extraction of Fe is briefly described as follows: weigh 0.20 g of the sediment or soil sample into a centrifuge tube, add 20 ml of 1 mol L−1 MgCl2 (pH 7) solution, shake at room temperature for 2 h, centrifuge for 10 min (4,000 r min−1) and separating, repeat this step twice, take two times of the supernatant, and mix it to obtain adsorbed Fe (II) to be measured; add 20 ml of 1 mol L−1 NaAc (pH 4.5) solution to the residue of the previous step, shake at 50°C for 48 h, centrifuge for 10 min (4,000 r min−1) for separation, repeat this step twice, take two times of the supernatant, and mix it to obtain carbonate-bound Fe (Fecarb) to be measured; add 20 ml of 1 mol L−1 hydroxylamine hydrochloride (25% acetic acid) solution to the residue of the previous step, shake at room temperature for 48 h, centrifuge for 10 min (4,000 r min−1) for separation, repeat this step twice, take two times of the supernatant, and mix it to obtain easily reducible (amorphous) Fe oxide (Feox1) to be measured; add 20 ml of 50 g L−1 sodium dithionite (0.35 mol L−1 acetic acid/0.2 mol L−1 trisodium citrate, pH 4.8) to the residue of the previous step, shake at room temperature for 2 h, centrifuge for 10 min (4,000 r min−1), repeat this step twice, take two times of the supernatant, and mix it to obtain reducible (crystalline) Fe oxide (Feox2) to be measured; add 20 ml of 0.2 mol L−1 ammonium oxalate/0.17 mol L−1 oxalic acid (pH 3) solution to the residue of the previous step, shake at room temperature for 4 h, centrifuge for 10 min (4,000 r min−1) for separation, repeat this step twice, take two times of the supernatant, and mix it to obtain the magnate (Femag) to be measured; add 10 ml of 12 mol L−1 concentrated hydrochloric acid to the residue of the previous step, heat to boiling for 1 min, fix the volume, and pass through the imported PES water system needle filter (0.45 μm) to obtain the low-activity silicate-bound Fe (Feprs) solution to be measured. Various fractions of Fe in the extract were determined by ICP-OES or phenanthroline spectrophotometry. The Fe fractions in each supernatant were measured three times, and the average value was reported.
Analysis of P adsorption and desorption equilibrium in sediments
According to the methods of Hoffman et al. (2009) and Nair et al. (1984), the adsorption isotherms of P (represented by phosphate) in sediment samples from the Xishan Reservoir are carried out to analyze the adsorption and desorption characteristics of P in sediments from the Xishan Reservoir. Finally, the equilibrium concentration for adsorption and desorption of P (EPC0) was analyzed. The experimental method is described briefly as follows: a series of P concentration gradients are configured, that is, the P concentration gradients prepared with potassium dihydrogen phosphate (KH2PO4) are that 0.000, 0.010, 0.020, 0.025, 0.050, 0.100, 0.200, 0.400, and 0.800 mg L−1, sediment and P-containing solutions of different concentrations are added according to the water–soil mass ratio of 100:1, and oscillated at 25°C for 48 h at room temperature; after centrifugation, the supernatant is taken and filtered through a 0.45-µm membrane, and then, the P concentration in the supernatant is determined by molybdate spectrophotometry. The adsorption capacity Q (g kg−1) of the unit mass sediment is calculated according to the following formula:
Here V is the volume of the solution added to the sample (L); W is the weight of the dry sediment sample (g); C0 is the P concentration in the original solution (mg L−1); Ce is the P concentration in the solution after the experiment (mg L−1). The adsorption/desorption equilibrium concentration can be calculated by the regression method.
When the concentration is low (Stumn and Morgan, 2012),
where Q is the adsorption capacity (mg kg−1); Ce is the equilibrium mass concentration (mg L−1); NAP is the sediment background-adsorbed P (mg kg−1); and m is the slope, which can be used to measure the P adsorption efficiency of sediments.
Statistical analyses
Pearson correlation analysis was applied to discuss the possible correlation of different fractions of P and Fe in sediments and soils. An independent sample t-test was used to explore the significant differences in P and Fe fractions in the sediment or soil samples from the reservoir and its upstream. Data were processed and analyzed by Excel 2019 and IBM SPSS statistics 26. Some figures were drawn by Origin 2018, and ArcGis 10.8.1 is also used for geological statistical analysis and mapping.
Results and discussion
Chemical properties of sediments and soils
SiO2 is the main oxide in the soil and sediment samples, which accounted for an average of 66.7% (Figure 2). The other components of oxides are decreasing in the order of their relative proportions: Al2O3, Fe2O3, K2O, CaO, and MnO (Figure 2). The relative proportion of Al2O3 varied from 18.0 to 23.0%, with an average of 20.5%. The relative proportion of Fe2O3 varied from 4.4 to 8.4%, with an average of 6.2%. The relative proportion of K2O varied from 2.4 to 3.6%, with an average of 2.9%. The relative proportion of CaO varied from 0.8 to 4.4%, with an average of 2.0%. The relative proportion of MnO varied from 0.1 to 0.3%, with an average of 0.2%. Al oxides, Fe oxides, Ca oxides, and Mn oxides are generally considered to be closely related to the migration and transformation of P, especially Fe oxides and Mn oxides (Zhu et al., 2016). The distribution characteristics of Fe oxides and Mn oxides in the soil and sediment samples of the watershed are decreasing in the order that soils > riverine sediments > reservoir sediments. The distribution characteristics of Al oxides are in the inverse order, which indicates that the inert Al oxides are gradually accumulated with the migration from soil to river and reservoir sediments. Compared with the average abundance of Al oxides in the upper crust of the Earth (Taylor and Mclennan, 1995), the upper crust of Eastern China (Yan et al., 1997), the Yangtze and Yellow River sediments (He and Liu, 1997), and the river sediments globally (Viers et al., 2009), the proportion of oxides such as Al2O3, Fe2O3 and MnO in the soil and sediment samples of the Xishan Reservoir watershed is relatively high, which is likely related to the high background values of these metal oxides in the watershed. This may have an important impact on the cycle of P in the Xishan Reservoir watershed.
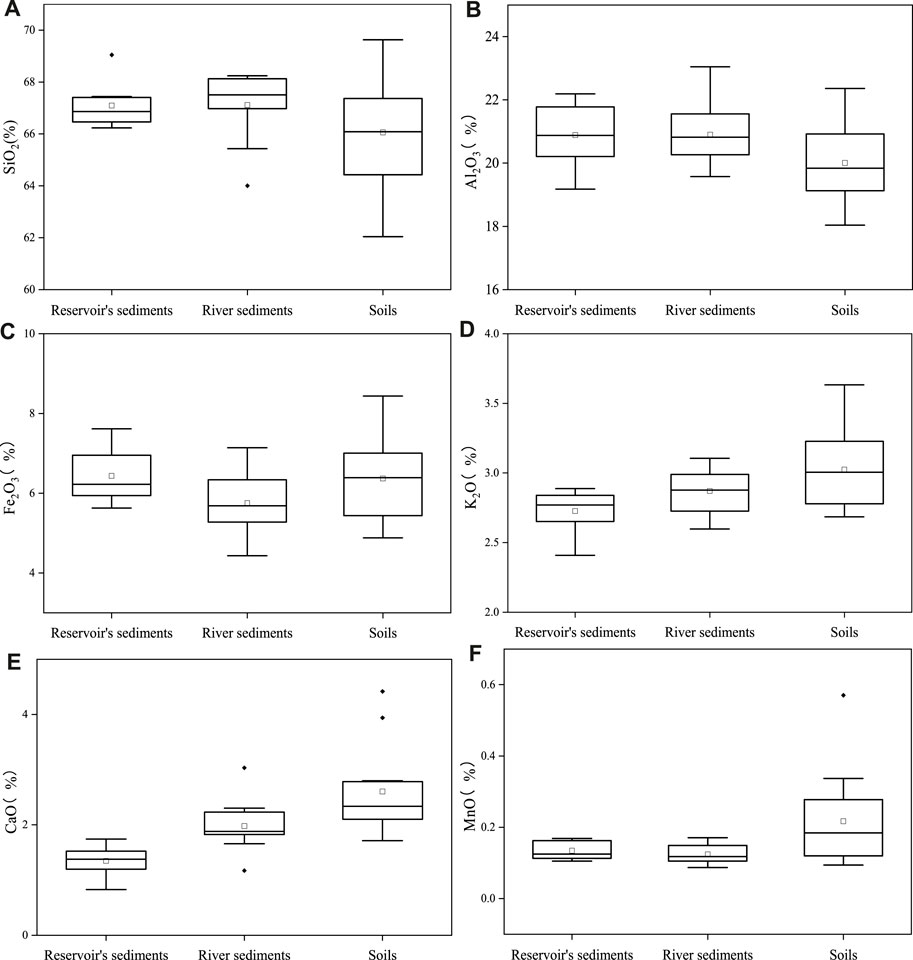
FIGURE 2. Compositions of SiO2 (A), Al2O3 (B), Fe2O3 (C), K2O (D), CaO (E), and MnO (F) in sediments, soils, and reservoir’s sediments in the upstream of the Xishan Reservoir.
The spatial distribution of TP content in soil and sediment samples of the Xishan Reservoir watershed is obviously different (Figure 3). The range of TP contents in soil and sediment samples varied from 574.4 to 7014.3 mg kg−1. The minimal value of TP was the sediment located at site R8 of the Cuiluan River. The maximal value of TP was the soil located at the site of R6-S of the Yao River. The average concentration of TP in the sediments from the upstream is 1444.7 mg kg−1, which is slightly lower than the average concentration of TP in the soil (1631.9 mg kg−1). However, at some sites, such as R1 in the Yao River and R9 and R10 in the Cuiluan River, the TP content in the riverine sediments was significantly higher than that in the soils. For different rivers in the upstream, the TP content in the sediments from the Yao River is relatively high, with the distribution characteristics of TP in the sediments increasing from the upstream to the downstream. The watershed of the Yao River has a large area, a large amount of vegetation is distributed, and organic matter accumulated in the soil, which would result in an accumulation of TP in the sediments of the river with runoff. The sediments of river are easy to adsorb P in the water, which would result in an accumulation of P from upstream to downstream with the river flow direction (Bowes et al., 2003). For the Dangshi River and Cuiluan River, the TP contents of soils and sediments were both slightly lower than those in the sediments from the reservoir, which may indicate the migration process of P from soil to river sediments and then accumulate in the reservoir finally.
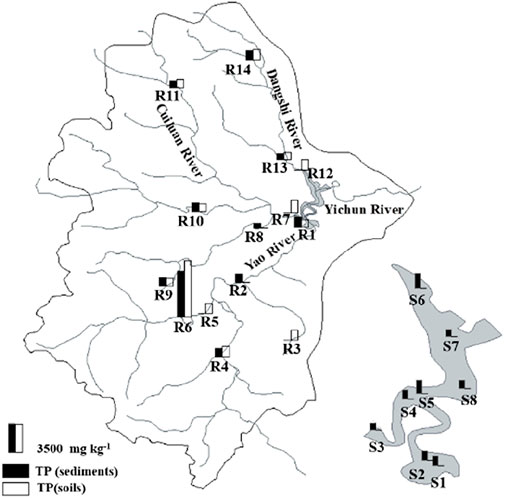
FIGURE 3. Spatial distribution characteristics of the TP content in soils and sediments of the Xishan Reservoir drainage area.
Contents of TFe varied from 11.98 to 32.77 mg g−1, with an average of 23.17 mg g−1 in soil and river sediment samples from upstream (Figure 4). Generally, the TFe contents in the soils were higher than those in the sediments from different rivers. The TFe contents varied from 24.98 to 36.42 mg g−1, with an average of 32.3 mg g−1 in sediments from the reservoir. The TFe content in the sediments from the reservoir was significantly higher than that in the soils and sediments from upstream (p < 0.05). The distribution of TFe at different sites in the reservoir varied in a narrow range but showed the accumulation of Fe sourced from upstream. Generally, the dam showed the interception of TP and TFe together (Liu et al., 2015; Maavara et al., 2020), which likely played an important role in the transformation of P and Fe together in the watershed.
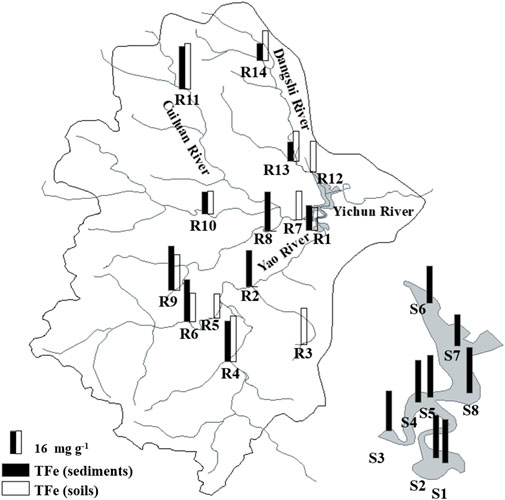
FIGURE 4. Spatial distribution characteristics of the TFe content in soils and sediments of the Xishan Reservoir drainage area.
Characteristics of P fractions in sediments and soils
The contents and relative percentage of different P fractions in soil and sediment samples from the upstream were decreasing in the order such that Res-P > Al-P > Ca-P > Fe-P > L-P. However, the sediment samples in the reservoir were decreasing in the order such that Res-P > Al-P > Fe-P > Ca-P > L-P (Figure 5). L-P is considered to be readily released into the overlying water, which is a very active component of P in the soils and sediments. L-P contains P loosely bound to CaCO3, or P leached from decaying cells in bacterial biomass and plant debris aggregated in sediments, and soluble P in pore water, representing the most bioavailable form of P (Gonsiorczyk et al., 1998; Pettersson, 2001). The contents of L-P ranged from 0.34 to 9.42 mg kg−1, accounting for only a small part of TP, with a relative proportion of <1% in the soil and sediment samples from the Xishan Reservoir watershed. The average values of the L-P content were 3.94 mg kg−1, 3.40 mg kg−1, and 6.40 mg kg−1 in the soils, riverine sediments, and reservoir’s sediments, respectively. The L-P tends to accumulate gradually from soils to river sediments and then to reservoir sediments.
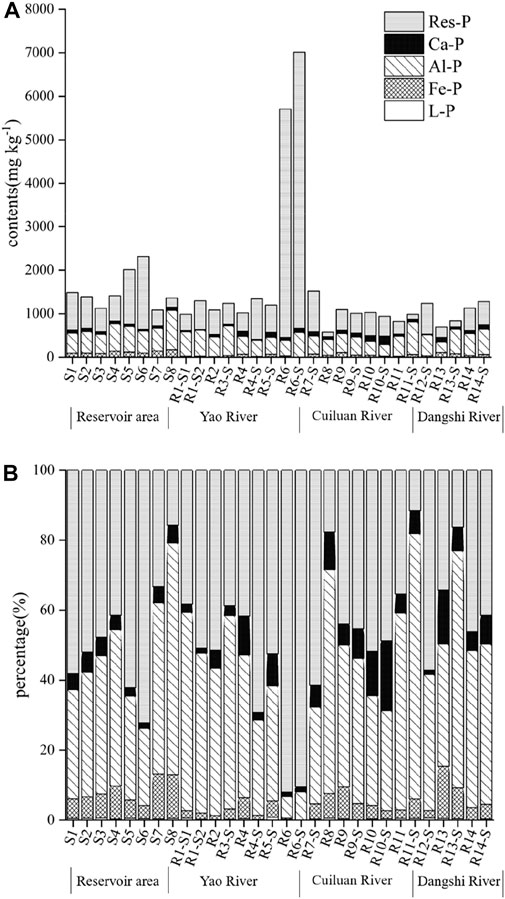
FIGURE 5. Fractions (A) and proportion (B) distribution of P in typical surface sediments and soils in the Xishan Reservoir drainage area.
Fe-P and Al-P represent the main metal-bound (especially Al and Fe oxides) bioavailable P fractions. When the redox conditions, pH, and other environmental conditions at the sediment–water interface are changed, these two fractions of P can be converted into soluble P and bioavailable for the growth of phytoplanktons (Ting and Appan, 1996). The average content of Fe-P in all soil and sediment samples was 60.61 mg kg−1. The average content of Fe-P was 39.61 mg kg−1 and 46.08 mg kg−1 in the soil samples and riverine sediments, respectively. The content of Fe-P ranged from 77.82 to 170.23 mg kg−1, with an average value of 110.30 mg kg−1 in the sediments from the reservoir. These results also indicated that Fe-P also gradually accumulated from soils to riverine sediments and then to reservoir’s sediments. In anaerobic conditions, Fe-P would be released with the reduction and dissolution of Fe (III). Also, in alkaline conditions, Fe-P will be released due to the competition between OH− and HPO42- for effective adsorption sites (House and Denison, 2000). Thus, the results may indicate that the dam interception promotes the contribution of Fe-P to the overlying water of reservoirs and their downstream. In addition, Al-P is the main form of P in the soils and sediments, whose content ranged from 243.96 to 900.73 mg kg−1, with an average of 493.08 mg kg−1, accounting for 41.0% of TP. Among these, the average content of Al-P was 504.72 mg kg−1, 416.49 mg kg−1, and 571.36 mg kg−1 in soils, riverine sediments, and reservoir’s sediments, respectively.
The average content of Ca-P is 70.33 mg kg−1in the soil and sediment samples. Ca-P is generally composed of clastic carbonate-bound P and authigenic apatite-bound P. Apatite in sediments is the only phosphate rock that maintains its original form for a long period (Pettersson et al., 1988). Therefore, Ca-P is generally considered to be relatively inert, which would be buried in sediments (Ma et al., 2022). Also, Ca-P is likely gradually released under some special conditions, such as acidic conditions (Ni et al., 2016). The average content of Ca-P was 75.57 mg kg−1, 73.34 mg kg−1, and 58.72 mg kg−1 respectively, in the soils, riverine sediments, and reservoir sediments. The content of Ca-P in the sediments of the reservoir is relatively low, which is likely due to the weak ability of migration and transformation of Ca-P. Res-P is mainly a stable mineral-bound inert P, as well as some lignin and organometallic complexes (Ivanoff et al., 1998), which is also generally considered as a stable chemical component (Turner et al., 2005). The content of Res-P in the soil and sediment in the Xishan Reservoir drainage area changed from 100.93 to 6346.49 mg kg−1, with an average value of 910.99 mg kg−1, accounting for 47.0% of TP. Some riverine sediment and soil samples have low content of Res-P, such as sites of R8, R11-S, and R13-S, which may be closely related to the high content of organic matter and low inert mineral components.
The principal component analysis of P fractions was carried out for the soil and sediment in the Xishan Reservoir watershed (Figure 6). The results showed that P in the sediments from the reservoir is in the same phase as some soils and riverine sediments in the upstream. Among them, R1-S1 and R1-S2 samples were located in the mouth of the Yao River, near to the sites of S1 and S2 in the reservoir, whose characteristics of P fractions were similar. This may indicate that the fractions and content of P would be changed during the migration and transformation from the upstream to the reservoir. In particular, the farther the distance of the migration of P, the greater the P fractions would be changed.
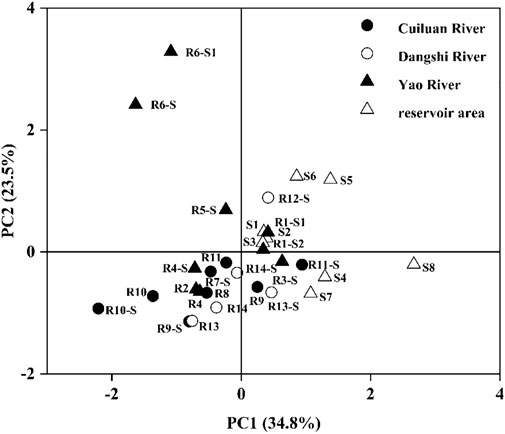
FIGURE 6. Principal component analysis of P fractions in sediments and surrounding soils of the Xishan Reservoir drainage area.
Characteristics of Fe fractions in sediments and soils
The contents and proportions of Fe fractions in soils and sediments of the Xishan Reservoir drainage area decreased in the order such that Feprs > Feox1 > Fecarb > Femag > Feox2 > adsorbed Fe (II) (Figure 7). The content of Feprs in the soils and sediments of the drainage area is the highest, with a content range from 13.74 to 25.84 mg g−1, with an average value of 19.89 mg g−1, accounting for 83.0% of the TFe content. The average content of Feprs in the upstream soil was 21.81 mg g−1; the average content of Feprs in the upstream river sediment was 18.59 mg g−1; the average content of Feprs in the sediment of the reservoir area was 21.81 mg g−1. It indicated that less Feprs was deposited in the rivers with fast flow velocity and gradually buried in the sediments of the reservoir area with the slow flow velocity in the reservoir. In the surface sediments of the Xishan Reservoir, Feprs was the main occurrence form, which was significantly higher than the average content of Feprs in the surface sediments of Tai Lake (4.92 mg g−1), the world shelf sediments (8.40 mg g−1), the global river particles (12.11 mg g−1), and the Yangtze River particles (17.20 mg g−1) (Raiswell and Canfield, 1998; Poulton and Raiswell, 2002).
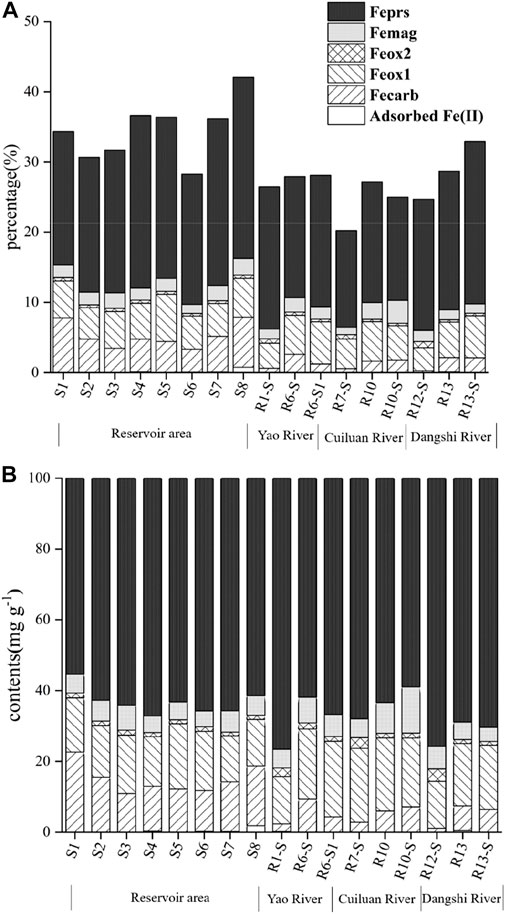
FIGURE 7. Fractions (A) and proportion (B) distribution of Fe in typical surface sediments and soils in the Xishan Reservoir drainage area.
The variation range of the Feox1 content in soils and sediments was 3.28–6.72 mg g−1, with an average of 5.05 mg g−1, accounting for about 21.3% of the TFe content. The average content of Feox1 in the upstream soil was 4.57 mg g−1; the average content of Feox1 in the upstream river sediments was 5.56 mg g−1; the average content of Feox1 in the sediments of the reservoir area was 5.22 mg g−1. It can be seen that Feox1 was likely transferred from the upstream soil to the river and reservoir. Femag, Feox2, and adsorbed Fe (II) are low at various points, accounting for 10.1% of TFe on average. Feox1 and Feox2 are derived from the primary minerals of Fe in sediments and the products of recrystallization of Fe silicate minerals after weathering, such as hematite and goethite. The average content of Feox1 in the drainage area (5.05 mg/g) is lower than that in the Mississippi Delta sediment rich in Fe (8.12 mg/g) (Canfield, 1989). The content of Feox2 is lower than that of Feox1 in the Xishan Reservoir drainage area. Femag is the Fe oxide mixture of Fe (II) and Fe (III). Its content in sediments is always low, which is mainly from mineral rock debris or volcanic rock debris, as well as fine-grained marine authigenic magnetite (Yang et al., 2016). The low content of Femag also indicated that the biologically controlled magnetite formation mechanism does not play an important role in the surface sediments of the Xishan Reservoir (Yang et al., 2016).
The content of Fecarb ranged from 0.27 to 7.77 mg g−1, with an average of 3.13 mg g−1, accounting for 11.4% of the TFe content. The content of Fecarb in the upstream soil ranged from 0.27 to 2.60 mg g−1, with an average of 1.32 mg g−1; the content of Fecarb in the upstream river sediments ranged from 1.22 to 2.02 mg g−1, with an average of 1.62 mg g−1; the content of Fecarb in the sediments of the reservoir area ranged from 3.26 to 7.77 mg g−1, with an average of 5.06 mg g−1. Fecarb in the Xishan Reservoir drainage area was gradually accumulated and increased from the soils to the riverine sediments and then to the reservoir’s sediment. The strict conditions for the formation of Fecarb were that the depositional environment had rich Fe and lack of sulfur and high carbonate contents under the sub oxygen and non-oxygen levels (Aller et al., 2004). Although the content of Fecarb in the soils and sediments was low, the fractions of Fecarb, like the fractions of Feox1 and Feox2, also belong to the high active Fe (III) (Yang et al., 2016), which is also important for the cycling of Fe.
Ferrihydrite, lepidocrocite, goethite, hematite, etc. in Feox1 and Feox2 are all the Fe oxides in the form of Fe (III). Their reduction and dissolution in anoxic conditions contribute to the formation of siderite and ankerite in crystalline Fecarb in the form of Fe (II) and may also form layered Feprs. This may result in the high content of Feprs in the sediments of the drainage area. The reductive dissolution and reoxidation of Fe (III) to form authigenic Fe (III) oxides were thought to be the main paths of the Fe cycle in sediments. The adsorbed Fe (II) in most samples was almost lower than the detection limit (<0.011 mg L−1), which meant that most Fe(II) in the water would be deposited on the surface sediment in the formation of hydroxide, and the content of soluble Fe (II) cations was low.
The principal component analysis (Figure 8) of Fe fractions for all samples showed that there is a strong correlation between Fe in the sediments from the reservoir area and the sediments and soils from the upstream, which may indicate that Fe in the sediments of the reservoir area is sourced from the upstream. Among them, sites of R13 and R13-S are located in the inlet of the Dangshi River, where Fe fractions are most similar to those from the sediments in the reservoir. These results may indicate that Fe in the sediments of the reservoir was mainly sourced from the upstream, and the contribution of the Dangshi River drainage area was likely the largest.
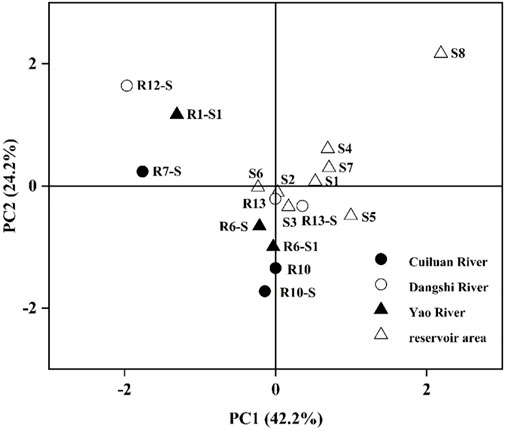
FIGURE 8. Principal component analysis of Fe fractions in sediments and surrounding soils of the Xishan Reservoir drainage area.
Relationships between P fractions, Fe fractions, and the main metal oxides
The relationships between P fractions and the compositions of metal oxides in soil and sediment samples were further analyzed. The results showed that the sum content of Fe-P and Al-P significantly correlated with the sum percentage of Al2O3 and Fe2O3 (Figure 9). There was no significant correlation found between other P fractions and metal oxides. This indicates that Fe and Al oxides play an important role in the migration and transformation of P in the watershed of the Xishan Reservoir. In particular, Fe oxides and P in soils and sediments can be combined with each other, exist in a soluble or insoluble state, or exist independently (Pflaumann and Jian, 1999), which would influence the migration and transformation processes of each other. The correlation analysis between P fractions and Fe fractions in soil and sediment samples further showed that Fe-P and Al-P are significantly correlated with adsorbed Fe (II), Fecarb, Feprs, and TFe (Table 1). This indicates that adsorbed Fe (II) exists in the form of weak adsorption, which would be desorbed and released into the water reactor, acts with other ions (such as PO43-), and may play an important role in the migration and transformation of Fe and P in the drainage area. Fecarb is also sensitive to the changes of environmental conditions, such as hydrodynamic conditions and physical and chemical factors’ change (Wang et al., 2008). With the decomposition of carbonate, Fe(III) and Fe(II) will be released from sediments in the form of active Fe, which will also be complexed or adsorbed with PO43- in water. Additionally, Feprs was significantly correlated with Fe-P and Al-P, which may be closely related to the coordinated migration and transformation processes of Fe, Al, and P-containing minerals such as hematite, bauxite, and fluorapatite in the watershed.
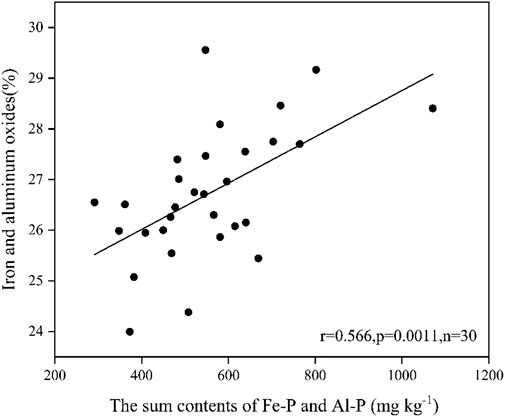
FIGURE 9. Correlation between the sum of Fe-P, Al-P, Al2O3, and Fe2O3 ratios in soils and sediments.
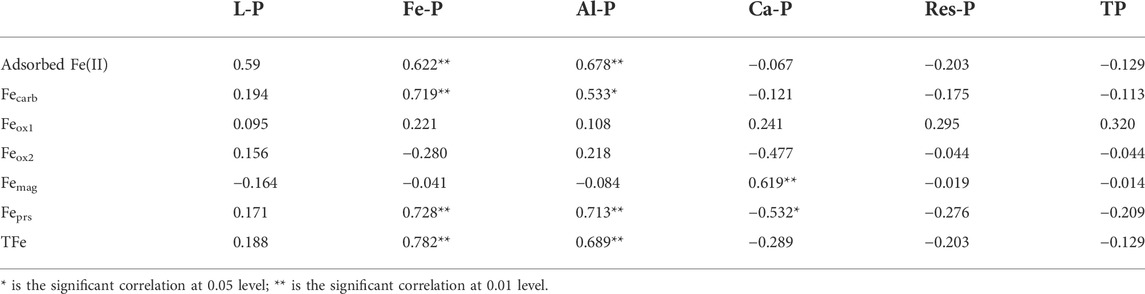
TABLE 1. Correlation analysis of P fractions and Fe fractions in soils and sediments of the Xishan Reservoir drainage area (n = 17).
Contents of Ca-P were positively correlated with contents of Femag but negatively correlated with contents of Feprs in soil and sediment samples. It is generally considered that Ca-P is a relatively stable phosphorus component in sediments, and dicalcium phosphate (Ca2-P) has certain activity, which is generally considered to be the most direct source of available phosphorus in soil. In addition, some microbial metabolic processes can also result in the solubility of some Ca-P (Lindsay and Moreno, 1960). Therefore, Ca-P may react with Fe-containing minerals by increasing solubility or morphological transformation when environmental conditions change. At the same time, it is also possible that the source of Ca-P and Feprs was similar. The mechanisms of migration and transformation of Ca-P at the watershed scale should be studied further. The correlations between different fractions of P and Fe in soil and sediment samples in the drainage area indicated that Fe and Fe oxides, and Al and Al oxides have an impact on the geochemical cycling of P, that is, the release of P in sediments is largely controlled by Fe and Al (Mortimer, 1941).
Implication for geochemical cycling of P and Fe influenced by construction of an artificial reservoir
The value of EPC0 is the equilibrium concentration of soluble reactive phosphate (SRP) for adsorption and desorption by sediments (Taylor and Kunishi, 1971). When the SRP concentration of overlying water is lower than the value of EPC0, P in the sediment would be potentially released into the overlying water. Inversely, when the SRP concentration in the overlying water is higher than the value of EPC0, the sediment tends to adsorb P from the overlying water. The value of EPC0 in the sediments of the Xishan Reservoir can be found in Table 2. The range of EPC0 varied from 0.074 to 0.123 mg L−1 in the Xishan Reservoir. Among them, the value of EPC0 in sediments at site S6 was the largest, which was 0.123 mg L−1. The value of EPC0 in sediments at site S1 was the smallest, which was 0.074 mg L−1. Based on the long-term monitoring data on TP concentrations, which ranged from 0.01 to 0.15 mg L−1, of overlying water from the Xishan Reservoir, it could be inferred that P intercepted by the dam of the Xishan Reservoir may have shown a release trend to the overlying water. Due to interception, P and Fe accumulate in the reservoir, and the content of active P and Fe in the sediment is enhanced, which may be the source of the high EPC0 value in the sediment.
Generally, L-P, Al-P, and Fe-P are considered as bioavailable P fractions (Bio-P) in sediments, which are easily released from sediments in the form of dissolved phosphate for absorption or utilization by aquatic organisms (Wang and Liang, 2016). The content of Bio-P in the sediments from the reservoir was significantly higher than that in soils and sediments from the upstream (p < 0.05). This is likely due to the fact that Bio-P with high mobility and bioavailability can be easily released to the water, or can directly reabsorb and settle in the form of particles in the reservoir. Thus, the Xishan Reservoir plays an important role in intercepting Bio-P from the upstream. After the dam interception, with the increase of water depth and the change of redox conditions at the water–sediment interface, the release of Bio-P buried in the sediment in the reservoir would be enhanced, which would also increase the concentrations of Bio-P in the downstream water (Yuepeng et al., 2021). The contents of Ca-P and Res-P in the soil and sediments upstream were not significantly different from those in the sediments from the reservoir (p > 0.05). This may indicate that Ca-P and Res-P were well buried in particles and could only migrate with particles and accumulate in the sediment of the reservoir. They are difficult to be dissolved in the overlying water, which also has little impact on the concentration of P in the overlying water. Research has shown that almost half of the global reservoirs retain 80% or more of the inflow sediment (Vӧrӧsmarty et al., 2003). This may indicate that Ca-P and Res-P are the main P fractions intercepted by the dam and buried for a long time.
The highly active Fe and P are easy to interact with the reservoir. The distribution of P in sediments depends not only on TFe but also on the fractions of Fe. Feox1 with a large surface area has a strong adsorption capacity for P, and Feox2 also has a certain adsorption capacity for P, but it is much weaker than Feox1 (Baldwin, 1996). Feox1 and Feox2 account for 17.40% of the TFe content in the sediment of the Xishan Reservoir, which may play an important role in the adsorption, sedimentation, and final interception of P in the reservoir. However, the synergistic release capacity and intensity of highly active fractions of Fe and active P in the sediment of the Xishan Reservoir would be enhanced after the dam interception. This would continue to influence the water quality of the downstream water flow, which has a great impact on the concentrations of P and Fe together in the downstream water under anaerobic conditions further. Due to the numerous rivers and dams in the Xiaoxing’an mountains, the interception of P and Fe by artificial reservoirs may have an important impact on the geochemical cycle of P and Fe in the region further.
Conclusion
The contents and relative proportions of P fractions were decreasing in the order such that Res-P > Al-P > Ca-P > Fe-P > L-P in the soil and sediment in the Xishan Reservoir watershed. The contents and relative proportions of Fe fractions were decreased in the order such that Feprs > Feox1 > Fecarb > Femag > Feox2 > adsorbed Fe (II). There were significant correlations between Fe-P, Al-P, and adsorbed Fe (II), Fecarb, Feprs, and TFe. Ca-P was significantly correlated with Femag and Feprs. The content of Bio-P (L-P, Al-P, Fe-P) in the sediment of the reservoir is significantly higher than that in the soil and sediment from upstream. The reservoir plays an important role in intercepting Bio-P from the upstream. The highly active Fe and P are easy to interact with the reservoir. However, the synergistic release capacity and intensity of highly active fractions of Fe and active P in the sediment of the reservoir would be enhanced after the dam interception under anaerobic conditions further. This also has a great impact on the concentrations of P and Fe together in the reservoir and its downstream water further. Due to the numerous rivers and dams in the Xiaoxing’an mountains, the interception of P and Fe by artificial reservoirs may have an important impact on the geochemical cycle of P and Fe in the region and even the oceans in the future.
Data availability statement
The raw data supporting the conclusions of this article will be made available by the authors without undue reservation.
Author contributions
XB, YZ, and JW designed the research, analyzed the data, wrote the manuscript, and improved the language of the manuscript. HM, FL, and JJ analyzed the samples and the data. JD designed the sampling sites and improved the language of the manuscript. All authors discussed the results and reviewed the manuscript.
Funding
This research was jointly supported by the National Natural Science Foundation of China (Nos. 41877380 and 41630645).
Conflict of interest
The authors declare that the research was conducted in the absence of any commercial or financial relationships that could be construed as a potential conflict of interest.
Publisher’s note
All claims expressed in this article are solely those of the authors and do not necessarily represent those of their affiliated organizations, or those of the publisher, the editors, and the reviewers. Any product that may be evaluated in this article, or claim that may be made by its manufacturer, is not guaranteed or endorsed by the publisher.
References
Aller, R. C., Heilbrun, C., Panzeca, C., Zhu, Z., and Baltzer, F. (2004). Coupling between sedimentary dynamics, early diagenetic processes, and biogeochemical cycling in the amazon–guianas mobile mud belt: Coastal French guiana. Mar. Geol. 208 (2-4), 331–360. doi:10.1016/j.margeo.2004.04.027
Babu, K. N., Ouseph, P. P., and Padmalal, D. (2000). Interstitial water–sediment geochemistry of N, P and Fe and its response to overlying waters of tropical estuaries: A case from the southwest coast of India. Environ. Geol. 39 (6), 633–640. doi:10.1007/s002540050475
Baldwin, D. S. (1996). The phosphorus composition of a diverse series of Australian sediments. Hydrobiologia 335 (1), 63–73. doi:10.1007/BF00013684
Bowes, M. J., House, W. A., and Hodgkinson, R. A. (2003). Phosphorus dynamics along a river continuum. Sci. Total Environ. 313 (1-3), 199–212. doi:10.1016/S0048-9697(03)00260-2
Canfield, D. E. (1989). Reactive iron in marine sediments. Geochimica Cosmochimica Acta 53 (3), 619–632. doi:10.1016/0016-7037(89)90005-7
Fitzwater, S., Johnson, K., Gordon, R., Coale, K., and Smith, W. (2000). Trace metal concentrations in the Ross Sea and their relationship with nutrients and phytoplankton growth. Deep Sea Res. Part II Top. Stud. Oceanogr. 47 (15-16), 3159–3179. doi:10.1016/S0967-0645(00)00063-1
Fytianos, K., and Kotzakioti, A. (2005). Sequential fractionation of phosphorus in lake sediments of Northern Greece. Environ. Monit. Assess. 100 (1), 191–200. doi:10.1007/s10661-005-4770-y
Gonsiorczyk, T., Casper, P., and Koschel, R. (1998). Phosphorus-binding forms in the sediment of an oligotrophic and an eutrophic hardwater lake of the Baltic Lake District (Germany). Water Sci. Technol. 37 (3), 51–58. doi:10.2166/wst.1998.0173
He, L., and Liu, Q. (1997). Chemical characteristics of clay minerals in the sediments from the Yellow River and the changjiang river. Chin. Sci. Bull. 42 (6), 488–492. doi:10.1007/BF02882600
Hoffman, A. R., Armstrong, D. E., Lathrop, R. C., and Penn, M. R. (2009). Characteristics and influence of phosphorus accumulated in the bed sediments of a stream located in an agricultural watershed. Aquat. Geochem. 15 (3), 371–389. doi:10.1007/s10498-008-9043-2
House, W. A., and Denison, F. H. (2000). Factors influencing the measurement of equilibrium phosphate concentrations in river sediments. Water Res. 34 (4), 1187–1200. doi:10.1016/S0043-1354(99)00249-3
Ivanoff, D., Reddy, K., and Robinson, S. (1998). Chemical fractionation of organic phosphorus in selected histosols1. Soil Sci. 163 (1), 36–45. doi:10.1097/00010694-199801000-00006
Jensen, H. S., Kristensen, P., Jeppesen, E., and Skytthe, A. (1992). Iron:phosphorus ratio in surface sediment as an indicator of phosphate release from aerobic sediments in shallow lakes. Hydrobiologia 235-236 (1), 731–743. doi:10.1007/978-94-011-2783-7_66
Kim, L. H., Choi, E., and Stenstrom, M. K. (2003). Sediment characteristics, phosphorus types and phosphorus release rates between river and lake sediments. Chemosphere 50 (1), 53–61. doi:10.1016/S0045-6535(02)00310-7
Lalonde, K., Mucci, A., Ouellet, A., and Gélinas, Y. (2012). Preservation of organic matter in sediments promoted by iron. Nature 483 (7388), 198–200. doi:10.1038/nature10855
Lehner, B., Liermann, C. R., Revenga, C., VӧrӧSmarty, C., Fekete, B., Crouzet, P., et al. (2011). High‐resolution mapping of the world's reservoirs and dams for sustainable river‐flow management. Front. Ecol. Environ. 9 (9), 494–502. doi:10.1890/100125
Lindsay, W. L., and Moreno, E. C. (1960). Phosphate phase equilibria in Soils1. Soil Sci. Soc. Am. J. 24 (3), 177–182. doi:10.2136/sssaj1960.03615995002400030016x
Liu, Q., Liu, S., Zhao, H., Deng, L., Wang, C., Zhao, Q., et al. (2015). The phosphorus speciations in the sediments up- and down-stream of cascade dams along the middle Lancang River. Chemosphere 120, 653–659. doi:10.1016/j.chemosphere.2014.10.012
Loeb, R., Lamers, L. P., and Roelofs, J. G. (2008). Prediction of phosphorus mobilisation in inundated floodplain soils. Environ. Pollut. 156 (2), 325–331. doi:10.1016/j.envpol.2008.02.006
Ma, H., Zhu, Y., Jiang, J., Bing, X., Xu, W., Hu, X., et al. (2022). Characteristics of inorganic and organic phosphorus in Lake Sha sediments from a semiarid region, Northwest China: Sources and bioavailability. Appl. Geochem. 137, 105209. doi:10.1016/j.apgeochem.2022.105209
Maavara, T., Chen, Q., Meter, K. V., Brown, L. E., Zarfl, C., Ni, J., et al. (2020). River dam impacts on biogeochemical cycling. Nat. Rev. Earth Environ. 1 (2), 103–116. doi:10.1038/s43017-019-0019-0
Maavara, T., Dürr, H., and Cappellen, P. V. (2015a). Worldwide retention of nutrient silicon by river damming: From sparse data set to global estimate. Glob. Biogeochem. Cycles 28 (8), 842–855. doi:10.1002/2014GB004875
Maavara, T., Parsons, C. T., Ridenour, C., Stojanovic, S., Dürr, H. H., Powley, H. R., et al. (2015b). Global phosphorus retention by river damming. Proc. Natl. Acad. Sci. U. S. A. 112 (51), 15603–15608. doi:10.1073/pnas.1511797112
Mortimer, C. H. (1941). The exchange of dissolved substances between mud and water in lakes. J. Ecol. 29 (2), 280–329. doi:10.2307/2256395
Murphy, J., and Riley, J. P. (1962). A modified single solution method for the determination of phosphate in natural waters. Anal. Chim. Acta X. 27 (C), 31–36. doi:10.1016/S0003-2670(00)88444-5
Nair, P. S., Logan, T. J., Sharpley, A. N., Sommers, L. E., Tabatabai, M. A., and Yuan, T. L. (1984). Interlaboratory comparison of a standardized phosphorus adsorption procedure. J. Environ. Qual. 13 (4), 591–595. doi:10.2134/jeq1984.00472425001300040016x
Ni, Z., Wang, S., and Wang, Y. (2016). Characteristics of bioavailable organic phosphorus in sediment and its contribution to lake eutrophication in China. Environ. Pollut. 219, 537–544. doi:10.1016/j.envpol.2016.05.087
Ni, Z., Wang, S., Yue, W., and Pu, J. (2019). Response of phosphorus fractionation in lake sediments to anthropogenic activities in China. Sci. Total Environ. 699, 134242. doi:10.1016/j.scitotenv.2019.134242
Pettersson, K., Boström, B., and Jacobsen, O.-S. (1988). “Phosphorus in sediments—Speciation and analysis,” in Phosphorus in freshwater ecosystems (Dordrecht: Springer), 91–101. doi:10.1007/978-94-009-3109-1_7
Pettersson, K. (2001). Phosphorus characteristics of settling and suspended particles in Lake Erken. Sci. Total Environ. 266 (1-3), 79–86. doi:10.1016/S0048-9697(00)00737-3
Pflaumann, U., and Jian, Z. (1999). Modern distribution patterns of planktonic foraminifera in the south China sea and Western pacific: A new transfer technique to estimate regional sea-surface temperatures. Mar. Geol. 156 (1–4), 41–83. doi:10.1016/S0025-3227(98)00173-X
Poulton, S., and Raiswell, R. (2002). The low-temperature geochemical cycle of iron: From continental fluxes to marine sediment deposition. Am. J. Sci. 302 (9), 774–805. doi:10.2475/ajs.302.9.774
Poulton, S. W., and Canfield, D. E. (2005). Development of a sequential extraction procedure for iron: Implications for iron partitioning in continentally derived particulates. Chem. Geol. 214 (3-4), 209–221. doi:10.1016/j.chemgeo.2004.09.003
Poulton, S. W., and Raiswell, R. (2005). Chemical and physical characteristics of iron oxides in riverine and glacial meltwater sediments. Chem. Geol. 218 (3-4), 203–221. doi:10.1016/j.chemgeo.2005.01.007
Psenner, R. (1988). Fractionation of phosphorus in suspended matter and sediment. Arch. Hydrobiol. Beih 30, 98–110.
Raiswell, R., and Canfield, D. E. (1998). Sources of iron for pyrite formation in marine sediments. Am. J. Sci. 298 (3), 219–245. doi:10.2475/ajs.298.3.219
Ruban, V., López-Sánchez, J., Pardo, P., Rauret, G., Muntau, H., and Quevauviller, P. (2001). Harmonized protocol and certified reference material for the determination of extractable contents of phosphorus in freshwater sediments--a synthesis of recent works. Fresenius'. J. Anal. Chem. 370 (2-3), 224–228. doi:10.1007/s002160100753
Rydin, E. (2000). Potentially mobile phosphorus in Lake Erken sediment. Water Res. 34 (7), 2037–2042. doi:10.1016/S0043-1354(99)00375-9
Skoog, A. C., and Arias-Esquivel, V. A. (2009). The effect of induced anoxia and reoxygenation on benthic fluxes of organic carbon, phosphate, iron, and manganese. Sci. Total Environ. 407 (23), 6085–6092. doi:10.1016/j.scitotenv.2009.08.030
Stauffer, R. E., and Armstrong, D. E. (1986). Cycling of iron, manganese, silica, phosphorus, calcium and potassium in two stratified basins of Shagawa Lake, Minnesota. Geochimica Cosmochimica Acta 50 (2), 215–229. doi:10.1016/0016-7037(86)90171-7
Stumn, W., and Morgan, J. J. (2012). Aquatic chemistry: chemical equilibria and rates in natural waters. New York: John Wiley & Sons.
Taillefert, M., Lienemann, C. P., Gaillard, J. F., and Perret, D. (2000). Speciation, reactivity, and cycling of Fe and Pb in a meromictic lake. Geochimica Cosmochimica Acta 64 (2), 169–183. doi:10.1016/S0016-7037(99)00285-9
Taylor, A. W., and Kunishi, H. M. (1971). Phosphate equilibria on stream sediment and soil in a watershed draining an agricultural region. J. Agric. Food Chem. 19 (5), 827–831. doi:10.1021/jf60177a061
Taylor, S. R., and Mclennan, S. M. (1995). The geochemical evolution of the continental crust. Rev. Geophys. 33 (2), 241–265. doi:10.1029/95RG00262
Ting, D. S., and Appan, A. (1996). General characteristics and fractions of phosphorus in aquatic sediments of two tropical reservoirs. Water Sci. Technol. 34 (7-8), 53–59. doi:10.2166/wst.1996.0601
Turner, B. L., Cade-Menun, B. J., Condron, L. M., and Newman, S. (2005). Extraction of soil organic phosphorus. Talanta 66 (2), 294–306. doi:10.1016/j.talanta.2004.11.012
Viers, J., Dupré, B., and Gaillardet, J. (2009). Chemical composition of suspended sediments in World Rivers: New insights from a new database. Sci. Total Environ. 407 (2), 853–868. doi:10.1016/j.scitotenv.2008.09.053
Vӧrӧsmarty, C. J., Meybeck, M., Fekete, B., Sharma, K., Green, P., and Syvitski, J. (2003). Anthropogenic sediment retention: Major global impact from registered river impoundments. Glob. Planet. Change 39 (1-2), 169–190. doi:10.1016/S0921-8181(03)00023-7
Wang, C., Zou, L. M., Wang, P. F., and Lin, Z. P. (2008). Distribution and correlation of P and Fe fractions in sediments of typical urban shallow lakes. Huan Jing ke Xue= Huanjing Kexue 29 (12), 3400–3404.
Wang, L., and Liang, T. (2016). Distribution patterns and dynamics of phosphorus forms in the overlying water and sediment of Dongting Lake. J. Gt. Lakes. Res. 42 (3), 565–570. doi:10.1016/j.jglr.2016.03.013
Wei, O., Hao, F., Song, K., and Xuan, Z. (2011). Cascade dam-induced hydrological disturbance and environmental impact in the upper stream of the Yellow River. Water Resour. manage. 25 (3), 913–927. doi:10.1007/s11269-010-9733-6
Wu, F., Qing, H., and Wan, G. (2001). Regeneration of N, P and Si near the sediment/water interface of lakes from southwestern China plateau. Water Res. 35 (5), 1334–1337. doi:10.1016/S0043-1354(00)00380-8
Yan, M., Chi, Q., Gu, T., and Wang, C. (1997). Chemical composition of upper crust in eastern China. Sci. China Ser. D-Earth. Sci. 40 (5), 530–539. doi:10.1007/BF02877620
Yang, W., Tang, H., Han, C., and Ding, S. (2016). Distribution of iron forms and their correlations analysis with phosphorus forms in the sedimentary profiles of Taihu Lake. China Environ. Sci. 36 (4), 1145–1156.
Yuepeng, Y., Wen, Z., Jinyong, T., Xuemei, C., Yu, Z., Xi, C., et al. (2021). Impact of river dams on phosphorus migration: A case of the pubugou reservoir on the dadu river in China. Sci. Total Environ. 809, 151092. doi:10.1016/j.scitotenv.2021.151092
Zhang, J. Z., and Huang, X. L. (2007). Relative importance of solid-phase phosphorus and iron on the sorption behavior of sediments. Environ. Sci. Technol. 41 (8), 2789–2795. doi:10.1021/es061836q
Zhong, Y. (2010). Spectrophotometric determination of iron in soil using phenanthroline. Guangdong Chem. Ind. 037 (003), 184–184,187. doi:10.1016/0026-265X(70)90101-3
Zhu, Y., Wu, F., Feng, W., Liu, S., and Giesy, J. P. (2016). Interaction of alkaline phosphatase with minerals and sediments: Activities, kinetics and hydrolysis of organic phosphorus. Colloids Surfaces A Physicochem. Eng. Aspects 495, 46–53. doi:10.1016/j.colsurfa.2016.01.056
Keywords: sediment, reservoir, Xiaoxing’an mountains, phosphorus fractions, iron fractions, migration and transformation
Citation: Bing X, Wang K, Ma H, Liu F, Jiang J, Ding J, Zhu Y and Wei J (2022) Geochemical cycling of phosphorus and iron in a typical reservoir in the area of Xiaoxing’an mountains, northeastern China. Front. Environ. Sci. 10:998046. doi: 10.3389/fenvs.2022.998046
Received: 19 July 2022; Accepted: 18 August 2022;
Published: 14 September 2022.
Edited by:
Jingfu Wang, Institute of Geochemistry (CAS), ChinaReviewed by:
Haiquan Yang, Institute of Geochemistry (CAS), ChinaGuanglong Liu, Huazhong Agricultural University, China
Copyright © 2022 Bing, Wang, Ma, Liu, Jiang, Ding, Zhu and Wei. This is an open-access article distributed under the terms of the Creative Commons Attribution License (CC BY). The use, distribution or reproduction in other forums is permitted, provided the original author(s) and the copyright owner(s) are credited and that the original publication in this journal is cited, in accordance with accepted academic practice. No use, distribution or reproduction is permitted which does not comply with these terms.
*Correspondence: Yuanrong Zhu, emh1eXVhbnJvbmcwN0BtYWlscy51Y2FzLmFjLmNu; Jian Wei, d2VpamlhbjA5MTFAMTYzLmNvbQ==