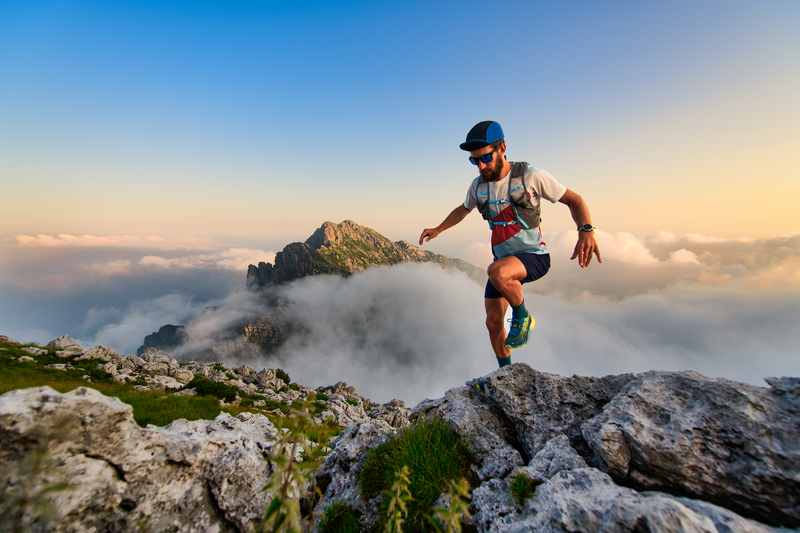
95% of researchers rate our articles as excellent or good
Learn more about the work of our research integrity team to safeguard the quality of each article we publish.
Find out more
ORIGINAL RESEARCH article
Front. Environ. Sci. , 25 October 2022
Sec. Soil Processes
Volume 10 - 2022 | https://doi.org/10.3389/fenvs.2022.979234
This article is part of the Research Topic Phosphorus Along the Soil-Freshwater-Ocean Continuum, volume II View all 7 articles
Integrated ecological farming combines rice growing with aquaculture, and is an effective way to improve soil productivity by increasing soil nutrient supply. However, the long-term effects of such integrated farming on phosphorus fractions and phosphorus availability of paddy soils in the Pearl River Delta (PRD) remain unknown. A four-year field experiment compared the phosphorus fractions with paddy field in rice-fish-duck system (RFD), rice-vegetable cropping system (RVS) and conventional rice system (CRS) in the PRD. SOC and phosphorus fractions were significantly influenced by cropping systems. RFD significantly increased SOC and phosphorus in the soil. Soil phosphorus was dominated by moderately labile P (40.67–49.41%). RFD also significantly increased soil microbial biomass carbon, microbial biomass phosphorus, and acid phosphatase activity (ACP) by 67.68, 46.68, and 15.87% compared to RVS, and by 134.14, 65.99, and 30.20% compared to CRS, respectively. SOC and ACP were the primary factors influencing the conversion and effectiveness of soil phosphorus. The RFD can alleviate low phosphorus activity in PRD paddy soils through the combined effect of chemical and biological process, while promoting a sustainable soil nutrient cycle within the ecosystem and guiding the sustainable development of rational soil fertilization in the PRD.
Phosphorus (P) is the second most limiting element among soil nutrients globally and is essential for the synthesis of plant tissues and energy metabolites (Hou et al., 2020; Divjot et al., 2021). Phosphorus also regulates the net primary productivity of soil ecosystems, as well as the decomposition and storage of soil carbon pools (Bronson et al., 2004; Shi et al., 2016), thereby playing an important overall role in maintaining the efficient functioning of soil ecosystems (Fisk et al., 2015). Moreover, adequate P supply can mitigate the negative effects of abiotic factors on plants, while promoting plant water and nutrient uptake and utilization, and improving the resilience of the plant body (Divjot et al., 2021; Cui et al., 2022). Plant P is primarily derived from the soil; however, in subtropical regions, the abundance of metal oxides and clay minerals in soils, as well as processes such as precipitation reactions and specific adsorption, all increase P fixation capacity while decreasing soil P bioavailability (Fan et al., 2019; Fu et al., 2021). Indeed, it has been shown that P fertilization can lead to an annual increase in soil P content at a rate of 11%·yr−1 (Macdonald et al., 2011), yet the seasonal bioavailability of P fertilizer is < 20% (Sun et al., 2007), while the fixed P via soil sorption becomes a potential risk of agricultural, non-point source pollution (Sharma et al., 2017).
Soil P exists in complex organic and inorganic forms, with significant differences in bioavailability. Moreover, different forms can be transformed, and together determine soil P availability (Teng et al., 2018). The forms of soil P are influenced by abiotic factors, such as soil composition, organic carbon, metal oxides, and biotic factors, such as phosphatase and soil microbial activity. The functional groups of organic carbon can combine with soil minerals to form organic complexes via ionic chemical bonds (Cavalcante et al., 2018; Fu et al., 2021), which are capable of reducing P loss due to runoff. Soil microorganisms can absorb some of available P to sustain their biological activities. Following death and decay of microorganisms, the sequestered P is returned to the soil and becomes an important source of P that can be absorbed for use by plants (Sokol et al., 2022). Additionally, phosphatases play an important role in mineralization of organic P and promotes the production of soil available P (Ning et al., 2021). The arable land in the Pearl River Delta (PRD) covers 602,500 ha (Guangdong Bureau of Statistics, 2018). However, water erosion in the PRD has increased severely over recent decades (Lai et al., 2015), resulting in the loss of large amounts of available P with surface runoff (Ortiz-Reyes and Anex, 2018). Moreover, the PRD soils are in a state of N-P imbalance due to high levels of atmospheric nitrogen deposition. This result increased the difficulty of P uptake by plant, and drove the soil ecosystem to P limitation (Vitousek et al., 2010; Chen et al., 2018). Therefore, it is important to study the transformation and control of soil P fractions to improve the efficiency of soil P utilization and promote the cyclic transformation of soil P in the PRD.
Integrated paddy ecological farming is a practice of organic ecological agriculture that integrates rice growth and aquaculture into the same field (Zheng et al., 2017). This cropping system has a long history and is growing particularly rapidly in China, and as of 2012, the use of it had spread to 2.23 Mha in China (Fishery Bureau of China’s Ministry of Agriculture, 2013). Various systems such as rice-fish, rice-shrimp, and rice-duck have been developed and applied in different regions based on the climate and production characteristics (Hu et al., 2016; Liu et al., 2019; Sun et al., 2021). Integrated ecological farming effectively promoted animal-plant-soil nutrient cycling (Wan et al., 2019; Lv et al., 2020). Rice-fish farming increases soil nutrients and is capable of bolstering rice production (Wan et al., 2019; Guo et al., 2020). Rice-fish and Rice-turtle systems influence the microbial community structure in soil and overlaying water (Afri et al., 2021; Wu et al., 2021). To date, fewer studies have focused on the effects of the rice-fish-duck system on soil P fractions and P availability. Previous studies have reported diverse results concerning the effects of different integrated ecological farming systems. During rice growing season, introducing frogs and fish could increase soil total P and available P (Lin and Wu, 2020). However, Yi et al. (2019) reported rice-fish farming system was not conducive to improve soil P availability. Therefore, whether rice-fish-duck farming changes P fractions and P availability of paddy soils in the PRD during long-term experimental studies. It is essential to understand how integrated ecological farming influence the soil P availability and its various fractions, which provides a scientific basis for alleviating the P limitation of rice soils in the PRD and for the scientific use of soil P resources.
In this study, rice-fish-duck system, rice-vegetation cropping system (rice-pepper), and conventional rice system were selected as the research objects for analyzing the relationships between soil P fractions, as well as basic physical and chemical properties, microbial biomass, and ACP activity of the soil. We hypothesized that 1) exogenous organic matter brought by rice-fish-duck farming can increase soil P content and improve P availability; and 2) difference in soil physiochemical properties of cropping systems and the effect of soil ACP activity may co-regulate the soil characteristics.
The study area was located in Zengcheng District, Guangzhou City, China (23°17'25.6'' N, 113°43'3.3'' E), which maintains a subtropical maritime monsoon climate, with an average annual temperature of 22.1°C, and an annual rainfall of 1746.7 mm (Supplementary Figure S1). Here, the soils are mainly Hydragric Anthrosols (FAO-UNESCO, 1974) developed from granite sediments, and they have a clay loam texture.
The test plots were all conventional rice farming soil in local area. The field experiment commenced in June 2017 under three designed cropping systems: rice-fish-duck system (RFD), rice-vegetable cropping system (RVS), and conventional rice system (CRS). Each type of cropping system was tested in a standard plot of 667 m2 with three replicates. The RFD abided by the rule of “wide rows and narrow plants”, and the row spacing for growing rice was about 30 cm in length × 18 cm in width. In the RPD, 7 days after seedling planting, about 10 cm-long fish (Cyprinus carpio var. color) were introduced into paddy field. After 20 days of growing time, 20-day-old ducklings (Tadorna) were introduced into paddy field. These paddy fields surrounded with high net (about 50 cm in high) and a shed for the ducks was also built in the corner of each field. Before fish and duck harvesting, the fields maintained a standing water depth of about 5 cm. Following rice tillering, fish and ducks were harvested, the field water was drained, and then the soil was allowed to dry for a week. Pepper (C. annuum var. grossum.) was a traditional and seasonal crop in the local area, and was used as a crop in the vegetable planting stage of the RVS. The RVS was a pepper-rice system, in which three crops of pepper were planted in the first half of the year, after which the land was prepared for subsequent rice planting in a manner consistent with the field management of conventional cropping systems, where a local, long-term, double-season rice crop in succession was grown. The CRS abided by the principle of local rice planting, meanwhile, keeping consistent field management. The local field management measures are conventional fertilizer application without rice straw returned to the field after harvesting, however rice stubble was treated and left in the field.
The tested rice variety of three cropping systems was all “Zengke Xinxuan Simiao rice”, which was bred by the Guangzhou Zengcheng District Agricultural Science Research Institute (https://www.ricedata.cn/variety/varis/624863.htm?624,863). In the RFD, 150 kg hm−2 (1 hm2 = 10,000 m2) of compound fertilizer (N:P2O5:K2O = 17:6:12) was applied before seedlings planting, and no further fertilizer was applied during subsequent rice growth. At the beginning of the rice-vegetable rotation and conventional rice growing, 300 kg hm−2 of compound fertilizer was applied as base fertilizer, and 150 kg hm−2 of urea was applied thereafter. The compound fertilizer and urea were prepared and supplied by Guangdong Fuli Long Compound Fertilizer Co.
We collected soil samples from a four-year field experiment in three cropping systems in October 2021. Following rice harvesting, topsoil (0–10 cm) and subsoil (10–20 cm) were collected from each field via 5-point sampling method, and the collected 5-point samples were evenly mixed into a single representative sample. Soil samples were divided into two subsamples by removing gravel and plant residue (e.g., roots), where one was naturally dried in the shade and ground through 2 mm and 0.25 mm sieves for determining its basic physical and chemical properties, and freshly ground through a 2 mm sieve and stored in a refrigerator at -4°C for the determination of microbial biomass carbon, microbial biomass P, and ACP content. The selected soil physicochemical properties are shown in Table 1.
TABLE 1. Selected soil physicochemical properties of three cropping systems at different soil depths.
Basic physical and chemical soil properties were determined by referring to the Laboratory Analysis Methods for Soil Investigation (Zhang and Gong, 2012), where pH was determined using the potentiometric method (water:soil ratio = 2.5:1); soil bulk density was determined using the ring knife method; SOC was determined using concentrated sulfuric acid-potassium dichromate external heating; soil organic nitrogen (STN) was determined by the Kjedahl acid-digestion method; soil total potassium (STK) was detected by a digestion procedure with HNO3-HF-HClO4; dissolved organic carbon (DOC) was determined using deionized water leaching (water:soil ratio 5:1) and an elemental analyzer (CE-440, American Gallian Instruments, Inc., United States); amorphous Fe-Mn was determined using oxalic acid and ammonium oxalate solution leaching, as well as an atomic absorption spectrometer (AA-7000, Daojing Inspection instrument Co., LTD.); soil particle composition was determined via the pipette method; the humus component (huminic acid [HA] and fulvic acid [FA]) was determined via leaching with sodium pyrophosphate and sodium hydroxide solution.
Soil P fractions were measured using a graded method modified by Tiessen, (1993). Resin film leaching was used to measure P (Resin-P), while organic (Po) and inorganic P (Pi) contents were also evaluated using sodium bicarbonate solution (NaHCO3), sodium hydroxide solution (NaOH), and HCl leaching methods. Additionally, ultrasonic treatment extraction was used to measure Po (Sonicate-Po), Pi (Sonicate-Pi), and residual state P from concentrated acid digestion (Residual-P). The sum of all the above P fractions was the total soil P content, and P fractions were all determined via continuous flow fractionation. According to Fan et al. (2019), the uptake and ease of utilization for different P fractions by plants can be classified into the following: 1) Soluble P—e.g., Resin-P, an inorganic P fraction that can be directly absorbed and utilized by plants; 2) labile P—e.g., NaHCO3-Po and NaHCO3-Pi; 3) moderately labile P—e.g., NaOH-Po and NaOH-Pi; 4) occluded P—e.g., Sonicate-Po, Sonicate-Pi, HCl-P, and Residual-P. According to the bio-effectiveness, P fractions were classified into Po (NaHCO3-Po, NaOH-Po, Sonicate-Po), and inorganic P (Resin-P, NaHCO3-Pi, NaOH-Pi, Sonicate-Pi, HCl-P, and Residual-P).
Soil ACP was determined using methods employed by Gai et al. (2021), where 1.00 g of fresh soil sample was extracted with 125 ml of 50 mmol L−1 acetate buffer solution (pH 5) and stirred until uniform with a magnetic stirrer (5 min). Subsequently, the solution was assayed with an umbelliferone substrate and multifunctional enzyme standard (Spectra Max M5, Molecular Devices Instruments Co., LTD., United States). Microbial biomass was also determined using the methods of Gai et al. (2021), where microbial biomass carbon (MBC) was assessed via chloroform fumigation and potassium sulfate leaching with an elemental analyzer (CE-440, American Gallian Instruments, Inc., United States), using a leaching coefficient of 0.45. Microbial biomass phosphorus (MBP) was determined by chloroform fumigation and sodium bicarbonate leaching with a continuous flow analyzer (F 9700, Qingdao Yiyi Instrument Co., LTD.), and a leaching coefficient of 0.40.
Data were processed in MS Excel 2019 and SPSS v.24.0 for statistical analyses. One-way ANOVA was used to analyze significance in SOC and P fractions, as well as MBP and ACP among different paddy cultivation systems (α = 0.05). Paired-sample t-test was used to analyze the significance of SOC and P fractions, MBP, and ACP activity at different soil depths (LSD method; α = 0.05). Two-way ANOVA was used to analyze the interaction effects of cropping systems and soil depth on SOC and P fractions, MBP, and ACP activity, while redundancy analysis (RDA) and variance decomposition analyses (VPA) were conducted in R v.4.03 using the Vegan package with soil physicochemical properties, organic carbon fractions, and microbial factors as environmental factors. Significance testing was performed via the Monte Carlo method, and Origin 2020b (Origin Lab. Inc., Massachusetts, United States) was used for graphical plotting.
The results of the two-way ANOVA showed that the cropping system, soil layer, and their interactions significantly affected SOC, DOC, MBC, HA, FA, and HA:FA, except for effect of the interaction on soil HA and FA; Figures 1A–F). Significant differences in SOC, DOC, MBC, and FA were all detected between cropping systems in topsoil. SOC, DOC, MBC, and FA in the RFD increased by 12.23, 21.36, 56.78, and 20.04% compared to RVS, and by 33.26, 38.37, 216.62, and 66.69% compared to CRS, respectively (Figures 1A–C,E). HA also peaked in RFD, although CRS significantly increased by 44.29% compared to RVS as well (Figure 1D). HA:FA of RVS soil significantly decreased by 88.86 and 100.42% compared to RFD and CRS, respectively (Figure 1F).
FIGURE 1. Soil organic carbon fractions under different cropping systems. (A) SOC. (B) DOC. (C) MBC. (D) HA. (E) FA. (F) the ratio of HA to FA. RFD, rice-fish-duck system; RVS, rice-vegetation cropping system; CRS, conventional rice system. Different lower-case letters in the same soil depth indicate a significant difference among cropping systems at p < 0.05, and different capital letters in the same cropping system indicate a significant difference among soil depths at p < 0.05. SOC, soil organic carbon; DOC, dissolved organic carbon; MBC, microbial biomass carbon; HA, huminic acid; FA, fulvic acid.
Similar to the topsoil, all organic carbon fractions of RFD subsoils were significantly higher than RVS and CRS, with MBC and HA:FA showing the greatest difference in CRS soils compared to RVS, and no significant difference was observed in HA content between CRS and RVS subsoils.
Soil Resin-P, NaHCO3-Pi, NaHCO3-Po, NaOH-Pi, and HCl-P fractions were all as the following order: RFD > RVS > CRS, with significant differences between different cropping systems (Figures 2A–D,H). Soil NaOH-Po and Sonicate-Pi contents in RFD were also significantly higher than those in the RVS and CRS, increasing by 75.30–80.24 and 45.07–53.08%, respectively. Soil Sonicate-Po and Residual-P contents in RFD were significantly greater than those of RVS and CRS by 25.61–53.65 and 26.45–46.17%, respectively (Figures 2G,I). Pi contents in the RFD increased by 56.74% compared to that in the RVS, and by 102.74% compared to those in the CRS, respectively (Figure 3A). Po content in the RFD was also significantly higher than that of the RVS and CRS by 68.28 and 72.09%, respectively (Figure 3B).
FIGURE 2. Soil P fractions under different cropping systems. (A) Resin-P. (B) NaHCO3-Pi. (C) NaHCO3-Po. (D) NaOH-Pi. (E) NaOH-Po. (F) Sonicate-Pi. (G) Sonicate-Po. (H) HCl-P. (I) Residual-P. RFD, rice-fish-duck system; RVS, rice-vegetation cropping system; CRS, conventional rice system. Different lower-case letters indicate a significant difference among cropping systems at p < 0.05.
FIGURE 3. Soil total organic phosphorus, inorganic phosphorus, and available phosphorus contents under different cropping systems. (A) Soil Po. (B) Soil Pi. (C) Soluble P. (D) Labile P. (E) Moderately labile P. (F) Occluded P. RFD, rice-fish-duck system; RVS, rice-vegetation cropping system; CRS, conventional rice system. Different lower-case letters in the same soil depth indicate a significant difference among cropping systems (p < 0.05), and different capital letters in the same cropping system indicate a significant difference among soil depths (p < 0.05). Po, organic phosphorus; Pi, inorganic phosphorus.
A two-way ANOVA indicated that the effects of cropping system and soil layer significantly affected soluble, labile, moderately labile, and occluded P; whereas the interaction effects were only significant on soil labile P content (Figures 3C–F). For the moderately labile P content in the RFD and RVS, soluble, labile, and occluded P contents in the two soil layers differed significantly between different cropping systems, and were significantly higher in topsoil than those in subsoil (Figures 3C–F). The P fraction contents of different cropping systems in the two soil layers showed the same trend, where the contents of soluble, labile, and occluded P showed significant differences between cropping systems (RFD > RVS > CRS). The moderately labile P content was also highest in the RFD, showing an increase of 84.68 and 85.38% compared to RVS and CRS, respectively.
Soil moderately labile P accounted for the largest proportion of total soil P (40.67–49.41% of total soil P; Figure 4); whereas the next most abundant fractions were occluded P (29.26–37.01%), labile P (16.55–23.88%), and soluble P (< 3% of total soil phosphorus content). Figure 4 also shows that RFD significantly increased the proportion of soluble P and reduced the proportion of moderately labile P and occluded P in the soil.
FIGURE 4. Proportion of soil available P fraction under different cropping systems. RFD, rice-fish-duck system; RVS, rice-vegetation cropping system; CRS, conventional rice system.
Soil microbial biomass phosphorus (MBP) content of topsoil was significantly higher than that of subsoil (Figure 5A). The MBP content of topsoil for the RFD was 1.57- and 3.17-times higher than that for RVS and CRS, respectively. The MBP content of subsoil showed the following order: RFD > RVS > CRS, with all differences between cropping systems being significant. ACP activity of topsoil was also significantly higher than that of subsoil (Figure 5B). The variation of topsoil ACP activity between different cropping systems was significant (RFD > RVS > CRS). Subsoil ACP activity in RFD and RVS increased by 18.89 and 16.29%, respectively, compared to that in CRS.
FIGURE 5. Soil microbial biomass phosphorus (A) and acid phosphatase activity (B) under different cropping systems. RFD, rice-fish-duck system; RVS, rice-vegetation system; CRS, conventional rice system. Different lower-case letters in the same soil depth indicate a significant difference among cropping systems (p < 0.05), and different capital letters in the same cropping system indicate a significant difference among soil depths (p < 0.05). MBP, microbial biomass phosphorus; ACP, acid phosphatase activity.
Soil MBP content was positively correlated with amorphous Mn (Mno) content and each organic carbon fraction, and negatively correlated with bulk density (BD; Figure 6A). Similarly, soil ACP activity was positively correlated with pH, each organic carbon fraction, and MBP content, whereas it was negatively correlated with BD. All soil physical and chemical properties, as well as organic carbon fractions, were correlated with soil P fractions, except for HA:FA and pH (Figure 6B).
FIGURE 6. Relationships between soil properties (A) and available P fractions (B). Ns, non-significant; *p < 0.05; **p < 0.01. BD, bulk density; DOC, dissolved organic carbon; SOC, soil organic carbon; Feo: amorphous iron; Mno: amorphous Mn; HA, huminic acid; FA, fulvic acid; HA:FA, the ratio of HA to FA; MBC, microbial biomass carbon; MBP, microbial biomass phosphorus; ACP, acid phosphatase activity.
Here, Resin-P was used as the response variable to explore the contribution of different P fractions to soil fast-acting P. The results of path analysis showed that the direct effect of P fractions was as following order: NaOH-Pi > HCl-P > Residual-P, all showing positive effects (Table 2). This indicated that NaOH-Pi was the primary contributor to fast-acting soil P in this region.
The redundancy analysis showed that axis one explained 82.90% of the variability observed, while axis two explained 10.07% of the variability (Figure 7A). The presence of significant clustering between different cropping systems indicated that different cropping systems can significantly change soil P fractions. By the Monte Carlo test, organic carbon fractions (DOC, HA:FA, HA, and FA), and soil microbial factors (ACP) were significantly correlated with soil P variability. The variability of P fractions were contributed by DOC (79.80%), and HA:FA (9.00%). By VPA, soil physicochemical properties (pH, BD, Feo, and Mno), organic carbon fractions (SOC, DOC, HA, FA, MBC, and HA:FA), and microbial factors (MBP and ACP) explained 2.35, 3.22, and 1.41% of the variability in the soil P fraction, respectively. Moreover, the interaction effects of organic carbon fraction and soil physicochemical properties explained 63.98% of the variation in soil P fraction (Figure 7B). The combination of soil physicochemical properties, SOC fractions, and soil microbial properties explained 16.77% of the variation in soil P fractionation.
FIGURE 7. Redundancy analysis (A) and variation partitioning analysis (B) of soil P fractions under different cropping systems. Points indicate cropping systems, vector lines indicate environmental factors, and red vector lines indicate the significant effects of environmental factors on soil phosphorus fractionation. RFD, rice-fish-duck system; RVS, rice vegetation cropping system; CRS, conventional rice system. BD, bulk density; DOC, dissolved organic carbon; SOC, soil organic carbon; Feo: amorphous iron; Mno: amorphous Mn; HA, huminic acid; FA, fulvic acid; HA:FA, the ratio of HA to FA; MBC, microbial biomass carbon; MBP, microbial biomass phosphorus; ACP, acid phosphatase activity.
As one of the most important limiting nutrients in the soil, the P availability determines soil productivity and is closely related to plant growth and development. Soil Po, Pi, and the transformation between soil P components are critical to the supply balance of effective P in regional soils. Po is an important component of the soil P pool. Especially in soils with low Pi content or high sequestration capacity, Po can be rapidly mineralized and converted into effective P for plant uptake/utilization through microbial metabolic activities. In our study, Po content accounted for 46.83–54.88% of total soil P, which is consistent with the results of Redel et al. (2008). Cropping systems had significant effects on soil Po and Pi contents, moreover, RFD soil had greater Po and Pi contents than those of RVS and CRS. It is thus argued here that fish and duck excrement into the soil increased the input of exogenous soil organic residue in the RFD, and the functional groups of organic matter abundance by ionic chemical bonds can combine with soil minerals to form organic complexes (Cavalcante et al., 2018; Fu et al., 2021). This process can improve the retention of soil P-elements. Additionally, bioturbation, such as fish and duck foraging, can change the biochemical transformation of organic matter at the aerobic and anaerobic interfaces, accelerating the decomposition of soil organic matter to produce organic acids and other substances (Fanjul et al., 2015). This action stimulated the mineralization of highly reactive soil Po to Pi through biochemical mechanisms.
The study of soil P fraction is important for understanding the cycling processes of P in paddy soil ecosystems. Here, the contents of soluble and labile P in the topsoil of the RFD were significantly greater than those of RVS and CRS, indicating that an integrated ecological cropping system can effectively improve the supply capacity of effective P in paddy soils in the PRD. Alternatively, the CRS was least effective in that the long-term rice succession soil conditions deteriorated, and soil P were more easily adsorbed or chemically fixed by soil colloids, such as clay particles, or iron and aluminum oxides. Studies have shown that pH can change the exchange between soil colloidal material and phosphate ions in the soil solution, affecting both soil P fixation and release (Ahmed et al., 2020). Higher pH soil solutions contain higher free hydroxyl groups that can bind to adsorption sites on the surface of iron and aluminum oxide colloids while releasing soil-fixed P (Lü et al., 2017; Ahmed et al., 2020). Alternatively, proper soil pH stimulates vital activities of soil microorganisms, as the phosphatase that mineralizes soil P is mainly derived from microorganisms, and its activity is closely related to the abundance of the microbial community (Wan et al., 2021).
Moderately labile P was dominated by NaOH leaching Po and Pi. NaOH-Po is closely related to the fraction and content of soil humus; whereas NaOH-Pi is mostly fixed by adsorption of soil iron and aluminum oxides (Maranguit et al., 2017). The input of fish and duck excreta from the RFD led to a large amount of unstable carbon entering the soil, while the microbial decomposition and mineralization of unstable carbon gradually consumed the soil’s dissolved oxygen. This process intensified the local anaerobic environment, and increased the activity of anaerobic reducing bacteria, promoting the conversion of hydroxytrivalent iron to hydroxydivalent iron, which is the primary component of amorphous iron, with a larger active surface area capable of increasing the ability to absorb and affix soil P (Chacon et al., 2006; Fan et al., 2019; Fu et al., 2021). While the undecomposed organic material underwent a humification process to form humic acid-like substances, the unique gel properties of these substances can combine with more active P components to form NaOH-Po, and reduce soil P availability (Maranguit et al., 2017). Simultaneously, humic substances decarboxylated slowly under anaerobic conditions and were easily complexed with Fe and Al oxides to form organic-inorganic composite colloids (Atere et al., 2020) capable of releasing phosphate ions by competing with them for adsorption sites on the surface of inorganic complexes.
Occluded P was primarily in the form of stable Ca-P, notably difficult to absorb and for plant use within short periods. Here, the percentage of refractory P to total soil P in the RFD was significantly reduced, likely because the input of exogenous organic matter acted on the P-solubilizing and P-dissolving bacterial communities, promoting the mineralization and release of the original refractory P in the soil.
There were significant differences in soil MBP with changes in rice cropping systems. Soil MBP content was related to soil organic carbon content. With sufficient soil carbon sources in RFD, soil microorganisms will adjust their own nutrient uptake strategy, and consume more energy to obtain more P, to maintain their own reasonable stoichiometric ratio (Fujita et al., 2017). The RVS and CRS provided insufficient carbon sources, and the decomposition and transformation of organic matter were biased towards P-free organic matter, which was neither acquired nor used by microorganisms at this time and thus shows a lower content (Zhang et al., 2022).
ACP plays an important role in soil P turnover and is significantly positive correlated with SOC, DOC, HA, and FA in the present study. Further, the high concentrations of these factors in the RFD provided abundant nutrients and energy for microbial activities and synthesis of soil ACP. Additionally, soil BD showed significant relationship with ACP, the former may indirectly affect ACP activity by influencing soil permeability, water and fertilizer storage capacity, as well as microbial reproduction. Dong et al. (2019) showed that microbial energy allocation is closely related to the ability to secrete extracellular enzymes. The observed microbial biomass (both MBC and MBP) and ACP activity changes in the present study tended to be consistent. This result strongly supports that microorganisms can use more energy for synthesizing ACP by regulating their energy allocation to mineralize more soil P, thereby obtaining available P (Fujita et al., 2017).
All soil P fractions in this study were highly positively correlated with the rapid-acting P fraction (Resin-P), indicating that all soil P fractions were important P sources that affected soil P availability. The results of path analysis showed that NaOH-Pi had the highest direct path coefficient (0.42) on soil fast-acting P, indicating that NaOH-Pi is a sensitive P source to regional soil P availability.
Hou et al. (2018) found that both soil physicochemical properties (pH, organic carbon, etc.) and biological factors (plant roots, microbial secretions, and their vital activities) strongly affect soil P fraction turnover. The results of redundancy analysis indicated that the interaction effects of soil physicochemical properties and organic carbon fractionation drove the observed changes in soil P fraction, especially for DOC, HA:FA, HA, and FA. Some studies have shown that after exogenous organic matter enters the soil, it will undergo mineralization and decomposition due to the soil excitation effect. Here, part of Po is converted into Pi, thus increasing the soil available P content. In the later stages, the mineralization and decomposition rates of soil organic matter are lower than the soil P sequestration rate, and Po content. Chen Y. et al. (2020) found that microorganisms require more carbon than P for mineralization and decomposition of soil Po, where enough carbon can promote higher mineralization rates of soil Po. Thus, RFD soils exhibit increased P activity and Po content. The mineralization and decomposition of organic matter continuously releases H+, which protonates the surface of soil colloids, while affecting the adsorption and sequestration of soil P (Blonska et al., 2021; Guo et al., 2021). Different organic carbon fractions affect the transformation of soil P fraction by different mechanisms. HA and low molecular weight organic acids inhibited the adsorption of P compounds by reducing the available sites on the soil surface. This inhibition effect increases with the number of carboxyl groups, and the molecular weight of organic acids (Lü et al., 2017). In contrast, DOC, a class of organic carbon molecules in a largely free and active state, can colloidize with iron oxides in the form of adsorption or co-precipitation to form nanoscale particles (Moens and Smolders, 2021). This process can activate a steady-state of P, and enhance the release of colloidal/nano-P from soil solutions (Gu et al., 2018; Moens and Smolders, 2021), block the contact of extracellular enzymes and heterotrophic microorganisms (Chen C. M. et al., 2020; Yu et al., 2021), and indirectly affect the mineralization turnover of soil Po.
Soil ACP activity is another key factor affecting the transformation of soil P fractionation. Some studies (Zhang et al., 2017; Zhou et al., 2020) have shown that ACP is secreted and synthesized into the soil by plants or microorganisms, and can break phosphodiester bonds to release bound P for plant uptake and utilization. These studies showed that 20–80% of soil Po can be hydrolyzed by ACP. In this study, ACP activity was strongly correlated with SOC fractions (SOC, DOC, HA and FA), primarily because organic carbon is the main carrier of organic P, and served as a substrate for soil ACP decomposition and mineralization; thus, an increase in substrate content within a certain range can significantly increase soil ACP activity (Hou et al., 2015). Further, PRD receives strong nitrogen deposition, which enhances soil microbial activity and stimulates ACP secretion; whereas ACP accelerates the decomposition of organic matter and the release of soil sequestered P, increasing the production of soil available P (Vitousek et al., 2010; Chen et al., 2018). In summary, soil microorganisms in PRD paddy fields promote the conversion of soil P fractionation by regulating organic carbon fractions and increasing ACP activity, thereby improving soil P bio-effectiveness. However, we do not yet know which soil microbial populations play a crucial role in Po mineralization, and this will be the subject of subsequent research.
Cropping systems significantly affected the content of total soil P and P fractions in the paddy fields of the PRD. Here, moderately labile P was the most abundant form, accounting for 40.67–49.41% of soil total P. The RFD significantly increased the soil total P, Po, Pi, and each P fraction content in paddy fields. The RFD also strongly increased soil MBC, MBP, and ACP activity, and could promote organic P mineralization and improve soil P bioavailability. The path analysis indicated that NaOH-Pi is a sensitive P source for biologically effective P in the paddy soils of the PRD. Organic carbon fraction and ACP activity are the key factors driving the conversion of soil P fractionation, indicating that soil P effectiveness is the result of the combined influential factors from biochemical process. Accordingly, the RFD can alleviate problems associated with low P activity by strongly improving phosphorus fractionation in the PRD paddy soils, representing an essential step towards achieving rational fertilization and sustainable use of regional soils.
The original contributions presented in the study are included in the article/Supplementary Material, further inquiries can be directed to the corresponding author.
CW analysed the data and wrote the manuscript. QY, CZ, XL, and KL designed the study, and contributed substantially to revisions. JC, XZ, and JC performed the experiment.
This study is supported by Guangdong Natural Fund (2021A1515011543), Guangdong Education Science 13th Five-Year Plan Project (2020GXJK116), and Key Project of Natural Science of Guangzhou Xinhua University (2020KYZD02).
We would like to thank Editage (www.editage.cn) for English language editing.
The authors declare that the research was conducted in the absence of any commercial or financial relationships that could be construed as a potential conflict of interest.
All claims expressed in this article are solely those of the authors and do not necessarily represent those of their affiliated organizations, or those of the publisher, the editors and the reviewers. Any product that may be evaluated in this article, or claim that may be made by its manufacturer, is not guaranteed or endorsed by the publisher.
The Supplementary Material for this article can be found online at: https://www.frontiersin.org/articles/10.3389/fenvs.2022.979234/full#supplementary-material
Afri, H., Murwantoko, M., and Indah, I. (2021). Dynamic change in bacterial communities in the integrated rice-fish farming system in Sleman, Yogyakarta, Indonesia. Aquac. Res. 52 (11), 5566–5578. doi:10.1111/are.15432
Ahmed, A. A., Gypser, S., Freese, D., Leinweber, P., and Kuhn, O. (2020). Molecular level picture of the interplay between pH and phosphate binding at the goethite-water interface. Phys. Chem. Chem. Phys. 22, 26509–26524. doi:10.1039/d0cp04698a
Atere, C. T., Gunina, A., Zhu, Z. K., Xiao, M. L., Liu, S. L., Kuzyakov, Y., et al. (2020). Organic matter stabilization in aggregates and density fractions in paddy soil depending on long term fertilization: Tracing of pathways by 13C natural abundance. Soil Biol. Biochem. 149, 107931. doi:10.1016/j.soilbio.2020.107931
Blonska, E., Piaszczyk, W., Staszel, K., and Lasota, J. (2021). Enzymatic activity of soils and soil organic matter stabilization as an effect of components released from the decomposition of litter. Appl. Soil Ecol. 157, 103723. doi:10.1016/j.apsoil.2020.103723
Bronson, K. F., Zobeck, T. M., Chua, T. T., Acosta-Martinez, V., Scott van Pelt, R., and Booker, J. D. (2004). Carbon and nitrogen pools of southern high plains cropland and grassland soils. Soil Sci. Soc. Am. J. 68, 1695–1704. doi:10.2136/sssaj2004.1695
Cavalcante, H., Araujo, F., Noyma, N. P., and Becker, V. (2018). Phosphorus fractionation in sediments of tropical semiarid reservoirs. Sci. Total Environ. 619, 1022–1029. doi:10.1016/j.scitotenv.2017.11.204
Chacon, N., Silver, W. L., Dubinsky, E. A., and Cusack, D. F. (2006). Iron reduction and soil phosphorus solubilization in humid tropical forests soils: The roles of labile carbon pools and an electron shuttle compound. Biogeochemistry 78, 67–84. doi:10.1007/s10533-005-2343-3
Chen, C. M., Hall, S. H., Coward, E., and Thompson, A. (2020a). Iron-mediated organic matter decomposition in humid soils can counteract protection. Nat. Commun. 11, 2255. doi:10.1038/s41467-020-16071-5
Chen, H., Chen, M. L., Li, D. J., Mao, Q. G., Zhang, W., and Mo, J. M. (2018). Responses of soil phosphorus availability to nitrogen addition in a legume and a non-legume plantation. Geoderma 322, 12–18. doi:10.1016/j.geoderma.2018.02.017
Chen, Y., Sun, R. B., Sun, T. T., Chen, P., Yu, Z. Y., Ding, Y., et al. (2020b). Evidence for involvement of keystone fungal taxa in organic phosphorus mineralization in subtropical soil and the impact of labile carbon. Soil Biol. Biochem. 148, 107900. doi:10.1016/j.soilbio.2020.107900
Cui, E. Q., Lu, R. L., Xu, X. N., Sun, H. F., Qiao, Y., Ping, J. Y., et al. (2022). Soil phosphorus drives plant trait variations in a mature subtropical forest. Glob. Chang. Biol. 28, 3310–3320. doi:10.1111/gcb.16148
Divjot, K., Rana, K. L., Tanvir, K., Yadav, N., Yadav, A. N., Kumar, M., et al. (2021). Biodiversity, current developments and potential biotechnological applications of phosphorus-solubilizing and-mobilizing microbes: A review. Pedosphere 31, 43–75. doi:10.1016/S1002-0160(20)60057-1
Dong, C. C., Wang, W., Liu, H. Y., Xu, X. T., and Zeng, H. (2019). Temperate grassland shifted from nitrogen to phosphorus limitation induced by degradation and nitrogen deposition: Evidence from soil extracellular enzyme stoichiometry. Ecol. Indic. 101, 453–464. doi:10.1016/j.ecolind.2019.01.046
Fan, Y. X., Zhong, X. J., Lin, F., Liu, C. C., Yang, L. M., Wang, M. H., et al. (2019). Responses of soil phosphorus fractions after nitrogen addition in a subtropical forest ecosystem: Insights from decreased Fe and Al oxides and increased plant roots. Geoderma 337, 246–255. doi:10.1016/j.geoderma.2018.09.028
Fanjul, E., Escapa, M., Montemayor, D., Addino, M., Alvarez, M. F., Grela, M. A., et al. (2015). Effect of crab bioturbation on organic matter processing in South West Atlantic intertidal sediments. J. Sea Res. 95, 206–216. doi:10.1016/j.seares.2014.05.005
Fishery Bureau of China's Ministry of Agriculture (2013). China fishery statistical Yearbook. China: China Agriculture Press.
Fisk, M., Santangelo, S., and Minick, K. (2015). Carbon mineralization is promoted by phosphorus and reduced by nitrogen addition in the organic horizon of northern hardwood forests. Soil Biol. Biochem. 81, 212–218. doi:10.1016/j.soilbio.2014.11.022
Fu, D., Xu, Z., Wu, X., Zhao, L., Zhu, A., Duan, C., et al. (2021). Land use effects on soil phosphorus behavior characteristics in the eutrophic aquatic-terrestrial ecotone of Dianchi Lake, China. Soil Tillage Res. 205, 104793. doi:10.1016/j.still.2020.104793
Fujita, K., Kunito, T., Moro, H., Toda, H., Otsuka, S., and Nagaoka, K. (2017). Microbial resource allocation for phosphatase synthesis reflects the availability of inorganic phosphorus across various soils. Biogeochemistry 136, 325–339. doi:10.1007/s10533-017-0398-6
Gai, X., Li, S. C., Zhang, X. P., Bian, F. Y., Yang, C. B., and Zhong, Z. K. (2021). Effects of chicken farming on soil extracellular enzyme activity and microbial nutrient limitation in Lei bamboo forest (Phyllostachys praecox) in subtropical China. Appl. Soil Ecol. 168, 104106. doi:10.1016/j.apsoil.2021.104106
Gu, S., Gruau, G., Malique, F., Dupas, R., Petitjean, P., and Gascuel-Odoux, C. (2018). Drying/rewetting cycles stimulate release of colloidal-bound phosphorus in riparian soils. Geoderma 321, 32–41. doi:10.1016/j.geoderma.2018.01.015
Guangdong Bureau of Statistics (2018). Guangdong statistical Yearbook. China: China Agriculture Press.
Guo, L., Hu, L. L., Zhao, L. F., Shi, X. Y., Zijun, J., Lilian, D., et al. (2020). Coupling rice with fish for sustainable yields and soil fertility in China. Rice Sci. 27, 175–179. doi:10.1016/j.rsci.2020.04.001
Guo, X. X., Wu, S. B., Wang, X. Q., and Liu, H. T. (2021). Impact of biochar addition on three-dimensional structural changes in aggregates associated with humus during swine manure composting. J. Clean. Prod. 280, 124380. doi:10.1016/j.jclepro.2020.124380
Hou, E. Q., Chen, C. R., Luo, Y. Q., Zhou, G. Y., Kuang, Y. W., Zhang, Y. G., et al. (2018). Effects of climate on soil phosphorus cycle and availability in natural terrestrial ecosystems. Glob. Chang. Biol. 24, 3344–3356. doi:10.1111/gcb.14093
Hou, E. Q., Chen, C. R., Wen, D. Z., and Liu, X. (2015). Phosphatase activity in relation to key litter and soil properties in mature subtropical forests in China. Sci. Total Environ. 515–516, 83–91. doi:10.1016/j.scitotenv.2015.02.044
Hou, E. Q., Luo, Y. Q., Kuang, Y. W., Chen, C. R., Lu, X. K., Jiang, L. F., et al. (2020). Global meta-analysis shows pervasive phosphorus limitation of aboveground plant production in natural terrestrial ecosystems. Nat. Commun. 11, 637. doi:10.1038/s41467-020-14492-w
Hu, L. L., Zhang, J., Ren, W. Z., Guo, L., Cheng, Y. X., Li, J. Y., et al. (2016). Can the co-cultivation of rice and fish help sustain rice production? Sci. Rep. 6, 28728. doi:10.1038/srep28728
Lai, C. G., Chen, X. H., Wang, Z. L., Wu, X. S., Zhao, S. W., Wu, X. Q., et al. (2015). Spatio-temporal variation in rainfall erosivity during 1960-2012 in the Pearl River basin, China. Catena 137, 382–391. doi:10.1016/j.catena.2015.10.008
Lin, K. M., and Wu, J. P. (2020). Effect of introducing frogs and fish on soil phosphorus availability dynamics and their relationship with rice yield in paddy fields. Sci. Rep. 10, 21. doi:10.1038/s41598-019-56644-z
Liu, G. B., Huang, H., and Zhou, J. W. (2019). Energy analysis and economic assessment of a rice-turtle-fish co-culture system. Agroecol. Sustain. Food Syst. 43, 299–309. doi:10.1080/21683565.2018.1510870
Lü, C., Yan, D., He, J., Zhou, B., Li, L., and Zheng, Q. (2017). Environmental geochemistry significance of organic phosphorus: An insight from its adsorption on iron oxides. Appl. Geochem. 84, 52–60. doi:10.1016/j.apgeochem.2017.05.026
Lv, W. W., Yuan, Q., Lv, W. G., and Zhou, W. Z. (2020). Effects of introducing eels on the yields and availability of fertilizer nitrogen in an integrated rice-crayfish system. Sci. Rep. 10, 14818. doi:10.1038/s41598-020-71884-0
Macdonald, G. K., Bennett, E. M., Potter, P. A., and Ramankutty, N. (2011). Agronomic phosphorus imbalances across the world's croplands. Proc. Natl. Acad. Sci. U. S. A. 108, 3086–3091. doi:10.1073/pnas.1010808108
Maranguit, D., Guillaume, T., and Kuzyakov, Y. (2017). Land-use change affects phosphorus fractions in highly weathered tropical soils. Catena 149, 385–393. doi:10.1016/j.catena.2016.10.010
Moens, C., and Smolders, E. (2021). Suwannee River Natural Organic Matter concentrations affect the size and phosphate uptake of colloids formed by iron oxidation. Geochim. Cosmochim. Acta 312, 375–391. doi:10.1016/j.gca.2021.07.028
Ning, J. F., Arenberg, M., and Arai, Y. (2021). Phosphorus mineralization affected by urea in an intensively managed agricultural soil. Soil Sci. Soc. Am. J. 85 (6), 2067–2076. doi:10.1002/saj2.20324
Ortiz-Reyes, E., and Anex, R. P. (2018). A life cycle impact assessment method for freshwater eutrophication due to the transport of phosphorus from agricultural production. J. Clean. Prod. 177, 474–482. doi:10.1016/j.jclepro.2017.12.255
Redel, Y., Rubio, R., Godoy, R., and Borie, F. (2008). Phosphorus fractions and phosphatase activity in an Andisol under different forest ecosystems. Geoderma 145, 216–221. doi:10.1016/j.geoderma.2008.03.007
Sharma, L. K., Bali, S. K., and Zaeen, A. A. (2017). A case study of potential reasons of increased soil phosphorus levels in the Northeast United States. Agron. (Basel). 7, 85. doi:10.3390/agronomy7040085
Shi, S. W., Peng, C. H., Wang, M., Zhu, Q., Yang, G., Yang, Y. Z., et al. (2016). A global meta—Analysis of changes in soil carbon, nitrogen, phosphorus and sulfur, and stoichiometric shifts after forestation. Plant Soil 407, 323–340. doi:10.1007/s11104-016-2889-y
Sokol, N. W., Slessarev, E., Marschmann, G. L., Nicolas, A., Blazewicz, S. J., Brodie, E. L., et al. (2022). Life and death in the soil microbiome: How ecological processes influence biogeochemistry. Nat. Rev. Microbiol. 20, 415–430. doi:10.1038/s41579-022-00695-z
Sun, G., Sun, M., Du, L. S., Zhang, Z., Wang, Z. C., Zhang, G. B., et al. (2021). Ecological rice-cropping systems mitigate global warming – a meta-analysis. Sci. Total Environ. 789, 147900. doi:10.1016/j.scitotenv.2021.147900
Sun, K. J., Zhao, B., Lu, Q. M., and Liao, Z. W. (2007). Study on release characteristics, fertilizer effect and activated mechanism of activated phosphoric fertilizers. Sci. Agric. Sin. 40, 1722–1729. doi:10.3321/j.issn:0578-1752.2007.08.018
Teng, Z. D., Zhu, Y. Y., Li, M., and Whelan, M. J. (2018). Microbial community composition and activity controls phosphorus transformation in rhizosphere soils of the Yeyahu Wetland in Beijing, China. Sci. Total Environ. 628, 1266–1277. doi:10.1016/j.scitotenv.2018.02.115
Tiessen, J. M. H. (1993). Characterization of available P by sequential extraction. Soil Sampl. methods analysis 7, 225–229. doi:10.1201/9781420005271
Vitousek, P. M., Porder, S., Houlton, B. Z., and Chadwick, O. A. (2010). Terrestrial phosphorus limitation: Mechanisms, implications, and nitrogen-phosphorus interactions. Ecol. Appl. 20, 5–15. doi:10.1890/08-0127.1
Wan, N. F., Li, S. X., Li, T., Cavalieri, A., Weiner, J., Zheng, X. Q., et al. (2019). Ecological intensification of rice production through rice-fish co-culture. J. Clean. Prod. 234, 1002–1012. doi:10.1016/j.jclepro.2019.06.238
Wan, W. J., Hao, X. L., Xing, Y. H., Liu, S., Zhang, X. Y., Li, X., et al. (2021). Spatial differences in soil microbial diversity caused by pH-driven organic phosphorus mineralization. Land Degrad. Dev. 32, 766–776. doi:10.1002/ldr.3734
Wu, B. L., Chen, J., Huang, L., Zhang, Y., Fang, Y., and He, J. X. (2021). Dynamics of soil fertility and microbial community response to stocking density in rice-turtle co-culture. Aquac. Rep. 20, 100765. doi:10.1016/j.aqrep.2021.100765
Yi, X., Yi, K., Fang, K., Gao, H., Dai, W., and Cao, L. (2019). Microbial community structures and important associations between soil nutrients and the responses of specific taxa to rice-frog cultivation. Front. Microbiol. 10, 1752–1767. doi:10.3389/fmicb.2019.01752
Yu, C. X., Xie, S. R., Song, Z. L., Xia, S. P., and Astrom, M. E. (2021). Biogeochemical cycling of iron (hydr-)oxides and its impact on organic carbon turnover in coastal wetlands: A global synthesis and perspective. Earth. Sci. Rev. 218, 103658. doi:10.1016/j.earscirev.2021.103658
Zhang, L., Jia, S. X., Li, S. L., Lu, Y. M., Lin, W. S., and Guo, J. F. (2022). Effects of litter and root inputs changes on soil phosphorus fractions in a subtropical natural forest of Castanopsis carlesii. Acta Ecol. Sin. 42, 656–666. doi:10.5846/stxb202101240250
Zhang, X. Y., Yang, Y., Zhang, C., Niu, S. L., Yu, G. R., Wang, H. M., et al. (2017). Contrasting responses of phosphatase kinetic parameters to nitrogen and phosphorus additions in forest soils. Funct. Ecol. 32, 106–116. doi:10.1111/1365-2435.12936
Zheng, H. B., Huang, H., Chen, C., Fu, Z. Q., Xu, H. Q., Tan, S. D., et al. (2017). Traditional symbiotic farming technology in China promotes the sustainability of a flooded rice production system. Sustain. Sci. 12, 155–161. doi:10.1007/s11625-016-0399-8
Keywords: rice-fish-duck farming, pearl river delta, paddy soils, phosphorus fraction, phosphorus availability, aquaculture
Citation: Wang C, Yang Q, Zhang C, Li X, Chen J, Zhang X, Chen J and Liu K (2022) Rice-fish-duck system regulation of soil phosphorus fraction conversion and availability through organic carbon and phosphatase activity. Front. Environ. Sci. 10:979234. doi: 10.3389/fenvs.2022.979234
Received: 27 June 2022; Accepted: 13 October 2022;
Published: 25 October 2022.
Edited by:
Ryuichiro Shinohara, National Institute for Environmental Studies (NIES), JapanReviewed by:
Tapan Kumar Adhya, KIIT University, IndiaCopyright © 2022 Wang, Yang, Zhang, Li, Chen, Zhang, Chen and Liu. This is an open-access article distributed under the terms of the Creative Commons Attribution License (CC BY). The use, distribution or reproduction in other forums is permitted, provided the original author(s) and the copyright owner(s) are credited and that the original publication in this journal is cited, in accordance with accepted academic practice. No use, distribution or reproduction is permitted which does not comply with these terms.
*Correspondence: Kexue Liu, MjgyNTc0NDhAcXEuY29t
Disclaimer: All claims expressed in this article are solely those of the authors and do not necessarily represent those of their affiliated organizations, or those of the publisher, the editors and the reviewers. Any product that may be evaluated in this article or claim that may be made by its manufacturer is not guaranteed or endorsed by the publisher.
Research integrity at Frontiers
Learn more about the work of our research integrity team to safeguard the quality of each article we publish.