- School of Ocean Science and Engineering, The University of Southern Mississippi, Ocean Springs, MS, United States
Seagrass beds are important submerged coastal habitats that support nearshore communities. Ruppia maritima (widgeon grass) is a widespread seagrass species that undergoes dramatic changes in morphology at the onset of reproduction. The goal of this study was to compare fish assemblages associated with reproductive and non-reproductive R. maritima, recognizing the morphological change undergone by the plant when flowering. During the peak reproductive season in August and September 2021, R. maritima meadows at the northern extent of the Chandeleur Islands, Louisiana were sampled to describe the spatial distribution and morphology of reproductive plants and investigate habitat use by fish assemblages. We assessed spatial trends in R. maritima presence and occurrence of reproductive plants and evaluated differences in shoot morphology. We calculated total fish density, Shannon diversity, and species richness to describe fish assemblages in reproductive and non-reproductive meadows. Additionally, general additive models were used to predict drivers of fish assemblage metrics. Results indicate that R. maritima was distributed along the entire length of the sampled area, but reproductive plants were only located in the central, protected portion of the island. Reproductive plants were more morphologically complex with longer shoots, greater surface area, and more leaves, but this did not impact fish assemblages. Rather, fish abundance was related to R. maritima biomass. This study provides information on patterns and drivers of habitat use by fish in R. maritima-dominated ecosystems that can be used to inform management and restoration.
Introduction
Seagrasses are submerged angiosperms that inhabit marine environments, and include over 60 species across 13 genera (Hartog and Kuo, 2006). The majority of seagrass genera occur at temperate and tropical latitudes in the northern and southern hemispheres (Hartog and Kuo, 2006), and species’ distributions are determined by taxon-specific light, nutrient, salinity, temperature, pH, and substrate requirements (Hemminga and Duarte, 2000; Hartog and Kuo, 2006; McKenzie et al., 2016). Seagrasses may exhibit changes in geographical range or localized abundance as a result of changes in underlying abiotic parameter baselines (Cho et al., 2009; Fourqurean et al., 2003; Johnson et al., 2003).
Seagrass expansion can occur through asexual or sexual processes. Clonal propagation (asexual reproduction) through fragmentation or rhizome extension is considered the primary mechanism for meadow formation and maintenance (Olesen et al., 2004); however, seagrasses also have the capacity for sexual reproduction, with species being either monecious (i.e., both male and female organs on a single plant; e.g., Ruppia maritima) or dioecious (i.e., separate male and female plants; e.g., Thalassia testudinum) (Hartog and Kuo, 2006). Whereas some species produce seeds that germinate on the shoot (e.g., T. testudinum), the majority of seagrasses (42 species) produce seeds that can remain dormant for extended periods of time and create seed reserves in the sediment that provide a buffer against disturbance (Darnell et al., 2021); in highly disturbed environments, dormant seeds can be essential to the recolonization process (Olesen et al., 2004; Orth et al., 2006).
Seagrass beds support faunal communities that are highly diverse and productive (Castillo-Rivera et al., 2002) and include valuable recreationally and commercially fished species (Bertelli and Unsworth, 2014; Nordlund et al., 2018). Seagrasses provide shelter from predators for small cryptic species and serve as productive feeding grounds (Heck et al., 2003; Vaslet et al., 2012; Nordlund et al., 2018). Animals often rely on seagrass habitats for particular life stages, most importantly as nursery habitat for juveniles. McDevitt-Irwin et al. (2016) conducted a meta-analysis of 51 studies and found that seagrasses tended to support greater abundances of juveniles with higher survival rates compared to marsh, reef, mangrove, and other benthic habitats. Juveniles of many species utilize the abundant food and resources within seagrasses to achieve faster growth rates prior to moving to more competitive adult habitats (McDevitt-Irwin et al., 2016).
Many drivers of fish assemblages have been identified and include local environmental conditions as well as the species and morphology of the seagrasses present, and at a broader scale, climate patterns. Distinct fish assemblages among seagrass species can be linked to differences in plant morphology (Rotherham and West, 2002; Nakaoka, 2005; Kiggins et al., 2019). Morphological characteristics (e.g., leaf number and length and shoot density) create microhabitats that may be favored by certain species because of the food, shelter, and/or other ecological benefits provided. Hyndes et al. (1996), for example, reported that whiting species (Sillaginidae) in southwestern Australia showed preference for Zostera spp. Because the less dense canopy allows for easier movement when compared to the other local seagrasses Posidonia australis and P. sinuosa. More recently, Belgrad et al. (2021) reported that abundances of several nekton species were influenced by both shoot density and canopy height of turtlegrass (T. testudinum) (Belgrad et al., 2021).
Ruppia maritima is a widely distributed seagrass with a broad tolerance to environmental conditions that allows it to thrive in waters ranging from fresh to hypersaline in both the northern and southern hemispheres from tropical to temperate latitudes (Orth and Moore, 1988; Reyes and Merino, 1991). Ruppia maritima is an early successional species that is often the first species to colonize an area following a disturbance event (Cho et al., 2009). This is due to high rates of shoot turnover and the species’ reliance on sexual reproduction that creates a persistent seed bank buried in the sediment (Kilminster et al., 2015). In some areas, R. maritima is perennial, whereas in other areas, plants are predominantly annual (Malea et al., 2004). Ruppia maritima is monecious, having both male and female reproductive structures on a single plant, and when reproductive, the morphology of R. maritima shoots change dramatically; whereas non-reproductive R. maritima shoots have several narrow (1–2 mm) leaves ranging from 5 to 20 cm in length (Kantrud, 1997; Hartog and Kuo, 2006), reproductive shoots branch extensively and can reach a length of 2.5 m (Hartog and Kuo, 2006) (Figure 1).
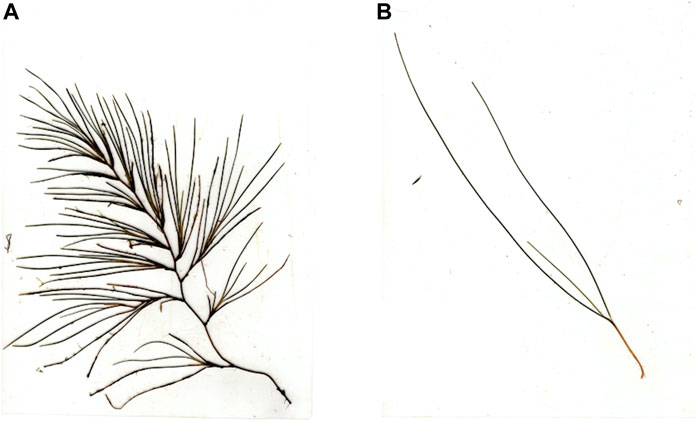
FIGURE 1. Comparison of non-reproductive and reproductive R. maritima shoots (A) reproductive R. maritima and (B) non-reproductive R. maritima.
Ruppia maritima plants at temperate latitudes undergo one annual reproductive cycle, typically in the summer months (Bigley and Harrison, 1986), while those found in more tropical regions usually complete two reproductive cycles (Pulich, 1985; Orth & Moore, 1988). Cho and Poirrier (2005) reported that R. maritima in Lake Pontchartrain, LA, flowers in spring (March to May) and again in late summer/early fall (August to October). The change in plant growth form with the onset of reproduction increases structural complexity and has the potential to impact its use as habitat, as the reproductive shoots may indirectly provide increased opportunities for food and shelter through the creation of additional microhabitats. Ruppia maritima is a known habitat for fish, and Kanouse et al. (2006) reported fish densities ranging from 10 to 102 individuals per meter square in R. maritima-dominated brackish ponds in Louisiana. It is critical to understand patterns in the distribution of reproductive plants as a predictor of the habitat value of R. maritima, especially in areas experiencing changes in seagrass distribution and shifts in species composition.
The Chandeleur Islands, LA, United States, a chain of barrier islands in the northern Gulf of Mexico, represent an area of shifting seagrass species distribution and composition. The islands are suffering chronic land loss due to a lack of sediment input, hurricane damage, and rising sea levels (Moore et al., 2014). Additionally, the Deepwater Horizon oil spill in 2010 impacted sediment and vegetation along the islands with both showing increased levels of total polycyclic aromatic hydrocarbons, the long-term impacts of which are unknown (Kenworthy et al., 2017). The back barrier shelf to the west of the Chandeleur Islands is protected from wave action which allows for the proliferation of extensive seagrass meadows (Ellinwood, 2008). There are five species of seagrass that grow along the leeward protected side of the islands: R. maritima, shoal grass (Halodule wrightii), turtlegrass, manatee grass (Syringodium filiforme), and star grass (Halophila engelmannii). Ruppia maritima, shoal grass, and turtlegrass are the three most abundant species along the islands, with manatee grass and star grass much less abundant (Kenworthy et al., 2017). Seagrass meadows at the Chandeleur Islands represent the only mixed meadows of these five species along 1,000 km of coastline from Perdido Key, Florida to the Texas Coastal Bend, United States (Darnell et al., 2017). Seagrass cover has decreased along the island chain from 15,758 acres in 1969 to only 2,614 acres in 2011 (Pham et al., 2014; Handley and Lockwood, 2020). The observed decline is linked to a reduction of shallow protected areas through the combined processes of changes in island geomorphology and storm-related land loss (Darnell et al., 2017). For example, in the aftermath of Hurricane Katrina, the Chandeleur Islands lost approximately 70% of their land mass and 20% of the seagrass cover (Bethel and Martinez, 2008). In the wake of these disturbances, there has also been an observed shift in seagrass species composition, with increase in cover of R. maritima and decrease in cover of other species such as turtlegrass (Kenworthy et al., 2017). Large seed banks and rapid growth are characteristics that allow R. maritima to thrive after large disturbances and outcompete other seagrass species (Poirrier and Handley, 2007; Cho et al., 2009). As disturbances increase in frequency, as projected with impacts of climate change (Collins et al., 2019), it is likely that R. maritima will continue to increase in cover at the Chandeleur Islands, with unknown impacts to the seagrass-associated communities.
The goal of this study was to understand the distribution of R. maritima, describe the morphology of reproductive plants at the Chandeleur Islands, and quantify the impacts of reproductive and non-reproductive R. maritima on habitat association by fishes. Specific objectives are as follows:
Objective 1: Evaluate reproductive R. maritima plant homogeneity across the Chandeleur Islands.
Objective 2: Compare fish assemblages between reproductive and non-reproductive R. maritima.
Methods
Study area
The Chandeluer Island chain stretches 72 km, with the northern end located 35 km south of Biloxi, MS and the southern end lying in an arch 25 km northeast of Venice, LA (Poirrier and Handley, 2007). Much of the land mass of the Chandeleur Islands is in the northern islands, with North Chandeleur Island being the northernmost and largest island (Figure 2).
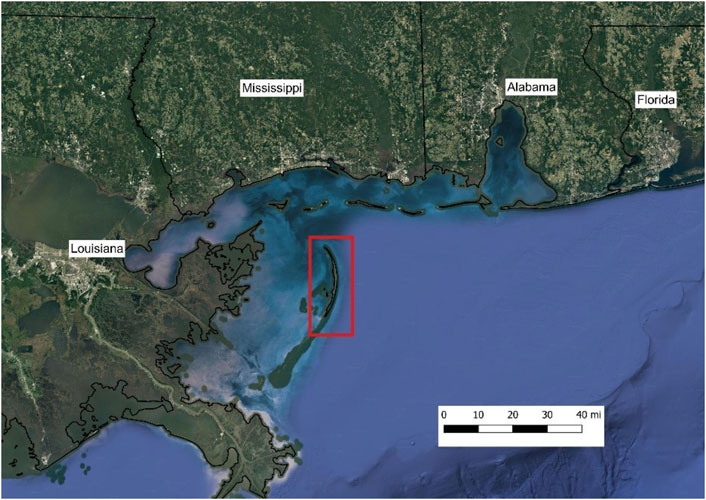
FIGURE 2. Map of the northern Gulf of Mexico with the North Chandeleur Island, LA study site bounded by the red box (Google Maps, 2021).
Objective 1: Evaluate reproductive R. maritima plant homogeneity across the Chandeleur Islands
To describe the distribution and morphology of reproductive and non-reproductive R. maritima at the Chandeleur Islands, LA, R. maritima was surveyed across its extent at the islands during late summer (August/September) 2021. Prior to sampling, sites with historic presence of reproductive and non-reproductive R. maritima were identified using seagrass monitoring data collected in previous years (K. Darnell, unpublished data). A total of 27 sites were chosen where R. maritima previously occurred, while ensuring sites were distributed across the entire area of seagrass occurrence at the Chandeleur Islands.
At each sampling site, a YSI handheld meter (Pro 2030; YSI Inc.) was used to measure bottom salinity, dissolved oxygen, and temperature; water depth was measured using a pole marked in 5 cm increments; and light irradiance at the surface and at depth was measured using two four-π (spherical quantum) sensors and a data logger (LI-1500, LI-Cor.). Light attenuation coefficients (kd) were calculated using the following equation:
where Iz is irradiance at depth, I0 is irradiance at the surface, and 0.58 represents the vertical distance (m) between the sensors.
At each site, percent cover of each seagrass species and bare sediment were quantified in three quadrats (50-cm × 50-cm). Additionally, the percent cover of reproductive and non-reproductive R. maritima was quantified. One seagrass core (9.5-cm diameter × 15-cm depth) was collected within R. maritima in each quadrat. Quadrats were only sampled at sites with seagrass present and cores were only collected from quadrats with seagrass cover. Cores were placed in plastic bags and transported to the Gulf Coast Research Lab (GCRL), United States where they were frozen until processing. Five reproductive and five non-reproductive plants were retained from the site for image analysis, with care taken to collect all aboveground (leaf and flower) and belowground (root and rhizome) tissue for each plant.
Seagrass cores were rinsed over a 500-μm sieve and plants were separated by species. The number of shoots of each species was counted, but only R. maritima plants were retained. The number of shoots, the reproductive status of each shoot, and the number of branching nodes per plant were recorded. Epiphytes were removed from leaves by gently scraping both sides of each leaf with a razor blade; epiphytes were then placed in a drying oven for 48 h at 60°C before being weighed to obtain a dry weight. Shoots were classified as reproductive or non-reproductive and separated. Reproductive shoots were defined as those with branching leaves and/or the presence of inflorescences. The aboveground and belowground tissues were separated, and the aboveground and belowground tissues for each shoot type (reproductive or non-reproductive) were grouped and placed in a drying oven for 48 h at 60°C, after which they were weighed to obtain a dry weight. Dried biomass was used to calculate a core-level root to shoot ratio (RSR), which is a useful proxy for plant condition and productivity, with higher values indicating greater biomass and energy in belowground structures (Hitchcock et al., 2017). Measurements from the 9-cm diameter core were then extrapolated to obtain bed characteristics per square meter.
Digital images (600 dpi) were taken of the handpicked plants using a flatbed scanner (Epson WF-3640). Prior to scanning, epiphytes were removed from each shoot and the aboveground and belowground tissues were separated. The aboveground biomass was scanned to produce a JPEG format image. Total leaf area was calculated using ImageJ (Version 1.53) with a threshold processing procedure (Easlon and Bloom, 2014). Additionally, total shoot length and the number of branching nodes were calculated from the image. Total leaf surface area, number of branching nodes, and shoot length were used to quantify overall plant complexity. Each individual shoot’s epiphyte, and aboveground and belowground biomass were dried separately in a drying oven for at least 48 h at 60°C, after which they were weighed to obtain dry weights. This information was used to calculate an individual plant RSR.
Sampling sites were mapped using QGIS (version 3.18.1) to display the spatial distribution of R. maritima along the Chandeleur Islands. Sites with reproductive R. maritima were also mapped to show the spatial distribution of reproductive plants. Each map was then evaluated for qualitative trends such as spatial clustering. The mean and standard error were calculated for abiotic parameters across all sites to characterize the distribution of site conditions across the study area.
Several metrics were selected to compare plant morphology between reproductive and non-reproductive shoots and included surface area, shoot length, leaf number, and RSR. Metrics were compared using unpaired two-sample t-tests (α = 0.05). Prior to testing each metric, the data were evaluated for violations of the parametric testing assumptions. If assumptions were violated the sample was bootstrapped with resampling and the test statistic calculated. This was repeated for 10,000 permutations and the reported test statistic was represented by the mean of bootstrapped test statistic distribution (R version 4.1.3).
Objective 2: Compare fish assemblages between reproductive and non-reproductive R. maritima
Fish were sampled at the Chandeleur Islands during a 6-day period from September 6–11, 2021, at the height of the R. maritima reproductive season in this area. This sampling time frame captures the greatest contrast in plant complexity between reproductive and non-reproductive plants. Fish were sampled using a throw trap at 22 sites along the island/Throw trapping provides a targeted approach to sampling patchy habitat that is not possible with other gears such as a benthic sled or trawl which cover larger areas (Jordan et al., 1997; Camp et al., 2011). The throw trap consisted of a 1-m × 1-m × 0.6-m high aluminum frame. Nylon mesh (3.175 mm) was sewn onto the frame sides as well as extended above the frame and attached to floats to extend the trap height to 1.5 m. Sampling was conducted in R. maritima patches across a range of reproductive plant coverage. Patches with desired characteristics (e.g., target cover of reproductive and non-reproductive plants) were identified from those sampled in the survey for plant distribution and morphology and marked with PVC poles prior to sampling. Sampling depth was limited to <1.5-m due to the height of the throw trap, and sites were separated by at least 15-m to minimize effects of disturbance. Thirty sites were selected to span a range of reproductive cover values.
After trap deployment, all vegetation within the throw trap was removed and retained for processing. A bar seine was used to remove organisms from within the trap. The bar seine (90-cm wide × 50-cm high) consisted of a PVC frame with handles and 3.175-mm mesh stretched between the handles. The pattern for seining organisms was modeled from Shakeri et al. (2020) and involved three sweeps from each side of the trap, with sweeps continuing until three consecutive sweeps yielded no additional fish. Collected fish were placed into plastic bags and stored on ice until returned to GCRL, where they were frozen until processing.
Seagrass collected from the throw trap was returned to GCRL and sorted for nekton, then the plant biomass was spun for 90 s using an industrial-sized salad spinner to remove excess water and sorted and weighed by species to obtain a species-specific wet weight (g). Individual seagrass species wet weights were then combined to get a total wet weight. Finally, a random subset of reproductive and non-reproductive R. maritima plants (at least 3 reproductive and 3 non-reproductive plants per throw trap sample) were imaged and dried as described above to quantify total leaf area and biomass.
Fish were identified to species using taxonomic keys and the number of individuals of each species was counted. All individuals were measured for standard length (SL, mm) (except for Dwarf Seahorses, which were only measured for total length), total length (TL, mm), and weighed. Fish assemblages were compared across sites using density, Shannon diversity, and species richness. Density was calculated as the number of individuals per square meter. Shannon diversity (H’) was calculated using standard methods (Freeman et al., 1984), and species richness was calculated as the number of unique species.
General Additive Models (GAM) were formulated to evaluate drivers of fish assemblage metrics (Shannon diversity, species richness, and density) with three potential predictor variables: water depth (cm), biomass of vegetation recovered from throw trap (g), and the proportion of reproductive R. maritima in the throw trap. Prior to running the GAMs, the predictor variables were plotted against each other to identify any significant relationships. Biomass of vegetation recovered from throw trap and the proportion of reproductive R. maritima in the throw trap were found to co-vary with a positive relationship. AIC scores were compared between models with biomass of vegetation recovered from throw trap and the proportion of reproductive R. maritima in the throw trap, and the final GAM formulations only included biomass of vegetation recovered from throw trap and depth as predictor variables. Poisson distributions were used to model species richness and density, while a Gaussian distribution was used for modeling Shannon diversity.
Results
Objective 1: Evaluate reproductive R. maritima plant homogeneity across the Chandeleur Islands
Across the 27 sites sampled between August 5 and 3 September 2021, mean (±SE) depth was 97.2 ± 7.60 cm, mean salinity was 18.97 ± 0.67 ppt, mean temperature was 30.8 ± 0.44°C, mean dissolved oxygen was 7.82 ± 0.52 mg/L, and mean light attenuation coefficient was 1.08 ± 0.06 m−1. Abiotic parameters were within the known ranges for seagrass meadows at the Chandeleur Islands (Darnell et al., 2017; Hayes, 2021).
Of the sites sampled, 24 (88.9%) had R. maritima present, and 17 of the sites (63.0%) had reproductive plants (Figure 3). Among 72 quadrats sampled across the 27 sites, mean (±SE) percent cover of non-reproductive R. maritima was 44.5 ± 3.5%, mean percent cover of reproductive R. maritima 21.5 ± 3.2%, and mean canopy height was 216.8 ± 6.3 mm. Sites with reproductive plants were primarily located near the latitudinal center of the islands, with sites near the northern and southern limits of the islands dominated by non-reproductive plants (Figure 3).
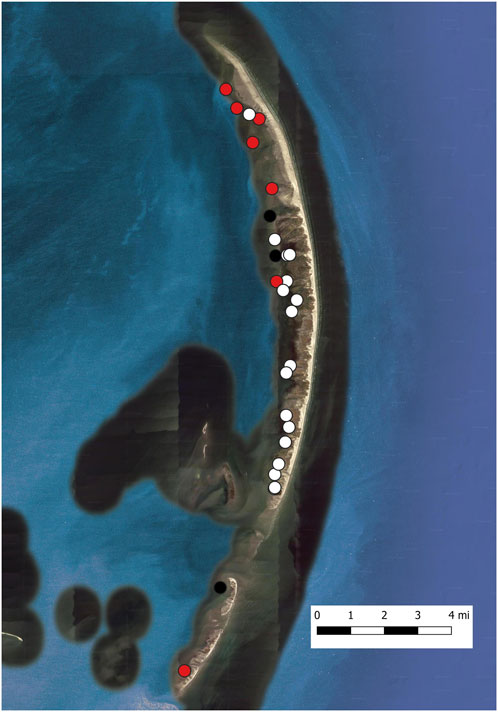
FIGURE 3. Presence of reproductive R. maritima at the Chandeleur Islands, LA during August and September 2021. White symbols indicate sampling stations with reproductive R. maritima present, while red symbols indicate stations where only non-reproductive R. maritima was present. Black symbols indicate stations where R. maritima was completely absent.
A total of 65 cores were collected across the sites with R. maritima present. Mean (±SE) overall shoot density was 3,866.71 ± 293.01 shoots per m2, mean non-reproductive R. maritima shoot density was 3,449.39 ± 268.69 shoots per m2, mean reproductive R. maritima shoot density was 804.15 ± 174.32 shoots per m2, and mean core RSR was 1.097 ± 0.111. Within the cores, the density of non-reproductive shoots was more than four times greater than the density of reproductive shoots (t = 8.2589, p < 0.05). Out of the 65 cores, only 19 cores had measurable epiphyte cover (0.068 ± 0.041 g for all cores combined). Mean epiphyte biomass for cores with only non-reproductive shoots (n = 10) was 0.098 ± 0.075 g and epiphyte biomass for cores containing both non-reproductive and reproductive shoots (n = 9) was 0.035 ± 0.026 g.
A total of 74 reproductive and 112 non-reproductive plants were hand-collected to assess differences in plant morphology. Reproductive plants had significantly longer leaves (t = 7.054, p < 0.05), greater total surface area (t = 11.509, p < 0.05), and a greater number of leaves (t = 15.005, p < 0.05), while non-reproductive plants had a higher RSR (t = 10.440, p < 0.05), indicating a larger contribution of belowground biomass (Table 1).
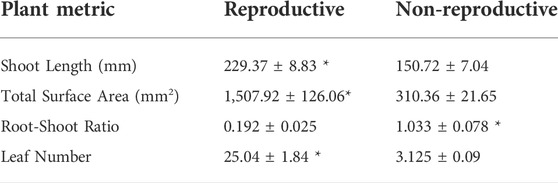
TABLE 1. Mean (±SE) morphological metrics for individual reproductive and non-reproductive hand-collected R. maritima plants. Values with “*” indicate that the value is significantly greater (p < 0.05) than the compared value.
Objective 2: Compare fish assemblages between reproductive and non-reproductive R. maritima
A total of 22 sites were sampled to investigate habitat use by fishes. Sites were predominant located near the center of the island chain where most reproductive R. maritima plants were observed (Figure 4). Mean (±SE) depth was 71.6 ± 2.8 cm, salinity was 19.46 ± 0.37 ppt, temperature was 28.81 ± 8.64°C, and dissolved oxygen was 8.15 ± 0.02 mg/L. Mean (±SE) total percent cover of seagrass was 69.1 ± 2.9%, percent cover of non-reproductive R. maritima was 38.9 ± 5.2%, percent cover of reproductive R. maritima was 30.2 ± 4.3% and total R. maritima wet biomass was 371.58 ± 27.61 g per m2.
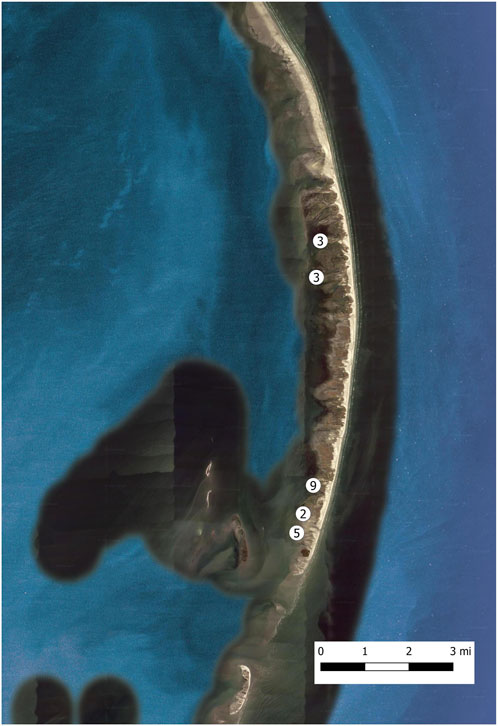
FIGURE 4. Sites sampled for fish assemblages within R. maritima during September 2021. Each white circle represents a general sampling area, with the number inside the white circle indicating the number of samples taken in that area.
Across all sites, a total of 224 individual fish were collected across 15 species. Mean (±SE) fish density was 10.2 ± 1.7 per m2, species richness was 3.4 ± 0.2, and Shannon diversity was 0.97 ± 0.07. Fish density showed no relationship with the proportion of reproductive R. maritima (Figure 5). The five most abundant species accounted for 86.6% of all individuals collected and included Darter Goby (n = 107), Blackcheek Tonguefish (n = 33), Gulf Pipefish (n = 23), Rough Silverside (n = 20), and Code Goby (n = 11). Mean weights, total lengths, and standard lengths for all species are listed in Table 2.
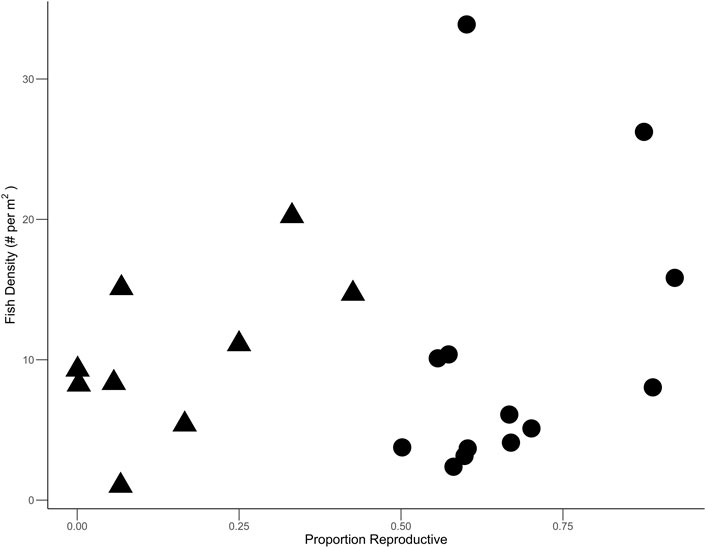
FIGURE 5. Fish density per meter square plotted against the proportion of R. maritima cover that was reproductive. Triangles indicate sites that were majority non reproductive cover while circles are those with majority reproductive cover.
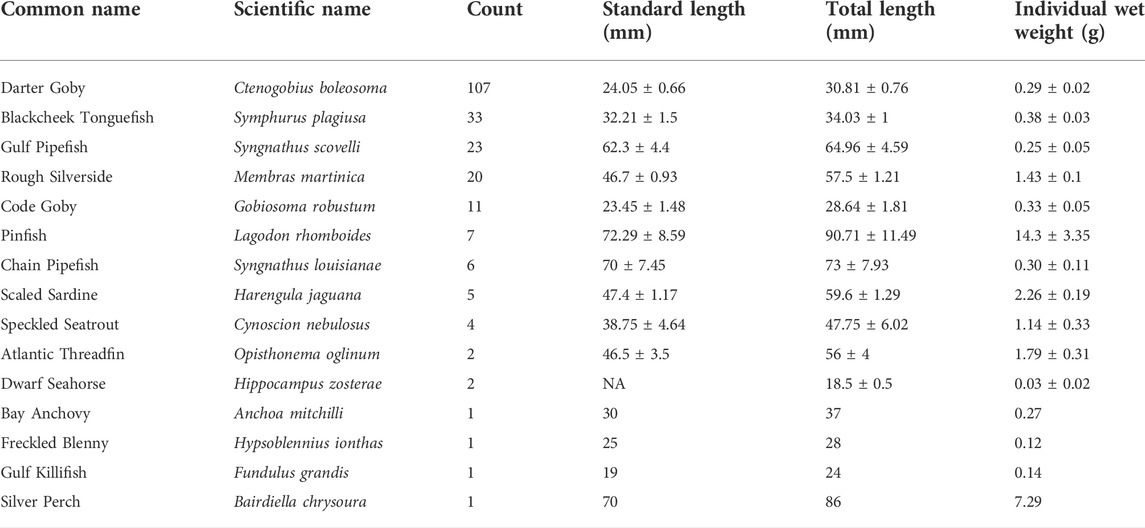
TABLE 2. Count and mean (±SE) morphological metrics for fish collected, NA values indicate data not collected or not applicable for metric.
Three GAMs were formulated to identify potential drivers of the fish assemblage metrics of density, species richness, and Shannon diversity. The first model with total number of fish as the response variable identified the wet biomass R. maritima of vegetation recovered from throw trap (χ2 = 4.506, p < 0.05) as the only significant predictor variable with a positive relationship (Table 3A), and the second and third models identified no significant predictor variables for species richness and Shannon diversity (Tables 3B, C).
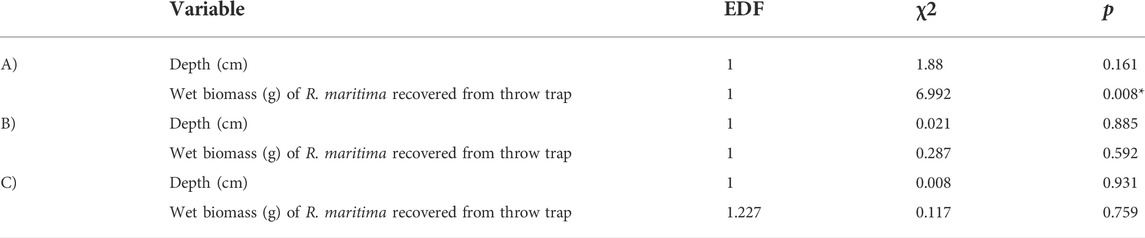
TABLE 3. General additive model outputs for models with dependent variables A) fish density, B) species richness, and C) Shannon diversity. Values with “*” indicate a significant relationship (p < 0.05) with the predictor variable.
Discussion
This study described the spatial distribution of reproductive R. maritima across the Chandeleur Islands and compared seagrass-associated fish assemblages between reproductive and non-reproductive plants. We conducted extensive seagrass surveys along the length of the Chandeleur Islands and used throw trapping to describe habitat associations by fish, and found that reproductive and non-reproductive plants displayed distinct morphologies, but the morphology of reproductive plants did not significantly impact species richness or Shannon diversity. The more robust measurement of total wet biomass of R. maritima recovered from the throw trap, however, was related to fish density, with more fish associated with greater plant biomass.
Ruppia maritma occurred along the entire distribution of seagrass at North Chandeleur Island. Given the robustness of R. maritima to environmental stress, the absence of the species at only three of the sampled sites is likely linked to localized physical disturbance (e.g., wave action). The only other species present at the stations sampled was turtlegrass (Thalassia testudinum). Turtlegrass is a climax species that requires relatively stable environmental conditions and low levels of physical disturbance (Hartog and Kuo, 2006). Turtlegrass occurred in centralized portions of North Chandeleur Island that likely represent areas with relatively more stable environmental and physical conditions than the northern and southern tips of the island.
Similar to turtlegrass, reproductive R. maritima plants were primarily restricted to the center of the island’s back shelf. The complex reproductive shoots have reduced structural tissue and rely on support from the surrounding water (Kantrud, 1997), making them susceptible to physical disturbances such as wave action. The central portion of the island represents an area with increased sheltering from wind driven wave action that dominates the system (Moore et al., 2014). The most northern and southern portions of the islands are characterized by reduced island elevation and more exposed shallow waters (Kahn, 1986; Miselis and Plant, 2021). It is possible that R. maritima is reproductive at the northern and southern tips of North Chandeleur island, but that shoots cannot sustain the physical disturbance and are dislodged, suggesting that the environmental requirements for sexually reproductive R. maritima are stricter than those of non-reproductive plants (de los Santos et al., 2016; Koch et al., 2006).
Core samples showed distinct differences between reproductive and non-reproductive shoot densities, with about one fourth of the shoots being reproductive. The production of flowers, fruits, and seeds is energetically costly, and as a result, the number of plants undergoing reproduction varies across space and time, which can lead to not all plants undergoing sexual reproduction (e.g., Bigley and Harrison, 1986; Strazisar et al., 2015; von Staats et al., 2021).
R. maritima exhibits morphological plasticity across its range in distribution (Cho et al., 2009; Lopez-Calderon et al., 2010; Ito et al., 2013; Martínez-Garrido et al., 2017), and the current study represents the first known description of R. maritima reproductive shoot morphology at the Chandeleur Islands. Results from this study of increased surface area, aboveground biomass and leaf number for reproductive shoots relative to non-reproductive shoots are consistent with descriptions for the species (Bigley and Harrison, 1986; Cho and Poirrier, 2005). In this study, the mean shoot length for reproductive shoots was 229.37 mm, which is much shorter than the maximum described shoot length of 2.5 m (Hartog and Kuo, 2006). The observed difference may be genetic, linked to environmental drivers such as salinity, and/or the presence of physical disturbances that limit the length of the fragile reproductive stems (Richardson, 1983). While plasticity in reproductive output for R. maritima is not well understood, studies of other species report increased reproductive effort for plants in more physically disturbed environments (Lee et al., 2005; Mishra and Apte, 2020). Epiphyte cover on seagrasses at the Chandeleur Islands can be high (Hayes 2021); however, among the cores collected for this study, less than one third (29.2%) showed measurable levels of epiphyte cover. This may be due to the sloughing off of older leaves and/or rapid growth of plants with the onset of reproductive plants which may not provide sufficient time for epiphytes to colonize to a measurable amount.
This study describes potential drivers of habitat use by fish in reproductive and non-reproductive R. maritima and suggests that total plant biomass rather than plant morphology most influences fish density in R. maritima meadows, despite the distinct morphological differences between reproductive and non-reproductive plants. These results indicate that the increase in biomass that occurs at the onset of reproduction is largely driving the relationship between fish density and proportion of R. maritima cover that was reproductive (Figure 5). Similar relationships have been described for nekton communities of R. maritima in brackish ponds in Louisiana (Kanouse et al., 2006), but our findings are contrary to studies with other seagrass species (e.g., Halophila ovalis, Halophila beccarii, Amphibolis griffithii, Posidonia sinuosa, Posidonia australis, Zostera capricorni) where interspecific differences in plant morphology/complexity have variable impacts on fish assemblages (Rotherham and West, 2002; Hyndes et al., 2003; Hori et al., 2009). GAMs for species richness and Shannon diversity showed that no predictor variables significantly affected richness or diversity, further suggesting that influence of the morphology of the reproductive plants is marginal when compared to the overall influence of seagrass presence for shaping the fish community. This conclusion aligns with previous studies investigating relationships between seagrass presence and biomass and animal abundance, diversity, and richness (Wyda et al., 2002; Heck et al., 2003; Strayer et al., 2003).
The lack of significant predictors among GAMs for species richness and Shannon diversity implies that other predictors are driving the relationships. One possible driver may be prey availability, which is linked to the function of seagrass as foraging grounds (K. Heck et al., 2003; Heck et al., 1997). Invertebrates were collected during the present study but were found to be outside the size range of those consumed by the fishes collected. Future studies designed to collect infauna and smaller invertebrates could help elucidate the relationship between prey availability and habitat use. Additionally, other environmental drivers such as salinity (Matheson et al., 1999), turbidity (Blaber and Blaber, 1980) and the availability of dissolved nutrients (Deegan et al., 2002) may impact the distribution of fishes within seagrass habitats due to physiological requirements of individual species. Although salinity was measured in the present study, there was little variation in the measured values which limited power in analyses.
The fish collected within R. maritima meadows in this study were predominately small benthic species with individuals under 100-mm total length. The majority of individuals were adults of their respective species, with notable exceptions being spotted seatrout (Cynoscion nebulosus) and pinfish (Lagodon rhomboides) which were primarily young-of-year individuals (FishBase, 2022). The family Gobiidae made up the majority (52.7%) of all species collected. Gobiidae are benthic-associated fish and are known to live within seagrass meadows during all life stages where they feed on meiofaunal prey and use the structure provided by seagrasses as shelter from predators (Carle and Hastings, 1982; Ara et al., 2010). Although adult pinfish are known to be abundant in seagrass beds at the Chandeleur Islands (e.g., Hayes 2021), they were collected in low numbers during this study. This may be due to the life history characteristics of the species, where adults leave seagrass beds between May and October to spawn (Faletti et al., 2019), which could have led to a decrease in abundance within seagrass beds at the time of this study (September).
Interestingly, the fish collected in this study included no tropical species. Recent studies have reported the occurrence of tropical fishes in Chandeleur Islands seagrass meadows, indicating tropicalization, or a northward movement species to this area (Fodrie et al., 2010; Hayes, 2021). The previous studies collected relatively low abundances of tropical species (e.g., Lane Snapper (Lutjanus synagris) 0.056 per m2 and Gag Grouper (Mycteroperca microlepis) 0.002 per m2 (Hayes, 2021)), and these were sampled using trawls and benthic sleds which cover a greater area than the throw trap. The absence of tropical species in our small-scale (1 m2) samples suggests that, although they may be present in seagrass meadows at the Chandeleur Islands, tropical species are not likely abundant in R. maritima meadows.
One potential limitation of the current study is the throw trap sampling method which may underrepresent the abundance of highly mobile species (Kushlan, 1981; Freeman et al., 1984) such as members of family Mugilidae. Despite this limitation, throw traps are known to have high rates of accuracy when describing fish assemblages (Jordan et al., 1997). Additionally, the removal of all vegetation within the throw trap contributed to our clearing efficiency, as animals were recovered from the collected plant material during processing.
Conclusion
R. maritima occurred along the entire latitudinal range of seagrass distribution at North Chandeleur Island. Despite the widespread distribution of non-reproductive plants, there was a tendency for reproductive plants to be located along the central portion of the island. Where reproductive plants occurred, their density was lower than that of non-reproductive shoots, but reproductive shoots had significantly greater shoot lengths, number of leaves, and surface area. Non-reproductive plants showed significantly greater RSR, likely due to the substantial increase in aboveground biomass with the onset of reproduction for flowering plants. These results of distinct spatial and morphological characteristics for reproductive R. maritima plants suggest that the ecosystem functions of R. maritima may change with the onset of reproduction.
Fish assemblages within R. maritima beds at the Chandeleur Islands are represented by an abundant group of small benthic species. Total seagrass biomass was the primary driver of habitat use, suggesting that future studies to investigate the role of R. maritima in structuring fish communities in similar study systems may be better served to focus effort on robust measures of seagrass complexity (e.g., biomass) rather than more laborious measures of plant complexity such as cores and image analyses. Assitionally, future studies designed to collect infauna and smaller invertebrates could help elucidate the relationship between prey availability and habitat use.
At the Chandeleur Islands, R. maritima has increased in cover by colonizing disturbed areas along the islands and displacing climax species such as T. testudinum. Future studies should also compare R. maritima to other co-occurring seagrass species to better understand possible functional differences for associated fishes.
Data availability statement
The raw data supporting the conclusions of this article will be made available by the authors, without undue reservation.
Ethics statement
The animal study was reviewed and approved by The University of Southern Mississippi Institutional Animal Care and Use Committee.
Author contributions
All authors listed have made a substantial, direct, and intellectual contribution to the work and approved it for publication.
Conflict of interest
The authors declare that the research was conducted in the absence of any commercial or financial relationships that could be construed as a potential conflict of interest.
Publisher’s note
All claims expressed in this article are solely those of the authors and do not necessarily represent those of their affiliated organizations, or those of the publisher, the editors and the reviewers. Any product that may be evaluated in this article, or claim that may be made by its manufacturer, is not guaranteed or endorsed by the publisher.
References
Ara, R., Arshad, A., Amin, S. M. N., Daud, S. K., Bujang, J. S., and Ghaffar, M. A. (2010). Feeding habits of larval fishes of the family Gobiidae (Actinopterygii: Perciformes) in seagrass beds of Sungai Pulai estuary, 7. Malaysia: Johor Strait.
Belgrad, B. A., Correia, K. M., Darnell, K. M., Darnell, M. Z., Hayes, C. T., Hall, M. O., et al. (2021). Environmental drivers of seagrass-associated nekton abundance across the northern Gulf of Mexico. Estuaries Coasts 44 (8), 2279–2290. doi:10.1007/s12237-021-00927-0
Bertelli, C. M., and Unsworth, R. K. F. (2014). Protecting the hand that feeds us: Seagrass (Zostera marina) serves as commercial juvenile fish habitat. Mar. Pollut. Bull. 83 (2), 425–429. doi:10.1016/j.marpolbul.2013.08.011
Bethel, M., and Martinez, L. (2008). Assessment of Current Seagrass Critical Habitat in Response to Dramatic Shoreline Change Resulting from the 2005 Hurricane Season for the Chandeleur Islands, 12.
Bigley, R. E., and Harrison, P. G. (1986). Shoot demography and morphology of Zostera japonica and Ruppia maritima from British Columbia, Canada. Aquat. Bot. 24 (1), 69–82. doi:10.1016/0304-3770(86)90118-X
Blaber, S. J. M., and Blaber, T. G. (1980). Factors affecting the distribution of juvenile estuarine and inshore fish. J. Fish. Biol. 17 (2), 143–162. doi:10.1111/j.1095-8649.1980.tb02749.x
Camp, E. V., Gwinn, D. C., Lauretta, M. V., Pine, W. E., and Frazer, T. K. (2011). Use of recovery probabilities can improve sampling efficiency for throw traps in vegetated habitats. Trans. Am. Fish. Soc. 140 (1), 164–169. doi:10.1080/00028487.2011.558778
Carle, K. J., and Hastings, P. A. (1982). Selection of meiofaunal prey by the darter Goby, gobionellus boleosoma (Gobiidae). Estuaries 5 (4), 316–318. doi:10.2307/1351755
Castillo-Rivera, M., Zavala-Hurtado, J. A., and Zárate, R. (2002). Exploration of spatial and temporal patterns of fish diversity and composition in a tropical estuarine system of Mexico. Rev. Fish. Biol. Fish. 12, 167–177. doi:10.1023/A:1025051027676
Cho, H. J., Biber, P., and Nica, C. (2009). THE RISE OF RUPPIA IN SEAGRASS BEDS: CHANGES IN COASTAL ENVIRONMENT AND RESEARCH NEEDS, 15.
Cho, H. J., and Poirrier, M. A. (2005). Seasonal growth and reproduction of Ruppia maritima L. s.l. In Lake Pontchartrain, Louisiana, USA. Aquat. Bot. 81, 37–49. doi:10.1016/j.aquabot.2004.10.002
Collins, M., Sutherland, M., Bouwer, L., Cheong, S.-M., Combes, H. J. D., Roxy, M. K., et al. (2019). IPCC Special Report on the Ocean and Cryosphere in a Changing Climate, 68.Extremes, abrupt changes and managing risk.
Darnell, K. M., Carruthers, T. J. B., Biber, P., Georgiou, I. Y., Michot, T. C., and Boustany, R. G. (2017). Spatial and temporal patterns in Thalassia testudinum leaf tissue nutrients at the Chandeleur islands, Louisiana, USA. Estuaries Coasts 40 (5), 1288–1300. doi:10.1007/s12237-017-0229-y
Darnell, K. M., Furman, B. T., Heck, K. L., Byron, D., Reynolds, L., and Dunton, K. H. (2021). Seed reserve hot spots for the sub-tropical seagrass halodule wrightii (shoal grass) in the northern Gulf of Mexico. Estuaries Coasts 44 (2), 339–351. doi:10.1007/s12237-020-00808-y
Deegan, L. A., Wright, A., Ayvazian, S. G., Finn, J. T., Golden, H., Merson, R. R., et al. (2002). Nitrogen loading alters seagrass ecosystem structure and support of higher trophic levels. Aquat. Conserv. 12 (2), 193–212. doi:10.1002/aqc.490
de los Santos, C., Onoda, Y., Vergara, J., Pérez-Lloréns, J., Bouma, T., La Nafie, Y., et al. (2016). A comprehensive analysis of mechanical and morphological traits in temperate and tropical seagrass species. Mar. Ecol. Prog. Ser. 551, 81–94. doi:10.3354/meps11717
Easlon, H. M., and Bloom, A. J. (2014). Easy Leaf Area: Automated digital image analysis for rapid and accurate measurement of leaf area. Appl. Plant Sci. 2 (7). doi:10.3732/apps.1400033
Ellinwood, M. C. (2008). Response of barrier island fish assemblages to impacts from multiple hurricanes: Assessing resilience of Chandeleur Island fish assemblages to hurricanes Ivan (2004) and Katrina (2005), 140.
Faletti, M. E., Chacin, D. H., Peake, J. A., MacDonald, T. C., and Stallings, C. D. (2019). Population dynamics of pinfish in the eastern Gulf of Mexico (1998-2016). PLOS ONE 14 (8), e0221131. doi:10.1371/journal.pone.0221131
FishBase (2022). FishBase. Retrieved Available at: https://www.fishbase.se/search.php January 19, 2022).
Fodrie, F. J., Heck, K. L., Powers, S. P., Graham, W. M., and Robinson, K. L. (2010). Climate-related, decadal-scale assemblage changes of seagrass-associated fishes in the northern Gulf of Mexico. Glob. Chang. Biol. 16 (1), 48–59. doi:10.1111/j.1365-2486.2009.01889.x
Fourqurean, J. W., Boyer, J. N., Durako, M. J., Hefty, L. N., and Peterson, B. J. (2003). Forecasting responses of seagrass distributions to changing water quality using monitoring data. Ecol. Appl. 13 (2), 474–489. doi:10.1890/1051-0761(2003)013[0474:FROSDT]2.0.CO;2
Freeman, B. J., Greening, H. S., and Oliver, J. D. (1984). Comparison of three methods for sampling fishes and macroinvertebrates in a vegetated freshwater wetland. J. Freshw. Ecol. 2 (6), 603–609. doi:10.1080/02705060.1984.9664643
Google Maps (2021). Google Maps. Available at: https://www.google.com/maps?hl=en&tab=rl.
Handley, L., and Lockwood, C. (2020). 2020 seagrass status and trends update. Available at: https://cnlworld-my.sharepoint.com/personal/handleyn_cnlworld_org/_layouts.
Hartog, C., and Kuo, J. (2006). “Taxonomy and biogeography of seagrasses,” in Seagrasses: BIOLOGY, ecologyand conservation. Editors A. W. D. LARKUM, R. J. ORTH, and C. M. DUARTE (Springer Netherlands), 1–23. doi:10.1007/978-1-4020-2983-7_1
Hayes, C. (2021). Patterns of Habitat Use and Trophic Structure in Turtle Grass (Thalassia testudinum)-Dominated Systems Across the Northern Gulf of Mexico, 283.
Heck, K., Hays, G., and Orth, R. (2003). Critical evaluation of the nursery role hypothesis for seagrass meadows. Mar. Ecol. Prog. Ser. 253, 123–136. doi:10.3354/meps253123
Heck, K. L., Nadeau, D. A., and Thomas, R. (1997). The nursery role of seagrass beds. Gulf Mex. Sci. 15 (1). doi:10.18785/goms.1501.08
Hitchcock, J. K., Courtenay, S. C., Coffin, M. R. S., Pater, C. C., and van den Heuvel, M. R. (2017). Eelgrass bed structure, leaf nutrient, and leaf isotope responses to natural and anthropogenic gradients in estuaries of the southern Gulf of st. Lawrence, Canada. Estuaries Coasts 40 (6), 1653–1665. doi:10.1007/s12237-017-0243-0
Hori, M., Suzuki, T., Monthum, Y., Srisombat, T., Tanaka, Y., Nakaoka, M., et al. (2009). High seagrass diversity and canopy-height increase associated fish diversity and abundance. Mar. Biol. 156 (7), 1447–1458. doi:10.1007/s00227-009-1184-3
Hyndes, G. A., Kendrick, A. J., MacArthur, L. D., and Stewart, E. (2003). Differences in the species- and size-composition of fish assemblages in three distinct seagrass habitats with differing plant and meadow structure. Mar. Biol. 142 (6), 1195–1206. doi:10.1007/s00227-003-1010-2
Hyndes, G. A., Potter, I. C., and Lenanton, R. C. J. (1996). Habitat partitioning by whiting species (Sillaginidae) in coastal waters. Environ. Biol. Fishes 45 (1), 21–40. doi:10.1007/BF00000625
Ito, Y., Ohi-Toma, T., Murata, J., and Tanaka, N. (2013). Comprehensive phylogenetic analyses of the Ruppia maritima complex focusing on taxa from the Mediterranean. J. Plant Res. 126 (6), 753–762. doi:10.1007/s10265-013-0570-6
Jordan, F., Coyne, S., and Trexler, J. C. (1997). Sampling fishes in vegetated habitats: Effects of habitat structure on sampling characteristics of the 1-m2 throw trap. Trans. Am. Fish. Soc. 126 (6), 1012–1020. doi:10.1577/1548-8659(1997)126<1012:SFIVHE>2.3.CO;2
Kahn, J. H. (1986). Geomorphic recovery of the Chandeleur islands, Louisiana, after a major hurricane. J. Coast. Res. 2 (3), 337–344.
Kanouse, S., La Peyre, M., and Nyman, J. (2006). Nekton use of Ruppia maritima and non-vegetated bottom habitat types within brackish marsh ponds. Mar. Ecol. Prog. Ser. 327, 61–69. doi:10.3354/meps327061
Kantrud, H. A. (1997). Wigeongrass (Ruppia maritima): A literature review. Washington, DC. Available at: https://agris.fao.org/agris-search/search.do?recordID=AV20120104488.
Kenworthy, W. J., Cosentino-Manning, N., Handley, L., Wild, M., and Rouhani, S. (2017). Seagrass response following exposure to deepwater Horizon oil in the Chandeleur islands, Louisiana (USA). Mar. Ecol. Prog. Ser. 576, 145–161. doi:10.3354/meps11983
Kiggins, R. S., Knott, N. A., New, T., and Davis, A. R. (2019). Fish assemblages in protected seagrass habitats: Assessing fish abundance and diversity in no-take marine reserves and fished areas. Aquac. Fish. 5, 213. doi:10.1016/j.aaf.2019.10.004
Kilminster, K., McMahon, K., Waycott, M., Kendrick, G. A., Scanes, P., McKenzie, L., et al. (2015). Unravelling complexity in seagrass systems for management: Australia as a microcosm. Sci. Total Environ. 534, 97–109. doi:10.1016/j.scitotenv.2015.04.061
Koch, E. W., Sanford, L. P., Chen, S.-N., Shafer, D. J., and Smith, J. M. (2006). Waves in seagrass systems: Review and technical recommendations. Def. Tech. Inf. Cent. doi:10.21236/ADA458760
Kushlan, J. (1981). Sampling characteristics of enclosure fish traps. Trans. Am. Fish. Soc. - TRANS AMER FISH SOC 110, 557–562. doi:10.1577/1548-8659(1981)110<557:SCOEFT>2.0.CO;2
Lee, S., Choi, C., Suh, Y., and Mukai, H. (2005). Seasonal variation in morphology, growth and reproduction of Zostera caespitosa on the southern coast of Korea. Aquat. Bot. 83, 250–262. doi:10.1016/j.aquabot.2005.03.003
Lopez-Calderon, J., Riosmena-Rodríguez, R., Rodríguez-Baron, J. M., Carrión-Cortez, J., Torre, J., Meling-López, A., et al. (2010). Outstanding appearance of Ruppia maritima along Baja California Sur, México and its influence in trophic networks. Mar. Biodivers. 40 (4), 293–300. doi:10.1007/s12526-010-0050-3
Malea, P., Kevrekidis, T., and Mogias, A. (2004). Annual versus perennial growth cycle in Ruppia maritima L.: Temporal variation in population characteristics in mediterranean lagoons (monolimni and drana lagoons, northern aegean sea). Bot. Mar. 47, 357–366. doi:10.1515/BOT.2004.052
Martínez-Garrido, J., Creed, J. C., Martins, S., Almada, C. H., and Serrão, E. A. (2017). First record of Ruppia maritima in West Africa supported by morphological description and phylogenetic classification. Bot. Mar. 60 (5), 583–589. doi:10.1515/bot-2016-0128
Matheson, R. E., Camp, D. K., Sogard, S. M., and Bjorgo, K. A. (1999). Changes in seagrass-associated fish and Crustacean communities on Florida bay mud banks: The effects of recent ecosystem changes? Estuaries 22 (2), 534. doi:10.2307/1353216
McDevitt-Irwin, J., Iacarella, J., and Baum, J. (2016). Reassessing the nursery role of seagrass habitats from temperate to tropical regions: A meta-analysis. Mar. Ecol. Prog. Ser. 557, 133–143. doi:10.3354/meps11848
McKenzie, L. J., Yaakub, S. M., Tan, R., Seymour, J., and Yoshida, R. L. (2016). Seagrass habitats of Singapore: Environmental drivers and key processes. RAFFLES Bull. ZOOLOGY 18, 60–70.
Miselis, J. L., and Plant, N. G. (2021). Satellite-derived barrier response and recovery following natural and anthropogenic perturbations, northern Chandeleur islands, Louisiana. Remote Sens. 13 (18), 3779. doi:10.3390/rs13183779
Mishra, A. K., and Apte, D. (2020). Reproductive effort of intertidal tropical seagrass as an indicator of habitat disturbance [Preprint]. Ecology. doi:10.1101/2020.07.19.177899
Moore, L. J., Patsch, K., List, J. H., and Williams, S. J. (2014). The potential for sea-level-rise-induced barrier island loss: Insights from the Chandeleur Islands, Louisiana, USA. Mar. Geol. 355, 244–259. doi:10.1016/j.margeo.2014.05.022
Nakaoka, M. (2005). Plant–animal interactions in seagrass beds: Ongoing and future challenges for understanding population and community dynamics. Popul. Ecol. 47 (3), 167–177. doi:10.1007/s10144-005-0226-z
Nordlund, L. M., Unsworth, R. K. F., Gullström, M., and Cullen‐Unsworth, L. C. (2018). Global significance of seagrass fishery activity. Fish. Fish. (Oxf). 19 (3), 399–412. doi:10.1111/faf.12259
Olesen, B., Marba, N., Duarte, C. M., Savela, R. S., and Fortes, M. D. (2004). Recolonization dynamics in a mixed seagrass meadow: The role of clonal versus sexual processes. Estuaries 27 (5), 770–780. doi:10.1007/BF02912039
Orth, R. J., Harwell, M. C., and Inglis, G. J. (2006). “Ecology of seagrass seeds and seagrass dispersal processes,” in Seagrasses: Biology, ecologyand conservation. Editors A. W. D. Larkum, R. J. Orth, and C. M. Duarte (Springer Netherlands), 111–133. doi:10.1007/978-1-4020-2983-7_5
Orth, R. J., and Moore, K. A. (1988). Distribution of Zostera marina L. and Ruppia maritima L. sensu lato along depth gradients in the lower Chesapeake Bay, U.S.A. Aquat. Bot. 32 (3), 291–305. doi:10.1016/0304-3770(88)90122-2
Pham, L. T., Biber, P. D., and Carter, G. A. (2014). Seagrasses in the Mississippi and Chandeleur Sounds and Problems Associated with Decadal-Scale Change Detection. Gulf Mex. Sci. 32 (1). doi:10.18785/goms.3201.03
Pulich, W. M. (1985). Seasonal growth dynamics of Ruppia maritima L. S.1, And Halodule wrightii aschers in southern texas and evaluation of sediment fertility status. Aquat. Bot. 23, 14. doi:10.1016/0304-3770(85)90020-8
Reyes, E., and Merino, M. (1991). Diel Dissolved Oxygen Dynamics and Eutrophication in a Shallow, Well-Mixed Tropical Lagoon (Cancun, Mexico). Estuaries 14 (4), 372–381. doi:10.2307/1352262
Richardson, F. (1983). Variation, adaptation and reproductive biology in Ruppia maritima L. Populations from New Hampshire coastal and estuarine tidal marshes. Ph.D. Dissertation. Ann Arbor: University of New Hampshire. Available at: https://www.proquest.com/docview/303170779/abstract/1DFCCCF60C204E5BPQ/1.
Rotherham, D., and West, R. J. (2002). Do different seagrass species support distinct fish communities in south-eastern Australia?: Seagrass FISH communities. Fish. Manag. Ecol. 9 (4), 235–248. doi:10.1046/j.1365-2400.2002.00301.x
Shakeri, L. M., Darnell, K. M., Carruthers, T. J. B., and Darnell, M. Z. (2020). Blue crab abundance and survival in a fragmenting coastal marsh system. Estuaries Coasts 43 (6), 1545–1555. doi:10.1007/s12237-020-00738-9
Strayer, D. L., Lutz, C., Malcom, H. M., Munger, K., and Shaw, W. H. (2003). Invertebrate communities associated with a native (Vallisneria americana) and an alien (Trapa natans) macrophyte in a large river. Freshw. Biol. 48 (11), 1938–1949. doi:10.1046/j.1365-2427.2003.01142.x
Strazisar, T., Koch, M. S., and Madden, C. J. (2015). Seagrass (Ruppia maritima L.) Life History Transitions in Response to Salinity Dynamics Along the Everglades-Florida Bay Ecotone. Estuaries Coasts 38 (1), 337–352. doi:10.1007/s12237-014-9807-4
Vaslet, A., Phillips, D. L., France, C., Feller, I. C., and Baldwin, C. C. (2012). The relative importance of mangroves and seagrass beds as feeding areas for resident and transient fishes among different mangrove habitats in Florida and Belize: Evidence from dietary and stable-isotope analyses. J. Exp. Mar. Biol. Ecol. 434 (435), 81–93. doi:10.1016/j.jembe.2012.07.024
von Staats, D. A., Hanley, T. C., Hays, C. G., Madden, S. R., Sotka, E. E., and Hughes, A. R. (2021). Intra-Meadow Variation in Seagrass Flowering Phenology Across Depths. Estuaries Coasts 44 (2), 325–338. doi:10.1007/s12237-020-00814-0
Keywords: seagrass, Chandeleur Islands, Ruppia maritima, seagrass reproduction, fish assemblage
Citation: Byrnes MA, Darnell KM and Darnell MZ (2022) Changes in the morphology of widgeon grass (Ruppia maritima) with the onset of reproduction and impacts on fish assemblages at the Chandeleur Islands, LA. Front. Environ. Sci. 10:978772. doi: 10.3389/fenvs.2022.978772
Received: 26 June 2022; Accepted: 30 August 2022;
Published: 16 November 2022.
Edited by:
Paola Lopez-Duarte, University of North Carolina at Charlotte, United StatesReviewed by:
Binsong Jin, Hangzhou Normal University, ChinaAmrit Kumar Mishra, The University of Hong Kong, Hong Kong SAR, China
Richard K. F. Unsworth, Swansea University, United Kingdom
Copyright © 2022 Byrnes, Darnell and Darnell. This is an open-access article distributed under the terms of the Creative Commons Attribution License (CC BY). The use, distribution or reproduction in other forums is permitted, provided the original author(s) and the copyright owner(s) are credited and that the original publication in this journal is cited, in accordance with accepted academic practice. No use, distribution or reproduction is permitted which does not comply with these terms.
*Correspondence: Kelly M. Darnell, a2VsbHkuZGFybmVsbEB1c20uZWR1