- 1Key Laboratory of Eco-Environments in the Three Gorges Reservoir Region (Ministry of Education), Chongqing Key Laboratory of Plant Resource Conservation and Germplasm Innovation, College of Life Sciences, Southwest University, Chongqing, China
- 2School of Tourism Management, Guilin Tourism University, Guilin, China
- 3Biological Science Research Center, Academy for Advanced Interdisciplinary Studies, Southwest University, Chongqing, China
Riparian plants are an integral part of the river ecosystem and have significant impacts on the water quality of the reservoir area. The special hydrological process of the riparian zone makes it possible to become a “source” or “sink” of organic matter and nutrients. This study quantifies the flooding period on the decomposition as well as nutrient dynamics of leaves from artificially regenerated Taxodium ascendens in the riparian zone of the Three Gorges Reservoir (TGR) in China. Five decomposition treatment groups were exposed to 10, 30, 60, 90, and 180 days of decomposition: conventional water (T1), mild drought stress (T2), saturated water content (T3), light flooding stress (T4), and severe flooding stress (T5). In T4 and T5, the decomposition rate of T. ascendens leaves was significantly higher than that of T1, T2, and T3. All the leaf C, N, P, and K concentrations at the end of the test were lower than their respective starting values. The concentrations of N and P in the overlying water decrease to various degrees due to decomposition. At the end of the study, the TN content was 1.34 times (T4) and 1.16 times (T5) higher than that of the control group, and the TP content was 3.97 times (T4) and 3.21 times (T5) higher than that of the control group. Leaf decomposition increases the content of N and P in the overlying water under flood conditions, which adversely affects the water environment. This study establishes a theoretical framework for understanding how hydrological processes affect leaf decomposition and nutrient release in riparian areas, providing a scientific basis for riparian zone management in TGR.
Introduction
In the Three Gorges Reservoir (TGR), the water level fluctuates periodically from 145 to 175 m above sea level (a.s.l.), with a vertical drop of 30 m and a total area of 349 km2, resulting in the formation of large areas with a periodically fluctuating water level, namely the riparian zone of the TGR area (Hu et al., 2021; Arif et al., 2022a; Ding et al., 2022). This newly formed transition zone between terrestrial and aquatic ecosystems is a special wetland ecosystem (Zheng et al., 2021a; Li et al., 2021; Yuan et al., 2021). After its impounding in 2003, the TGR area changed from the high water level of the natural rivers in summer to the artificially adjusted high water level in winter. Also, the slowing of the flow rate and the accumulation of nutrients caused by the change in the water level have led to algal blooms in the water bodies in many bays of the reservoir (Chen et al., 2021; He et al., 2021; Muhammad et al., 2022). Water quality in the impoundment has become a prominent issue (Gul et al., 2022). To be sure, this large constructed wetland ecosystem, especially the aquatic environment, will be disturbed by hydrological rhythms. Meanwhile, the plants in the wetland ecosystem play an important role in the nutrient cycle of the TGR area, which can regulate soil nutrient availability as well as the aquatic environment (Ding et al., 2021; Hu et al., 2022; Yuancai et al., 2022).
Taxodium ascendens Brongn. (Pond cypress), a deciduous tree with good flood tolerance, has been proven to be one of the dominant tree species, that, is useful in riparian plantations of the TGR (Wang et al., 2016; Zheng et al., 2021b; He et al., 2021). In the drying period after the water recedes, the pond cypress in the riparian of the TGR recover rapidly and reach their peak growth period in July and August (Xiao et al., 2017), absorbing and fixing large amounts of nutrients from both soil and the overlying water (Dosskey et al., 2010; Peng et al., 2014). During the period of inundation in the TGR, the pond cypress in the riparian zone will be under flooding stress for a long time. Its leaves will inevitably fall and decompose during prolonged flooding, and a large amount of organic matter and nutrients may be released into the body of water of the reservoir. The special hydrological processes in the TGR make the decomposition of plants in the reservoir area possibly become the “source” or “sink” of organic matter and nutrients. As an endogenous factor, which may increase the pressure of excessive nutrients in the aquatic environment of the reservoir, it becomes a source of pollution affecting water quality, resulting in the water quality degrading (Wang et al., 2012; Xu et al., 2013; Yuan et al., 2013). However, as more and more reconstructed vegetation has been constructed, researchers have paid little attention to the flooding decomposition and nutrient release of this reconstructed vegetation. In addition, due to the annual seasonal water level regulation in the reservoir area, the flooding process of the reconstruction of suitable vegetation in the TGR also showed a series of gradient characteristics. Vegetation at different elevations and slope positions experiences drought, water saturation, water flooding, and other conditions (Chen et al., 2021). The leaf decomposition of pond cypress plantations in the TGR area must be affected by these water gradients. However, it is not clear whether the leaf decomposition and nutrient dynamics of the predominant tree species, pond cypress, will affect the reservoir water environment in very large plantations in the riparian zone, and this special habitat.
Many studies have shown that the chemical properties and nutrient status of leaves are important factors affecting the rate of foliage decomposition in rivers (Gessner et al., 2010; Alvim et al., 2015). In addition, abiotic factors such as hydrological regimes also significantly affect foliage decomposition (Anderson and Smith, 2002; Sun et al., 2012; Simões et al., 2021). Different flooding conditions often produce different physical and chemical environments (Behzad et al., 2022), which in turn regulate the decomposition rate of leaves (Anderson and Smith, 2002; Xie et al., 2016; Chen et al., 2020). Previous studies on the decomposition of some herbs in the riparian zone of the TGR have shown that the flooded environment can accelerate the decomposition rate and increase the release of nutrients from these herbs, and different species had significant differences in the decomposition of plants and the release of nutrients (Xiao et al., 2017). Besides, the herbaceous vegetation and trees growing at different altitudes and with different initial levels of nutrients (Wang et al., 2018), the decomposition and nutrient release of leaves of trees may be different from those of herbs (Rincón and Covich, 2014). More importantly, under the same growing conditions in the riparian zone, the relative biomass of trees in the same area is usually higher than that of herbs; so, if the leaves of trees are submerged and decompose faster than in the terrestrial environment, they may release more nutrients into the water.
The controlled experiments were conducted in light of the actual situation of hydrological dynamic changes in the TGR in order to better quantify the process of decomposition. Five moisture treatments over 180 days were conducted to explore the effects of decomposition and characteristics of the nutrient dynamics of the dominant species of pond cypress under different hydrological conditions. The main objectives of this study were to: 1) quantify the decomposition process and rates of the leaves of pond cypress; 2) evaluate the organic matter and nutrient status of the leaves and overlying water under different flooding conditions; and 3) explore the impact of hydrological processes on nutrient cycling of the leaves of pond cypress in the TGR area and thus provide a scientific basis for the management of the riparian zone in the TGR area.
Materials and methods
Study site and experimental material
The leaves and soil used in the decomposition experiment were taken from the riparian zone at the heart of the TGR area of China in the Ruxi River Basin, Zhong County, Chongqing Municipality, at 107°32′–108°14′E, 30°03′–30°35′N; the artificial restoration plantation covers an area of 13.3 hm2. The soil is mainly purple soil of the calcareous purple sand shale parent material developed in a subtropical area (Arif et al., 2022b). Two-year-old seedlings of pond cypress were transplanted in the riparian zone here in 2012. Before the experiment, the landscape had experienced the hydrologic rhythm of the reservoir area for 5 years, and the seedlings had grown well; pond cypress has been proven to be one of the dominant flood-tolerant species suitable for planting in the riparian zone (Wang et al., 2016).
In June 2017, fully developed, healthy, and mature leaves were selected in the pond cypress plantation of the riparian zone, mixed thoroughly, and brought back to the laboratory for natural air-drying for subsequent experiments. The properties of the leaves (based on dry weight) were as follows: carbon (C), 469.83 ± 1.04 g kg−1; N, 20.40 ± 0.28 g kg−1; P, 10.52 ± 0.06 g kg−1; potassium (K), 6.18 ± 0.05 g kg−1; C/N ratio, 23.05 ± 0.27; C/P ratio, 44.66 ± 0.29; and N/P ratio, 1.94 ± 0.03.
At the same time, nine 5 m × 5 m standard plots were randomly set up in the pond cypress plantation, and five 1 m × 1 m sampling plots were randomly selected for each standard plot. The surface vegetation and litter were removed before collecting the surface soil (0–20 cm), which was mixed and transported back to the laboratory; a total of 200 kg of soil was collected in situ. The soil properties (by dry weight) included: pH, 7.10 ± 0.04; organic matter (OM) content, 15.68 ± 0.25 g kg−1; total nitrogen (TN) content, 0.94 ± 0.03 g kg−1; total phosphorus (TP) content, 5.90 ± 0.13 g kg−1; and total potassium (TK) content, 14.65 ± 0.38 g kg−1.
Experimental design
For experimentation, 3 g (accurate to 0.01 g) of natural air-dried leaves were placed into each nylon mesh litterbag (8 cm × 12 cm) with a pore diameter of 0.25 mm; a total of 75 bags were used in the experiment. At the same time, 75 blank litterbags of the same size were prepared as controls, so a total of 150 litterbags were prepared. The mixed soil retrieved from the riparian zone was divided into 150 plastic containers with a volume of 15 L each (diameter 8 cm, height 23 cm, sealed at the bottom and open at the top). Each container was filled with 10 cm of soil; the dry weight of each soil sample was about 640 g. Then, the 150 prepared litterbags were laid flat on the soil surface and fixed to ensure that the decomposition bags were in full contact with the soil surface.
Then, the change in the characteristics of soil moisture conditions in the riparian zone was simulated; the soil moisture content was measured by weighing the litterbag. Five soil moisture treatment groups were set up as follows: T1, conventional water treatment [control check (CK)]; T2, mild drought stress; T3, saturated water content; T4, light flooding stress; T5, and severe water flooding stress. The soil water content of the T1 and T2 groups was 60%–63% and 47%–50% of the field water holding capacity, respectively. The soil surface of the T3 group was in a moist state, but there was no accumulation of water. The soils in the T4 and T5 groups were all submerged so that water covered the soil surface by 2 and 10 cm, respectively. The 150 samples were randomly divided into five groups, including 30 samples in each treatment group (15 litterbags with leaves and 15 without leaves in each group, referred to as the leaf addition and leafless groups, respectively). After the experiment began, each sample was weighed every day, and deionized water was added to supplement the lost water so that the soil water content in all treatment groups was always maintained at a set level during the experiment.
The experiment started on 13 July 2017, and was conducted at room temperature in the Key Laboratory of Ecological Environment of the TGR of Southwest University in China. The climatic conditions were basically the same as the climatic conditions of a pond cypress plantation. Because foliage decomposition typically occurs rapidly at first and then slows over time, the samples were recovered on days 10, 30, 60, 90, and 180. On each sampling day, six samples were randomly selected from each treatment group (that is, three repetitions each for the leaf addition and leafless groups) for destructive sampling. After the litterbags were taken out, the soil and impurities attached to the litterbags and leaves were gently rinsed away with pure water. The samples were dried naturally, placed in an envelope, and dried in an oven at 60°C for 72 h to a constant weight; the samples were then used to determine the dry weight and element content of the leaves. Next, the dry leaf samples were ground into a powder and passed through a 0.25 mm sieve to determine the C, N, P, and K content of the samples. For the T4 and T5 treatment groups, each set of samples (including the leaf addition and leafless groups) was stirred with water in the container, placed into corresponding marked plastic bottles, and returned to the laboratory for subsequent water index determination at the same time.
Sampling measurements
The C and N contents of the crushed samples were determined by a CHNS-O elemental analyzer (Heraeus Elementar, Hanau, Germany); the P and K contents of the crushed samples were determined by heat digestion with a nitric acid and hydrogen peroxide mixture in a microwave digestion system (SpeedWave MWS-4; Berghof Co., Eningen, Germany) and then measured by inductively coupled plasma mass spectrometry (ICP-OES, Thermo Fisher Scientific iCAP 6300, United Kingdom). The TN and TP contents in water were determined by the potassium persulfate digestion-UV spectrophotometer method. The NO3−-N, NH4+-N, and PO43−-P contents in water were determined by ultraviolet spectrophotometry, Nessler’s reagent photometric method, and ultraviolet spectrophotometry, respectively (Wei, 2002).
Statistics and analysis
The dry mass loss rate of leaves, L (%), was calculated as follows:
where Wt is the leaf dry weight (g) after the decomposition time t (d), W0 is the initial dry weight of the leaves (g), k is the decomposition coefficient (d−1) that can characterize the decomposition rate of the leaves, and a is a constant.
The Olson exponential model was used to fit the change process of the remaining dry mass rate (Wt/W0) of the leaf dry mass (Wt/W0) with the decomposition time (t) (Olson 1963):
All data were analyzed and graphed using SPSS 22.0 (IBM, Chicago, United States) and Origin 8.5 (OriginLab Corp., Northampton, MA, United States). Repeated measure analysis of variance was used to determine the effects of sampling time and their interaction on leaf mass loss and leaf C, N, P, and K contents. One-way analysis of variance was used to analyze the effects of different treatments at the same time on leaf mass loss and element content. A difference significance test (α = 0.05) was used (Duncan’s multiple range test).
Results
Species leaf dry mass loss and decomposition rates
During the 180-days decomposition process, the decomposition time, water treatment, and their interaction had extremely significant effects on the mass-loss rate for pond cypress (Table 1) (p < 0.01). During the initial stage of decomposition (0–10 days), the mass-loss rate of all treatment groups reached more than 30% of the total mass, which was 39% (T1), 50% (T2), 44% (T3), 52% (T4), and 49% (T5) (Figure 1). At the end of the experiment, the ranking of mass loss in the groups from greatest to least was T5 > T4 > T3 > T1 > T2; that is, the dry mass loss rate increased with an increase in soil moisture content. In general, the mass loss of all flooded treatment groups (T4, T5) was greater than that of all non-flooded treatment groups (T1, T2, T3) (all p < 0.05), and the mass-loss rate in severe water flooding stress (T5) was significantly higher than that in light flooding stress (T4) (p < 0.05) (Figure 1).
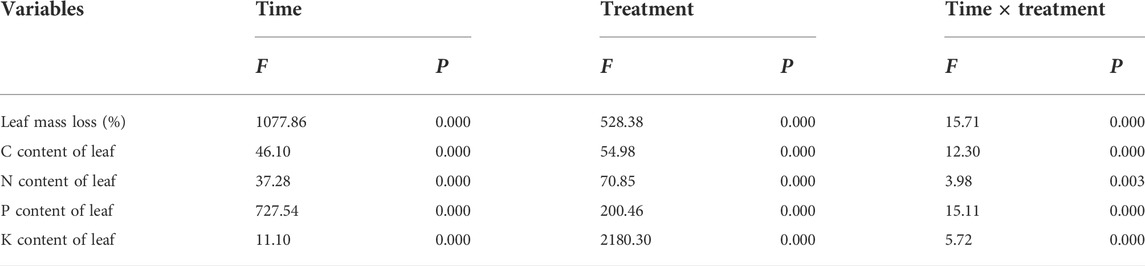
TABLE 1. Results of repeated measure analysis of variance for mass loss and nutrient content under decomposition times, water treatments, and their interactions in the Three Gorges Reservoir, China.
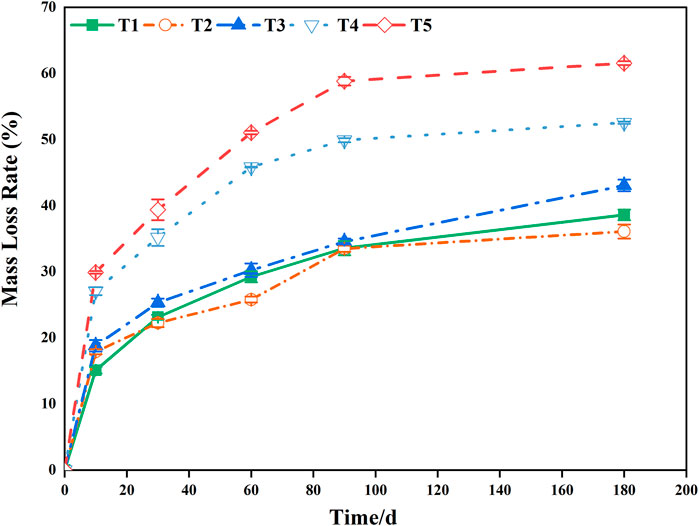
FIGURE 1. Changes in the rate of foliar mass loss during decomposition in the Three Gorges Reservoir, China. T1 represents conventional water treatment [control check (CK)], T2 represents mild drought stress, T3 represents saturated water content, T4 represents light flooding stress, and T5 represents severe water flooding stress.
The rate of leaf decomposition increased with an increase in water content in the treatment groups (Table 2). The decomposition coefficients (k) of the two flooded treatment groups were higher than those of the three non-flooded treatment groups. Compared with T1, the decomposition coefficient (k) of T2 was 11% lower, and that of T3 was 9% higher. More obviously, the decomposition coefficients (k) of the T4 and T5 groups were 55% and 102% higher than those of the T1 group, respectively.
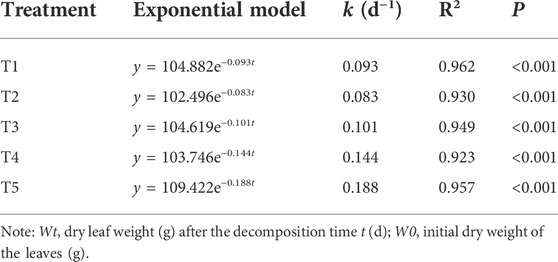
TABLE 2. Exponential simulation of foliar mass remaining rates (Wt/W0) in the Three Gorges Reservoir, China.
Content dynamics of C, N, P, and K in leaves
Decomposition time, water treatment, and their interaction had significant effects on the C, N, P, and K contents of pond cypress (Table 1). Among them, the C content of leaves was relatively stable and remained in the range of 423.90–478.77 g kg−1 (Figure 2). The N content of leaves showed an overall upward trend over time under all water treatments. At the end of the experiment, the N content of leaves of the T2 group was the lowest (25.70 ± 1.22 g kg−1), which was significantly lower than that of the control group (T1) (28.13 ± 0.54 g kg−1), while the N contents of the T3, T4, and T5 treatment groups (29.43 ± 0.32, 31.20 ± 0.38, 31.37 ± 0.84 g kg−1, respectively) were significantly higher than that of the control group (p < 0.05) (Figure 2).
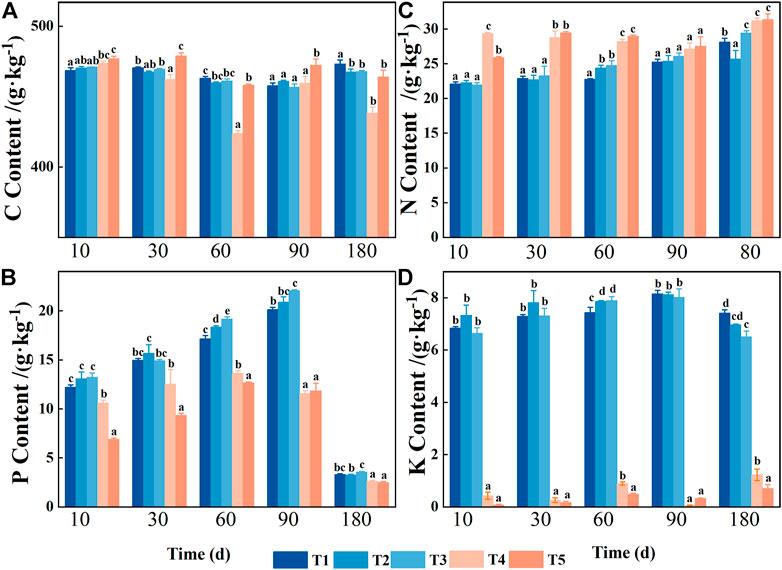
FIGURE 2. Variations in foliar nutrients during decomposition in the Three Gorges Reservoir, China: Leaf carbon content (C) in (A); Leaf phosphorus content (P) in (B); Leaf nitrogen content (N) in (C); and Leaf potassium content (K) in (D). T1 stands for conventional water treatment [control check (CK)], T2 stands for mild drought stress, T3 stands for saturated water content, T4 stands for light flooding stress, and T5 stands for severe water flooding stress. At the 0.05 level, different letters show significant differences between treatments at the same time.
Unlike the change in the N content, the P content of the leaves initially increased and then decreased during the experiment under each treatment (Figure 2). During the 10–90-day decomposition process, the P content of the leaves in each treatment increased significantly. However, during the 90–180-day period, the P content of the leaves in each treatment decreased significantly (p < 0.05). At the end of the experiment, compared with the initial value, the loss of P content of the leaves in each treatment was 69% (T1), 69% (T2), 66% (T3), 75% (T4), and 76% (T5), which showed an overall decrease in P content. In addition, the P content of leaves in the unflooded group was significantly higher than that in the flooded group at all sampling times (p < 0.05). Except for the sampling time of 60 days (p < 0.05), there was no significant difference in the P content of leaves among the other three unflooded groups (p > 0.05). Except for the sampling times of 90 and 180 days (p > 0.05), the P content of leaves in the other two flooded treatment groups decreased with the increase in water content (T4 > T5, p < 0.05).
The dynamic change of K content of the leaves in the T1, T2, and T3 groups was similar to that of P content (Figure 2), which showed an initial increase and then a decrease. However, it was still significantly higher than the initial value at the end of the experiment. The K content of the leaves in the two flooded treatment groups was low and did not change much, remaining at 0.06 ± 0.01–1.23 ± 0.22 g kg−1, but the K content of the leaves in the three non-flooded treatment groups was significantly lower at each sampling time (all p < 0.05).
Total dynamics of C, N, P, and K on leaves
During the entire experiment, the total amount of C in the leaves decreased with an increase in decomposition time in all treatments and was significantly lower than its initial value (p < 0.05), representing a net release of C. In addition, the total amount of C in the leaves in the two flooded treatment groups was significantly lower than that of the three non-flooded treatment groups at each sampling time (p < 0.05) (Table 3). The total amount of N in the leaves in the T1, T2, T4, and T5 groups decreased gradually with an increase in the treatment time during the 10–90 days of decomposition, but the total amount of N in the T3 group decreased throughout the entire experiment. During the 90–180 days of decomposition, the total amount of N in the leaves increased but was significantly lower than the initial value at the end of the experiment (p < 0.05), representing a net release of N. The total amount of P in the leaves initially increased and then decreased (p < 0.05); at the end of the experiment, the total amount of P in each treatment was significantly lower than the initial value (p < 0.05), also representing a net release of P. The K content of the leaves fluctuated alternately during decomposition in the T1, T2, T4, and T5 groups; however, the total amount of K in the T3 group gradually decreased over time. At the end of the experiment, the total amount of K in the leaves in the three non-flooded treatment groups was significantly lower than the initial experimental value (p < 0.05), still showing a net release of K. In addition, during the entire experiment, the total amount of K in the leaves in the two flooded treatment groups was always significantly lower than that of the three non-flooded treatment groups (p < 0.05), compared with the initial value of the experiment, that is, it also showed a net release of K (Table 3).
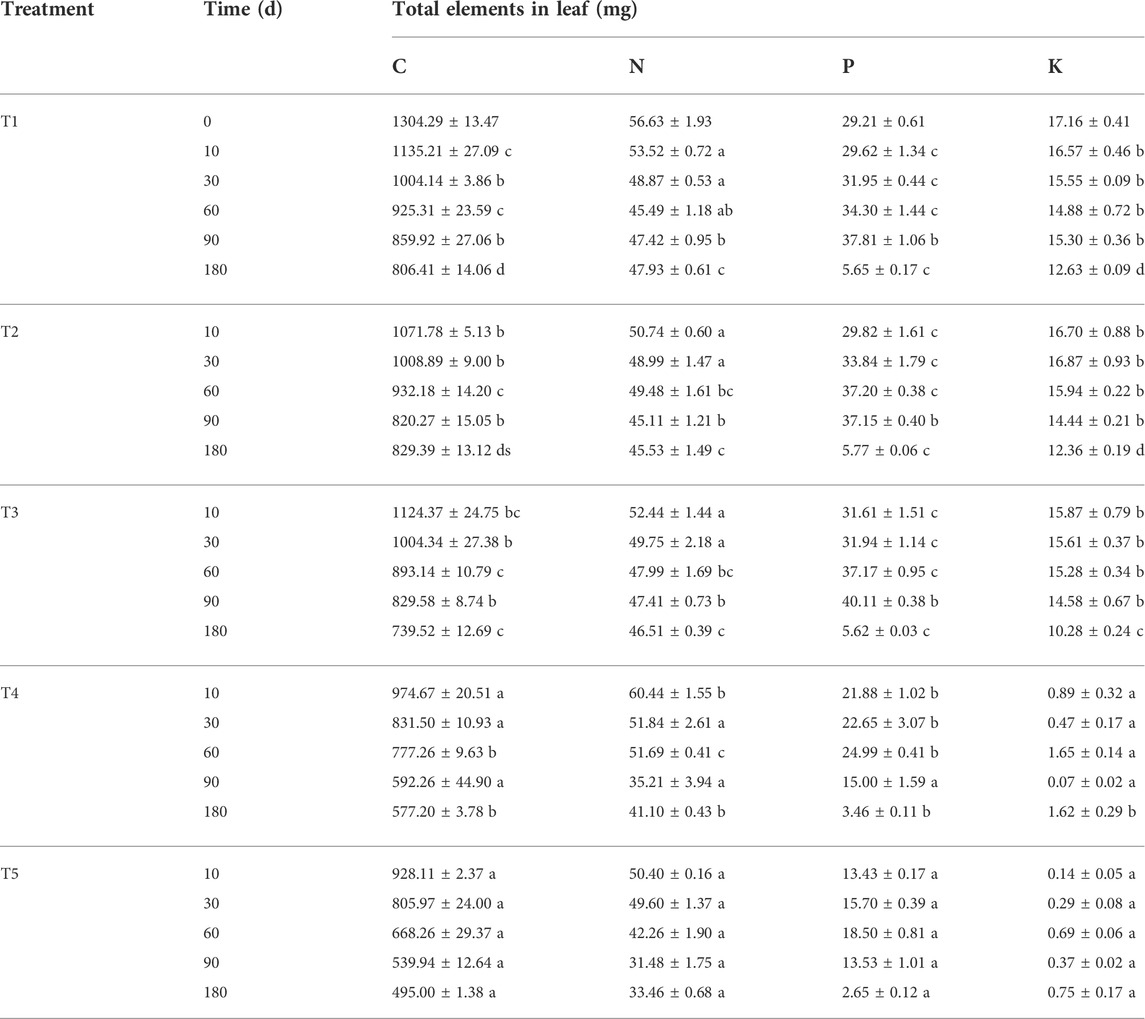
TABLE 3. The total amount of each element in the leaves of pond cypress trees in the Three Gorges Reservoir, China.
Dynamics of N and P contents in overlying water during flooding
The sampling time, the foliage samples (including leaf addition or leafless groups), and their interaction had significant effects on the N and P content of overlying water in the two flooded treatment groups (except for the interaction between sampling time and the samples on the NO3−-N content). Moreover, water treatment, the interaction of sampling time and water treatment, the interaction of water treatment and samples, and the interaction of sampling time, water treatment, and samples also significantly affected the NO3−-N content in the overlying water (Table 4).
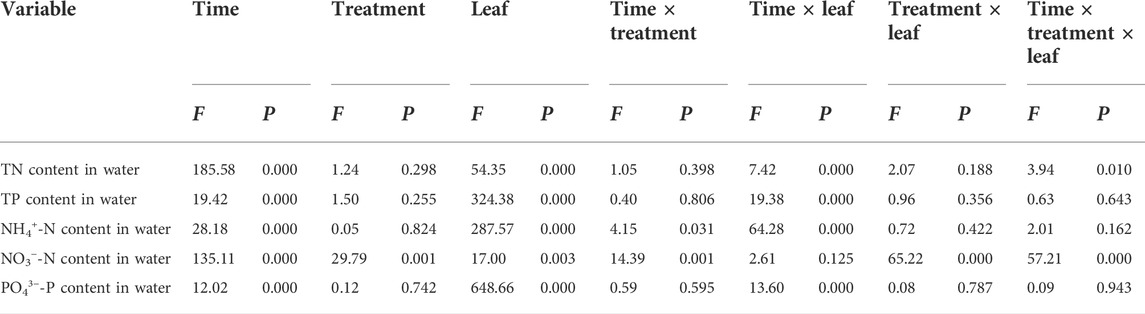
TABLE 4. Results of repeated measures analysis of variance on nutrient content in overlying water under conditions of decomposition time, water treatment, and interaction in the Three Gorges Reservoir in China.
At the beginning of the experiment (0–10 days), the N and P contents in the leaf addition treatment of the overlying water in the two flooded treatment groups increased significantly (Figure 3), and were significantly different from the initial values of the experiment (p < 0.05). Among them, the TN content in the overlying water changed consistently during the experiment, showing alternating changes. At the end of the experiment, the TN content of the overlying water in two flooded treatments with leaf addition groups was significantly higher than that in each group without leaf addition (p < 0.05) and increased by 1.34 times (T4) and 1.16 times (T5). The TP content in the overlying water increased rapidly during the early stage of decomposition (0–10 days), reached a peak of 1.88 (T4) and 1.56 mg L−1 (T5) at 10 days, and then gradually decreased in the subsequent 10–180 days. At the end of the experiment, the TP content of the overlying water of the leaf addition group was 3.97 times (T4) and 3.21 times (T5) of the respective leafless group, while the TP content of the overlying water in the leafless group did not change significantly during the whole experiment period (0.08–0.16 mg L−1). The content of NH4+-N in the overlying water was similar to that of TN during the whole experiment, which showed an alternate fluctuation, but the range of change was greater than that of TN. At the end of the experiment, the content of NH4+-N in the overlying water in the leaf addition group was also significantly higher than that in the leafless group (p < 0.05) (Figure 3). The NO3−-N content in the overlying water increased rapidly at the beginning of the experiment (0–10 days) and then decreased gradually. At the end of the experiment, the NO3−-N content in the overlying water was contrary to the content of TN, TP, and NH4+-N, which was significantly higher in the leafless group than in the leaf addition group (p < 0.05). The content of PO43−-P in the overlying water of the leaf addition group also reached the peak at the beginning of the experiment (0–10 days) and then gradually decreased (Figure 3); in addition, the PO43−-P content of the leaf addition group at each sampling time was significantly higher than that of the respective leafless group (p < 0.05), but there was no significant difference in PO43−-P content between the leaf addition groups of two flooded treatment groups (p > 0.05). In general, at the end of decomposition, except for the NO3−-N content of the overlying water, the NO3−-N content of the leafless group was higher than that of the leaf addition group, while TN, TP, NH4+-N, and PO43−-P contents were significantly higher in the leaf addition groups than in the respective leafless groups (p < 0.05).
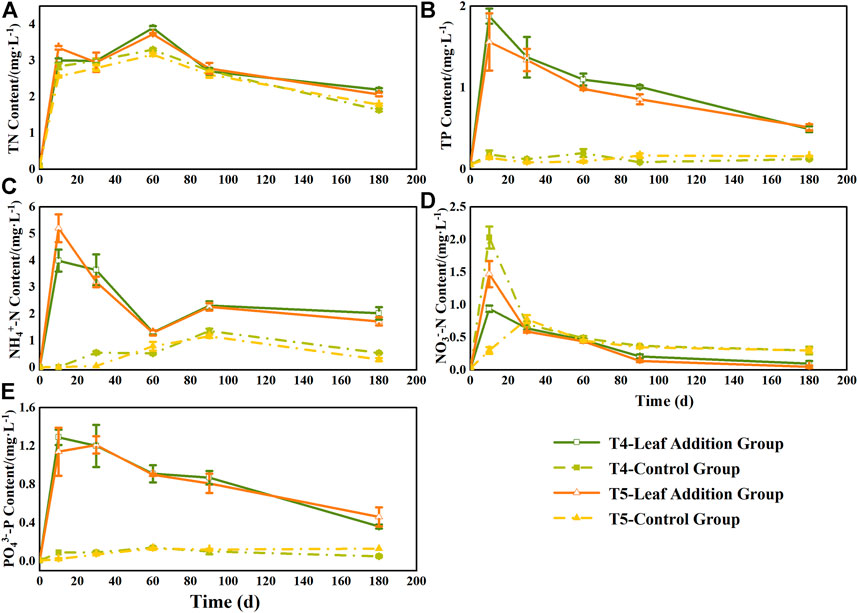
FIGURE 3. Changes in the levels of N and P in the overlying water during decomposition in Three Gorges Reservoir, China: (A) total nitrogen (TN); (B) total phosphorus (TP); (C) NH4+-N (D) NO3−-N; and (E) PO3−-P.
Discussion
Effects of flooding treatments on foliage mass loss rate of pond cypress
Leaf mass loss is attributed to litter species and many physical conditions, such as incubation time, temperature, moisture, and leaching methods (Zhang et al., 2019; Kou et al., 2020; Migliorini and Romero, 2020). In some ecosystems, the effects of environmental factors on mass loss impact overrode litter quality itself (Yu et al., 2020). Among them, moisture is one of the important factors affecting material circulation and energy in a riparian ecosystem (Yue et al., 2016; Arif et al., 2022c). It can indirectly affect the aeration of plant residues, thus affecting their mineralization and decomposition processes (Sun et al., 2012). In our study, the rate of mass loss of the two flooded treatment groups, T4 and T5, was significantly higher than that of the three non-flooded treatment groups, T1, T2, and T3 (Figure 1, Table 2). That is, flooding promotes the foliage decomposition of pond cypress, which was similar to our previous studies in situ (Chen et al., 2020; Chen et al., 2021). Prolonged flooding accelerates the leaching rate and the degree of fragmentation of leaves (Wallis and Raulings, 2011), and sufficient soil water content can also maintain a high colonization capacity and vitality of bacteria or fungi (Gessner et al., 2010), thereby accelerating the decomposition rate. The rate of foliage mass loss of pond cypress was the largest during the initial stage of decomposition (0–10 days) (Figure 1), which was caused by the rapid leaching of water-soluble organic and inorganic compounds at that time (Aponte et al., 2012), as well as the high temperature in summer when the experiment began (Kennedy and El-Sabaawi, 2017). The foliage mass loss rate gradually decreased during 10–90 days, which may be caused by a decrease in the availability of nutrients needed for the microbial decomposition of leaves (Mora-Gómez et al., 2018). Meanwhile, the gradual decrease in environmental temperature during decomposition slows down the activity of microorganisms, thus reducing the mass loss rate (Davidson and Janssens, 2006). During the 90–180 days of decomposition, the mass loss of leaves gradually increased, but the rate slowed down. At this time, the components in the leaves that were available for microbial decomposition gradually decreased, and the easily decomposable substances were basically exhausted. Studies have shown that even though some microorganisms are still active at low temperatures, the diffusion of substrates and extracellular enzymes will be blocked to a certain extent (Davidson and Janssens, 2006). At this time, the decomposition process is in the biological metabolism course of microorganisms to the refractory components such as phenolic substances and lignin in the residual leaves (Mora-Gómez et al., 2018). Therefore, the decomposition rate of the foliage of pond cypress increases with an increase in water content.
Effects of flooding treatments on foliage nutrient dynamics of pond cypress
Nutrient dynamics during foliage decomposition are related to litter input composition (quality) and microbial activities (Bradford et al., 2016; Lidman et al., 2017). Microorganisms need a corresponding proportion of elements to cooperate in the process of decomposition. If they lack a certain element, it can also be obtained from the external environment, such as the soil and atmosphere (Bani et al., 2018). As decomposition proceeded, the N content of the leaves in the two flooded treatment groups, T4 and T5, increased significantly because there were some nitrogen-fixing microorganisms in the leaves. When the nutrient content of the plant residue itself cannot meet the growth needs of the microorganisms, the microorganisms will absorb the N in the external environment and fix it, thereby increasing the N content in the leaves (Bani et al., 2018; Mora-Gómez et al., 2018). The N content of the two flooded treatment groups was significantly higher than that of the T1 group (control), while the N content of the T2 group was significantly lower than that of the T1 group (Figure 2). That is, flooding promoted an increase in the N content of the leaves, which may be closely related to microbial activity and water movement (Soong et al., 2014). However, the total amount of N in the leaves of each water treatment group showed a continuous decreasing trend during the experiment, which was different from the change in N content of the leaves. This may be caused by the non-synchronization of the mass-loss rate and the rate of element release from pond cypress (Gessner et al., 2010; Kou et al., 2020).
The dynamics of P in the leaves were unlike those of N; both the content of P and the total amount of P increased initially and then decreased during the experiment. At the end of the experiment, the content of P of the leaves in each treatment group was significantly lower than the initial value of the experiment. In comparison, P content in the two flooded treatment groups was significantly lower than that in the three non-flooded treatment groups (Figure 2). Previous studies have found that the release of N and P occurs in a short time after litter enters the water body, which is caused by the decomposition of plant residues by microorganisms (Mora-Gómez et al., 2018). When the nutrients fixed by the microorganisms and bacteria in the water body can meet their own growth and reproduction needs, the community is in a stable state, and the microorganisms begin to bio-decompose the leaves (Soong et al., 2014), which shows that the content of N and P in water increases rapidly at the initial stage of decomposition (Figure 3). During 0–90 days of decomposition, the foliage P content increased continuously after the initial rapid leaching (Figure 2), which may be due to the reverse process of the mycelium of pathogens such as fungi and bacteria caused by bio-fixation to migrate P from the soil to the decomposed part of the litter (Gessner et al., 2010; Stutter et al., 2012). During 90–180 days of decomposition, especially at the end of the experiment, the P content of the leaves in each treatment group was significantly lower than its initial value because a large amount of soluble P mainly exists in the form of bioactive substances in plants, such as phosphate radicals or phosphoric acid compounds, which are easier to release by leaching (Jacobson et al., 2011).
During the decomposition process, the K content of the leaves of the three non-flooded treatment groups was relatively stable. In comparison, the K content of the two flooded treatment groups decreased sharply at the beginning of the experiment (0–10 days) (Figure 2). In terms of the total amount of K, the K of the leaves in the three non-flooded treatments showed a declining trend, while the K in the two flooded treatments showed a fluctuating trend. This is because K mainly exists in the form of inorganic salts in plants, which are released more easily than C, N, and P (Jacobson et al., 2011). In addition, K is easily soluble in water, resulting in greater mass loss based on water conditions during leaching, and it has poor stability and high mobility in the ecosystem (Jacobson et al., 2011). Therefore, compared with the terrestrial environment, the change characteristics of the K of the leaves of the pond cypress under flooding conditions were also different.
Dynamic characteristics of N and P content in the overlying water
The soaking decomposition experiments of dominant herbaceous vegetation in the TGR area showed that N and P released by herbaceous vegetation were one of the main nutrient sources in the TGR, which has a significant impact on the water environment and may increase the probability of water eutrophication (Xiao et al., 2017). Our study showed that TP and PO43−-P contents in the overlying water in the leaf addition groups, T4 and T5, were significantly higher than those in two groups without leaves (Figure 3), indicating that the P content in water was mainly affected by P mineralization in leaves during the early decomposition stage. During 10–180 days of decomposition, the contents of TP and PO43−-P in water showed a decreasing trend, which may be caused by the gradual migration of a part of the P in the waterbody to the lower soil during the process of decomposition or the loss of part of the P to the air in the form of phosphine (Stutter et al., 2012).
As a result of microbial activity, the N of foliage was possibly released into water or transported in other forms such as gases (Zhang and Zak, 1995). The dynamic change of the N content in the overlying water was more complicated when compared with the P in the overlying water (Figure 3). The total amount of N in the leaves was in a state of net release (Table 3), and the content of TN, NO3−N, and NH4+-N in the overlying water increased rapidly at the beginning of the experiment (0–10 days) (Figure 3). During the decomposition process, the released N from the leaf enters the overlying water; meanwhile, energy flow and material exchange are constantly occurring between the overlying water body and the underlying soil under flooding conditions (Soong et al., 2014). During 10–180 days of decomposition, the TN, NO3−N, and NH4+-N content generally showed a downward trend, which was possibly caused by the nitrification and denitrification of N during the flooding period; in particular, denitrification would cause an overflow of N in the overlying water in the form of gas (Vymazal, 2007). In addition, other complex effects on N may occur in water, such as microbial absorption, adsorption, precipitation, and complexation, which may also be the reason the N content in water gradually decreased as decomposition proceeded (Pascoal and Cassio, 2004).
The contents of N and P in the overlying water of the two flooded treatment groups, T4 and T5, increased rapidly at the initial stage of the decomposition and then decreased as the decomposition proceeded (Figure 3). Nevertheless, at the end of the experiment, the N and P contents of the overlying water in the two flooded treatment groups were still significantly higher than in the respective leafless groups. The decomposition of the leaves of pond cypress in water will increase the content of nutrients N and P in the overlying water, which may cause potential adverse effects on the aquatic environment of the reservoir.
Conclusion
The flooding environment significantly promoted the decomposition of pond cypress, and there were significant differences among the different treatments. Different water treatments resulted in varying effects on the content dynamics of different elements in the leaves. Generally, the water treatments had little effect on the leaf C content during decomposition. The N content of leaves increased during the experiment, while the P content of leaves decreased during the experiment, and flooding may have exacerbated these changes. As compared to the two flooded treatment groups, the leaves in the three non-flooded treatment groups contained a higher level of K. At the end of the experiment, the C, N, P, and K contents of the leaves of pond cypress all demonstrated a net release. During the early stages of decomposition, the higher water content in the leaves would release N and P, thereby increasing the levels of N and P in the overlying water. At the end of the experiment, however, there was a decrease in the amount of N and P in the overlying water. It was worth noting that the N and P contents of the leaves in the overlying water in the leaf addition group remained significantly higher than in the leafless group at the end of the experiment, which means that the N and P released by the leaves during flooding decomposition would have a potential negative impact on the aquatic environment. The TGR area is a unique wetland ecosystem with a complex ecological environment. The studies of foliage decomposition and nutrient release from a single dominant tree species under different water conditions can provide valuable information on flooding decomposition as well as nutrient dynamics from riparian vegetation in the TGR area. Thereafter, it is possible to examine the possibility of expanding the number of suitable tree species for reconstructions and conducting long-term, in-depth experimental research in situ at the reservoir site.
Data availability statement
The raw data supporting the conclusions of this article will be made available by the authors, without undue reservation.
Author contributions
ZC, MA, and CL contributed to the conception and design of the study. ZC and MA organized the database. ZC and HS collected research samples. ZC, HS, and MA performed the statistical analysis. ZC and MA wrote and revised the manuscript. MA and CL supervised the research. All authors contributed to the article and approved the submitted version.
Funding
This research has been supported by the Chongqing Municipality Key Forestry Research Project (No. 2021-9), Chongqing Municipality Housing and Urban Construction Committee (No. Chengkezi 2022-6-3), Forestry Extension Project of China Central Finance (No. Yulinketui 2020-2), the Science Foundation of College of Life Sciences of Southwest University (No. 20212005406201), International Young Talents Program (No. QN2022168001L), Ningxia Key Research and Development Project (No. 2020BFG03006), and Ningxia Natural Science Foundation Project (No. 2020AAC03107).
Acknowledgments
We wish to thank all the partners in the research team and all the people who helped with the fieldwork throughout the experiments.
Conflict of interest
The authors declare that the research was conducted in the absence of any commercial or financial relationships that could be construed as a potential conflict of interest.
Publisher’s note
All claims expressed in this article are solely those of the authors and do not necessarily represent those of their affiliated organizations, or those of the publisher, the editors and the reviewers. Any product that may be evaluated in this article, or claim that may be made by its manufacturer, is not guaranteed or endorsed by the publisher.
References
Alvim, E. A. C. C., de Oliveira Medeiros, A., Rezende, R. S., and Gonçalves, J. F. (2015). Small leaf breakdown in a Savannah headwater stream. Limnologica 51, 131–138. doi:10.1016/j.limno.2014.10.005
Anderson, J. T., and Smith, L. M. (2002). The effect of flooding regimes on decomposition of Polygonum pensylvanicum in playa wetlands (Southern Great Plains, USA). Aquat. Bot. 74, 97–108. doi:10.1016/S0304-3770(02)00049-9
Aponte, C., García, L. V., and Marañón, T. (2012). Tree species effect on litter decomposition and nutrient release in Mediterranean oak forests changes over time. Ecosystems 15, 1204–1218. doi:10.1007/s10021-012-9577-4
Arif, M., Behzad, H. M., Tahir, M., and Changxiao, L. (2022a). Environmental literacy affects riparian clean production near major waterways and tributaries. Sci. Total Environ. 834, 155476. doi:10.1016/j.scitotenv.2022.155476
Arif, M., Behzad, H. M., Tahir, M., and Li, C. (2022b). The impact of ecotourism on ecosystem functioning along main rivers and tributaries: Implications for management and policy changes. J. Environ. Manage. 320, 115849. doi:10.1016/j.jenvman.2022.115849
Arif, M., Jie, Z., Tahir, M., Xin, H., and Changxiao, L. (2022c). The impact of stress factors on riparian and drawdown zones degradation around dams and reservoirs. Land Degrad. Dev. 4310, 2127–2141. doi:10.1002/ldr.4310
Bani, A., Pioli, S., Ventura, M., Panzacchi, P., Borruso, L., Tognetti, R., et al. (2018). The role of microbial community in the decomposition of leaf litter and deadwood. Appl. Soil Ecol. 126, 75–84. doi:10.1016/j.apsoil.2018.02.017
Behzad, H. M., Jiang, Y., Arif, M., Wu, C., He, Q., Zhao, H., et al. (2022). Tunneling-induced groundwater depletion limits long-term growth dynamics of forest trees. Sci. Total Environ. 811, 152375. doi:10.1016/j.scitotenv.2021.152375
Bradford, M. A., Berg, B., Maynard, D. S., Wieder, W. R., and Wood, S. A. (2016). Understanding the dominant controls on litter decomposition. J. Ecol. 104, 229–238. doi:10.1111/1365-2745.12507
Chen, Z., Wang, C., Chen, X., Yuan, Z., Song, H., and Li, C. (2020). Heterogeneous leaves of predominant trees species enhance decomposition and nutrient release in the riparian zone of the Three Gorges Reservoir. Sci. Rep. 10, 17382–17389. doi:10.1038/s41598-020-74062-4
Chen, Z., Arif, M., Wang, C., Chen, X., and Li, C. (2021). Effects of hydrological regime on foliar decomposition and nutrient release in the riparian zone of the Three Gorges Reservoir, China. Front. Plant Sci. 12, 661865. doi:10.3389/fpls.2021.661865
Davidson, E. A., and Janssens, I. A. (2006). Temperature sensitivity of soil carbon decomposition and feedbacks to climate change. Nature 440, 165–173. doi:10.1038/nature04514
Ding, D., Liu, M., Arif, M., Yuan, Z., Li, J., Hu, X., et al. (2021). Responses of ecological stoichiometric characteristics of carbon, nitrogen, and phosphorus to periodic submergence in mega-reservoir: Growth of Taxodium distichum and Taxodium ascendens. Plants 10 (10), 2040. doi:10.3390/plants10102040
Ding, D., Arif, M., Liu, M., Li, J., Hu, X., Geng, Q., et al. (2022). Plant-soil interactions and C:N:P stoichiometric homeostasis of plant organs in riparian plantation. Front. Plant Sci. 13, 979023–979117. doi:10.3389/fpls.2022.979023
Dosskey, M. G., Vidon, P., Gurwick, N. P., Allan, C. J., Duval, T. P., and Lowrance, R. (2010). The role of riparian vegetation in protecting and improving chemical water quality in Streams1. J. Am. Water Resour. Assoc. 46, 261–277. doi:10.1111/j.1752-1688.2010.00419.x
Gessner, M. O., Swan, C. M., Dang, C. K., McKie, B. G., Bardgett, R. D., Wall, D. H., et al. (2010). Diversity meets decomposition. Trends Ecol. Evol. 25, 372–380. doi:10.1016/j.tree.2010.01.010
Gul, Z., Tang, Z.-H., Arif, M., and Ye, Z. (2022). An insight into abiotic stress and influx tolerance mechanisms in plants to cope in saline environments. Biology 11 (4), 597. doi:10.3390/biology11040597
He, X., Wang, T., Wu, K., Wang, P., Qi, Y., Arif, M., et al. (2021). Responses of swamp cypress (Taxodium distichum) and Chinese willow (Salix matsudana) roots to periodic submergence in mega-reservoir: Changes in organic acid concentration. Forests 12 (2), 203. doi:10.3390/f12020203
Hu, X., Xie, T., Arif, M., Ding, D., Li, J., Yuan, Z., et al. (2021). Response of annual herbaceous plant leaching and decomposition to periodic submergence in mega-reservoirs: Changes in litter nutrients and soil properties for restoration. Biology 10, 1141. doi:10.3390/biology10111141
Hu, X., Arif, M., Ding, D., Li, J., He, X., and Li, C. (2022). Invasive plants and species richness impact litter decomposition in riparian zones. Front. Plant Sci. 13, 955656–955714. doi:10.3389/fpls.2022.955656
Jacobson, T. K. B., Bustamante, M. M. D. C., and Kozovits, A. R. (2011). Diversity of shrub tree layer, leaf litter decomposition and N release in a Brazilian Cerrado under N, P and N plus P additions. Environ. Pollut. 159, 2236–2242. doi:10.1016/j.envpol.2010.10.019
Kennedy, K. T. M., and El-Sabaawi, R. W. (2017). A global meta-analysis of exotic versus native leaf decay in stream ecosystems. Freshw. Biol. 62, 977–989. doi:10.1111/fwb.12918
Kou, L., Jiang, L., Hättenschwiler, S., Zhang, M., Niu, S., Fu, X., et al. (2020). Diversity-decomposition relationships in forests worldwide. ELife 9, e55813. doi:10.7554/eLife.55813
Li, J., Li, L., Arif, M., Ding, D., Hu, X., Zheng, J., et al. (2021). Artificial plantation responses to periodic submergence in massive dam and reservoir riparian zones: Changes in soil properties and bacterial community characteristics. Biology 10, 819. doi:10.3390/biology10080819
Lidman, J., Jonsson, M., Burrows, R. M., Bundschuh, M., and Sponseller, R. A. (2017). Composition of riparian litter input regulates organic matter decomposition: Implications for headwater stream functioning in a managed forest landscape. Ecol. Evol. 7, 1068–1077. doi:10.1002/ece3.2726
Migliorini, G. H., and Romero, G. Q. (2020). Warming and leaf litter functional diversity, not litter quality, drive decomposition in a freshwater ecosystem. Sci. Rep. 10, 20333. doi:10.1038/s41598-020-77382-7
Mora-Gómez, J., Duarte, S., Cássio, F., Pascoal, C., and Romaní, A. M. (2018). Microbial decomposition is highly sensitive to leaf litter emersion in a permanent temperate stream. Sci. Total Environ. 621, 486–496. doi:10.1016/j.scitotenv.2017.11.055
Muhammad, A., Behzad, H. M., Tahir, M., and Changxiao, L. (2022). Nature-based tourism influences ecosystem functioning along waterways: Implications for conservation and management. Sci. Total Environ. 842, 156935. doi:10.1016/j.scitotenv.2022.156935
Olson, J. S. (1963). Energy storage and the balance of producers and decomposers in ecological systems. Ecology 44, 322–331. doi:10.2307/1932179
Pascoal, C., and Cassio, F. (2004). Contribution of fungi and bacteria to leaf litter decomposition in a polluted river. Appl. Environ. Microbiol. 70 (9), 5266–5273. doi:10.1128/aem.70.9.5266-5273.2004
Peng, C., Zhang, L., Qin, H., and Li, D. (2014). Revegetation in the water level fluctuation zone of a reservoir: An ideal measure to reduce the input of nutrients and sediment. Ecol. Eng. 71, 574–577. doi:10.1016/j.ecoleng.2014.07.078
Rincón, J., and Covich, A. (2014). Effects of insect and decapod exclusion and leaf litter species identity on breakdown rates in a tropical headwater stream. Rev. Biol. Trop. 2, 143–154. doi:10.15517/rbt.v62i0.15784
Simões, S., Martínez, A., Gonçalves, A. L., Capela, N., Alves, J., da Silva, A. A., et al. (2021). Annual patterns of litter decomposition in the channel and riparian areas of an intermittent stream. Aquat. Ecol. 55, 519–526. doi:10.1007/s10452-021-09841-w
Soong, J. L., Calderón, F. J., Betzen, J., and Cotrufo, M. F. (2014). Quantification and ftir characterization of dissolved organic carbon and total dissolved nitrogen leached from litter: A comparison of methods across litter types. Plant Soil 385, 125–137. doi:10.1007/s11104-014-2232-4
Stutter, M. I., Shand, C. A., George, T. S., Blackwell, M. S. A., Bol, R., MacKay, R. L., et al. (2012). Recovering phosphorus from soil: A root solution? Environ. Sci. Technol. 46, 1977–1978. doi:10.1021/es2044745
Sun, Z., Mou, X., and Liu, J. S. (2012). Effects of flooding regimes on the decomposition and nutrient dynamics of Calamagrostis angustifolia litter in the Sanjiang Plain of China. Environ. Earth Sci. 66, 2235–2246. doi:10.1007/s12665-011-1444-7
Vymazal, J. (2007). Removal of nutrients in various types of constructed wetlands. Sci. Total Environ. 380, 48–65. doi:10.1016/j.scitotenv.2006.09.014
Wallis, E., and Raulings, E. (2011). Relationship between water regime and hummock-building by Melaleuca ericifolia and Phragmites australis in a brackish wetland. Aquat. Bot. 95, 182–188. doi:10.1016/j.aquabot.2011.05.006
Wang, Q., Yuan, X., Liu, H., Zhang, Y., Cheng, Z., and Li, B. (2012). Effect of long-term winter flooding on the vascular flora in the drawdown area of the Three Georges Reservoir, China. Pol. J. Ecol. 60, 95–106.
Wang, C., Li, C., Wei, H., Xie, Y., and Han, W. (2016). Effects of long-term periodic submergence on photosynthesis and growth of Taxodium distichum and Taxodium ascendens saplings in the hydro-fluctuation zone of the Three Gorges Reservoir of China. Plos One 11, e0162867. doi:10.1371/journal.pone.0162867
Wang, C., Xie, Y., Ren, Q., and Li, C. (2018). Leaf decomposition and nutrient release of three tree species in the hydro-fluctuation zone of the Three Gorges Dam Reservoir, China. Environ. Sci. Pollut. Res. 25, 23261–23275. doi:10.1007/s11356-018-2357-8
Wei, F. (2002). Methods for monitoring and analysis of water and wastewater. Fourth Edition. Beijing: China Environmental Science Press.
Xiao, L., Zhu, B., Nsenga Kumwimba, M., and Jiang, S. (2017). Plant soaking decomposition as well as nitrogen and phosphorous release in the water-level fluctuation zone of the Three Gorges Reservoir. Sci. Total Environ. 592, 527–534. doi:10.1016/j.scitotenv.2017.03.104
Xu, X., Tan, Y., and Yang, G. (2013). Environmental impact assessments of the Three Gorges Project in China: Issues and interventions. Earth Sci. Rev. 124, 115–125. doi:10.1016/j.earscirev.2013.05.007
Xie, Y., Xie, Y., Hu, C., Chen, X., and Li, F. (2016). Interaction between litter quality and simulated water depth on decomposition of two emergent macrophytes. J. Limnol. 75 (1), 36–43. doi:10.4081/jlimnol.2015.1119
Yu, X., Ding, S., Lin, Q., Wang, G., Wang, C., Zheng, S., et al. (2020). Wetland plant litter decomposition occurring during the freeze season under disparate flooded conditions. Sci. Total Environ. 706, 136091. doi:10.1016/j.scitotenv.2019.136091
Yuan, X., Zhang, Y., Liu, H., Xiong, S., Li, B., and Deng, W. (2013). The littoral zone in the three Gorges reservoir, China: Challenges and opportunities. Environ. Sci. Pollut. Res. 20, 7092–7102. doi:10.1007/s11356-012-1404-0
Yuan, Z. X., Ni, X. L., Arif, M., Dong, Z., Zhang, L. M., Tan, X., et al. (2021). Transcriptomic analysis of the photosynthetic, respiration, and aerenchyma adaptation strategies in bermudagrass (Cynodon dactylon) under different submergence stress. Int. J. Mol. Sci. 22, 7905. doi:10.3390/ijms22157905
Yuancai, Q., Muhammad, A., Dong, Z., Ting, W., Qin, Y., Bo, P., et al. (2022). The effect of hydrological regimes on the concentrations of nonstructural carbohydrates and organic acids in the roots of Salix matsudana in the Three Gorges Reservoir, China. Ecol. Indic. 142, 109176. doi:10.1016/j.ecolind.2022.109176
Yue, K., Yang, W., Peng, C., Peng, Y., Zhang, C., Huang, C., et al. (2016). Foliar litter decomposition in an alpine forest meta-ecosystem on the eastern Tibetan Plateau. Sci. Total Environ. 566-567, 279–287. doi:10.1016/j.scitotenv.2016.05.081
Zhang, M., Cheng, X., Geng, Q., Shi, Z., Luo, Y., and Xu, X. (2019). Leaf litter traits predominantly control litter decomposition in streams worldwide. Glob. Ecol. Biogeogr. 28, 1469–1486. doi:10.1111/geb.12966
Zhang, Q. T. T. U., and Zak, J. C. (1995). Effects of gap size on litter decomposition and microbial activity in a subtropical forest. Ecology 76, 2196–2204. doi:10.2307/1941693
Zheng, J., Muhammad, A., Zhang, S., Yuan, Z., Zhang, L., Dong, Z., et al. (2021a). The convergence of species composition along the drawdown zone of the Three Gorges Dam Reservoir, China: Implications for restoration. Environ. Sci. Pollut. Res. 28 (31), 42609–42621. doi:10.1007/s11356-021-13774-0
Keywords: hydro-fluctuation zone, conifers, leaf decomposition, nutrient dynamics, submergence environment
Citation: Chen Z, Song H, Arif M and Li C (2022) Effects of hydrological regime on Taxodium ascendens plant decomposition and nutrient dynamics in the Three Gorges Reservoir riparian zone. Front. Environ. Sci. 10:990485. doi: 10.3389/fenvs.2022.990485
Received: 15 July 2022; Accepted: 23 August 2022;
Published: 09 September 2022.
Edited by:
Jin Hur, Sejong University, South KoreaReviewed by:
Vladimir Razlutskij, State Scientific and Production Amalgamation “Scientific-Practical Center of the National Academy of Sciences of Belarus for Biological Resources”, BelarusHua Ma, Chongqing University, China
Copyright © 2022 Chen, Song, Arif and Li. This is an open-access article distributed under the terms of the Creative Commons Attribution License (CC BY). The use, distribution or reproduction in other forums is permitted, provided the original author(s) and the copyright owner(s) are credited and that the original publication in this journal is cited, in accordance with accepted academic practice. No use, distribution or reproduction is permitted which does not comply with these terms.
*Correspondence: Changxiao Li, bGljaGFuZ3hAc3d1LmVkdS5jbg==