- 1Instituto Politécnico Nacional, Escuela Nacional de Ciencias Biológicas, Lab. de Hidrobiología Experimental, México City, México
- 2Département de Chimie, Université de Montréal, Montréal, QC, Canada
The Valle de Bravo dam is an important source of drinking water supply for the Mexico City Conurbation (>26 million inhabitants) and is also a hotspot for nautical sports, recreational fishing, and tourism. However, anthropogenic pressures in the upper reaches of the basin have led to increased eutrophication and numerous harmful cyanobacterial blooms (HCBs). To determine the effect of abiotic variables on the characteristics of the phytoplanktonic community, as well as their influence on the diversity of toxigenic cyanobacteria and related cyanotoxins, we evaluated monthly variations in environmental factors, phytoplankton biovolume and composition and total microcystins in Valle de Bravo dam during the year of 2019. Overall, 75 phytoplanktonic species were identified, mostly belonging to the divisions Cyanobacteria, Chlorophyta, and Bacillariophyta. Cyanobacteria presented the highest biomass throughout the year, with water temperature and rainfall being the best correlated factors. The highest concentrations of total microcystins were recorded from July to September (maximum of 71 μg L−1), associated with the dominance of species such as M. smithii, M. aeruginosa, M. viridis M. flos-aquae, Aphanocapsa planctonica, and Dolichospermum crassum, and environmental factors such as rainfall, total dissolved solids, water temperature, and specific trace metals. In all months except December, the total cyanobacterial biovolume was above the World Health Organization alert level two, indicating potentially high risks to human health. The predominance of cyanobacteria and high biovolumes classified water quality as poor to very poor during most of the year. To avoid the development of HCBs in this dam, the contribution of nutrients by the tributary rivers and sewage must be controlled, since the poor-quality conditions of the water threaten the aquatic biota, the sports and recreation activities and affect the potable water supply.
Introduction
Eutrophication in limnetic ecosystems has increased in recent decades worldwide, threatening freshwater availability for human consumption and food production. Different human activities release waste streams, notably into epicontinental water bodies. Aquatic pollution caused by agrochemicals (fertilizer carryover and pesticides) and domestic wastewater (with and without treatment) cause an increase of mineral nutrients in the water. Of particular concern are phosphorus (P) and nitrogen (N), which are normally macronutrients limiting the growth of primary producers (phytoplankton and macrophytes); surplus of N and P increases the trophic state of aquatic ecosystems (Le Moal et al., 2019). Seasonal successions and shifts in phytoplankton communities have been well studied in inland water systems, and can be associated with eutrophication, water inflow and temperature, water stratification, (Lee et al., 2015), and consumption by invasive species.
Seasonal changes in N and P can also promote the development of harmful cyanobacterial blooms (HCBs) (Fernández et al., 2015), which can result in cyanotoxin production. Cyanotoxins are biologically active secondary metabolites that have different modes of action (hepatotoxic, neurotoxic, and cytotoxic) (Pineda-Mendoza et al., 2016; Buratti FM et al., 2017) and can pose severe health risks to aquatic biota, wildlife, cattle, and humans (Wood, 2016; Huisman et al., 2018; Svirčev, Z. et al., 2019). Microcystins (MCs) are the cyanobacterial toxins most frequently reported in freshwater environments, being synthetized by some species of the genus Microcystis, Nostoc, Dolichospermum, Planktothrix, and Synechocystis (Pineda-Mendoza et al., 2016). More than 85 different MCs have been reported, with microcystin-LR the most common (Pineda Mendoza et al., 2012). Human exposure to microcystins can occur through dermal contact and accidental ingestion during recreational and sport water activities, through the consumption of contaminated food, and also from drinking water containing cyanotoxins given that it is difficut to remove these toxins in water purification plants, (Smith et al., 2015). Exposure to cyanotoxins produce a wide range of symptoms including abdominal pain, vomiting, diarrhea, nausea, dizziness, muscle tremors and paralysis, fever, blurred vision, headache, and skin irritation. A well-known case is the 2014 Toledo water crisis (Ohio, United States) when an HCB in Lake Erie resulted in exceedances of the World Health Organization provisional guideline value for microcystins (1 μg L−1 in drinking water). This led to the issuance of ‘Do not drink’ advisories and more than 400,000 residents remained without access to potable tap water for several days (Steffen et al., 2017). Another case ocurred with the massive death of cattle exhibiting acute liver damage after drinking water from a reservoir in which microcystin-LR was detected at a concentration of 3 mg L−1 during a cyanobacterial bloom in Oregon, United States (Dreher et al., 2019). There is a critical need to understand the environmental factors most related with blooms of toxigenic cyanobacteria and other noxious phytoplankters, especially for those freshwater ecosystems that provide a major source of drinking water production.
The population of the Mexico City Conurbation (Metropolitan Area of the Valley of Mexico—Zona Metropolitana del Valle de México, ZMVM) has increased by more than 1,000% since 1940 (Pradilla, 2016), with a current population exceeding 26 million inhabitants (INEGI, 2020). Drinking water supplies for this megalopolis are obtained mainly from aquifers of the area. About 30% of the supply originates from the so-called Sistema Cutzamala, a set of seven reservoirs that includes the Valle de Bravo dam, which supplies 13 municipalities in Mexico City and 14 municipalities within the State of Mexico (CONAGUA and World Bank Group, 2015). Of the Sistema Cutzamala, the Valle de Bravo dam is the one with the highest capacity supplying 6 m3 s−1 and contributing 40% of the raw water (Carnero-Bravo et al., 2015) that enters the Los Berros Drinking Water Treatment Plant, from where it supplies the ZMVM (Mota, 2011). In addition to drinking water for human consumption, Valle de Bravo is an important area for recreation, tourism, sports activities, and commercial fishing.
The evolution of the trophic status of the Valle de Bravo reservoir has been very rapid. In 1980 it was classified as oligotrophic (Gaytán-Herrera et al., 2011), in 1987 as mesotrophic, and by 1992 its status was already eutrophic (Olvera, 1992). The main sources of nutrients in the reservoir are point discharges and allochthonous compounds incorporated mainly by the Amanalco River, which increased the concentration of N and P by more than 203% and 276%, respectively, in just a decade (Ramírez-Zierold et al., 2010). The Valle de Bravo reservoir is of monomictic type, with a marked seasonality for chlorophyll concentration; the highest primary productivity values were reported in the stratification season (Merino-Ibarra et al., 2008). The P and N budgets was extensively studied in VB dam by Ramírez-Zierold et al. (2010), measuring DO and ORP each 4 weeks from January 2002 to August 2005 in 17 sampling stations; they determined the loadings of 120.8 × 103 and 591.8 × 103 kg y−1 of P and N, respectively, condition that were deemed responsible of the accelerated change in the trophic status of this artificial lake.
Eutrophic condition in limnetic waterbodies is related with a high primary production, as observed in Valle de Bravo dam throughout the year; this condition differs notably from the seasonal blooms normally recorded in temperate lakes. The presence of permanent blooms does not necessarily indicate that toxigenic cyanobacteria are dominating in this dam, and it is possible that cyanotoxins synthesis and release can vary along the year, influenced by the environmental conditions and the phytoplankton dynamics.
In the present study, a monthly sampling was carried out for a full year at six different locations in the Valle de Bravo reservoir. This allowed us to determine the phytoplankton composition and biovolume, and their relationship with the physicochemical conditions in water and the environmental conditions. The development of toxigenic cyanobacteria and the synthesis and release of cyanotoxins were also monitored along the year. Though the phytoplankton community of the Valle de Bravo reservoir has been previously studied, it was performed monthly but in only one sampling station (Gaytán-Herrera et al., 2011), and monthly in five sampling stations almost two decades ago (Valeriano-Riveros et al., 2014), but none of these studies looked at the cyanotoxin concentrations and only one study reports phytoplankton biovolume. This is the first study to report on the relationship between specific richness and ecological diversity of phytoplankton that relates to the frequency and dominance of toxigenic cyanobacteria in this area throughout the year. The determination of water quality based on the different phytoplankton groups and biovolume is also a new contribution. The aim of this study was to determine how changes in the phytoplankton community may drive the cyanobacteria dominance, affect the variations in total microcystin concentrations, explore the effects in water quality based on the phytoplankton species and their relative biovolume. This information is crucial to determine the influence of environmental factors and water quality that shape the composition of the phytoplankton community and determine the variations of toxin-producing cyanobacteria.
Materials and methods
Study site description
The Valle de Bravo reservoir (Figure 1) is located in the eponymous municipality, in the State of Mexico (19°21′30″ N, 100°11′00″ W). The climate is temperate sub-humid and semi-warm, with an average annual temperature greater than 18°C (Olvera, 1992) and average annual rainfall of 836 mm with a rainy season ranging from June to September. The topography is characterized by hills with plains. The vegetation is composed of mixed pine-oak forests, low deciduous forests, pastures, and seasonal agriculture (Olvera-Viascán et al., 1998). The body of water has an approximate surface of 18.55 km2, a maximum depth of 38.6 m (average of 21 m) and an average volume of 391 million m3 (Monroy, 2004). This is classified as a warm monomictic dam, stratified from April to October, and well mixed from November to February (Ramírez-Zierold et al., 2010). This dam receives water from the tributaries Amanalco, Molino, Santa Mónica, González, and El Carrizal rivers, as well as from the Tizates river that collects wastewaters from irregular settlements around the reservoir; from these the Amanalco and Tizates rivers are the major contributors of pollutants to the dam (Olvera 1992; Banderas and González-Villela, 2019).
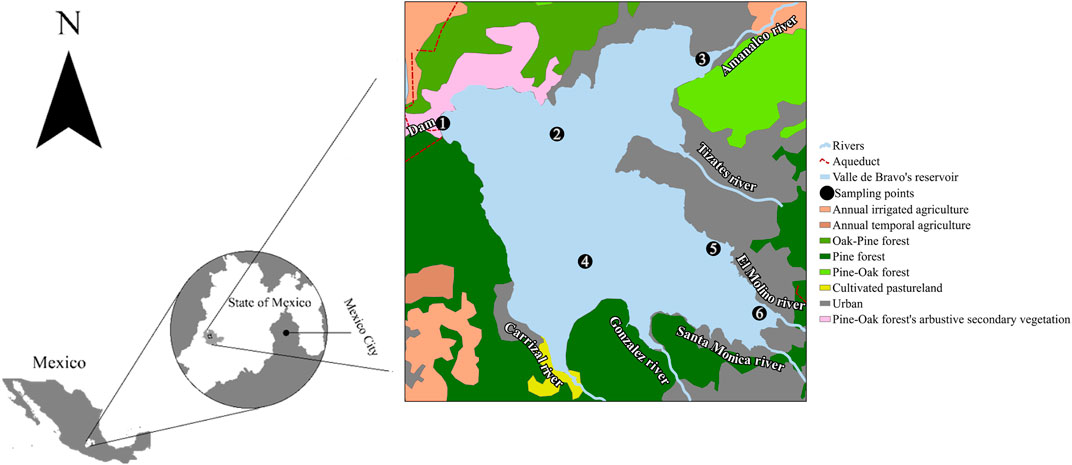
FIGURE 1. General location of Valle de Bravo dam, Mexico, and geographic distribution of the six sampling sites (1–6) across the reservoir.
Six sampling locations were selected to represent the main conditions prevalent in this waterbody, as well as the influence of the tributaries and the population established in the locality (Figure 1). Site #1 is closest to the curtain of the reservoir where the maximum depth is located, while site #2 is approximately at the central point of the reservoir. Site #3 is in the shallower part of the dam and receive waters from the Rio Amanalco, including agricultural discharges from the upper part of the stream. Site #4 is influenced by waters of the rivers Carrizal and González. Site #5 is the closest to the urban area of Valle de Bravo and receives discharges from the urban area and from the tourism facilities. Finally, site #6 is influenced by inputs from the rivers Santa Monica and El Molino.
Sample collections
Sample collection was carried out monthly in 2019, at the six sites in the reservoir (Figure 1).
Duplicate water samples for metals and total microcystin analysis were collected, processed, and stored as detailed in Supplementary Table S1; these analysis were carried out by the Université de Montreal (UdeM), Canada and collaborators. Water samples for the determination of dissolved microcystins were vacuum filtered in situ, using GHP 0.45 µm filters; biomass retained in filter were processed for the determination of intracellular microcystins; the sum of that determined in the filtrated water and in biomass provided the total concentration of microcystins. The samples were kept at −70°C ultra-frozen in a cooler with dry ice and periodically shipped to UdeM. Single water samples for other chemical determinations (see below) were collected and stored in a cooler.
Phytoplankton samples were obtained at each site by filtering 50 L of surface water using a phytoplankton net (25 µm mesh size). These samples were preserved with 1% acidic Lugol solution and were kept protected from light exposure (Supplementary Table S1).
Water quality parameters
Abiotic variables were measured in situ at the water surface and subsurface (1 m depth) with a Hanna® (HI9829) multiparametric probe, including water temperature (°C), pH, conductivity (μS cm−1), salinity (ups), total dissolved solids (TDS, mg L−1), dissolved oxygen (DO, mg L−1), oxidation-reduction potential (ORP, mV), and turbidity (NTU). The transparency was measured with a Secchi disk (cm). The other environmental parameters recorded were air temperature (°C), barometric pressure (mm Hg), relative humidity (%), wind speed (Km h−1) and direction, cloudiness, and UV radiation. Dam water level (hm3) and precipitation data (mm) were collected from the webpages belonging to the National Water Information System and the National Meteorological Service, respectively (National Water Information System, 2020; National Weather Service, 2020). In situ measurements of chlorophyll concentrations (μg L−1) and phycocyanin (μg L−1) were recorded using fluorometry (AlgaeTorch®).
In the laboratory, the concentration of total and dissolved nitrogen (DN), nitrates, nitrites, ammonium, total and dissolved phosphorus (DP), soluble reactive phosphorus (SRP), total and dissolved organic carbon, hardness, and chloride (all in mg L−1) were determined spectrophotometrically by HACH® (HACH DR-6000).
Trace metals and metalloids
Concentrations of the following metals and metalloids (μg L−1) were measured in surface water: aluminum (Al), barium (Ba), cadmium (Cd), chromium (Cr), cobalt (Co), copper (Cu), iron (Fe), magnesium (Mg), manganese (Mn), molybdenum (Mo), nickel (Ni), zinc (Zn), potassium (K), and sodium (Na). Water samples (125 ml) were collected in HDPE bottles, supplemented with 2% nitric acid (HNO3), transported, and stored at room temperature prior ICP-MS analysis. Total extractable metals were analyzed using standardized procedures by Bureau Veritas Laboratories (Mississauga and Montreal, Canada) accredited to ISO/IEC 17025:2005 using standardized procedures (reference EPA 60208 m).
Total microcystins
Surface water samples were analyzed for total microcystins (ΣMC) via a Lemieux-von Rudloff oxidation, which generates 2-methyl-3-methoxy-4-phenylbutyric acid (MMPB) from oxidative cleavage of most microcystins (Wu et al., 2009). As standards for individual microcystins are available to only a small fraction of the microcystins, analysis of total microcystins may be more suitable to capture the entirety of microcystins in the samples, even though not all microcystins would form MMPB. Details on analytical method optimization and validation are provided elsewhere (Munoz et al., 2017). Surface water samples were supplemented with potassium permanganate (50 mM) and sodium periodate (50 mM) and adjusted to pH 9 with potassium carbonate. After magnetic stirring for 1 h, the oxidation reaction was quenched with sodium bisulfite. A 1-ml aliquot was analyzed by on-line solid-phase extraction (on-line SPE) coupled to ultra-high-performance liquid chromatography tandem mass spectrometry (Thermo TSQ Quantiva UHPLC-MS/MS). The deuterated isotopologue D3-MMPB was used as the internal standard. The method limit of detection was 0.005 μg L−1 ΣMC. Matrix-matched calibration curves were constructed in MC-free lake surface water and used for quantification, with suitable linearity performance (R2 > 0.99). Matrix spikes were run in each LC-MS/MS batch sequence and were required to fall within ± 30% of the true value. Participation in a recreational water interlaboratory proficiency study (Abraxis/Eurofins) also attested to the accuracy performance, with ΣMC within ± 30% of the consensus value.
Phytoplanktonic diversity and biovolume
The taxonomic identification was performed by microscopic analysis with an Olympus CKX53 phase contrast microscope directly in the Lugol-preserved samples. Microphotographs were obtained and the CellSens software (Olympus®) was used for morphological analysis and dimension assessment. The identification was carried out at the lowest possible taxonomic level using morphological criteria and based on specialized literature (Komárek and Fott, 1983; Krammer and Lange-Bertalot, 1988; Komárek and Anagnostidis, 1999; Komárek and Anagnostidis, 2005; Komárek and Komárkova, 2006; Taylor et al., 2007; Komárek and Zapomelová, 2007; Komárek and Zapomelová, 2008; Bellinger and Sigee, 2010; Komárek et al., 2013; Moestrup and Calado, 2018). For the dominant taxa, the geometric biovolume was determined as proposed by Hillebrand et al. (1999) using the software ImageJ 1.53 K with Java 1.8.0_172 (64 bits). The specific biovolume was multiplied by the number of organisms quantified according to the method of Lackey drop or micro-transects methods (American Public Health Association (Apha), 1999) to obtain the total biovolume by species identified at each sampling point.
Water quality index (Phytoplankton Community Index)
The ecological condition regarding the water quality determined by the phytoplankton community and the dominance in biovolume of the main groups, was established using the Phytoplankton Community Index (PhyCOI) (Katsiapi et al., 2016). The PhyCOI was calculated as follows:
TB = Total Biovolume of phytoplankton, according to Phillips et al. (2013).
WG = World Health Organization Guidelines, according to Bartram et al. (1999).
NB = Nygaard Biomass (Biovolume) Index (Katsiapi et al., 2016).
NS = Nygaard Species Index (Nygaard, 1949).
Both Nygaard Indexes were calculated as follows:
QG = Number of species in each quality group, determined with the equation:
According to the PhyCOI index, the water quality in the studied waterbody can be classified as: Very Poor (0.7–1), Poor (>1–2), Regular (>2–3), Good (>3–4), or Excellent (>4–5).
Statistical analyses
All abiotic variables were subjected first to a correlation analysis to eliminate those with co-linearity. Principal component analysis (PCA) was performed, selecting only variables that had a communality (common variance) greater than 0.5, which resulted in the selection of 20 abiotic variables for the PCA. Biological and ecological variables such as specific richness (S), biovolume (mm3 L−1), Shannon’s exponential [Exp(H′)], Simpson’s inverse (1/D) and Pielou equity (J′) were calculated with the R Studio software (R Core Team, 2021) using the vegan R package (Oksanen et al., 2020). To elucidate temporal changes in biological variables, Kruskal–Wallis analyses were performed with multiple post-hoc comparisons using the R-package agricolae (de Mendiburu and Yassen, 2020). The canonical correspondence analysis (CCA) was developed with the PAST version 4 program (Hammer et al., 2001). Correlations between biotic and abiotic variables were investigated with the R-package correlation (Makowski et al., 2019). The Phytoplanktonic Community Index (PhyCOI) was calculated for each sample and the water quality was compared among months by conducting a Kruskal–Wallis test and post-hoc multiple comparisons.
Results
Water quality parameters
Figure 2 shows the physicochemical parameters measured across the six sampling sites throughout the year. Water temperature, turbidity, TDS, conductivity, and pH had the highest values during the stratification season (February to October). The average water temperature in the reservoir was 22.0 ± 0.2°C, with an average low of 18.8°C in February and a high of 24.7°C in July and August. The rainfall season ranged from July to October, with a high of 207.4 mm in July. Water temperature and rainfall are environmental factors that responded directly to regional seasonality in this subtropical location. Dissolved nitrogen, nitrates, ammonium, TP, DP, and SRP in general have low values during the stratification season (coincident with the reduction in the water level) and increased during the mixing period. Variations in the water level are related to the management of the reservoir by the National Water Commission (CONAGUA), the entity responsible for the management and regulation of Mexico’s water resources. Redox potential decreased continuously from January to November, coincidently with the stratification period.
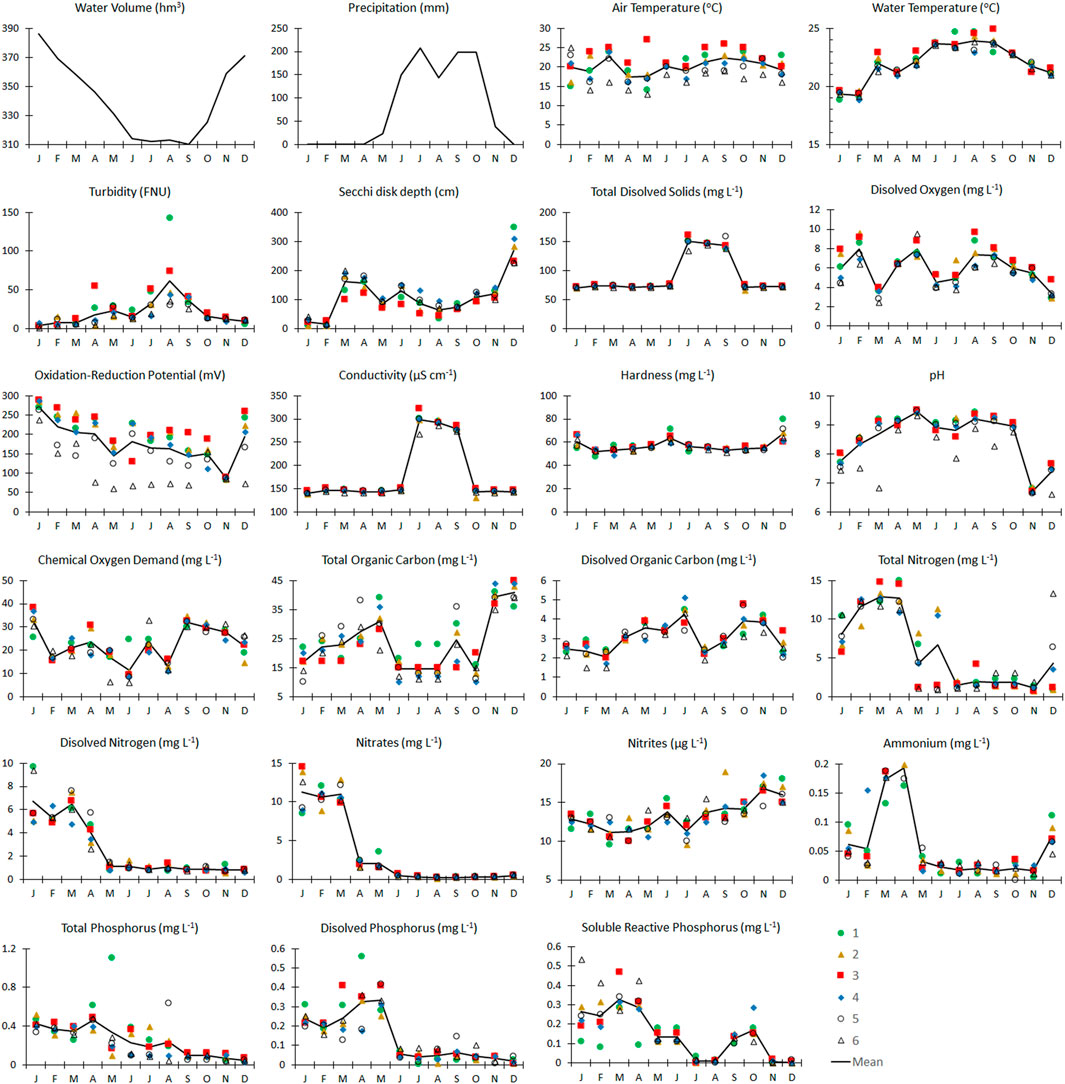
FIGURE 2. Monthly variations in chemical water quality, environmental factors, and water level in Valle de Bravo dam during 2019. Values in each station and average (solid line) except for pluvial precipitation and water level in the dam. Color legend represents the site number.
As shown in Figure 2, variations along the year were recorded and differences among sampling points were observed for most parameters. Increases in pH values could be related to the phytoplankton growth during the peaks of primary productivity through the consumption of HCO3−1 (as C source). Dissolved oxygen, COD, DOC, and TOC followed no consistent patterns along the year. Secchi disk transparency was always lower than 2 m, except for December when values higher than 3 m were observed; this low transparency was related with high phytoplankton biomass abundance, typical of the eutrophic condition in this dam.
Total N and P, as well as some readily usable forms of these nutrients were higher during the first 4 months of the year and could be the responsible for the high primary productivity observed during the warm months.
Trace metals and metalloids
Variation of concentration of micronutrients and non-essential metals along the year are shown in Figure 3. The concentration of nutrients such as K, Mg, Mn, Cu, and Fe varied along the year without any regular pattern; Zn was only detected in 3 months probably because this metal was caught and retained by phytoplankton. Non-essential metals such as aluminum increased in concentration from June to November (average 85 ± 10 μg L−1) coinciding with the lowest N and P concentrations (Figure 2). Potentially toxic concentrations of essential metals, such as Cu (0.58 ± 0.15 μg L−1), and the non-essential Al were recorded between March and October, although the values did not exceed the maximum permissible limits set out in the Mexican guideline NOM-001-SEMARNAT-1996 (4 mg L−1) (Ministry of the Environment and Natural Resources (Semarnat), 1996).
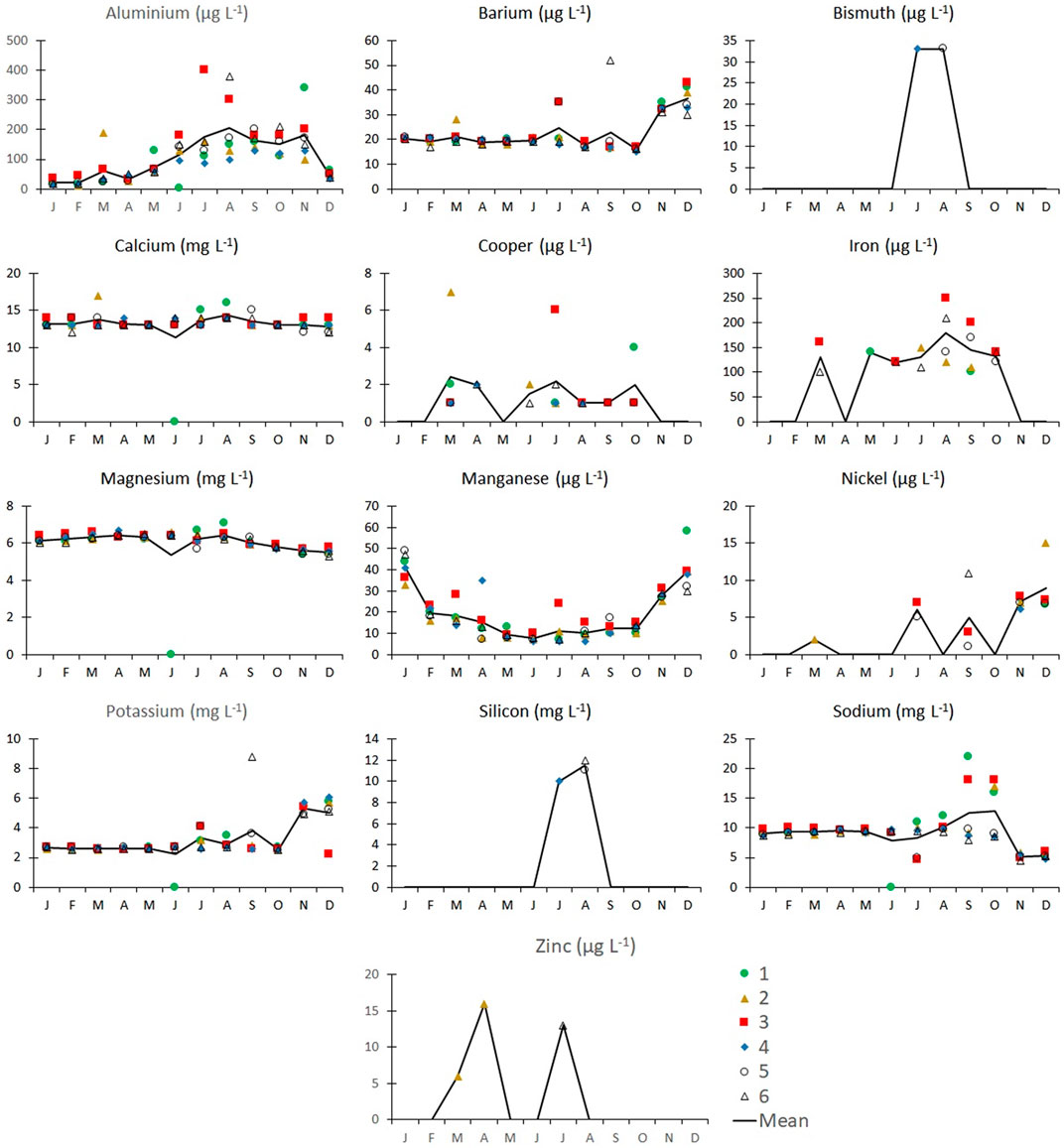
FIGURE 3. Monthly variation in metals and metalloids measured in six sampling sites in Valle de Bravo dam during 2019. Values in each sampling station and average value (solid line). Color legend represents the site number.
Total microcystins
Total microcystins (∑MC, MMPB oxidation method) were detected in all samples, with a concentration range of 1.5–71 μg L−1 (overall average: 11 μg L−1; median: 5.2 μg L−1). A marked seasonality was observed (Figure 4), as the highest microcystin production and highest biovolume occurred in the stratification season months.
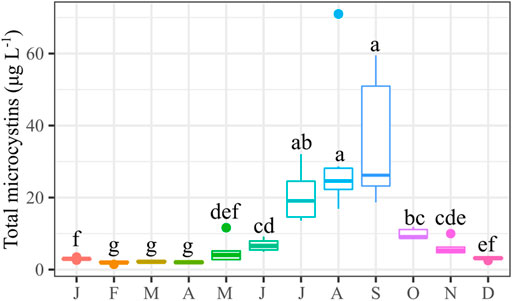
FIGURE 4. Boxplot graphs for the total microcystin concentrations (∑MC, μg L−1) recorded in six sampling points in Valle de Bravo’s reservoir during 2019. Kruskal-Wallis test and multiple comparisons; different letters indicate significant differences (p < 0.05).
The sum of MC (∑MC) was low in winter months (2–3 μg L−1) and increased significantly starting in May (5.1 ± 3.4 μg L−1) (Figure 4). The highest mean concentration (and higher variance) was observed in the summer months (20–35 μg L−1 and decreased again by October (9.8 ± 1.5 μg L−1). The highest punctual concentration was observed in August (71 μg L−1) and the minimum in February (1.5 μg L−1). The maximum observed ∑MC at 71 μg L−1 is considerably higher than existing guidelines for drinking water (0.3–1.6 μg L−1) or for recreational activities (20 μg L−1).
Phytoplanktonic diversity and biovolume
We identified in total 75 phytoplankton species in the Valle de Bravo reservoir belonging to the Divisions Chlorophyta (24), Cyanobacteria (20), Bacillariophyta (13), Charophyta (6), Miozoa (5), Cryptophyta (3), Euglenozoa (2) and Ochrophyta (2). The total biovolume consisted mainly of cyanobacteria (6,766.59 mm3 L−1) while Charophyta (18.94 mm3 L−1), Bacillariophyta (17.12 mm3 L−1), Miozoa (8.46 mm3 L−1) and Chlorophyta (2.71 mm3 L−1) had lower biovolumes. Euglenozoa, Ochrophyta and Cryptophyta were the Divisions with minor contribution to the total biovolume (Figure 5). All the identified phytoplankton species and the specific determined biovolumes are shown in Supplementary Table S2.
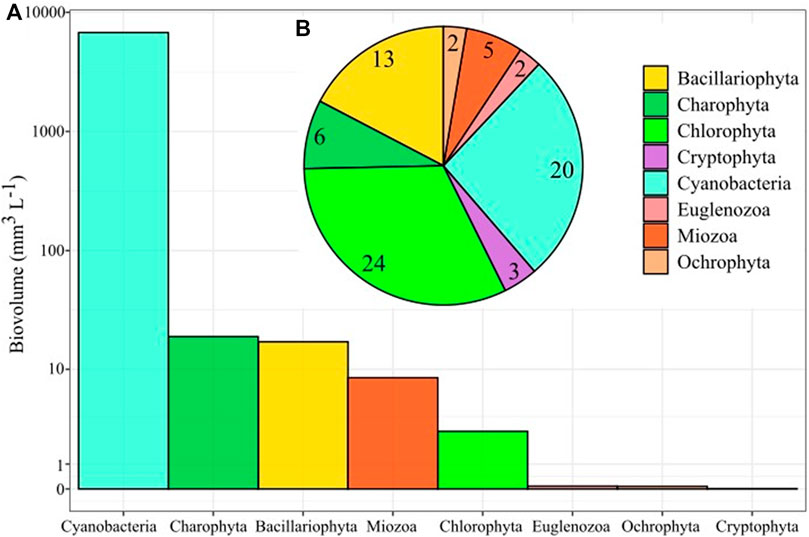
FIGURE 5. Total biovolume (A) (mm3 L−1) and specific richness (B) of the main division identified in the phytoplankton community of the Valle de Bravo reservoir during 2019.
Regarding biovolume, cyanobacteria were the most abundant taxa in the phytoplankton community during 2019. The 18 cyanobacteria species with the highest biovolumes are shown in order from the highest to the lowest total values in Figure 6. The cyanobacterium with the highest biovolume was M. smithii, being the dominant species from August to September (995.9 mm3 L−1 in August). From August to November M aeruginosa had the highest biovolumes, ranging from 299 to 341 mm3 L−1), whereas M. wesenbergii had the highest biovolumes values in January (351.3 mm3 L−1) and October (297 mm3 L−1). The presence of Microcystis was, in general, minor from January to May and in December (Figure 6). Filamentous cyanobacteria had a lower presence in the phytoplankton community, but some peaks of Dolichospermum species were observed in the middle of the year. All the species of Microcystis, including those that flourish during the mixing season, had an increase from May to October, with the highest biovolumes in August and September. Aphanocapsa planctonica had its maximum biovolume in September. We note the high contribution to biovolume of M. smithii during the thermocline. The highest biovolume of Woronichinia karelica was recorded in July (10.78 mm3 L−1). W. naegeliana displayed four peaks with the highest in February (3.67 mm3 L−1). Coccoids (Chroococcales) had higher biovolumes than filamentous cyanobacteria (Nostocales and Oscillatoriales). Nevertheless, biovolume data indicate that the Valle de Bravo dam had a circannual permanent cyanobacterial bloom that was dominated by different species with Microcystis being the dominant genus.
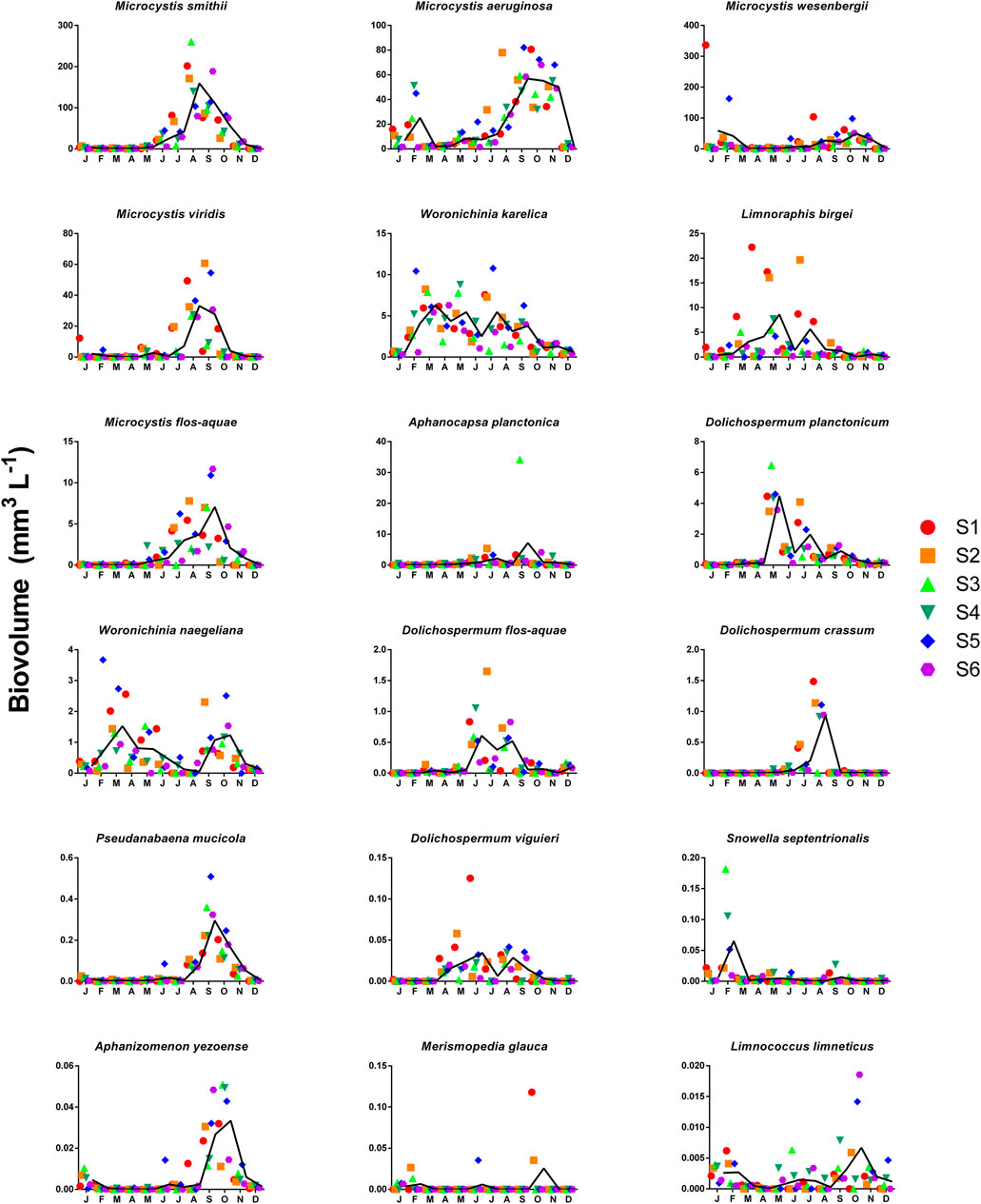
FIGURE 6. Monthly variations (year of 2019) in the cyanobacterial specific biovolume (mm3 L−1) in Valle de Bravo’s reservoir for the 18 phytoplankton species with the highest total biovolume values in the different sampling points, arranged in decreasing sequence (left to right, up to down). The continuous black lines represent the average values recorded in each month. Color legend represents the site number.
Throughout the year, the specific richness decreased reaching its lowest value in May; from July to December, it was roughly constant, coinciding with the stratification season (Figure 7A), with the lowest observed value in August (24 ± 1 species), increasing again in the mixing period, registering the maximum richness in December (41 ± 1). In contrast, the biovolume had an inverse behavior (Figure 7B increasing throughout the year with a peak in August (265 ± 37 mm3 L−1) and a minimum of 5.7 ± 1.0 mm3 L−1 in December. In all months, Except for December, the biovolume exceed the WHO alert level two (Chorus and Welker, 2021).
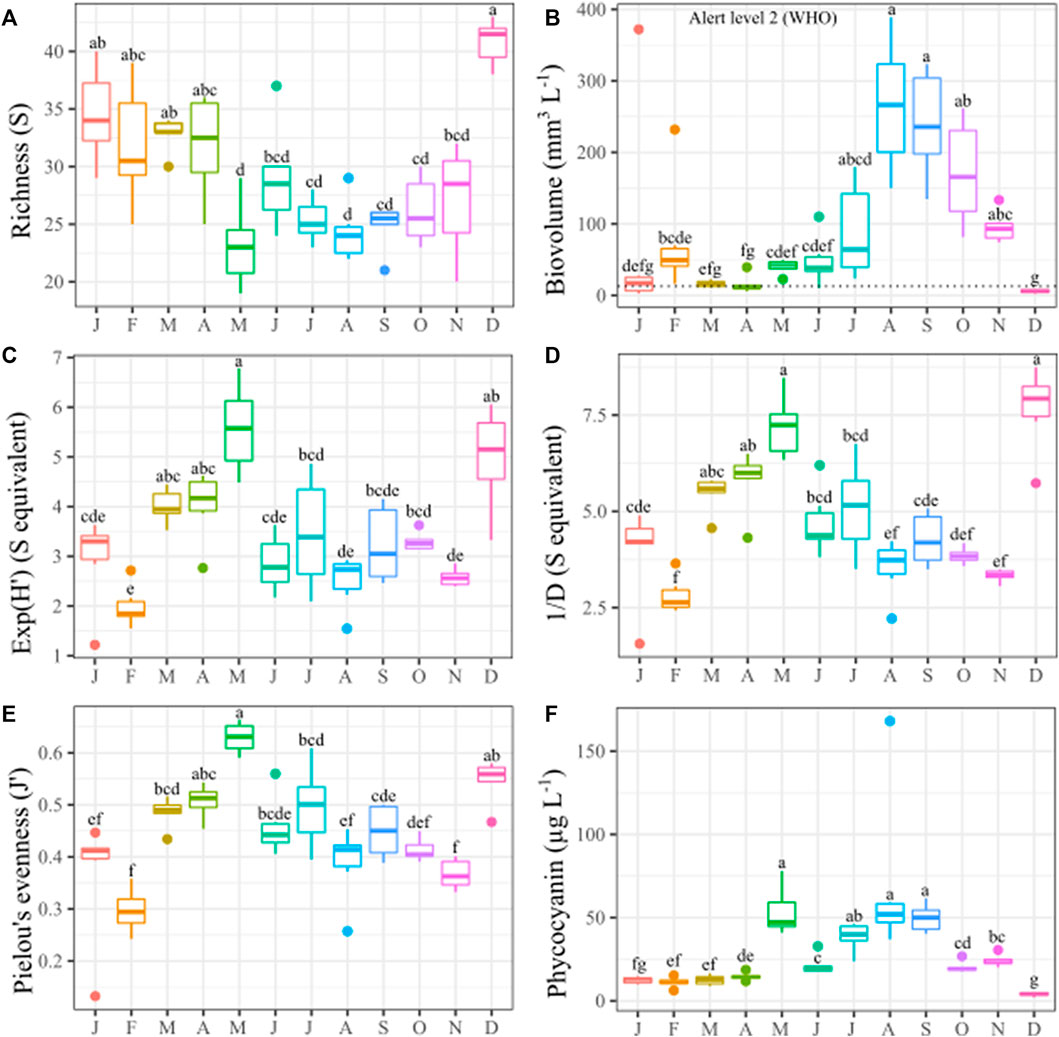
FIGURE 7. Boxplot graphs for phytoplankton diversity patterns in each month along 2019. (A) Species richness (S); (B) Biovolume (mm 3 L−1); (C) Shannon exponential index (Exp(H′)); (D) Simpson inverse (1/D); (E) Pielou’s evenness (J′); (F) Phycocyanin concentration (μg L−1). Kruskal Wallis test and multiple comparisons (p < 0.05).
The two diversity indices (Shannon’s exponential Exp(H') and Simpson’s inverse 1/D), and Pielou’s equity (J’) exhibited similar variations (Figures 7C–E), with a maximum in May (Exp(H") = 7.21 ± 0.33; 1/D = 5.57 ± 0.36; J’ = 0.63 ± 0.01), decreasing afterwards to increase again in December (D1 = 7.66 ± 0.43; D2 = 4.98 ± 0.41; J’ = 0.55 ± 0.02). The phycocyanin level (a proxy for cyanobacteria presence) was higher during most of the stratification season and lower during the mixing season; the lowest value was recorded in December (max. = 5.05 μg L−1) (Figure 7F). A mix of genus (mainly Microcystis, Woronichinia, Limnoraphis, and Dolichospermum) were responsible for these blooms with a lower richness in the stratification period.
A first canonical correspondence analysis (CCA) was performed that included biological and ecological factors (richness, biovolume, Shannon’s exponential, Simpson’s inverse, Pielou’s equity, phycocyanin, and the biovolume contributed by each of the ten most abundant species), and 20 abiotic variables (Supplementary Table S3). CCA explored the monthly behavior of biological variables with respect to abiotic factors (Figure 8); the first axis explained 43.1% and the second axis 29.6% of the total variation. From January to April, and December 2019, values of richness, equity, Shannon’s diversity, and Simpson’s inverse, were higher than in the rest of the year and were associated with factors such as water level, chlorides, nitrates, Mg, SRP, ammonium and hardness; Woronichinia naegeliana, W. karelica, Limnoraphis birgei and Dolichospermum planctonicum were the related cyanobacterial species, although at lower biovolumes than Chroococcales. From May to July, the concentration of phycocyanin was the highest and was positively related to metals such as zinc and copper. The remaining months (August to November) were influenced by variables such as precipitation and temperature and were associated with higher biovolume of Microcystis species (M. aeruginosa, M. flos-aquae, M. smithii, M. viridis and M. wesenbergii) and Aphanocapsa planctonica. Total dissolved solids, pH, dissolved organic carbon, total microcystins and dissolved oxygen increased along a big cell proliferation, and this phenomenon was also positively associated with higher concentrations of potassium iron, and aluminum.
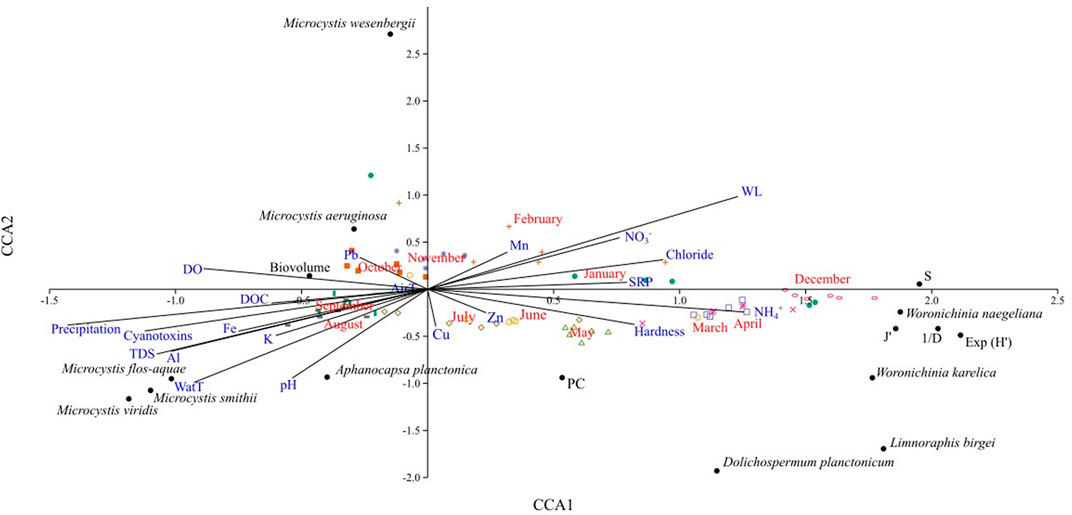
FIGURE 8. CCA triplot of diversity index, phycocyanin, and specific biovolume regarding abiotic variables and total microcystins in Valle de Bravo’s dam. Months are in red font, diversity index and phycocyanin concentrations in black font, abiotic features in blue font, and cyanobacterial species in italicized black font.
The second canonical correspondence analysis (Supplementary Table S4) explains 71.3% of the total variation (Figure 9), and total microcystins were found to be closely related to abiotic variables such as rainfall, total dissolved solids, air and water temperature, aluminum, iron, and dissolved organic carbon. The highest concentration of cyanotoxins was recorded from July to September, associated with the proliferation of potentially toxigenic species such as Microcystis (M. flos-aquae, M. smithii, M. viridis), Dolichospermum (D. cf. crassum and D. cf. flos-aquae) and Aphanocapsa planctonica. Pseudanabaena mucicola is endogloeic in Microcystis and it is also associated with the season of higher cyanotoxin production. Variables such as water level, nutrients (N, P, and manganese), dissolved oxygen, chloride and lead were inversely correlated with cyanotoxin synthesis, whose minimum concentrations were recorded in the mixing season in the reservoir—associated with species of the genera Snowella, Limnococcus, Merismopedia, Aphanizomenon and M. wesenbergii (non-toxigenic), and M. aeruginosa (potentially toxigenic). Cyanobacteria that proliferated from March to May (such as Limnoraphis, Planktothrix, Woronichinia and Dolichospermum, Supplementary Table S4) do not seem to correlate with total microcystins.
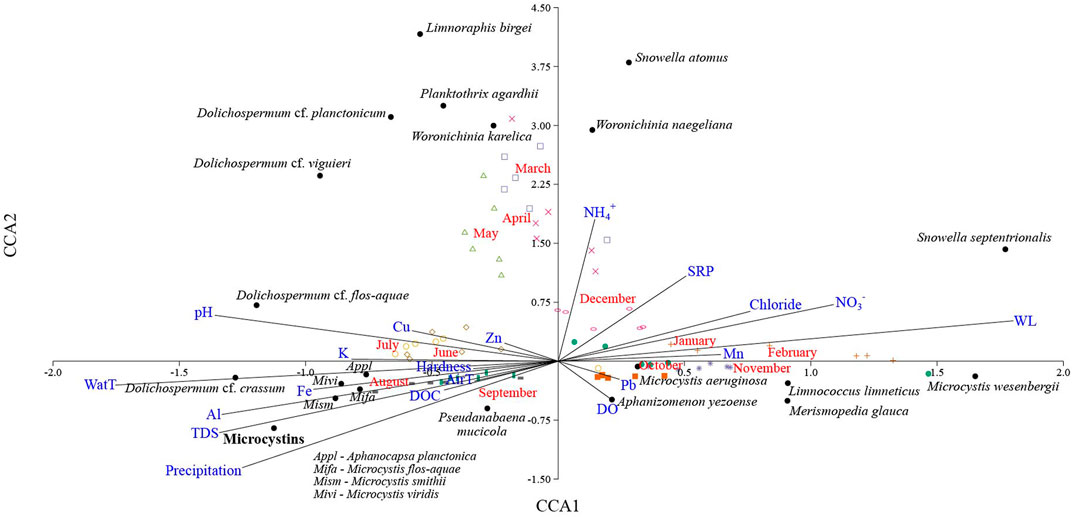
FIGURE 9. CCA triplot for total microcystins and all cyanobacterial species regarding abiotic variables in Valle de Bravo’s reservoir. Months are displayed in red font, cyanotoxins in black font, species in italicized black font, and abiotic features in blue font. The first axis explains 41.9% and the second axis 29.3% of the total variation.
Regarding the correlation analysis (Supplementary Table S5), the variables that positively influenced the specific richness inversely correlated with the biovolume and the phycocyanin concentration (water level, hardness, chloride, nitrates, ammonium and SRP). Additionally, the factors that correlate negatively with species richness are those that do so directly with the biovolume and the phycocyanin concentration (water temperature, pH, total dissolved solids, precipitation, dissolved oxygen, aluminum, iron, and total microcystins). The biovolume and the phycocyanin concentration were also positively related to potassium. Species richness was inversely related to the dissolved organic carbon, and directly related to hardness. The Shannon diversity index Exp (H’) was significantly correlated with hardness and ammonium, and inversely correlated with precipitation and dissolved oxygen. The inverse of Simpson’s Diversity was correlated to water hardness. Pielou’s evenness had an inverse correlation with nitrates and manganese, and was positively correlated to water temperature, pH, and hardness. Regarding total microcystins, this was negatively correlated with species richness but positively correlated with biovolume and phycocyanin concentration; this confirms that the synthesis and release of these toxins happens when the biovolume of cyanobacteria is high (confirmed with the high PC content) and associated with a reduced number of species.
Water quality index (PhyCOI)
The PhyCOI values, calculated as described previously, are shown in Figure 10. According to these determinations, water quality in Valle de Bravo dam fluctuated from very poor to poor in 10 months of the year and was moderate in April. December was the only month with good water quality (PhyCOI = 3.10 ± 0.10), this condition coinciding with the beginning of mixing season. The PhyCOI values were higher during the mixing season than in the stratification season, but water quality is classified has poor most of the year, except for December, when the highest PhyCOI value was determined. The lowest PhyCOI value was found in September (0.73 ± 0.02). Combining these results with the microcystins information, most of the months during the stratification season had the worst water quality and the risk to aquatic biota wellness and human health by exposure to cyanotoxins.
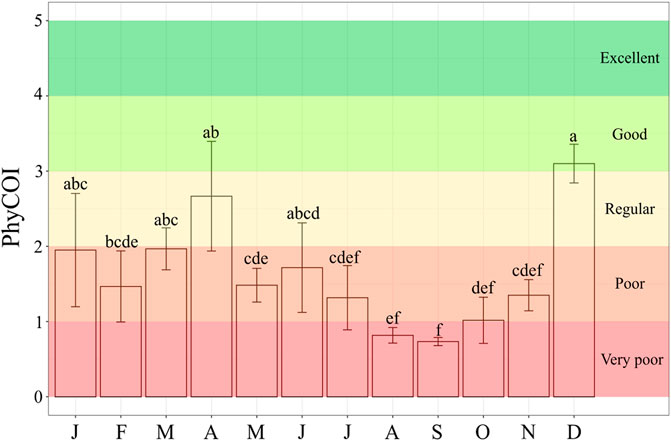
FIGURE 10. PhyCOI values and water quality classification based on the analysis of the phytoplankton community determined monthly in six sampling points during the year 2019 in Valle de Bravo dam. Average values are included with bars for standard error. Significant differences, after ANOVA and Kruskall-Wallis post hoc tests, are denoted by different letters in each column (p < 0.05).
Discussion
Phytoplankton community and the main environmental factors related to water quality in Valle de Bravo varied throughout the year, influenced mainly by climatic conditions (Figure 2). The increase in ambient temperature during the summer months causes the thermal stratification of the water column. This implies the formation of water layers differentiated by their density. The Valle de Bravo reservoir is known to behave as a warm monomictic lake with mixing in winter (Merino-Ibarra et al., 2008). Nutrient concentrations and oxide reduction potential were higher in the 1 months of the year, coinciding with the mixing period that allows nutrients trapped in the hypolimnion to be resuspended into the water column and that can contribute to the increase in the phytoplankton biomass during the stratification months (Carnero-Bravo et al., 2015). Hence, the stratification season (the warmest months) also coincides with the development of cyanobacterial blooms. The water level in the reservoir, which is controlled by the National Water Commission (CONAGUA), was lower in the stratification season because it also coincides with rainfall and therefore increases the flow of water out of the reservoir to satisfy drinking water requirements in the Cutzamala System and to prevent flooding events. The higher amount of mineral nutrients recorded in January–April and December 2019, is partly attributed to internal nutrient recovery during mixing but it is also exacerbated by the introduction of agrochemical pollutants, fertilizers, and wastewaters containing excreta and detergents contributed mainly by the Amanalco River, the local market, and land urbanization (Ramírez-Zierold et al., 2010). These conditions increased the total dissolved solids in the summer, also contributing to the reduction in the water level in the dam.
Physicochemical conditions in water varied during the study period. Dissolved oxygen was related to cyanobacterial growth owing to their oxygenic autotrophic metabolism during daylight hours (Paerl et al., 2001); however, upon senescence and death of this biomass this can generate a large amount of organic matter. This organic matter increases total organic carbon and biochemical oxygen demand through bacterial degradation, thus reducing available dissolved oxygen mainly in the bottom and during nighttime hours. Results for essential and non-essential metals, none of the trace elements included in the Mexican guideline NOM-001-SEMARNAT-1996 exceeds the maximum permissible limits for any type of water use or for the conservation of water resources (4–6 mg L−1). Wu et al. (2017) report toxic effects of copper on cyanobacteria at concentrations greater than 0.1 mg L−1; but as copper concentrations in the present study are lower, they could rather serve as essential micronutrients. Nevertheless, aluminum concentrations increased during the stratification season and were positively correlated to the total MCs concentrations in this period; this is a non-essential metal that could produce toxicity in sensitive phytoplankton species, other than cyanobacteria (Hornstrom et al., 1995; Gensemer and Playle, 1999; Jančula and Maršalek. 2011). Organic matter produced during the summer blooms or carried by rain and currents from the watershed, sinks to the hypolimnion during the stratification. After bacterial transformation, this organic matter is recovered as nutrients that move from the deeper layer to the epilimnion during mixing and promote the growth of eukaryotic phytoplankton in addition to Cyanobacteria.
Ecological indicators such as diversity and abundance in the phytoplankton community, biovolume of dominant species, and total biovolume are closely associated with trophic status in lentic water bodies. In this study, 75 phytoplanktonic species were identified, mostly belonging to the Divisions Cyanobacteria, Chlorophyta and Bacillariophyta; these are frequent phytoplanktonic groups in inland waters (Bellinger and Sigee, 2010). The number of species here identified is higher than that reported by Gaytán-Herrera et al. (2011) (68 species), but lower than the value determined by Valeriano-Riveros et al. (2014), who identified 103 species.
Gaytán-Herrera et al. (2011) reported monthly homogeneity in the phytoplankton and environmental factors in the five sampling points they studied in the Valle de Bravo dam, but they found seasonal differences along the year. Opposite to this, in our study we determined that many of the environmental and physicochemical factors vary not only varying seasonally but also varied among sampling sites in each month, more clearly observed for nutrients (N and P), Secchi disk transparency, and ORP, as well as micronutrients (Figures 2, 3). These variations were associated to differences both in species richness and biovolume among sampling sites each month. The three most diverse Divisions they determined were Chlorophyceae (30 species), Cyanobacteria (12 species) and Bacillariophyceae (10 species). They mention that the highest density occurs at the beginning (April) and end of the stratification season (October), and was mainly represented by the species Microcystis botrys, M. flos-aquae, M. wesenbergii, Mougeotia sp. and Snowella septentrionalis. In particular, the difference in the number of cyanobacteria that they reported (12) with the 20 reported here is notable; it is worth noting the case of some species of Microcystis and other genera of cyanobacteria that were not recorded in that study.
Valeriano-Riveros et al. (2014) carried out a study during 24 months in a single sampling site located near the pier and the town of Vale de Bravo (close to our sampling point five), collecting phytoplankton samples at a depth of 4 m. These authors reported a total of 34 Chlorophyceae, 26 Cyanobacteria and 13 Bacillariophyceae as the dominant Divisions, in comparison with the 24 Chlorophyceae and 20 Cyanobacteria we identified (for Bacillariophyceae we also identified 13 species). They determined that the total biomass increases in the stratification season and decreases in the mixing period. No comparison for the total taxa is possible because they did not report the complete list of species but only the dominants. Regarding the biovolume, Valeriano-Riveros et al. (2014) reported that Woronichinia naegeliana and Microcystis wesenbergii were the dominant cyanobacteria during this season. In our study M. smithi, M. aeruginosa and M. viridis were dominant during stratification months, while M. wesenbergii reached the highest values at the end of stratification and had the highest values just before the start of stratification in some sampling points (Figure 6).
Alillo-Sánchez et al. (2014) detected 18 species of cyanobacteria, and the maximum richness was recorded in the warm season, before the rains, and was represented by the orders Nostocales and Oscillatoriales, subsequently dominating species of the order Chroococcales, with M. wesenbergii the species that contributed most to the biovolume.
On the other hand, Ramírez-García and Chicalote-Castillo (2020) report that the relative abundance of species in Valle de Bravo is dominated by cyanobacteria, with Microcystis biovolume greater than 3,000 mm3 L−1 in the rainy season, relating the predominance of cyanobacteria with parameters such as temperature, pH, transparency, turbidity and concentration of phosphates and nitrates.
Predominant cyanobacterial species not only vary at the seasonal scale in Valle de Bravo reservoir, but they can also fluctuate at annual/decadal time scales—though certain species are commonly identified throughout the years. Back in 2000, the species that contributed most to phytoplankton abundance were Microcystis spp, Snowella septentrionalis, Merismopedia trolleri, and Mougeotia sp. (Gaytán-Herrera et al., 2011). In 2008 and 2009, abundant species included M. trolleri, Aphanothece sp, M. wesenbergii, M. aeruginosa, W. naegeliana, S. septentrionalis and Dactylococcopsis sp. (Valeriano-Riveros et al., 2014). A 2010 study reported the predominance of D. planctonicum, D. aff. vigueri, P. agardhii, M. wesenbergii, W. naegeliana, Pseudanabaena mucicola and Limnoraphis birgei (Alillo-Sánchez et al., 2014). Similarly, in 2017, the dominant phytoplanktonic species were P. agardhii, Aphanocapsa planctonica, Aphanizomenon yezoense, M. aeruginosa, M. wesenbergii, W. naegeliana, Pseudanabaena mucicola, and L. birgei (Nandini et al., 2019; Ramírez-García and Chicalote-Castillo 2020). Although similar species were found in the present study, the 10 cyanobacteria that contributed most to the biovolume as of 2019 (our study) were M. smithii, M. aeruginosa, M. wesenbergii, M. viridis, Woronichinia karelica, L. birgei, M. flos-aquae, Aphanocapsa planctonica, D. planctonicum, and W. naegeliana (Figure 5). Nandini et al., 2008.
Although Chlorophyceae were the phytoplankters with the highest number of species, their contribution to the phytoplankton biovolume was one of the smallest, coinciding with what was observed by Gaytán-Herrera et al. (2011), and Valeriano-Riveros et al. (2014). In general, the differences observed at different times in both species richness and dominance of species may be due to the management of water resources in this waterbody by the CONAGUA. Variations in environmental conditions also had influence, mainly related to the differences in the period and intensity of the rains and the corresponding runoffs of the main tributaries. What was constant is the predominance in biovolume of cyanobacteria, regardless of the dominant species.
The total number of species identified in Valle de Bravo during 2019 was lower in the stratification season. This can be related with the reduction of nutrients and the hydrodynamic variations in the dam during and after the stratification (Monroy, 2004). The stability of the water column during stratification contributes to the predominance of cyanobacteria, their buoyancy being favored by intracellular aerotopes that enable their ubication in the photosynthetically optimal zone in the water column. They can thus be maintained at depths suitable for photosynthesis and could interfere with the amount of light reaching other phytoplankters that do not have similar strategies (Olvera, 1996). The success of these adaptations is reflected in the biovolume produced by the different phytoplankton Divisions, with a clear predominance of cyanobacteria. The larger size of Microcystis colonies compared with microalgae cells also contribute to their predominance. Other competing characteristics that cyanobacteria have include nitrogen fixation in some species, the presence of accessory pigments (phycobiliproteins), and mechanisms for carbon accumulation. Their resistance to consumption by filter-feeding species is another advantage (Ibelings et al., 2021), due to their growth as colonies and filaments, and their low nutritional value. High nutrient levels during mixing season, contributed to the proliferation of cyanobacterial species mainly of the genera Limnoraphis, Dolichospermum, and Woronichinia, which are smaller than Microcystis colonies. Comparatively smaller species (lacking aerotopes) do not form buoyant layers, allowing other phytoplankters to proliferate thus leading to higher indexes of diversity, specific richness, and equity in the mixing season. From August to November the community was dominated by different species of the genus Microcystis, which predominately contributed to the high biovolumes. The specific richness decreased gradually towards the stratification season and increased in the mixture season, while the opposite was observed for the biovolume, mainly due to the adaptations presented by cyanobacteria, making them more competitive. The latter is confirmed by the phycocyanin records and by the seasonal dynamics of diversity and equity indices.
In the present study, specific richness was significantly correlated with parameters such as the water level, chlorine, nitrate, ammonium and SRP, while biovolume and phycocyanin concentration were inversely related (Supplemental Table S5). The water level was lower in the warm season due to CONAGUA management, but also due to the increase in the potable water demand and the evaporation rate. This can lead to decrease in the concentration of nutrients that were rapidly consumed by cyanobacteria, reducing the specific richness in the stratification season. This reduction in the water level also increased sediment resuspension in the shallower zones, generating inorganic turbidity and decreasing the amount of available light, resulting in a change in the phytoplankton community composition. Hardness positively influences specific richness and negatively influences biovolume. The high consumption of bicarbonate as the main C source during cyanobacterial proliferation makes bicarbonate ions less bioavailable to eukaryotic phytoplankters, related also with the increase in pH and in the displacement in the dissolved CO2 in water to carbonates, a non-assimilable C source for phytoplankton. This also implies that, under mixed conditions (higher hardness), there is a greater amount of carbon source as bicarbonates, which is reflected in higher richness, diversity, and equity indexes.
Despite the fact that the total biovolume was lower during the mixing season (Figure 6, Supplementary Table S2) it remained above the WHO alert level two for drinking (4 mm3 L−1) and recreational water (8 mm3 L−1) for the whole year, indicating a high potential risk to human health if the water would not be adequately treated before consumption (Chorus and Welker, 2021).
Permanent cyanobacterial blooms were observed throughout our annual monitoring study. The exponential growth of cyanobacteria during the summer bloom season caused an increase in pH due to the uptake of bicarbonate ions through photosynthesis (Ibelings et al., 2021). Chemical oxygen demand was highest in the cyanobacterial bloom season and at the end of the bloom season, with an abrupt drop in August affecting cyanobacterial growth (Kagalou et al., 2003). DOC was higher due to the decomposition by bacterioplankton of the large biomass accumulated during blooms, which is carried out with consumption of dissolved oxygen (Lignell, 1990).
In addition to the nuisance caused by cyanobacterial blooms, there are also potential health risks associated with the production of cyanotoxins. Total microcystins were associated with potentially toxic species of the genus Microcystis (M. flos-aquae, M. smithii and M. viridis). The association of different cyanobacteria can contribute to toxin synthesis. Paerl and Otten (2013) mentioned that together the genera Aphanocapsa, Dolichospermum, Microcystis and Pseudanabaena can synthesize cyanotoxins. Toxins were likely produced by species such as Aphanocapsa planctonica, Dolichospermum cf. crassum, M. flos-aquae, M. smithii, M. viridis and Pseudanabaena mucicola, which have been previously reported as toxigenic. These cyanobacteria were recorded simultaneously in the present study, and they had a strong association as suggested by the canonical correspondence analysis (Figure 9).
The concentration of total microcystins was directly correlated with variables such as hardness (sum of calcium and magnesium ions). Gu et al. (2020) found that high concentrations of calcium can contribute to the formation of cyanobacterial scums by promoting exopolysaccharide (EPS) secretion, activating antioxidant enzymes, inducing cell aggregation, and enhancing colony buoyancy. Total microcystins were strongly associated with metals such as aluminum (potentially toxic to cyanobacteria), iron (a micronutrient that can be chelated by these molecules), and with total dissolved solids, rainfall precipitation and temperature. Throughout the year, microcystin synthesis was observed to be enhanced in the warmest months (July–September) in Valle de Bravo reservoir (Figure 4). Canadian lakes with high phytoplankton biovolumes were also associated with high concentrations of MC-LR (400 μg L−1 from July to September), N, and total P in the summer season (Kotak and Zurawell, 2007). In Spanish reservoirs, Carrasco et al. (2006) reported the highest values of microcystins in September–November. Microcystin production was associated with cyanobacteria of the orders Chroococcales, Nostocales and Oscillatoriales, also with higher values in the warm months in other Mediterranean reservoirs (Mariani et al., 2015). Forastier et al. (2016) found that microcystin-LR production events occur in cyanobacterial proliferation, mainly in the month of March (end of summer) in Argentina.
The World Health Organization established in 2020 provisional guideline values (GVs) for MCs (lifetime drinking-water GV: 1 μg/L; short term drinking-water GV: 12 μg/L; recreational water GV: 24 μg/L). The high levels of microcystins in surface water during the bloom season raise concerns with respect to potential drinking water exposure and were above the recreational water guideline during the summer months (August–September). The cyanotoxin contamination of other supply reservoirs within the Sistema Cutzamala is largely unknown. In light of our findings, a comprehensive assessment of cyanotoxins in raw and treated drinking water sources serving the State of Mexico is warranted.
Water temperature, pH, total dissolved solids, precipitation, dissolved oxygen, aluminum, iron, and cyanotoxins were negatively correlated with the number of taxa encountered and directly correlated with the biovolume and phycocyanin concentration (Supplementary Table S5) so these conditions could be identified as promoters of HCBs in Valle de Bravo dam. Rainfall led to higher levels of total dissolved solids (incorporated by runoff) and allochthonous compounds (mainly agrochemicals, including chemical fertilizers) that likely contribute to sustaining the cyanobacterial bloom through the supply of limiting macronutrients.
Finally, the PhyCOI water quality index, based on the species richness and the biovolume of the main phytoplanktonic groups, indicates a very poor to poor water quality during most of the stratification season, coinciding with the dominance of some species of cyanobacteria, the highest values of cyanobacterial biovolume, and the highest concentrations of total microcystins, especially from May to October. These results must be considered for the authority in charge of the management of this reservoir to search for measures aimed to revert the trophic status, control the runoff of agrochemicals and urban wastes discharges in order to avoid risks for the use of this waterbody as a source of drinking water. Also, it is important to warn people about the risks for tourism, and for the development of recreational and sport activities. Because the phytoplankton richness and diversity can vary from year to year, a constant monitoring of the water quality and the measuring of microcystins is required for a safe use of this reservoir.
Conclusion
In summary, Cyanobacteria were the largest contributor to the biovolume, although the Chlorophyta division had the largest number of taxa. Permanent blooms were observed throughout the year; despite biomass concentrations that were not uniform in all the dam surface, high cyanobacterial biovolumes were always recorded, and mostly associated with a prevalence of potentially toxigenic cyanobacteria. The permanent eutrophic status of this drinking water reservoir has not been previously reported in this magnitude. Throughout the year, there was a predominance of species of the genus Microcystis, although filamentous cyanobacteria of the genera Limnoraphis and Dolichospermum, and colonial ones such as Woronichinia and Aphanocapsa also proliferate occasionally. In the stratification and biomass proliferation season, Microcystis grows predominately, associated with variables such as temperature (ambient and water), precipitation, total dissolved solids, dissolved oxygen, dissolved organic carbon, total microcystins and metals. Microcystins concentrations reach levels of concerns from May to November, coinciding with the stratification season. Overall, according to the PhyCOI index, water quality in Valle de Bravo fluctuates from very poor to poor, and was good only in 1 month during the mixing season (December); a clear abatement was observed from March-April to November, the months of highest cyanobacterial proliferation. The high levels of microcystins during the bloom season raise concerns as regards potential drinking water exposure and require a follow-up monitoring of treated water produced from the reservoir. The microcystin levels in the bloom season are also of concern for recreational usage of this reservoir.
Data availability statement
The original contributions presented in the study are included in the article/Supplementary Material, further inquiries can be directed to the corresponding authors.
Author contributions
PA-G; Taxonomic identification, formal analysis, data curation, investigation, manuscript preparation. MH-Z; Formal analysis, data curation, project administration, investigation. LM-J; Formal analysis, investigation. GM; Formal analysis, writing-reviewing and editing. DFS; Project administration, supervision, formal analysis, data curation, writing-reviewing and editing. SS; Conceptualization, funding acquisition, supervision, investigation, writing-reviewing and editing. FM-J; Funding acquisition, conceptualization, project administration, supervision, validation, original draft writing, writing-reviewing and editing.
Funding
We thank the Consejo Nacional de Ciencia y Tecnología for the support granted through a binational collaboration with the Fonds de Recherche du Québec (CONACYT-FRQ 279444). We also thank financial support from Genome Canada and Génome Québec trough the ATRAPP project (Algal Blooms, Treatment, Risk Assessment, Prediction and Prevention through Genomics).
Acknowledgments
We are thankful to the Secretaría de Marina, Capitanía de Puerto Valle de Bravo, for the support provided during all the collecting campaigns, including the naval personnel and the use of ships. Thanks to the Comisión Nacional del Agua (CONAGUA) for supporting with statistical information. The authors are grateful to the team of the Laboratorio de Hidrobiología Experimental for its contribution to sample collections in Valle de Bravo. We acknowledge administrative support from Alejandra Guitron (UdM) during the sampling season. The authors thank Quoc Tuc Dinh and Sung Vo Duy for their participation in the microcystins analysis (UdM), and Bureau Veritas (Canada) for the metals analysis.
Conflict of interest
The authors declare that the research was conducted in the absence of any commercial or financial relationships that could be construed as a potential conflict of interest.
Publisher’s note
All claims expressed in this article are solely those of the authors and do not necessarily represent those of their affiliated organizations, or those of the publisher, the editors and the reviewers. Any product that may be evaluated in this article, or claim that may be made by its manufacturer, is not guaranteed or endorsed by the publisher.
Supplementary material
The Supplementary Material for this article can be found online at: https://www.frontiersin.org/articles/10.3389/fenvs.2022.984365/full#supplementary-material
References
Alillo-Sánchez, J. L., Gaytán-Herrera, M. L., Martínez-Almeida, V. M., and Ramírez-García, P. (2014). Microcystin-LR equivalents and their correlation with Anabaena Spp. in the main reservoir of a hydraulic system of Central Mexico. Inland Waters 4 (3), 327–336. doi:10.5268/iw-4.3.573
American Public Health Association (Apha) (1999). “Phytoplankton counting techniques,” in Standard Methods for the examination of water and wastewater. Editors L. S. Clesceri, A. E. Greenberg, and A. D. Eaton. 20th edition (Washington D.C: Water Environment Federation).
Banderas, A. G., and González-Villela, R. (2019). “Evaluation of the sustainability of the Valle de Bravo reservoir, Mexico, as a water-supply source,” in WIT transactions on ecology and the environment. Editors S. Mambretti, and J. L. Mi Garcia (UK: WIT press Southampton), 239, 79–93.
Bartram, J., Carmichael, W. W., Chorus, I., Jones, G., and Skulberg, O. M. (1999). “Chapter 1. Introduction,” in Toxic cyanobacteria in water: A guide to their public health consequences, monitoring and management. Editors I. Chorus, and J. Bartram (London: WHO), 400.
Bellinger, E. G., and Sigee, D. C. (2010). Freshwater algae, identification and use as bioindicators. Oxford: Wiley-Blackwell, 271.
Buratti, F. M., Manganelli, M., Vichi, S., Stefanelli, M., Scardala, S., Testai, E., et al. (2017). Cyanotoxins: Producing organisms, occurrence, toxicity, mechanism of action and human health toxicological risk evaluation. Arch. Toxicol. 91 (3), 1049–1130. doi:10.1007/s00204-016-1913-6
Carnero-Bravo, V., Merino-Ibarra, M., Ruiz-Fernández, A. C., Sanchez-Cabeza, J. A., and Ghale, B. (2015). Sedimentary record of water column trophic conditions and sediment carbon fluxes in a tropical water reservoir (Valle de Bravo, Mexico). Environ. Sci. Pollut. Res. 22, 4680–4694. doi:10.1007/s11356-014-3703-0
Carrasco, D., Moreno, E., Sanchis, D., Wörmer, L., Paniagua, T., Del Cueto, A., et al. (2006). Cyanobacterial abundance and microcystin occurrence in mediterranean water reservoirs in Central Spain: Microcystins in the madrid area. Eur. J. Phycol. 41 (3), 281–291. doi:10.1080/09670260600801724
Chorus, I., and Welker, M. (2021). in Toxic cyanobacteria in water: A guide to their public health consequences, monitoring and management. Editors I. Chorus, and M. Welker. 2nd Edition (Boca Raton, Fla, USAGeneva, CH: WHO, CRC Press on behalf of the World Health Organization).
de Mendiburu, F., and Yaseen, M. (2020). Agricolae: Statistical procedures for agricultural research. R package version 1.4.0. Available at: https://myaseen208.github.io/agricolae/https://cran.r-project.org/package=agricolae.
Dreher, T. W., Collart, L. P., Mueller, R. S., Halsey, K. H., Bildfell, R. J., Schreder, P., et al. (2019). Anabaena/Dolichospermum as the source of lethal microcystin levels responsible for a large cattle toxicosis event. Toxicon. X, 100003. doi:10.1016/j.toxcx.2018.100003
Fernández, C., Estrada, V., and Parodi, E. R. (2015). Factors triggering cyanobacteria dominance and succession during blooms in a hypereutrophic drinking water supply reservoir. Water Air Soil Pollut. 226 (73), 1. doi:10.1007/s11270-014-2290-5
Forastier, M. E., Zalocar, Y., Andrinolo, D., and Domitrovic, H. G. (2016). Presencia y toxicidad de Microcystis aeruginosa (Cianobacteria) en el río Paraná, aguas abajo de la represa Yacyretá (Argentina). Rev. Biol. Trop. Int. J. Trop. 64 (1), 203–211. doi:10.15517/rbt.v64i1.8993
Gaytán-Herrera, M. L., Martinez-Almeida, V., Oliva-Martinez, M. G., Duran-Diaz, A., and Ramirez-Garcia, P. (2011). Temporal variation of phytoplankton from the tropical reservoir Valle de Bravo, Mexico. J. Environ. Biol. 32 (1), 117 http://www.jeb.co.in/journal_issues/201101_jan11/paper_21.pdf.
Gensemer, R. W., and Playle, R. C. (1999). The bioavailability and toxicity of aluminum in aquatic environments. Crit. Rev. Environ. Sci. Technol. 29 (4), 315–450. doi:10.1080/10643389991259245
Gu, P., Li, Q., Zhang, W., Zheng, Z., and Luo, X. (2020). Effects of different metal ions (Ca, Cu, Pb, Cd) on formation of cyanobacterial blooms. Ecotoxicol. Environ. Saf. 189, 109976. doi:10.1016/j.ecoenv.2019.109976
Hammer, Ø., Harper, D. A. T., and Ryan, P. D. (2001). Past: Paleontological Statistics software package for education and data analysis. Palaeontol. Electron. 4 (1), 9 https://palaeo-electronica.org/2001_1/past/past.pdf.
Hillebrand, H., Dürselen, C. D., Kirschtel, D., Pollingher, U., and Zohary, T. (1999). Biovolume calculation for pelagic and benthic microalgae. J. Phycol. 35, 403–424. doi:10.1046/j.1529-8817.1999.3520403.x
Hornstrom, E., Harbom, A., Edberg, F., and Andrén, C. (1995). The influence of pH on aluminium toxicity in the phytoplankton species Monoraphidium dybowskii and M. griffithii. Water Air Soil Pollut. 85, 817–822. doi:10.1007/bf00476930
Huisman, J., Codd, G. A., Paerl, H. W., Ibelings, B. W., Verspagen, J. M., and Visser, P. M. (2018). Cyanobacterial blooms. Nat. Rev. Microbiol. 16 (8), 471–483. doi:10.1038/s41579-018-0040-1
Ibelings, B. W., Kurmayer, R., Azevedo, S. M. F. O., Wood, S. A., and Chorus, I. Y. W. M. (2021). Understanding the occurence of cyanobacteria and cyanotoxins.” in Toxic cyanobacteria in water, A guide to their public health consequences, monitoring and management. I. V. W. M. Chorus Editor.Oxon: CRC Pres. 213.
CONAGUA and World Bank Group (2015). “Cutzamala. Diagnóstico Integral. Diagnostico para el manejo integral de las subcuencas Tuxpan, El Bosque,” in Victoria pertenecientes al Sistema Cutzamala. Editors O. Ixtapan del, B. Valle de, and V. Colorines-Chilesdo (Washington, D. C., USA, 200.
Jančula, D., and Maršalek, B. (2011). Critical review of actually available chemical compounds for prevention and management of cyanobacterial blooms. Chemosphere 85, 1415–1422. doi:10.1016/j.chemosphere.2011.08.036
Kagalou, I., and Petridis, D., (2003). Seasonal variation of water quality parameters and plankton in a shallow Greek lake. J. Freshw. Ecol. 18 (2), 199–206. doi:10.1080/02705060.2003.9664485
Katsiapi, M., Moustaka-Gouni, M., and Sommer, U. (2016). Assessing ecological water quality of freshwaters: PhyCoI- a new phytoplankton community index. Ecol. Inf. 31, 22–29. doi:10.1016/j.ecoinf.2015.11.004
Komárek, J., and Anagnostidis, K. (1999). “Cyanoprokaryota 1. Teil: Chroococcales,” in Süsswasserflora von mitteleuropa. EttlH. GärtnerG. Heynig. Editor D. Mollenhauer (Jena: Gustav Fischer), 548.
Komárek, J., and Anagnostidis, K. (2005). “Cyanoprokaryota 2. Teil: Oscillatorial,” in Süsswasserflora von mitteleuropa. BüdelB. KrienitzL. Gärtner. Editor M. Schagerl (Jena: Elsevier), 759.
Komárek, J., and Fott, B. (1983). “Chlorophyceae (grünalgen) ordung: Chlorococcales 7. Teil, 1. Hälfte,” in Das Phytoplankton des Süsswassers. Editor G. Huber-Pestalozzi Stuttgard: Schweizerbartische verlagsbuchhandlung.
Komárek, J., and Komárková, J. (2006). Diversity of aphanizomenon-like cyanobacteria. Czech Phycol. Olomouc 6, 1 https://fottea.czechphycology.cz/pdfs/fot/2006/01/01.pdf.
Komárek, J., and Zapomelová, E. (2008). Planktic morphospecies of the cyanobacterial genus anabaena = subg. Dolichospermum - 2. Part: Straight types. Fottea 8 (1), 1–14. doi:10.5507/fot.2008.001
Komárek, J., and Zapomelova, E. (2007). Planktic morphospecies of the cyanobacterial genus anabaena = subg. Dolichospermum – 1. Part: Coiled types. Fottea 7 (1), 1–31. doi:10.5507/fot.2007.001
Komárek, J., Zapomélova, E., Smarda, J., Kopecký, J., Rejmánková, E., Woodhouse, J., et al. (2013). Polyphasic evaluation of Limnoraphis robusta, awater-bloom forming cyanobacterium from Lake Atitlan, Guatemala, with a description of Limnoraphis gen. nov. Fottea 13, 39–52. doi:10.5507/fot.2013.004
Kotak, B. G., and Zurawell, R. W. (2007). Cyanobacterial toxins in Canadian freshwaters: A review. Lake Reserv. Manag. 23, 109–122. doi:10.1080/07438140709353915
Krammer, K., and Lange-Bertalot, H. (1988). “Bacillariophyceae 2. Teil: Bacillariaceae, epithemiaceae and surirellaceae,” in Süsswasserflora von mitteleuropa. EttlH. GerloffJ. Editors H. Heynig, and D. Mollenhauer (Jena: Gustav Fischer Verlag), 306.
Le Moal, M., Gascuel-Odoux, C., Ménesguen, A., Souchon, Y., Étrillard, C., Levain, A., et al. (2019). Eutrophication: A new wine in an old bottle? Sci. Total Environ. 651, 1–11. doi:10.1016/j.scitotenv.2018.09.139
Lee, T. A., Rollwagen-Bollens, G., and Bollens, S. M. (2015). The influence of water quality variables on cyanobacterial blooms and phytoplankton community composition in a shallow temperate lake. Environ. Monit. Assess. 187 (6), 315–319. doi:10.1007/s10661-015-4550-2
Lignell, R. (1990). Excretion of organic carbon by phytoplankton: Its relation to algal biomass, primary productivity, and bacterial secondary productivity in the baltic sea. Mar. Ecol. Prog. Ser. 68, 85–99. doi:10.3354/meps068085
Makowski, D., Ben-Shachar, M. S., Patil, I., and Lüdecke, D. (2019). Methods and algorithms for correlation analysis in R. J. Open Source Softw. 5 (51), 2306. doi:10.21105/joss.02306
Mariani, M. A., Padedda, B. M., Kaštovský, J., Buscarinu, P., Sechi, N., Virdis, T., et al. (2015). Effects of trophic status on microcystin production and the dominance of cyanobacteria in the phytoplankton assemblage of Mediterranean reservoirs. Sci. Rep. 5 (17964), 17964–18016. doi:10.1038/srep17964
Merino-Ibarra, M., Monroy-Rios, E., Vilaclara, G., Castle, F. S., Gallegos, M. E., and Ramírez-Zierold, J. (2008). Physical and chemical limnology of a wind-swept tropical highland reservoir. Aquat. Ecol. 42, 335–345. doi:10.1007/s10452-007-9111-5
Ministry of the Environment and Natural Resources (Semarnat) (1996). NOM-001-SEMARNAT-1996. Establishing maximum permissible limits Pollut. discharges wastewater into Natl. waters goods. Mexico: SEMARNAT, 6.
Moestrup, O., and Calado, A. J. (2018). “Dinophyceae,” in Süsswasserflora von mitteleuropa. BüdelB. GärtnerG. Editors L. Krienitz, and M. Schagerl (Berlin: Springer Spektrum), 564.
Monroy, R., E. (2004). Hydrology of the Valle de Bravo reservoir, Mexico. Master's thesis. Mexico City: UNAM, 102.
Mundial Banco and Conagua (2015). Cutzamala: Diagnóstico integral. Washington: World Bank Group, 200.
Munoz, G., Duy, S. V., Roy-Lachapelle, A., Husk, B., and Sauvé, S. (2017). Analysis of individual and total microcystins in surface water by on-line preconcentration and desalting coupled to liquid chromatography tandem mass spectrometry. J. Chromatogr. A 1516, 9–20. doi:10.1016/j.chroma.2017.07.096
Nandini, S., Merino-Ibarra, M., and Sarma, S. S. S. (2008). Seasonal changes in the zooplankton abundances of the reservoir valle de bravo (State of Mexico, Mexico). Lake Reserv. Manag. 24, 321–330. doi:10.1080/07438140809354842
Nandini, S., Sánchez-Zamora, C., and Sarma, S. S. S. (2019). Toxicity of cyanobacterial blooms from the reservoir Valle de Bravo (Mexico): A case study on the rotifer Brachionus calyciflorus. Sci. Total Environ. 688, 1348–1358. doi:10.1016/j.scitotenv.2019.06.297
National Institute of Statistics and Geography (INEGI) (2020). Censuses and population and housing counts. INEGI. [Online] Available at: https://www.inegi.org.mx/programas/ccpv/2020/ (Accessed June 17, 2021).
National Water Information System (SINA) (2020). Monitoring of Mexico's main dams. CONAGUA. [Online] Available at: http://sina.conagua.gob.mx/sina/almacenamientoPresas.php (Accessed August 7, 2020).
National Weather Service (SMN) (2020). Monthly summaries of temperatures and rainfall. CONAGUA. [Online] Available at: https://smn.conagua.gob.mx/es/climatologia/temperaturas-y-lluvias/resumenes-mensuales-de-temperaturas-y-lluvias (Accessed August 5, 2020).
Nygaard, G. (1949). Hydrobiological studies on some Danish ponds and lakes, II: The quotient hypothesis and some little known or new phytoplankton organisms. Det. K. Dan. Vidensk. Selsk. Kobenhavn., 301.
Oksanen, J., Guillaume, B., F., Friendly, M., Kindt, R., Legendre, P., McGlinn, D., et al. (2020). Vegan: Community ecology package. R. package version 2.5-7. URLAvailable at: https://CRAN.R-project.org/package=vegan.
Olvera, V. V. (1992). Eutrophication study of the Valle de Bravo dam, Mexico. Hydraulic Eng. Mexico 148, 161.
Olvera-Viascán, V., Bravo-Inclán, L., and Sánchez-Chávez, J. (1998). Aquatic ecology and management assessment in Valle de Bravo reservoir and its watershed. Aquatic Ecosyst. Health Manag. 1, 277–290. doi:10.1016/s1463-4988(98)00031-1
Paerl, H., and Otten, T. G. (2013). Harmful cyanobacterial blooms: Causes, consequences, and controls. Microb. Ecol. 65, 995–1010. doi:10.1007/s00248-012-0159-y
Paerl, H. W., Fulton, R. S., Moisander, P. H., and Dyble, J. (2001). Harmful freshwater algal blooms, with an emphasis on cyanobacteria. Sci. World J. 1, 76–113. doi:10.1100/tsw.2001.16
Phillips, G., Lyche-Solheim, A., Skjelbred, B., Mischke, U., Drakare, S., Free, G., et al. (2013). A phytoplankton trophic index to assess the status of lakes for the Water Framework Directive. Hydrobiologia 704, 75–95. doi:10.1007/s10750-012-1390-8
Pineda-Mendoza, R., Olvera-Ramírez, R., and Martínez-Jerónimo, F. (2012). Microcystins produced by filamentous cyanobacteria in urban lakes. A case study in Mexico City. Hidrobiologica 22 (3), 290 https://hidrobiologica.izt.uam.mx/index.php/revHidro/article/view/730.
Pineda-Mendoza, R., Zúñiga, G., and Martínez-Jerónimo, F. (2016). Microcystin production in Microcystis aeruginosa: Effect of type of strain, environmental factors, nutrient concentrations, and N:P ratio on mcyA gene expression. Aquat. Ecol. 50, 103–119. doi:10.1007/s10452-015-9559-7
Pradilla, C., E. (2016). Metropolitan area of the Valley of Mexico: Neoliberalism and urban contradictions. Sociology 18 (42), 54. doi:10.1590/15174522-018004203
R Core Team (2021). R: A language and environment for statistical computing. Vienna: R Foundation for Statistical Computing. https://www.R-project.org/ URL.
Ramírez-García, P., and Chicalote-Castillo, D. (2020). Changes in cyanobacterial density due to application of artificial floating island model with macrophytes: An experimental case study in a tropical reservoir. J. Limnol. 80 (1), 1959. doi:10.4081/jlimnol.2020.1959
Ramirez-Zierold, J. A., Merino-Ibarra, M., Monroy-Ríos, E., Olson, M., Castillo, F. S., Gallegos, M. E., et al. (2010). Changing water, phosphorus and nitrogen budgets for Valle de Bravo reservoir, water supply for Mexico City Metropolitan Area. Lake Reserv. Manag. 26, 23–34. doi:10.1080/07438140903539790
Smith, D. R., King, K. W., and Williams, M. R. (2015). What is causing the harmful algal blooms in Lake Erie? J. soil water conservation 70 (2), 27–29. doi:10.2489/jswc.70.2.27a
Steffen, M. M., Davis, T. W., McKay, R. M. L., Bullerjahn, G. S., Krausfeldt, L. E., Stough, J. M., et al. (2017). Ecophysiological examination of the Lake Erie Microcystis bloom in 2014: Linkages between biology and the water supply shutdown of Toledo, OH. Environ. Sci. Technol. 51 (12), 6745–6755. doi:10.1021/acs.est.7b00856
Svirčev, Z., Lalić, D., Bojadžija Savić, G., Tokodi, N., Drobac Backovic, D., Chen, L., et al. (2019). Global geographical and historical overview of cyanotoxin distribution and cyanobacterial poisonings. Arch. Toxicol. 93, 2429–2481. doi:10.1007/s00204-019-02524-4
Taylor, J. C., Harding, W. R., and Archibald, C. G. M. (2007). An illustrated guide to some common diatom species from South Africa. Pretoria: Water Research Commission, 225.
Valeriano-Riveros, M. E., Vilaclara, G., Castillo-Sandoval, F. S., and Merino-Ibarra, M. (2014). Phytoplankton composition changes during water level fluctuations in a high-altitude, tropical reservoir. Inland Waters 4, 337–348. doi:10.5268/iw-4.3.598
Wood, R. (2016). Acute animal and human poisonings from cyanotoxin exposure—a review of the literature. Environ. Int. 91, 276–282. doi:10.1016/j.envint.2016.02.026
Wu, H., Wei, G., Tan, X., li, L., and Li, M. (2017). Species-dependent variation in sensitivity of Microcystis species to copper sulfate: Implication in algal toxicity of copper and controls of blooms. Sci. Rep. 7, 40393. doi:10.1038/srep40393
Keywords: eutrophication, tropical dams, harmful cyanobacterial blooms (HCBs), cyanotoxins, phytoplanktonic community index (PhyCOI), Microcystis, water quality
Citation: Martínez-Jerónimo F, Antuna-González PdC, Hernández-Zamora M, Martínez-Jerónimo L, Munoz G, Simon DF and Sauvé S (2022) Year-long monitoring of phytoplankton community, toxigenic cyanobacteria, and total microcystins in a eutrophic tropical dam supplying the Mexico megacity. Front. Environ. Sci. 10:984365. doi: 10.3389/fenvs.2022.984365
Received: 01 July 2022; Accepted: 06 October 2022;
Published: 20 October 2022.
Edited by:
Carmen Rubio Armendáriz, Universidad de La Laguna, SpainReviewed by:
Xana Álvarez, University of Vigo, SpainEkaterina Chernova, St. Petersburg Federal Research Center of the Russian Academy of Sciences, Russia
Copyright © 2022 Martínez-Jerónimo, Antuna-González, Hernández-Zamora, Martínez-Jerónimo, Munoz, Simon and Sauvé. This is an open-access article distributed under the terms of the Creative Commons Attribution License (CC BY). The use, distribution or reproduction in other forums is permitted, provided the original author(s) and the copyright owner(s) are credited and that the original publication in this journal is cited, in accordance with accepted academic practice. No use, distribution or reproduction is permitted which does not comply with these terms.
*Correspondence: Fernando Martínez-Jerónimo, Zmplcm9uaUBpcG4ubXg=; Sébastien Sauvé, c2ViYXN0aWVuLnNhdXZlQHVtb250cmVhbC5jYQ==
†These authors have contributed equally to this work