- 1College of Veterinary Medicine, China Agricultural University, Beijing, China
- 2Laboratory of Quality and Safety Risk Assessment for Animal Products on Chemical Hazards (Beijing), Ministry of Agriculture and Rural Affairs, Beijing, China
- 3College of Aminal Science and Technology, Anhui Agricultural University, Hefei, China
- 4Laboratory of Detection for Veterinary Drug Residues and Illegal Additives, Ministry of Agriculture and Rural Affairs, Beijing, China
Cefquinome (CEQ), the fourth generation of cephalosporins, has been widely used in practice for the treatment of bacterial infections due to its broad antimicrobial spectrum, stability, and stronger antibacterial activity. However, the wide use of CEQ can cause an environmental risk via animal excretion. In the current study, the environmental fate of CEQ was investigated. The migration rate of CEQ from soil to the aquatic environment was approximately 60%. The half-life (T1/2) of CEQ degradation in the water ranged from 0.96 to 13.75 d. Our results showed that the temperature, illumination, and pH had an effect on the degradation rate, and the rate of CEQ degradation was significantly accelerated under high temperatures. CEQ was more stable in acidic environments than in alkaline. E-isomer of CEQ and △3-isomer of CEQ were the major degradation metabolites identified by UPLC-Q-Orbitrap MS. The product ion m/z 293.041 was the typical fragment ion for E-isomer of CEQ, and m/z 201.044 and m/z 152.016 were the typical fragment ion for △3-isomer of CEQ. The degradation metabolites exhibited lower antibacterial activity, simultaneously, the toxicity of the E-isomer of CEQ should pay more attention to.
Introduction
Since antibiotics were invented, they are crucial in human healthcare, animal husbandry, and plant protection because of their significant effects on pathogenic infections. Over 250 different substances are registered as antibiotics for human or animal health (Kümmerer and Henninger, 2003). However, it has been extensively reported that antibiotics as well as their metabolites were excreted into the effluent and reached the soil and water, posing a great risk to human health. The concentrations of antibiotics in drinking water, seawater, surface waters, and groundwater were higher than 0.01 μg/L (Batt and Aga, 2005; Peng et al., 2008; Watkinson et al., 2009; Zhang et al., 2013a), even, high up to 1,000 mg/L in some Asian countries (Larsson et al., 2007; Review on Antimicrobial, 2015). The emissions from production plants (Larsson et al., 2007; Li et al., 2008; Šimatović et al., 2020), human medicine usage (Wang et al., 2018; Aydin et al., 2019; Khan et al., 2019), livestock and poultry breeding (Zhang et al., 2015; Quaik et al., 2020), aquaculture (Pham et al., 2015; Pham et al., 2018), and plant agriculture are the main sources of antibiotics in the environment.
The persistence, diffusion, and metabolism of antibiotics in the environment is an issue of global concern. Antibiotics from the environmental compartments can be transferred to drinking water sources, threatening human health. With the longtime exposure to antibiotics in water and soil, a negative shift greatly was observed in the structure and function of aquatic ecosystems (Sarmah et al., 2006; Marti et al., 2013). More important, the aquatic environment is a global reservoir for bacteria to acquire and disseminate antibiotic resistance, antibiotic-resistant bacteria (ARB), and antibiotic-resistant genes (ARGs) (Khare et al., 2020).
The β-lactam antibiotics are preeminent in the treatment of bacterial infection due to their excellent clinical efficacy and safety, accounting for more than half of the world’s antibiotic market (Testero et al., 2003; Meliá et al., 2015). Cefquinome (CEQ), the fourth generation of cephalosporins only used in animals with a C-3 bicyclic pyridinium group, is stable and effective against chromosomal and plasmid-encoded β-lactamases (Chin et al., 1992; Ahmad et al., 2015). Therefore, CEQ has a broad antimicrobial spectrum in vitro or in vivo compared with the second and third-generation cephalosporins (Chin et al., 1992; Guerin Faublee et al., 2003; Smiet et al., 2012; Ahmad et al., 2015). It has been used for the treatment of many diseases including calf septicemia, respiratory tract diseases, metritis-mastitis-agalactia syndrome in sows, acute mastitis and foot rot, foal septicemia, and respiratory diseases in horses (Shan et al., 2014; Xiao et al., 2021). Only a small amount of CEQ in animal bodies was transformed into metabolites after administration, most of them were excreted by kidney in urine in parent form which may be released into the environment. The degradation of cephalosporin in the environment will be affected by many factors. According to reported studies, initial concentration, microorganisms, and light will affect the degradation of cephalosporin in the environment. Generally, cephalosporins present low octanol-water partition coefficient (Kow) values (Mrestani and Neubert, 2000). Therefore, they will be hardly eliminated through biotransformation and soil sorption (Alexy et al., 2004). At the same time, different cephalosporins present different degradation rates at the same pH condition. Those drugs may be positively or negatively charged, as well as be a zwitterion because the structures of cephalosporins generally have two or more ionization centers (Ribeiro and Schmidt, 2017). Most previous studies have reported the removal efficiency of cephalosporins, but lack the study on the possible degradation mechanism of cephalosporins degradation in the environment through UPLC-MS/MS analysis.
The objective of this study was to study the absorption, degradation kinetics and mechanisms of CEQ in the environment, and to evaluate the influence of incubation conditions, including concentration, temperature, pH, and light concentrations, on the degradation rate of CEQ. The results of this study will help to predict the fate and degradation of CEQ under different environmental conditions and provide a reference for the risk assessment of CEQ in the water.
Materials and methods
Reagent and chemicals
The standard of Cefquinome sulfate (purity, 96.3%) was purchased from Dr. Ehrenstorfer (Augsburg, Germany). Acetonitrile (LC-MS grade) and formic acid (LC-MS grade) were obtained from Fisher Scientific (Fair Lawn, NJ, United States), and the water was purified by the Milli-Q system (Darmstadt, Germany). All other chemicals were of analytical grade obtained from Sinopharm Group Chemical Reagent (Shanghai, China).
Soil adsorption experiments
The experiment was carried out according to the classification of Organization for Economic Co-operation and Development (OECD) Test Guideline 106 (von Oepen et al., 1991; Drillia et al., 2005). The soil samples were collected from the agricultural fields of China Agricultural University (Beijing, China) by using the multipoint sampling method. The soil samples were air-dried and sieved (2 mm) for the adsorption and degradation experiments. The organic matter content (OM) was determined using the potassium dichromate external heating method, and the cation exchange capacity (CEC) was determined using the ammonium acetate method, which results were shown in Supplementary Table S1. The operation was as follows: 10.0 g of dry soil was weighed and placed in a 50 ml centrifuge tube, followed by the addition of 25 ml 0.01 mol/L CaCl2 solution with the CEQ concentration of 20 μg/ml. The samples were vortexed at 25°C with a speed of 250 rpm. Then, the samples were collected after 8, 15, 30, 45 min, 1, 3, 6, 9, 12, and 24 h respectively, and centrifugated at the speed of 12,000 rpm for 20 min at −4°C. The supernatant was analyzed by ultra-performance liquid chromatography (UPLC).
Method validation was carried out. A standard curve of CEQ in water was constructed (10, 50, 100, 500 1,000, 5,000, 10,000 μg/L), and the correlation coefficient was greater than 0.99. The precision and accuracy were also examined, and the intra-relative standard deviation (RSD) and average recovery were measured in triplicate at each concentration level. The intra-relative standard deviation was calculated repeatedly over 3 days. The mean recoveries of CEQ were in the range of 76.68%–100.60% and the intra-RSD was less than 9.57% and inter-RSD was less than 8.00%, the results were listed in Supplementary Table S2. The limit of quantitation (LOQ) was the concentration when S/N ≥ 10, and the LOQ was 10 μg/L.
Degradation experiments
The control variable method was adopted to study the degradation kinetics of CEQ in water. The degradation experiment was designed with the following conditions: 1) three initial concentrations (2.5, 5, and 10 μg/ml), 2) three incubator temperatures (25, 35, and 45°C), 3) different pH condition (pH = 5, 7, and 9), 5) light. Sodium hydroxide and sulfuric acid were used to adjust the initial pH.
The procedure was as follows. Firstly, the 20 ml of water was put into a 50 ml tube, and 100 µl of different concentrations of CEQ solution were added in to tube to obtain an initial concentration sample. Then, the sample was set in different conditions and collected at 0, 12, 24, 48, 72, 96, and 120 h. Finally, the collected samples were analyzed by up UPLC and UPLC-Q-Orbitrap MS after being centrifugated at −4°C, with the speed of 12,000 rpm for 20 min. In order to avoid the influence of light, the tinfoil was wrapped on the surface of the tube. On the contrary, in order to study the influence of light on the degradation of CEQ, the natural light condition was simulated in the test, giving 12 h of light and 12 h of darkness until the end of the experiment.
Ultra-performance liquid chromatography and UPLC-Q-Orbitrap MS analysis
The UPLC (Agilent 1,290, Agilent Crop, United States) was used for the determination of Cefquinome in samples, and the CEQ was quantified by ultraviolet detector (Agilent 1,290, Agilent Crop, United States) with the wavelengths of 270 nm. Separation was performed using an Agilent C18 column (50 mm × 2.1 mm i.d; particle size, 1.8 μm; Agilent Crop, United States), and the constant proportion of mobile phase is composed of 10% ACN and 90% water containing 0.1% Formic acid. The flow rate and the injection volume were 0.3 ml/min and 20 μl, respectively.
The UPLC-Q-Orbitrap MS equipped with an electrospray ionization source (quadrupole-Orbitrap™ Q Exactive™ Plus, Thermo, Milford, MA) was used to identify the degradation products of CEQ. The scan type was full scan with the range from 150.0 to 2000.0 m/z; the electrospray condition was spray voltage 3800 V in positive mode; capillary temperature at 320°C; and auxiliary gas flow rate of 10 arb at 350°C; sweep gas flow rate was 40 arb. A BEH C18 column (100 mm × 2.1 mm i. d; particle size, 1.7 μm; Waters Crop, United States) was used to separate the products with the flow rate of 0.3 ml/min at 35°C; the mobile phase A was water containing 0.1% formic acid and mobile phase B was acetonitrile containing 0.1% formic acid. The gradient elution program was as follows: 5% B (0–2 min), 5–20% B (2–10 min), 20%–40% B (10–12 min), 40%–70%B (12–15 min), 70%–100% B (15–16 min), 100% B (16–17 min), 100–5% B (17–17.5 min), 5%B (17.5–19 min).
Results and discussion
Adsorption of cefquinome in soil
The adsorption kinetic curve of CEQ in soil was shown in Figure 1A. The adsorption of CEQ in soil can be divided into two stages: the first stage is the rapid adsorption stage, and the second stage is the dynamic equilibrium stage of adsorption and desorption. In the second stage, the speed of adsorption of CEQ turned slow down gradually. The average adsorption of CEQ in soil was 39.92% ± 0.57 after 24 h, and CEQ has a weak adsorption capacity in soil. Most of the CEQ will enter the water environment after being excreted by animals, and a corresponding conclusion was drawn for drugs with similar chemical structures (Rath et al., 2019; An et al., 2021).
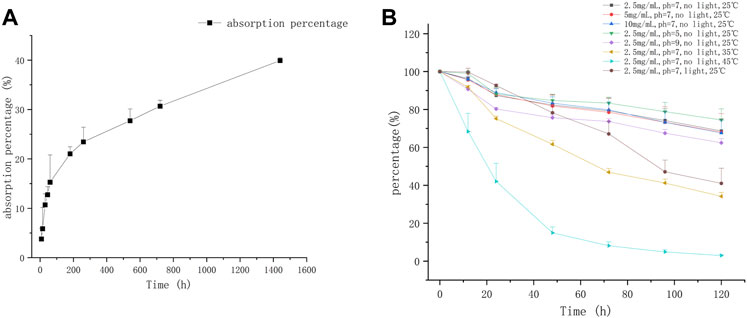
FIGURE 1. The adsorption and degradation curve of CEQ in environment (A) The kinetic adsorption curve of CEQ in soil; (B) The kinetic degradation curve of CEQ in water).
Degradation kinetics of cefquinome in water
Most of studies shown that the kinetics of hydrolysis or degradation of antibiotics in the water environment conforms to the first-order kinetics model (Wang et al., 2006a; Zaranyika et al., 2015; Biošić et al., 2017). Therefore, the first-order kinetic model was used to describe the degradation kinetics of CEQ in water: lnC = -Kt + lnC0, C0 (μmol/L) is the initial concentration of CEQ, C (μmol/L) is the concentration of CEQ at time t, and the K is the rate constant of hydrolysis and total degradation. Consequently, the half-life of CEQ can be calculated as follows: T1/2=(ln2)/k.
To simulate the in-field situation closely, three concentrations were selected in this study. As seen in Figure 1B and Table 1, no obvious impact of different initial concentrations was observed on the degradation of CEQ, and the T1/2 values of CEQ at three different initial concentrations in water were 9.63, 9.32, and 9.32 days. Similar results have been reported for other β-lactam drugs, there was few differences between the degradations of ceftiofur in agricultural soils at different concentrations, and the T1/2 is about 3 days (An et al., 2021). The composition of soil is more complex than water, which may speed up drug degradation in the environment.
The CEQ was degraded in acidic, alkaline, and neutral water bodies as shown in Figure 1B and Table 1. When the solution pH is 5, the degradation rate of CEQ was relatively slow. When the solution pH is 7, the degradation rate of the target substance increases, and the degradation rate of the target compound increases greatly when pH is 9. At the same time, the elimination half-life of the CEQ in the water gradually decreases as the pH value increases. Some other β-lactam drugs, including Ceftiofur, Cefpodoxime, and Cefotaxime sodium (Sunkara et al., 1999; An et al., 2021), were verified to be degraded rapidly in an alkaline condition. β-lactam drugs contain a four-membered β-lactam ring, which is unstable and easy to be broken (Deshpande et al., 2004). At the same time, the β-lactam ring undergoes nucleophilic attack, followed by protonation of the N atom and carbon-nitrogen bond cleavage and a fast degradation (Hou and Poole, 1971; Meliá et al., 2015). Because a methoxyimino group was introduced into the 7-position side chain of β-lactam ring, making CEQ was not suitable to be destroyed due to steric hindrance.
The degradation curves of CEQ were performed at 25°C, 35°C, and 45°C, respectively. As shown in Figure 1B and Table 1, the CEQ was degraded at various temperatures, the degradation rate was faster and the half-life was shortened significantly as the temperature goes higher. After 120 h, more than 90% of CEQ was degraded at 45°C. A similar effect of temperature on the degradation of β-lactam drugs has been reported including Ceftiofur, Ceftazidim, Cefotaxim, and Cefuroxim (Sunkara et al., 1999; An et al., 2021). The reason for this effect is that the hydrolysis reaction is endothermic, and the endothermic reaction requires energy, which mainly comes from the collision between molecules. The collision between molecules becomes more intense when the temperature was higher and more energy can be generated, thereby accelerating the hydrolysis reaction.
The same conclusion about the impact of light on degradation was observed for drugs with similar structures including Cefradine, Ceftriaxone, and Cefepime (Jiang et al., 2010). the degradation rate of CEQ would be accelerated with light in the water body. The degradation curves of CEQ were performed with light or not were shown in Figure 1B and the degradation kinetic parameters as shown in Table 1.
Degradation mechanism and pathway
To clarify the main degradation pathway of CEQ in different water bodies, the samples under different conditions were analyzed by UPLC-Q-OrbitrapMS. The peak of the parent compound CEQ, with a retention time (RT) of 6.78 min (Figure 2A), was confirmed by molecular ions of [M + H] at m/z 529.132. At the same time, two major degradation products were found in all different treatments, and the retention time (RT) was 5.62 min (D1) and 8.00 min (D2) respectively (Figure 2A). After further study of their molecular ion peak, both of two had the same molecular ions at m/z 529.132 which was same as CEQ, which indicated that two major degradation products were isomers of CEQ.
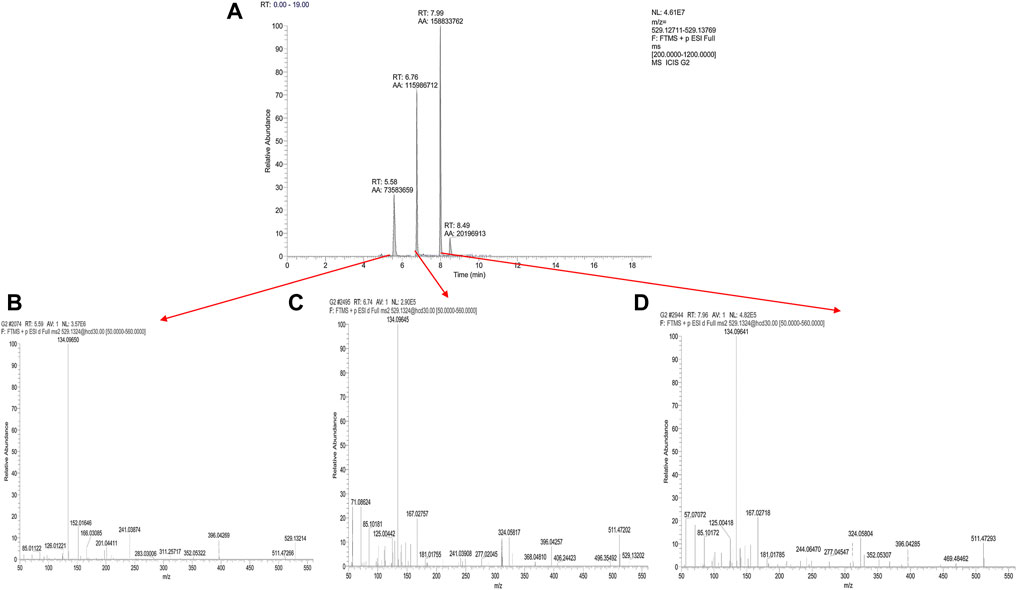
FIGURE 2. The degradation pathway of CEQ was performed on UPLC-OrbitrapMS (Accurate extracted ion chromatograms (EICs) of molecular ions of [M + H] at m/z 529.132 (A) Positive ion ESI-MS/MS spectra of Cefquinome (C), Δ3-isomer of Cefquinome (B), and E-isomer of Cefquinome (D).
To verify the relationship between CEQ and two degradation products, the information of product ion was analyzed. The first step in the collision-induced dissociation (CID) of CEQ is the cleavage of the side chain on position 3, resulting in the generation of m/z 134.096 (side chain) and m/z 396.042 product ions, Meanwhile, the ion at m/z 396.042 could then lose a molecule of carbon dioxide to form the ion at m/z 352.053, and lose a molecule of carbon monoxide to generate m/z 368.047. Further the fragmentation of m/z 352.053 and m/z 368.047 could generate product ion m/z 324.058. Eventually, product ion m/z 277.020 and 167.027 could be generated from product ion m/z 324.058. Figure 2C revealed that [M + H] of CEQ appeared in the full scan of ESI, and the proposed fragmentation pathways of CEQ in the positive ion mode were shown in Figure 3B.
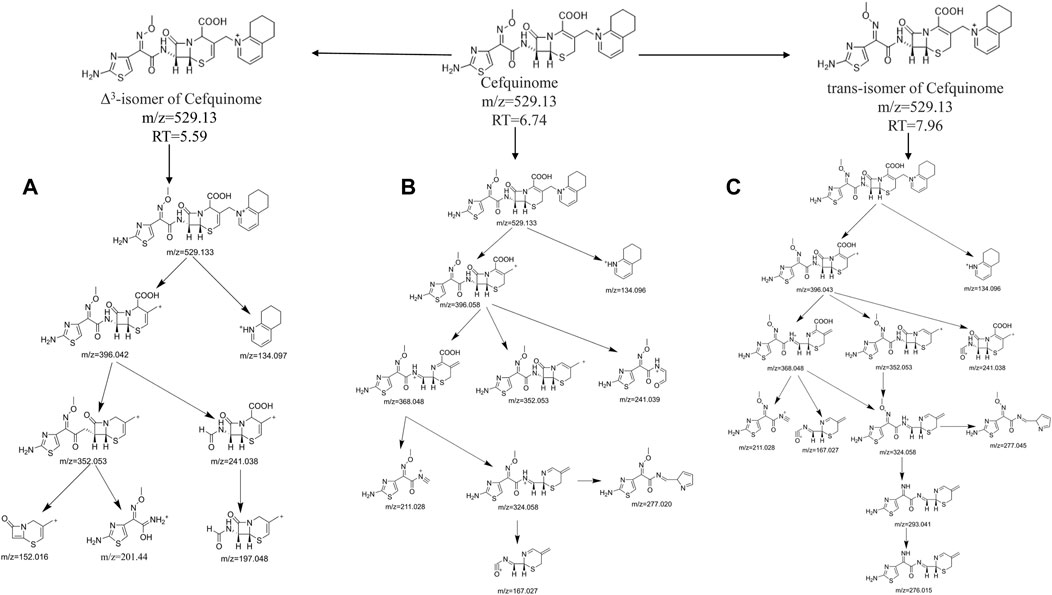
FIGURE 3. The proposed fragmentation pathways of Cefquinome (B), Δ3-isomer of Cefquinome (A), and E-isomer of Cefquinome (C) in the positive ion mode.
For D1, CID produced product ions m/z 396.042, m/z 134.097, and m/z 352.053 were same as CEQ, whereas, m/z 277.020, m/z 368.048, and m/z 167.027 were not found, and were replaced by m/z 201.044, m/z 152.016, m/z 197.048 and m/z 166.031. As reported, m/z 201 and m/z 152 were the typical fragment ion for △3-isomer of cephalosporin which double bond of carbon is translocated from the 2-position to the 3-position at high pH or when heated which was found to have less or no microbiological activity (Zhao et al., 2005; Albishri, 2012; Wang et al., 2013; Wang et al., 2016). For △3-isomer of CEQ, the conjugation was formed between the double bond and S, because the double bond is located between dihydrothiazide C3 and C4, resulting in a change in the electron density of the six-membered ring and preventing Retro Diels–Alder reaction (RDA) cracking. So, m/z 277.020, m/z 368.048, m/z 167.027 were not found in this degradation product. At the same time, product ion m/z 201.044, m/z 197.048, m/z 166.031 and m/z152.016 could be generated from product ion m/z 352.053 after McLafferty rearrangement. Figure 2B revealed that [M + H] of △3-isomer of CEQ appeared in the full scan of ESI, and the proposed fragmentation pathways of △3-isomer of CEQ in the positive ion mode were depicted in Figure 3A. Based on the above reason, the D1 was identified as △3-isomer of CEQ.
For D2, CID produced product ions m/z 396.058, m/z 134.096, m/z 368.048, m/z 352.053, and m/z 324.058, which were similar to those of CEQ, but m/z 167.027 was not found, m/z 293.041 and 276.015 were found instead. Overwhelming studies have indicated that m/z 293.041 was the typical fragment ion for the E-isomer of cephalosporin with lower antibacterial activities (Hu et al., 2005; Wang et al., 2006b; Yu et al., 2017). For the E-isomer of cephalosporin, the methoxy group of the oxime function was easy to lose, because it is difficult to form an N-O hydrogen bond with amines which is far away, resulting in the generation of m/z 293.041. On the other hand, the methoxy group of the oxime in CEQ was more closely to amine which result in the formation of N-O hydrogen bonding, therefore the m/z 277.045 was generated, Figure 2D revealed that [M + H] of E-isomer of CEQ appeared in the full scan of ESI and the proposed fragmentation pathways of E-isomer of CEQ in the positive ion mode were depicted in Figure 3C. According to the abovementioned study, we believed that the D2 was the E-isomer of CEQ.
For the toxicity and biological activity of metabolites, studies reported that the C-7 and C-3 substituents of cephalosporins are toxic and antibacterial activity sites, and the structure change of cephalosporin stereoisomers leads to a change in toxicity and antibacterial activity (Zhang et al., 2013b; Qian et al., 2018; Han et al., 2019). According to Figure 2A, the RT of E-isomers is larger than that of CEQ, indicating that CEQ is more polar than E-isomers. Due to 2-amino-5-thiazolyl residues at CEQ, there are two intramolecular hydrogen bonds formed in E-isomer of CEQ. The distal amine group of the C-7 side chain formed a hydrogen bond with the carboxylic group on the six-member ring. At the same time, the amide nitrogen of the C-7 side chain formed a bond with the nitrogen atom in the thiazole ring. Those hydrogen bonds forced the E-isomers to adopt a more folded conformation, resulting in a decrease the polarity and an increase the passive absorption, which is more likely to give rise to toxicity. Lots of studies have proved a similar result that the E-isomer has stronger strength of teratogenicity and embryotoxicity but lower antibacterial activity (Monguzzi et al., 1985; Zhang et al., 2013b; Qian et al., 2018; Han et al., 2019). For Δ3-isomer of CEQ, there is no change at C-7 and C-3 substituents, meanwhile, it has higher polarity than CEQ based on the RT in reversed-phase liquid chromatography. We guessed that the toxicity of Δ3-isomer of CEQ is similar to or lower than CEQ.
Conclusion
The adsorption of CEQ in agricultural soil and the degradation kinetics and degradation mechanism under different water conditions were studied. The average adsorption of CEQ in soil was 39.92% ± 0.57%. The kinetics of degradation of CEQ was successfully fitted to the first-order kinetics model. Temperature, illumination, and pH significantly affected the degradation of CEQ in the water, and the E-isomer of CEQ and △3-isomer of CEQ were the major degradation metabolites. These results are helpful to evaluate the fate and risk of CEQ in the environment.
Data availability statement
The original contributions presented in the study are included in the article/Supplementary Material, further inquiries can be directed to the corresponding authors.
Author contributions
Conceived and designed the experiments: XC and FS; Performed the experiments: JQ, YY, YC, and YL; Analyzed the data: JQ and JK. The manuscript was discussed and reviewed by all authors.
Funding
This work was financially supported by the National Key Research and Development Program (No. 2018YFD0500301); Anhui Province Key Laboratory of Livestock and Poultry Product Safety Engineering (No. XM 2001).
Conflict of interest
The authors declare that the research was conducted in the absence of any commercial or financial relationships that could be construed as a potential conflict of interest.
Publisher’s note
All claims expressed in this article are solely those of the authors and do not necessarily represent those of their affiliated organizations, or those of the publisher, the editors and the reviewers. Any product that may be evaluated in this article, or claim that may be made by its manufacturer, is not guaranteed or endorsed by the publisher.
Supplementary material
The Supplementary Material for this article can be found online at: https://www.frontiersin.org/articles/10.3389/fenvs.2022.990946/full#supplementary-material
References
Ahmad, I., Hao, H., Huang, L., Sanders, P., Wang, X., Chen, D., et al. (2015). Integration of PK/PD for dose optimization of Cefquinome against Staphylococcus aureus causing septicemia in cattle. Front. Microbiol. 6 (588), 588. doi:10.3389/fmicb.2015.00588
Albishri, H. M. (2012). Simple stereoselective HPLC method for studying the isomerisation of cefditoren pivoxil and its isomeric purity in pharmaceuticals. Chromatographia 75 (21), 1327–1333. doi:10.1007/s10337-012-2313-6
Alexy, R., Kümpel, T., and Kümmerer, K. (2004). Assessment of degradation of 18 antibiotics in the closed bottle test. Chemosphere 57 (6), 505–512. doi:10.1016/j.chemosphere.2004.06.024
An, B., Xu, X., Ma, W., Huo, M., Wang, H., Liu, Z., et al. (2021). The adsorption-desorption characteristics and degradation kinetics of ceftiofur in different agricultural soils. Ecotoxicol. Environ. Saf. 222, 112503. doi:10.1016/j.ecoenv.2021.112503
Aydin, S., Aydin, M. E., Ulvi, A., and Kilic, H. (2019). Antibiotics in hospital effluents: Occurrence, contribution to urban wastewater, removal in a wastewater treatment plant, and environmental risk assessment. Environ. Sci. Pollut. Res. 26 (1), 544–558. doi:10.1007/s11356-018-3563-0
Batt, A. L., and Aga, D. S. (2005). Simultaneous analysis of multiple classes of antibiotics by ion trap LC/MS/MS for assessing surface water and groundwater contamination. Anal. Chem. 77 (9), 2940–2947. doi:10.1021/ac048512+
Biošić, M., Mitrevski, M., and Babić, S. (2017). Environmental behavior of sulfadiazine, sulfamethazine, and their metabolites. Environ. Sci. Pollut. Res. 24 (10), 9802–9812. doi:10.1007/s11356-017-8639-8
Chin, N-X., Gu, J-W., Fang, W., and Neu, H. C. (1992). In vitro activity of cefquinome, a new cephalosporin, compared with other cephalosporin antibiotics. Diagnostic Microbiol. Infect. Dis. 15 (4), 331–337. doi:10.1016/0732-8893(92)90019-p
Deshpande, A. D., Baheti, K. G., and Chatterjee, N. R. (2004). Degradation of β-lactam antibiotics. Curr. Sci. 87 (12), 1684–1695.
Drillia, P., Stamatelatou, K., and Lyberatos, G. (2005). Fate and mobility of pharmaceuticals in solid matrices. Chemosphere 60 (8), 1034–1044. doi:10.1016/j.chemosphere.2005.01.032
Guerin Faublee, V., Carret, G., and Houffschmitt, P. (2003). In vitro activity of 10 antimicrobial agents against bacteria isolated from cows with clinical mastitis. Veterinary Rec. 152 (15), 466–471. doi:10.1136/vr.152.15.466
Han, Y., Zhang, J., Hu, C. Q., Zhang, X., Ma, B., and Zhang, P. (2019). In silico ADME and toxicity prediction of ceftazidime and its impurities. Front. Pharmacol. 10, 434. doi:10.3389/fphar.2019.00434
Hou, J. P., and Poole, J. W. (1971). β-Lactam antibiotics: Their physicochemical properties and biological activities in relation to structure. J. Pharm. Sci. 60 (4), 503–532. doi:10.1002/jps.2600600402
Hu, M., Hu, C-q., and Liu, W-y. (2005). Identification of degradation compounds of cephalosporins by LC/MS. Chin. J. Pharm. Analysis 25 (3), 369–373.
Jiang, M., Wang, L., and Ji, R. (2010). Biotic and abiotic degradation of four cephalosporin antibiotics in a lake surface water and sediment. Chemosphere 80 (11), 1399–1405. doi:10.1016/j.chemosphere.2010.05.048
Khan, F. A., Söderquist, B., and Jass, J. (2019). Prevalence and diversity of antibiotic resistance genes in Swedish aquatic environments impacted by household and hospital wastewater. Front. Microbiol. 10 (688), 688. doi:10.3389/fmicb.2019.00688
Khare, N., Kaushik, M., Martin, J. P., Mohanty, A., and Gulati, P. (2020). Genotypic diversity in multi-drug-resistant E. coli isolated from animal feces and Yamuna River water, India, using rep-PCR fingerprinting. Environ. Monit. Assess. 192 (11), 681. doi:10.1007/s10661-020-08635-1
Kümmerer, K., and Henninger, A. (2003). Promoting resistance by the emission of antibiotics from hospitals and households into effluent. Clin. Microbiol. Infect. 9 (12), 1203–1214. doi:10.1111/j.1469-0691.2003.00739.x
Larsson, D. G. J., de Pedro, C., and Paxeus, N. (2007). Effluent from drug manufactures contains extremely high levels of pharmaceuticals. J. Hazard. Mater. 148 (3), 751–755. doi:10.1016/j.jhazmat.2007.07.008
Li, D., Yang, M., Hu, J., Zhang, Y., Chang, H., and Jin, F. (2008). Determination of penicillin G and its degradation products in a penicillin production wastewater treatment plant and the receiving river. Water Res. 42 (1), 307–317. doi:10.1016/j.watres.2007.07.016
Marti, E., Jofre, J., and Balcazar, J. L. (2013). Prevalence of antibiotic resistance genes and bacterial community composition in a river influenced by a wastewater treatment plant. PloS one 8 (10), e78906. doi:10.1371/journal.pone.0078906
Meliá, C., Ferrer, S., Moliner, V., and Bertran, J. (2015). Theoretical studies of the hydrolysis of antibiotics catalyzed by a metallo-β-lactamase. Archives Biochem. Biophysics 582, 116–126. doi:10.1016/j.abb.2015.01.013
Monguzzi, R., Pinza, M., Pifferi, G., Visconti, M., and Broccali, G. (1985). Semisynthetic beta-lactam antibiotics. VI. Synthesis and antimicrobial activity of alpha-hydrazonobenzylcephalosporins. Farm. Sci. 40 (12), 956–969.
Mrestani, Y., and Neubert, R. (2000). Characterization of cephalosporin transfer between aqueous and colloidal phases by micellar electrokinetic chromatography. J. Chromatogr. A 871 (1), 439–448. doi:10.1016/s0021-9673(99)00875-4
Peng, X., Tan, J., Tang, C., Yu, Y., and Wang, Z. (2008). Multiresidue determination of fluoroquinolone, sulfonamide, trimethoprim, and chloramphenicol antibiotics in urban waters in China. Environ. Toxicol. Chem. 27 (1), 73–79. doi:10.1897/06-650.1
Pham, D. K., Chu, J., Do, N. T., Brose, F., Degand, G., Delahaut, P., et al. (2015). Monitoring antibiotic use and residue in freshwater aquaculture for domestic use in vietnam. EcoHealth 12 (3), 480–489. doi:10.1007/s10393-014-1006-z
Pham, T. T. H., Rossi, P., Dinh, H. D. K., Pham, N. T. A., Tran, P. A., Ho, T. T. K. M., et al. (2018). Analysis of antibiotic multi-resistant bacteria and resistance genes in the effluent of an intensive shrimp farm (Long An, Vietnam). J. Environ. Manag. 214, 149–156. doi:10.1016/j.jenvman.2018.02.089
Qian, J., Han, Y., Li, J., Zhang, J., and Hu, C. (2018). Toxic effect prediction of cefatirizine amidine sodium and its impurities by structure-toxicity relationship of cephalosporins. Toxicol. Vitro 46, 137–147. doi:10.1016/j.tiv.2017.09.021
Quaik, S., Embrandiri, A., Ravindran, B., Hossain, K., Al-Dhabi, N. A., Arasu, M. V., et al. (2020). Veterinary antibiotics in animal manure and manure laden soil: Scenario and challenges in Asian countries. J. King Saud Univ. - Sci. 32 (2), 1300–1305. doi:10.1016/j.jksus.2019.11.015
Rath, S., Fostier, A. H., Pereira, L. A., Dioniso, A. C., de Oliveira Ferreira, F., Doretto, K. M., et al. (2019). Sorption behaviors of antimicrobial and antiparasitic veterinary drugs on subtropical soils. Chemosphere 214, 111–122. doi:10.1016/j.chemosphere.2018.09.083
Review on Antimicrobial, R. (2015). Securing new drugs for future generations: The pipeline of antibiotics: Review on antimicrobial resistance.
Ribeiro, A. R., and Schmidt, T. C. (2017). Determination of acid dissociation constants (pKa) of cephalosporin antibiotics: Computational and experimental approaches. Chemosphere 169, 524–533. doi:10.1016/j.chemosphere.2016.11.097
Sarmah, A. K., Meyer, M. T., and Boxall, A. B. A. (2006). A global perspective on the use, sales, exposure pathways, occurrence, fate and effects of veterinary antibiotics (VAs) in the environment. Chemosphere 65 (5), 725–759. doi:10.1016/j.chemosphere.2006.03.026
Shan, Q., Yang, F., Wang, J., Ding, H., He, L., and Zeng, Z. (2014). Pharmacokinetic/pharmacodynamic relationship of cefquinome against Pasteurella multocida in a tissue-cage model in yellow cattle. J. Vet. Pharmacol. Ther. 37 (2), 178–185. doi:10.1111/jvp.12076
Šimatović, A., and Udiković-Kolić, N. (2020). “Antibiotic resistance in pharmaceutical industry effluents and effluent-impacted environments,” in Antibiotic resistance in the environment : A worldwide overview. Editors C. M. Manaia, E. Donner, I. Vaz-Moreira, and P. Hong (Cham: Springer International Publishing), 101–122.
Smiet, E., Haritova, A., Heil, B. A., Fink-Gremmels, J., and Wijnberg, I. D. (2012). Comparing the pharmacokinetics of a fourth generation cephalosporin in three different age groups of New Forest ponies. Equine Vet. J. 44 (41), 52–56. doi:10.1111/j.2042-3306.2011.00501.x
Sunkara, G., Navarre, C. B., and Kompella, U. B. (1999). Influence of pH and temperature on kinetics of ceftiofur degradation in aqueous solutions. J. Pharm. Pharmacol. 51 (3), 249–255. doi:10.1211/0022357991772411
Testero, S. A., Fisher, J. F., and Mobashery, S. (2003). β-Lactam antibiotics. New Jersey, United States: Burger's Medicinal Chemistry and Drug Discovery, 257–402.
von Oepen, B., Kördel, W., and Klein, W. (1991). Sorption of nonpolar and polar compounds to soils: Processes, measurements and experience with the applicability of the modified OECD-Guideline 106. Chemosphere 22 (3), 285–304. doi:10.1016/0045-6535(91)90318-8
Wang, J., Ruan, D., and Shan, W. (2013). Separation and characterization of the impurities and isomers in cefmenoxime hydrochloride by HPLC-UV-MSn. J. Liq. Chromatogr. Relat. Technol. 36 (15), 2125–2141. doi:10.1080/10826076.2012.712935
Wang, Q., Guo, M., and Yates, S. R. (2006). Degradation kinetics of manure-derived sulfadimethoxine in amended soil. J. Agric. Food Chem. 54 (1), 157–163. doi:10.1021/jf052216w
Wang, Q., Wang, P., and Yang, Q. (2018). Occurrence and diversity of antibiotic resistance in untreated hospital wastewater. Sci. Total Environ. 621, 990–999. doi:10.1016/j.scitotenv.2017.10.128
Wang, S. M., Zou, D. P., Zhang, J. Y., Li, H. Y., and Liu, H. M. (2006). Rapid identification of Z/E cefepime dihydrochloride by high performance liquid chromatography/tandem-time of flight mass spectrometry. Chin. J. Anal. Chem. 34 (9), 1278–1282.
Wang, X., Zhang, K., Zhang, L., Fei, C., Wang, M., Xue, F., et al. (2016). Identification of the isomeric impurity of cefquinome by UPLC-TOF and MS/MS. Chin. Veterinary Science/Zhongguo Shouyi Kexue. 46 (5), 623–629.
Watkinson, A. J., Murby, E. J., Kolpin, D. W., and Costanzo, S. D. (2009). The occurrence of antibiotics in an urban watershed: From wastewater to drinking water. Sci. Total Environ. 407 (8), 2711–2723. doi:10.1016/j.scitotenv.2008.11.059
Xiao, X., Chen, X., Yan, K., Jiang, L., Li, R., Liu, Y., et al. (2021). PK/PD integration and pharmacodynamic cutoff of cefquinome against cow mastitis due to Escherichia coli. J. Vet. Pharmacol. Ther. 45, 83–91. doi:10.1111/jvp.13012
Yu, Y., Zhou, Y., Wang, Z., Torres, O. L., Guo, R., and Chen, J. (2017). Investigation of the removal mechanism of antibiotic ceftazidime by green algae and subsequent microbic impact assessment. Sci. Rep. 7 (1), 4168. doi:10.1038/s41598-017-04128-3
Zaranyika, M. F., Dzomba, P., and Kugara, J. (2015). Degradation of oxytetracycline in the aquatic environment: A proposed steady state kinetic model that takes into account hydrolysis, photolysis, microbial degradation and adsorption by colloidal and sediment particles. Environ. Chem. 12 (2), 174–188. doi:10.1071/en14116
Zhang, J., Qian, J., Tong, J., Zhang, D., and Hu, C. (2013). Toxic effects of cephalosporins with specific functional groups as indicated by zebrafish embryo toxicity testing. Chem. Res. Toxicol. 26 (8), 1168–1181. doi:10.1021/tx400089y
Zhang, Q-Q., Ying, G-G., Pan, C-G., Liu, Y-S., and Zhao, J-L. (2015). Comprehensive evaluation of antibiotics emission and fate in the river basins of China: Source analysis, multimedia modeling, and linkage to bacterial resistance. Environ. Sci. Technol. 49 (11), 6772–6782. doi:10.1021/acs.est.5b00729
Zhang, R., Tang, J., Li, J., Zheng, Q., Liu, D., and Chen, Y. (2013). Antibiotics in the offshore waters of the bohai sea and the yellow sea in China: Occurrence, distribution and ecological risks. Environ. Pollut. 174, 71–77. doi:10.1016/j.envpol.2012.11.008
Keywords: cefquinome, degradation, water, isomer, UPLC-Q-Orbitrap MS
Citation: Qiu J, Yang Y, Kong J, Cao Y, Liu Y, Sun F and Cao X (2022) Environmental fate of cefquinome: Adsorption and degradation. Front. Environ. Sci. 10:990946. doi: 10.3389/fenvs.2022.990946
Received: 11 July 2022; Accepted: 07 October 2022;
Published: 26 October 2022.
Edited by:
Peter S. Hooda, Kingston University, United KingdomReviewed by:
Shizong Wang, Tsinghua University, ChinaLaure Wiest, UMR5280 Institut des Sciences Analytiques (ISA), France
Copyright © 2022 Qiu, Yang, Kong, Cao, Liu, Sun and Cao. This is an open-access article distributed under the terms of the Creative Commons Attribution License (CC BY). The use, distribution or reproduction in other forums is permitted, provided the original author(s) and the copyright owner(s) are credited and that the original publication in this journal is cited, in accordance with accepted academic practice. No use, distribution or reproduction is permitted which does not comply with these terms.
*Correspondence: Feifei Sun, c3VuZmZAYWhhdS5lZHUuY24=; Xingyuan Cao, Y3h5QGNhdS5lZHUuY24=