- 1LIEC, CNRS, Université de Lorraine, Metz, France
- 2LTSER-Zone Atelier Moselle, Metz, France
- 3Institut des Substances et Organismes de La Mer, ISOMer, UR 2160, Nantes University, Nantes, France
Rare Earth elements (REE) have become essential in strategical sectors such as high- and green-technologies. Their increasing use in human activities worldwide leads to anthropogenic REE releases detectable in all compartments of the environment, transforming REE into emerging contaminants. However, their potential impacts on ecosystems are still poorly understood. In order to have a comprehensive understanding of REE ecotoxicology and to properly assess their environmental risk, we analysed the toxicity of three representative REE (neodymium Nd, gadolinium Gd, and ytterbium Yb). Following recommendations of the European Chemicals Agency, we assessed REE hazard by performing standard ecotoxicological tests on three freshwater species belonging to different trophic levels (algae, crustacean and fish). EC50 were calculated using different modes of expression of REE exposure concentration (based on nominal, measured total and dissolved concentrations) in order to more properly and accurately determine REE toxicity. In order to get closer to environmental conditions, we also tested the toxicity of REE in mixture because all of them occur naturally as such in the environment. Moreover, we added dissolved organic matter (DOM) in the test medium because DOM is ubiquitous and drives REE speciation in freshwater systems. The Results showed that DOM significantly reduced REE bioaccumulation and toxicity, probably by formation of non-bioavailable REE-DOM complexes. The algal species was the most sensitive to REE. Despite slight differences between Nd, Gd and Yb in behaviour and bioaccumulation, the three REE exhibited comparable toxicity and additive effects in mixture to all tested organisms. Thus, we considered REE as a uniform group and, for the first time, we used mixture toxicity values and environmental mixture concentrations to assess the risk of REE in freshwater (instead of considering different REE separately). The results revealed that the risk is currently limited to wastewater treatment plants, and industrial and mining activities, where released quantities of REE can induce severe damage to exposed freshwater organisms. However, the risks are likely more widespread in the future because anthropogenic REE releases are expected to increase.
1 Introduction
Rare Earth elements (REE) are a group of seventeen metals including the lanthanide series, scandium (Sc) and yttrium (Y). They are naturally present in the Earth crust and thus occur all together in the environment. These elements share similar physicochemical properties and they are divided essentially into three subgroups based on their atomic number: LREE (Light Rare Earth Elements), MREE (Medium Rare Earth Elements) and HREE (Heavy Rare Earth Elements). Nowadays, REE are used in a large variety of applications in high technologies (smartphone, computer), in the production of “clean energy” (wind turbine, solar panel) and many other fields. REE are considered as critical raw materials (European Commission, 2011) because they are essential in strategic sectors and increasingly in demand. REE are released along their life cycle into all environmental compartments (water, soil, and atmosphere). Possible sources are mining (Liang et al., 2014; Hao et al., 2016; Guo et al., 2019; Liu et al., 2019), industry (Kulkarni et al., 2006; Kulaksız and Bau, 2013, 2011; Klaver et al., 2014), medical uses (Bau and Dulski, 1996; Trapasso et al., 2021), agriculture (Sadeghi et al., 2013; Möller et al., 2014) and e-waste management (Gwenzi et al., 2018). The presence of anthropogenic REE in aquatic systems leads to positive anomalies (Song et al., 2017), raising environmental concern. REE ecotoxicology is still poorly understood, despite the increasing number of studies (Arienzo et al., 2022). The existing data are variable and there is no consensus concerning the potential uniformity of their behaviour and toxicity, which limits their environmental risk assessment (ERA) (Blinova et al., 2020). A reliable ERA of REE is urgent because anthropogenic REE are already detectable in the environment and their use and associated releases are expected to increase in the future (Balaram, 2019). Regulatory agencies, such as the European Chemicals Agency (ECHA), recommend assessing substance hazards in ecosystems by performing ecotoxicological standard tests. These tests are convenient because they are relatively easy to set up and because methods are described in detail in the guidelines from Organisation for Economic Co-operation and Development (OECD) and International Organization of Standardization (ISO), which make them reproducible and repeatable. However, standard tests are sometimes considered to be too simplistic and to do not accurately reflect environmental conditions (Bednarska et al., 2013).
In the current study, we aimed to assess REE hazard in freshwater ecosystems by performing standard ecotoxicological tests in more realistic conditions, in order to propose an integrated and more relevant ERA. We selected three representative REE of specific concern, neodymium (Nd) as LREE, gadolinium (Gd) as MREE and ytterbium (Yb) as HREE. Nd is one of the most used REE, especially in permanent magnets (Campbell, 2014). Gd is the most common anthropogenic REE found in worldwide natural waters (Rogowska et al., 2018; Louis et al., 2020; Trapasso et al., 2021). Yb ecotoxicity has been very poorly studied compared to other REE, and thus, should be investigated as a priority (Blinova et al., 2020). We tested these three elements alone and in mixture in order to determine if REE, regardless of their subgroup, have a similar behaviour and toxicity. Following ECHA guidelines, we evaluated their toxicity on three freshwater species belonging to different trophic levels, using standard tests.
2 Material and methods
First, a test of growth inhibition with a microalga (primary producer) was performed, followed by a mobility inhibition test with a crustacean (primary consumer). Finally, we conducted a test evaluating mortality of a fish species (secondary consumer). Standard tests are usually used to assess the toxicity of individual substances. In order to get closer to environmental conditions where REE occur together, Nd, Gd and Yb toxicity was also tested in mixture. Standard tests based only on mineral media without dissolved organic matter are usually used to assess the toxicity of individual substances, but this approach does not reflect environmental conditions in freshwater systems. To get an higher environmental realism, we added dissolved organic matter (DOM) to the media because DOM is ubiquitous and largely drives REE speciation in natural freshwaters (Tang and Johannesson, 2003; Johannesson et al., 2004; Sonke, 2006; Pourret et al., 2007; Marsac et al., 2011; Davranche et al., 2017). It likely influences the subsequent REE toxicity, but there has been little study done so far. Nd, Gd and Yb were tested in individual single experiments, and in a ternary mixture experiment in the absence (0 mg L−1 Dissolved Organic Carbon = DOC) and presence (8 mg L−1 DOC) of DOM. We worked with this DOC concentration because it is representative of worldwide river and lake concentrations (Thurman, 1985). Our experimental approach is summarized in Figure 1.
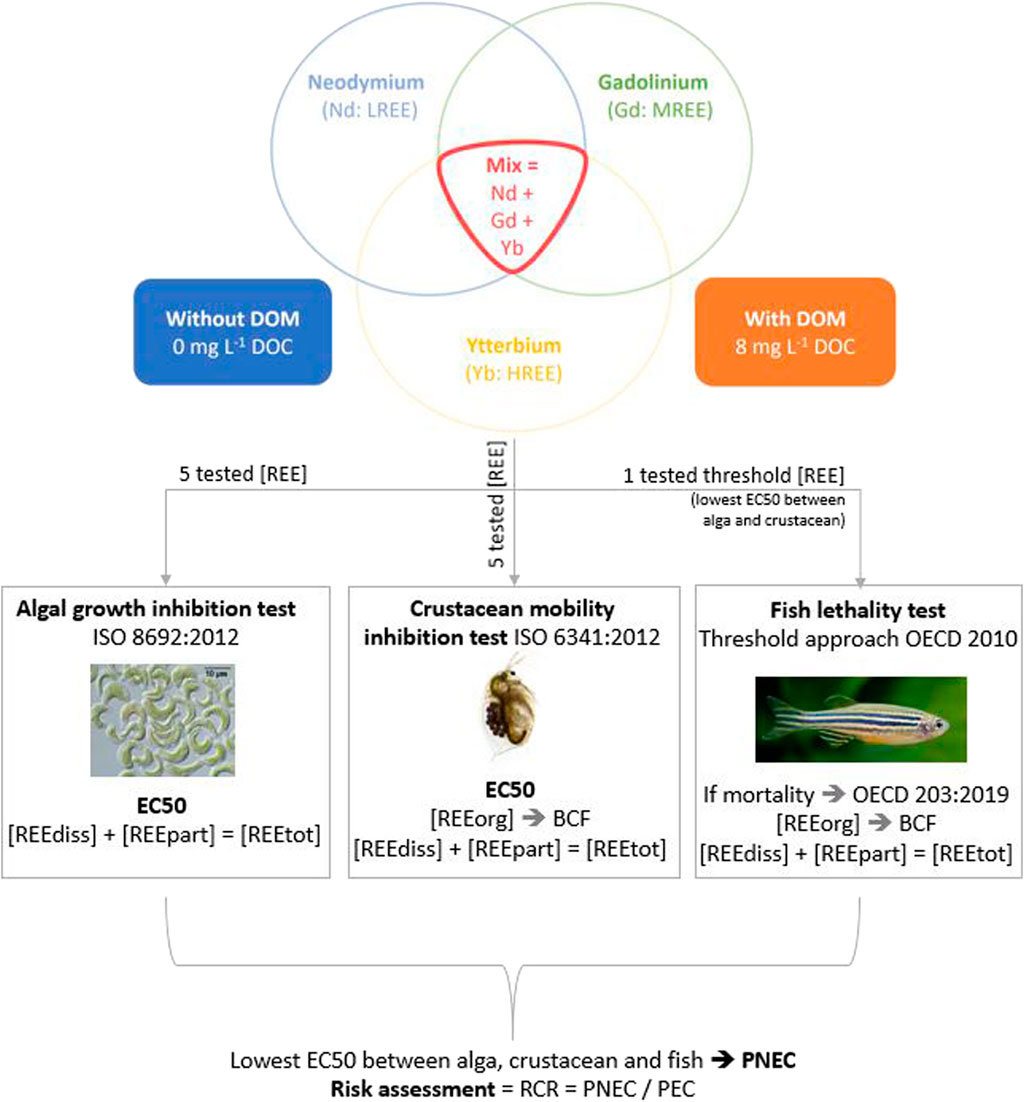
FIGURE 1. Synthetic experimental approach used to assess the risk of REE in freshwater systems. LREE, light rare earth element; MREE, medium rare earth element; HREE, heavy rare earth element; [REE], rare earth element concentration. Text below the pictures refer to the parameters calculated or measured at the end of each test: EC50 = effective concentration 50%; [REEdiss] = dissolved (<0.45 µm) REE concentration measured at t1; [REEpart] = particulate (>0.45 µm) REE concentration measured at t1; [REEtot] = calculated total ([REEdiss] + [REEpart]) REE concentration; BCF = bioconcentration factor. PNEC, predicted no effect concentration; PEC, predicted environmental concentration; RCR, risk characterisation ratio.
2.1 Rare earth elements (REE), dissolved organic matter (DOM) and test medium preparation
REE stock solutions were prepared by dissolution in MilliQ water (5 g L−1 REE salt; neodymium nitrate (Nd(NO3)3.6H2O), gadolinium chloride (GdCl3.6H2O), ytterbium chloride (YbCl3.6H2O); all purity >99%; Sigma Aldrich). Salts of chlorides and nitrates were used because the complexation of Nd, Gd and Yb with Cl− and NO3− is negligible. REE concentrations were measured in the stock solutions and precipitation was not observed. Prior to the experiment, the flasks (cleaned with a HCl 2%–3% aqueous solution) were exposed to the ISO (algal and crustacean tests) or OECD (fish test) medium spiked with REE during at least 12 h in order to equilibrate glass wall adsorption sites with REE and to limit REE losses during the experiment. After this preconditioning, the flasks were emptied and the medium was renewed. Then, ISO/OECD medium, DOM (in experiments with DOM) and REE were added consecutively to the flask constituting the test medium. The start of the experiment (t0) began by the addition of the respective test organism. For each tested REE concentration, an intermediate solution was prepared by dilution of the REE stock solution in MilliQ water so that an equivalent volume of REE solution could be added to all test flasks.
The tested DOM had been prepared at Wageningen University, the Netherlands, by purification of local groundwater. It is mainly composed of fulvic acid (>80%) as determined by the method of van Zomeren and Comans, (2007). The stock solution was stored in the dark at 5°C. The DOM stock solution was diluted in MilliQ water to obtain the desired DOC concentration of 8 mg C L−1 in the test flasks (Lachaux et al., 2022).
2.2 Algal growth inhibition test (primary producer)
Growth inhibition of the unicellular green alga Raphidocelis subcapitata was assessed according to the guideline ISO 8692:2012 (International Organization for Standardization, 2012a). Briefly, algae from our laboratory culture were exposed during 72 h to five different REE concentrations (Supplementary Table S1) allowing the determination of effective concentrations (ECx). After testing their individual toxicity, we tested the toxicity of REE in ternary equitoxic mixture, meaning that each REE was present in the mixture at an equivalent toxicity (ECx). Then, REE concentrations tested in the mixture (Table 2) were selected according to the nominal ECx values (EC5, EC10, EC30, EC50, EC65, EC75, EC80) of each REE determined in the single experiments (Lachaux et al., 2022). One third of each ECx was used (because there were 3 compounds in the mixture) leading to an expected mixture effect of x% in case of additivity (ECxMix = ECxNd/3 + ECxGd/3 + ECxYb/3) (Minguez et al., 2018). A control (no REE) was systematically included to the experiments. Each concentration was tested in triplicate. The experiment was conducted in 150 ml Erlenmeyer flasks (50 ml of test medium per flask) at 23°C (±2°C), pH 8.1 (±0.2) with permanent shaking at 110 rpm and continuous light at 90 (±10%) µmol photon/m2/s. When the experiment ended (72 h), cell density was estimated measuring chlorophyll b fluorescence (λ absorption = 485 nm; λ emission = 640 nm) with a FLUOStar microplate reader (BGM LABTECH, Champigny s/Marne, France). The experiment was repeated twice to check repeatability.
2.3 Crustacean mobility inhibition test (primary consumer)
Mobility inhibition of the cladoceran Daphnia magna was evaluated according to the ISO 6341:2012 guideline (International Organization for Standardization, 2012b). Briefly, first instar daphnids (less than 24 h) originating from our laboratory culture were exposed to five REE concentrations (Supplementary Table S2) in glass test tubes (10 ml of test medium per tube) during 48 h at 20°C (±2°C). The procedure for mixture experiments was the same as in the algal test. Each concentration was tested with at least four replicates (5 daphnids per replicate, 20 per concentration). The pH of the ISO medium was adjusted to 6.5 (±0.2), with a 3.2% HCl solution, instead of pH 7.8 in order to enhance REE solubility and bioavailability. This pH value is included in the range (pH = [6–9]) tolerated by D. magna (International Organization for Standardization, 2012b). The daphnids were considered as non-mobile when they did not move during the 15 s following a gently tube shaking. The experiment was repeated twice.
2.4 Fish lethality test (secondary consumer)
We applied a “threshold approach” according to OECD (2010) as an alternative to the OECD TG 203 fish lethality test. Juveniles (1 month) of zebrafish Danio rerio originating from a certified fish farming for laboratory activities (Elevage de la grande rivière, Saint Forgeux, France) were exposed to a single concentration called “threshold concentration (TC).” The TC was derived from the lowest EC50 between the alga and crustacean species. The aim of the threshold approach is to determine if fish are more or less sensitive than algae or crustacean. After a gradual acclimatization period of 7 days, the fish were exposed to REE during 96 h in crystallising dishes (8 fishes in 1 L respecting the maximum load of 1 g of fish per litre) at pH 7.7 (±0.2) and 25°C (±2°C). In the mixture experiment, the three REE were present at an equivalent concentration (1/3 of each REE). The test medium was renewed daily in order to limit the decrease of REE concentration during the experiment. In addition to mortality, we checked twice a day for potential abnormal behaviour (OECD, 2019a). If mortality occurs in exposed groups, the full OECD TG 203 fish acute toxicity test (OECD, 2019b) should be performed. If no mortality is observed, the TC can be used as a surrogate of the EC50 value in the further hazard and risk assessment. The experiment was conducted according to the regulation on the protection of animals used for scientific purposes (European Commission, 2010) by reducing the number of animals in accordance with the 3Rs principles (Reduction, Replacement and Refinement). The experiment was performed once with one replicate (= 8 fish) per concentration according the previous cited guidelines.
2.5 REE concentration measurements and analysis
REE concentrations were determined in the test medium and in the organisms for all tested concentrations and for one experiment repetition. They were measured at the end of the experiment (t1) by Inductively Coupled Plasma–Mass Spectrometry (ICP-MS, Nexion 350x PerkinElmer). The test medium was filtered using a mixed cellulose ester filter (MF-Millipore, reference: HAWP04700) in order to separate the particulate phase (>0.45 µm) retained by the filter and the dissolved phase (<0.45 µm) constituting the filtrate. For each tested concentration, REE concentration was measured in the filtrate. We consider this concentration as the measured dissolved (<0.45 µm) concentration at t1 (denoted [REEdiss]). In addition, the amount of REE retained on the filter was quantified after complete mineralization of the filter with 2 ml HNO3 65% (Fischer Scientific) at 80°C. The concentration of particulate REE (>0.45 µm) in the test medium was then calculated and added to the dissolved concentration to determine the total REE concentration at t1 (denoted [REEtot]). Prior to ICP-MS analysis, the filtrate was acidified at 1% [v/v] with HNO3 69% in MilliQ water and stored at 4°C.
REE recovery at t1 was calculated as the ratio between the total REE mass (sum of REE mass in the water and the organisms) measured at t1 and the nominal total REE mass introduced at t0. Calculated recovery lower than 80% or higher than 120% indicate that nominal and measured REE concentrations were significantly different. In that case, the measured concentration was used to analyse toxicity data (International Organization for Standardization, 2012b). For substances such as REE where the exposure concentration decreases significantly during the experiment (e.g., Weltje et al., 2002; Vukov et al., 2016; Blinova et al., 2018; Romero-Freire et al., 2019), the use of a mean concentration is more suitable than the unique concentration at the beginning or at the end of the experiment (OECD, 2000). Thus, REE exposure concentrations in the three tests were corrected by calculating the respective geometric mean concentrations:
with C0 being the nominal concentration at t0 and C1 being the measured (total or dissolved) concentration at t1. When using [REEtot] to calculate the geometric mean, the latter is denoted [REEtot]mean and when using [REEdiss] it refers to [REEdiss]mean. The main objective in correcting exposure concentrations (use of the mean of measured concentration) was to get closer to the real exposure in order to properly estimate and analyse bioaccumulation and toxicity data.
REE concentration was measured in the organisms (denoted [REEorg]) in order to estimate REE bioaccumulation and to better understand observed biological effects. For each tested concentration, REE concentration was measured in a pool of 20 daphnids (in crustacean test) or in 8 fish (in fish test). The concentrations were not determined in the algae due to the low collected biomass preventing an accurate estimation of algal bioaccumulation. The organisms were dried at 50°C during 24 h and digested with 1 ml of 65% HNO3 at 80°C before analysis. Bioconcentration factor (BCF) was calculated using the formula:
For ICP-MS analyses, all samples, blanks and standards were diluted to a final concentration of 2% HNO3. The following isotopes were measured: 146Nd, 157Gd and 173Yb. Rhenium (187Re) was used as internal standard. Limits of detection (LOD) and quantification (LOQ) were determined and ranged from 0.1 to 0.3 µg L−1 for each element in aqueous samples and from 10 to 40 µg g−1 dry weight in solid samples (daphnid, fish, filter). Analytical and procedural blanks were prepared and analysed using the same analytical procedure as for samples. The accuracy of the analytical protocol was validated using international certified materials (BCR-667 and BCR-668) consisting, respectively, of estuarine sediments and mussel tissues from LGC standards. The recovery of Nd, Gd and Yb were, respectively 111, 113, and 100%.
REE mass balance at t1 was calculated in each test in order to describe the distribution of REE among three different compartments: the organisms (crustaceans and fish), the dissolved and particulate phases of the water. First, REE recovery was calculated in order to estimate REE losses possibly due to adsorption and precipitation. Then, the proportion of REE measured in a given compartment was calculated as a function of the total mass (sum of REE mass in all compartments) measured at t1.
2.6 Data analysis
The dose response-curves, the ECx values and their confidence intervals (95% certainty) were obtained using R (version 3.6.1), package “drc” (Ritz et al., 2015). A log-logistic model with two parameters (LL.2) was used to fit the data. EC50 were calculated and expressed based on the nominal (theoretical) or the geometric mean of total ([REEtot]mean) and dissolved ([REEdiss]mean) concentrations. EC50 value comparisons were based on confidence interval overlap. The relationship between two variables was evaluated through a simple linear regression test with the function “lm” in R. BCF values between the different experimental groups were compared by performing a non-parametric Kruskal–Wallis test because normality of data distribution was not respected (Shapiro-Wilk test, p-value < 0.05).
We investigated the potential interaction of REE in mixture. There is no interaction when mixture toxicity is similar to the sum of single compounds toxicity, thus, mixture toxicity is considered as additive. This is defined by the concept of concentration addition (CA) (also called additivity model) (Loewe and Muischnek, 1926) expressed as:
with ci = concentration of compound i in the mixture; ECx,i = effective concentration of compound i inducing x% effect when tested individually (in single experiment).
The use of the CA concept is recommended to describe mixture toxicity because it is more accurate and protective relative to other concepts such as the independent action model (Olwenn Martin et al., 2021). The CA model assumes a similar mode/site of action of mixture compounds and thus it is especially suitable to REE because they share similar physicochemical properties that could lead to similar toxic mechanisms.
We compared observed and predicted (according to CA model) mixture effects by calculating along the dose response-curve:
with observed ECx (mixture) corresponding to the ECx (mixture) obtained from the mixture toxicity experiment and predicted ECx (mixture) corresponding to the ECx (mixture) calculated according to Eq. 3 (CA model).
Stronger or lower observed effects relative to predicted effects by CA means that there is an interaction between mixture compounds. A mixture effect is considered synergistic (more than-additive) if the ratio (calculated according to Eq. 4) is ≤0.5 and antagonistic (less than-additive) if the ratio is ≥2 (Belden et al., 2007). We used these ratios because they limit the bias in the interpretation of mixture effects due to intra- and inter-laboratory variability of experimental design, conduct and data analysis (Olwenn Martin et al., 2021). The use of narrower ratios, such as 0.8–1.2 (Broderius et al., 1995) can lead to overestimated interactive effects (Olwenn Martin et al., 2021).
We assessed the REE environmental risk according to ECHA procedures following three steps: 1) hazard assessment; 2) exposure assessment and 3) risk characterisation. We assessed REE hazard by calculating predicted no effect concentration (PNEC), which represents the EC50 of the most sensitive species among the different trophic levels [Min (EC50)] divided by an assessment factor (AF). The use of AF is a conservative and protective approach that takes into account the uncertainty due to intra- and inter-laboratory variation of toxicity data, intra- and inter-species variations (biological variance), short-term to long-term toxicity extrapolation and laboratory data to field impact extrapolation (European Commission, 2003). For exposure assessment, expressed by the predicted environmental concentration (PEC), we selected in the literature several measured REE concentrations representing different exposure scenarios in freshwater (European Chemicals Agency, 2016a). REE concentration can change drastically according to the geographic region and the environmental context, which is mainly due to human activities. Finally, we calculated the risk characterisation ratio (RCR):
RCR aims to determine if the hazard concentration is higher or lower than the exposure concentration. Risk is not controlled when RCR >1 and risk is controlled where RCR <1 (European Chemicals Agency, 2016b).
3 Results
3.1 REE concentrations in the test medium and organisms
Measured REE concentrations were significantly lower than nominal concentrations. REE recovery in the media ranged from 46% to 105% in the algal test (Supplementary Table S3), from 34% to 89% in the crustacean test (Supplementary Table S4) and from 67% to 85% in the fish test (Supplementary Table S5), below 80% for most tested concentrations. Recovery and dissolved concentrations tend to be higher with DOM than without DOM (Table 1). In the algal test, between 57% and 97% of REE were measured in the dissolved phase in the presence of DOM against less than 2% in the absence of DOM, meaning that almost the totality of REE was in the particulate phase without DOM (Supplementary Table S3). In the crustacean and fish tests, the proportion of REE in the dissolved phase tended to be higher with than without DOM, especially for tested REE concentrations lower than 10 mg L−1 (Supplementary Tables S4, S5).
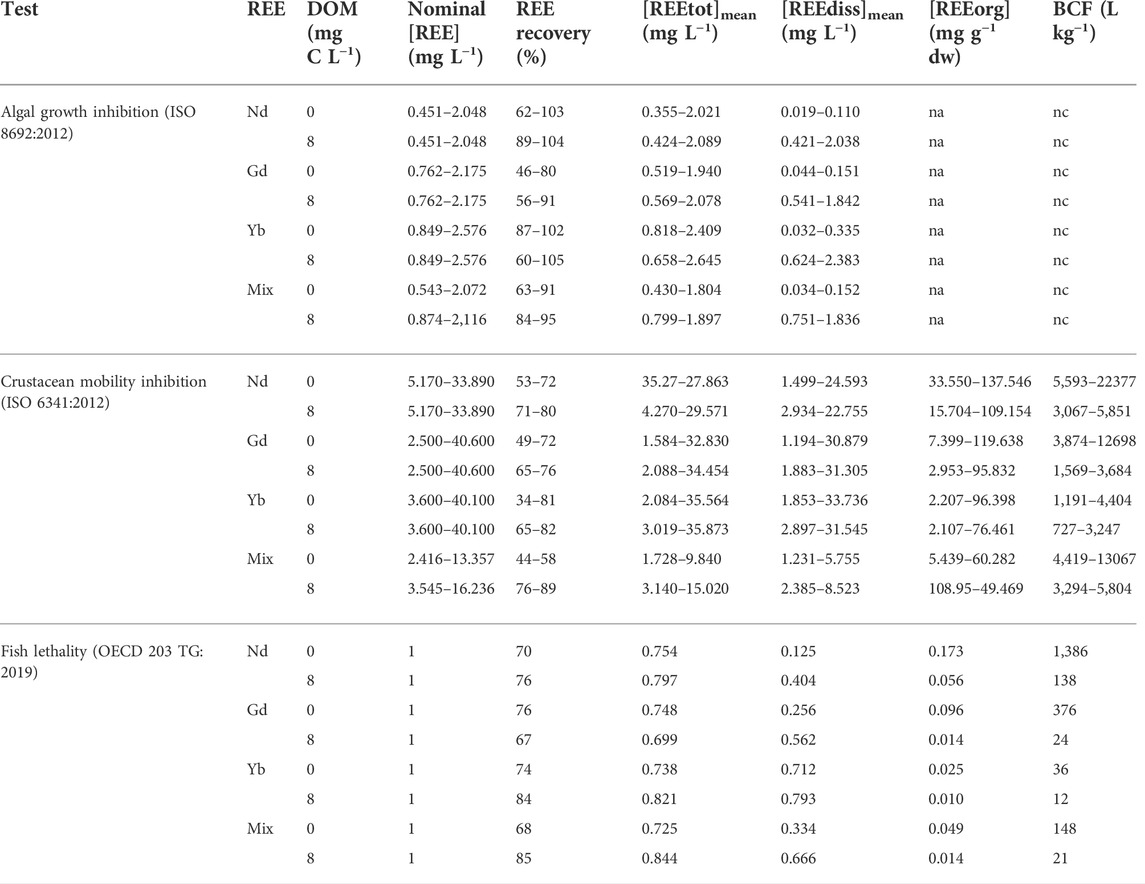
TABLE 1. REE concentrations in the medium and the organisms. Data represent the interval (min-max) of five values each. REE recovery at t1 was calculated as the ratio between the total REE mass (sum of REE mass in the water and the organisms) measured at t1 and the nominal total REE mass introduced at t0. [REEtot]mean and [REEdiss]mean correspond to the geometric mean (=√(C0*C1)) where C0 refers to the nominal concentration at t0 and C1 refers to the total or dissolved concentration measured at t1. [REEorg] corresponds to the mean of REE concentrations measured at t1 in 8 fishes (in fish test) or to the REE concentration measured at t1 in a pool of daphnids (≥20 daphnids) (in crustacean test). dw = dry weight. BCF = Bioconcentration factor = [REEorg]/[REEdiss]mean. na = non available. nc = not calculated.
In the crustacean and fish tests, we measured significantly higher REE concentrations in the exposed organisms relative to the controls (not exposed). The concentrations of Nd, Gd, Yb measured in D. magna were positively correlated to REE dissolved concentrations ([REEdiss]mean) (linear regressions: p-values < 0.05). REE accumulation in D. magna was significantly higher than in D. rerio. The crustaceans accumulated between 2 and 138 mg g−1 REE, which resulted in BCFs between 727 and 22,377 L kg−1, while the fish accumulated between 0.010 and 0.173 mg g−1 REE, corresponding to BCFs ranging from 12 to 1386 L kg−1 (Table 1). The crustaceans accumulated in average 6% of total REE introduced in the medium (Supplementary Table S4) and fish less than 1% (Supplementary Table S5), regardless of the tested condition. REE bioaccumulation decreased with increasing atomic number. In the single (Table 1) and mixture (Table 2) experiments, BCFs for D. magna and D. rerio tended to follow the order Nd > Gd > Yb. In the crustacean experiments with single REE, BCFs of Yb were significantly lower than those of Nd (Table 1) (Kruskal–Wallis test, p-value < 0.05). Gd bioaccumulation was not significantly different from those of Nd and Yb. REE accumulation by crustaceans and fish decreased mostly in the presence of DOM. In average, the crustaceans accumulated 9% and 4% of total REE introduced in the medium in the absence and presence of DOM, respectively (Supplementary Table S4). In the crustacean test, BCFs of Nd, Gd and the mixture significantly decreased with DOM (Kruskal–Wallis tests, p-values < 0.05) but not for Yb (Table 1). Mean BCFs were 2.8, 2.6, 2.1, and 1.3 fold lower in the presence compared to in the absence of DOM for Nd, Gd, the mixture and Yb, respectively. In the fish test, BCFs of Gd, Nd, the mixture and Yb were 16, 10, 7, 3 fold, respectively, lower with DOM.
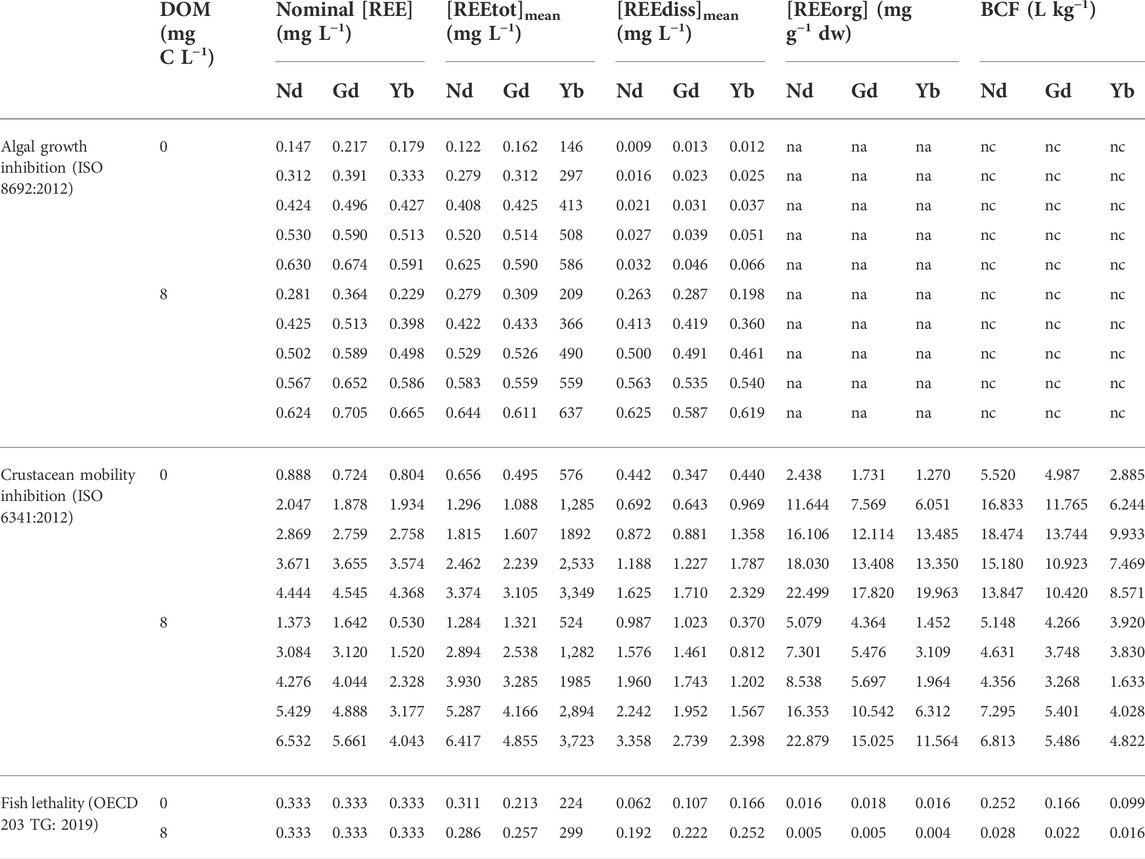
TABLE 2. Concentrations of Nd, Gd and Yb in the medium and the organisms of mixture experiments. [REEtot]mean and [REEdiss]mean correspond to the geometric mean (=√(C0*C1)) where C0 refers to the nominal concentration at t0 and C1 refers to the total or dissolved concentration measured at t1. [REEorg] corresponds to the mean of REE concentrations measured at t1 in 8 fishes (in fish test) or to the REE concentration measured at t1 in a pool of daphnids (≥20 daphnids) (in crustacean test). dw = dry weight. BCF = Bioconcentration factor = [REEorg]/[REEdiss]mean. na = non available. nc = not calculated.
3.2 REE biological effects
3.2.1 Mode of expression of REE toxicity values
The majority of total REE concentrations measured at the end of the experiment ([REEtot]) represented less than 80% of the nominal concentration in the algal (Supplementary Table S1), crustacean (Supplementary Table S2) and fish (Table 1) tests. Consequently, in addition to the use of nominal concentrations (EC50nominal), REE EC50 values were systematically calculated according to the geometric mean of total ([REEtot]mean) and dissolved ([REEdiss]mean) concentrations (c.f. Section 2.5).
3.2.2 Comparison between the different species and REE
In the algal and crustacean tests, the EC50 values followed the order nominal > [REEtot]mean > [REEdiss]mean. The EC50 determined for R. subcapitata were significantly lower than those determined for D. magna (Table 3).
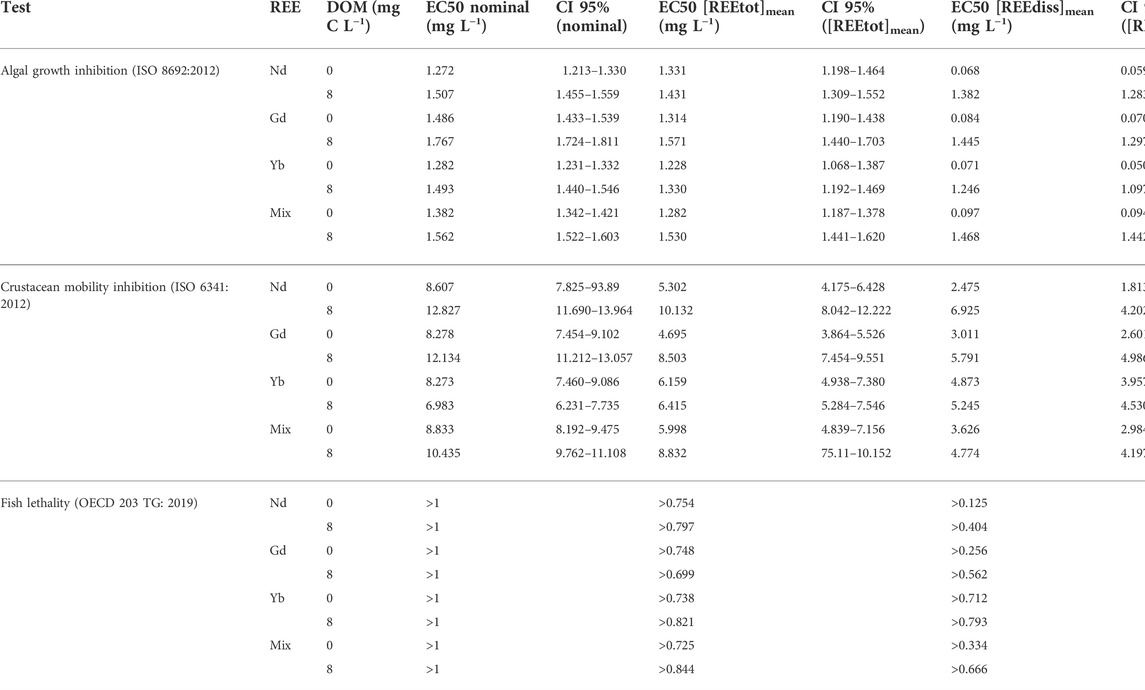
TABLE 3. EC50 values of Nd, Gd, Yb and the mixture for Raphidocelis subcapitata, Daphnia magna and Danio rerio. EC50 values and their confidence intervals (CI) were calculated with R software according to nominal concentrations, and the geometric means of total [REEtot]mean or dissolved [REEdiss]mean concentrations.
In the algal tests, REE EC50 ranged from 1.272 to 1.767 (nominal concentration), 1.228 to 1.620 ([REEtot]mean) and 0.068 to 1.468 ([REEdiss]mean) mg L−1. EC50[REEdiss]mean were around 18 and 1.2 fold lower than EC50nominal, with and without DOM, respectively. EC50[REEdiss]mean confidence intervals of Nd, Gd and Yb overlapped, both in the absence and presence of DOM (Table 3). In addition to growth inhibition, we observed an algal cell aggregation and precipitation in the presence of REE for all tested concentrations. This phenomenon occurred exclusively in the absence of DOM.
In the crustacean tests, REE EC50 ranged between 7 and 12.8 (nominal concentration), 4.7 and 10.1 ([REEtot]mean), 2.5 and 6.3 ([REEdiss]mean) mg L−1. EC50[REEdiss]mean were around 2–3 fold lower than EC50nominal. EC50[REEdiss]mean confidence intervals of Nd, Gd, Yb overlapped, except the one for Yb without DOM, which is above Nd and Gd (Table 3). Mobility inhibition was positively correlated to REE dissolved ([REEdiss]mean) and bioaccumulated ([REEorg] in D. magna) concentrations, in the absence and presence of DOM (linear regressions: p-values < 0.05).
The difference of EC50[REEdiss]mean between the three REE, individually and in mixture, did not exceed a factor 1.4 and 2, in the algal and crustacean tests, respectively (Table 3).
According to the threshold approach (OECD, 2010), the EC50 determined in the algal test (lower than the EC50 in crustacean test) was selected as the threshold concentration (TC) in the fish test to determine if the latter is more or less sensitive than the algal species. Because Nd, Gd and Yb exhibited comparable EC50 values, we tested, for the three REE, a unique TC of 1 mg L−1 close to the EC50nominal obtained in the algal test (Table 3). No mortality nor abnormal behaviour was observed in the fish exposed to 1 mg L−1 REE. Consequently, the performance of the full OECD TG 203 test was not required (OECD, 2010). One fish died in the control group, the first day of the experiment but the test stayed valid because the OECD guideline (OECD, 2019a) tolerates one dead fish among controls due to inter-individual variability.
3.2.3 REE mixture effects
In the algal test, the EC50[REEdiss]mean of the mixture (0.097 and 1.468 mg L−1 without and with DOM, respectively) were relatively close to the mean of the individual EC50[REEdiss]mean of Nd, Gd and Yb (0.074 and 1.358 mg L−1 without and with DOM, respectively). A similar trend was observed in the crustacean test with the EC50[REEdiss]mean of the mixture at 3.6 and 4.8 mg L−1 and the mean of the EC50[REEdiss]mean of Nd, Gd and Yb at 3.4 and 5.9 mg L−1 [each without or with DOM, respectively (Table 3)].
Thus, in both the algal and crustacean tests, experimental mixture effects were relatively close to the effects predicted by the CA model (Figure 2). Along the dose-response curves, the ratios between observed and predicted mixture effects were within the range of 0.5–2 in the algal (Supplementary Table S6) and crustacean (Supplementary Table S7) tests, indicating the absence of interactions between the three REE. The mean ratio along the dose-response curve was 1.33 and 1.08 in the algal test; 1.06 and 0.80 in the crustacean test (each without or with DOM, respectively).
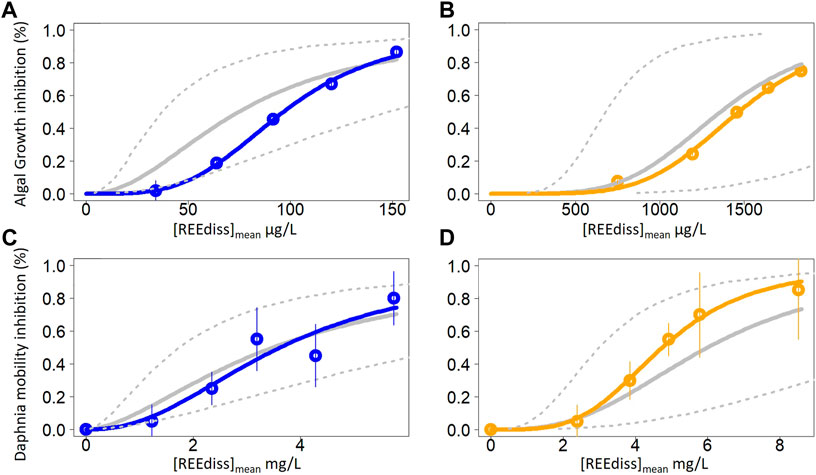
FIGURE 2. Dose response curves of the ternary mixtures for Raphidocelis subcapitata (A,B) and Daphnia magna (C,D) in the absence (A,C) and presence (B,D) of dissolved organic matter. The coloured dots and solid line refer to the observed effects. Each dot represents the mean of 3 and 4 replicates in algal and crustacean test, respectively. Vertical bars represent standard deviation. The grey solid line corresponds to the predicted effects according to concentration addition model. The dashed lines represent the ratios 0.5 (upper line) and 2 (lower line) corresponding to the interval of additivity predictions. Mixture effects are additive when observed effects are included between the two dashed lines, synergistic or antagonistic when observed effects are above the upper line or below the lower line, respectively. The dose response curves and confidence interval were plotted with R software. A log logistic 2-parameter model was used to fit the data [REEdiss]mean = √(C0*C1)) where C0 refers to the nominal concentration at t0 and C1 refers dissolved concentration measured at t1.
3.2.4 DOM influence on REE toxicity
In the algal and crustacean tests, REE EC50[REEdiss]mean determined with DOM were significantly higher than the EC50 without DOM, except for Yb in the crustacean test where no significant difference was observed (Table 3). In the presence of DOM, the EC50[REEdiss]mean were 1.1–2.8 and 15–20 fold higher than the EC50 determined without DOM, in the crustacean and algal tests, respectively. In the presence of DOM, no algal cell aggregation and precipitation were observed, unlike in the absence of DOM.
4 Discussion
4.1 REE in the medium and exposure concentrations
The relatively low recovery calculated in the three test media (Table 1) indicates a decrease of REE exposure during the experiment, which has been already described (González et al., 2015; Blinova et al., 2018; Romero-Freire et al., 2019). This decrease could be explained by metal adsorption on tips and/or on glass material despite the previous saturation, and/or by metal precipitation (OECD, 2019b). The preconditioning of the test flasks may have reduced but not completely prevented REE adsorption on glass walls.
The formation of REE-DOM complexes probably maintained REE in the dissolved/colloidal phase (<0.45 µm) (Johannesson et al., 2004) explaining the higher metal recovery and the higher dissolved metal concentrations observed in the presence of DOM. In the crustacean test (at tested REE concentrations >10 mg L−1), the lower proportion of dissolved REE with DOM compared to without DOM can be explained by the saturation of complexation sites of DOM due to the high metal loading (Marsac et al., 2010).
In the algal tests conducted without DOM, REE were mostly measured in the particulate phase (>0.45 µm), which explains the low measured dissolved concentration. This suggest a relevant REE precipitation in the medium, also reported in other ecotoxicological studies (González et al., 2015; Joonas et al., 2017). We assume that REE precipitated with phosphates (present in the algal medium in the form of KH2PO4) because REE-phosphate complexes are poorly soluble (Johannesson et al., 1995). We hypothesize that REE bound preferentially to DOM (in the experiments with DOM) rather to phosphates because DOM concentration (8 mg L−1) in the medium was higher than KH2PO4 concentration (1.6 mg L−1). REE-DOM complexation could reduce REE precipitation with phosphates, which would explain the lower REE concentration measured in the particulate phase and consequently the higher dissolved concentration in the presence of DOM.
These results suggest that the use of nominal and total measured concentrations could be misleading and cause REE toxicity underestimation. The relatively low recovery of REE makes the use of nominal concentrations inappropriate. The total concentration (particulate + dissolved) does not properly represent the real exposure because it can include a large proportion of REE being poorly soluble and bioavailable, mainly due to REE precipitation. The dissolved phase could also contain some chemical forms of REE that are poorly or not bioavailable and toxic (such as colloidal REE bound to DOM) but to a lesser extent than the total phase. Thus, we consider that the geometric mean of measured dissolved concentrations ([REEdiss]mean) is more suitable for the proper interpretation of REE bioavailability and toxicity data because it is closer to the bioavailable and toxic fractions of REE than nominal and total concentrations.
4.2 REE bioaccumulation
4.2.1 DOM influence on REE bioaccumulation
The formation of REE-DOM complexes decreased REE bioavailability, explaining the reduced bioaccumulation in the presence of DOM observed in the crustacean test (Lachaux et al., 2022). We assume a similar REE behaviour in the fish test because the composition of the crustacean and fish test medium is identical. Our results corroborate those of MacMillan et al. (2019), which showed that DOM reduced REE bioavailability and bioaccumulation by zooplankton from Canadian lakes. A decrease of REE accumulation by different alga species was also observed in the presence of organic ligands such as fulvic and humic acids (El-Akl et al., 2015; Rowell et al., 2018), ethylenediamine tetraacetic, nitrilotriacetic acid, citric acid, malic acid, diglycolic acid, iminodiacetic acid (Yang et al., 2014; Zhao and Wilkinson, 2015; Tan et al., 2017; Yang and Wilkinson, 2018; Aharchaou et al., 2020). Contrary to Nd, Gd and the mixture, we observed that the difference of Yb accumulation with and without DOM was not as large in the crustacean and fish tests. This could be explained by an unchanged bioavailability of Yb due to Yb(OH)3 precipitation with and without DOM in the single experiments (Lachaux et al., 2022). Precipitation may influence Yb bioavailability and the subsequent bioaccumulation with and without DOM while Nd, Gd, mixture bioavailability was mainly driven by REE-DOM complexation (no precipitation with hydroxides occurred for Nd, Gd and the mixture), as shown earlier (Lachaux et al., 2022).
4.2.2 Comparison between the different REE and species
Contrary to the fish, daphnids were not washed in a “clean” medium (without REE) before the measurement of REE concentration in the organisms. Consequently, some REE could be adsorbed on the external carapace of the daphnids, which would lead to an overestimation of the measured REE bioaccumulation. However, we assume that the proportion of adsorbed REE of the total REE concentration measured in D. magna is negligible. The daphnids were collected on the filter after the filtration of the test medium. In that case, the airflow from the vacuum system should flush the water included in the carapace removing most of the potentially adsorbed REE.
The calculated proportion of REE accumulated by the organisms is highly dependent on the total volume of the medium, which defined the total mass of REE introduced at t0. The relatively high proportion of REE accumulated by the crustaceans (Supplementary Table S4) compared to the fish (Supplementary Table S5) could be explained by the difference of medium volume in the crustacean (10 ml) and fish (1 L) tests. Thus, we considered that the use of BCFs (based on concentration) is more appropriate to compare REE bioaccumulation between the species and REE.
The lower bioaccumulation of Yb relative to Nd that we observed in the crustaceans and fish could be explained by Yb(OH)3 precipitation in the test media, which may have decreased Yb bioavailability. A lower bioaccumulation of HREE relative to LREE was also observed in several species belonging to different trophic levels (Qiang et al., 1994; Yang et al., 1999; Moermond et al., 2001; Weltje et al., 2002).
The higher REE accumulation by D. magna compared to D. rerio (Table 1) corroborates the “trophic dilution” observed in natural environments, which consists in an higher REE accumulation by primary consumers (zooplankton, benthic invertebrates) compared to secondary consumers (fish, seal) (Li et al., 2016; Squadrone et al., 2016; Amyot et al., 2017; MacMillan et al., 2017). Similar results were observed in laboratory experimental approaches with a decreasing REE bioaccumulation along the trophic chain: duckweed > daphnia > shellfish > goldfish (Yang et al., 1999). This could be explained by inter-species differences in life traits, defence mechanisms and strategies concerning subcellular distribution of metals among toxic and detoxified fractions (Wallace et al., 2003). Cardon et al. (2019) observed that D. magna accumulated more REE than Oncorhynchus mykiss. They showed that the crustaceans accumulated most (75%) of Y in detoxified form (as metal-rich granules) while the fish did not exhibit any notable detoxification strategy accumulating Y primarily (32%) in a metal-sensitive fraction (mitochondria). In other words, crustaceans may not limit REE uptake as most of the accumulated metal can be detoxified. On the contrary, fish might regulate REE uptake as already low levels of metal accumulated can be toxic.
4.3 REE biological effects
4.3.1 REE toxicity on algae
EC50nominal determined in the algal tests without DOM (1.272–1.486 mg L−1) corroborate the results of Joonas et al. (2017) (EC50nominal = 1.2–1.4 mg L−1). The authors hypothesized a potential indirect effect of REE because of REE precipitation with phosphates. Thus, the observed growth inhibition might have been caused by P starvation instead of direct REE toxicity because REE-phosphate precipitation would reduce REE and phosphate (essential element for algal growth) bioavailability. The algal cell aggregation and precipitation observed in the presence of REE (without DOM) could be a potential indirect effect of REE affecting algal growth. Algal aggregation in the presence of REE was observed also in other studies (Fujiwara et al., 2008; Lürling and Tolman, 2010; Joonas et al., 2017; Blinova et al., 2018). This could be explained by REE interactions with some algal cell surface compounds like membranous phospholipids, carboxylic acids or cellular exudates released by the alga. Algal aggregation by REE was not observed in the presence of DOM probably because of limited interactions of REE with algae due to REE-DOM complexation.
4.3.2 REE toxicity on crustaceans
In the crustacean test, the determined Gd EC50nominal in the absence of DOM (8.3 mg L−1) is in accordance with the results obtained by González et al. (2015) who found that Gd EC50nominal for D. magna was higher than 6.4 mg L−1. On the contrary, Nd and Gd EC50[REEtot] obtained by Blinova et al. (2018) were much lower (0.2–1.5 mg L−1) than those we calculated in the current study (4.7–5.3 mg L−1). The authors stated that a relevant REE precipitation occurred in their test medium (pH 7.8), which can explain the low measured concentrations and subsequent lower EC50 value. In the current study, less precipitation occurred because we used a lower pH (6.5).
4.3.3 REE biological effects on fish
The absence of mortality and abnormal behaviour in the fish exposed to 1 mg REE L−1, corroborate the absence of REE toxicity on zebrafish larvae reported at 1.39 mg L−1 La and 1.73 mg L−1 Yb (Cui et al., 2012) and below 6.71 mg L−1 of Ce, Gd and Lu (Romero-Freire et al., 2019). The threshold approach enabled to determine that fish were not more sensitive than algae. Further, reducing the number of fish used for the experiment is in accordance with the 3Rs principles (European Commission, 2010). Several studies observed also that alga species were the most sensitive to REE (Guida et al., 2016; Romero-Freire et al., 2019; Siciliano et al., 2021). On the contrary, Bergsten-Torralba et al. (2020) showed that Daphnia similis was more sensitive than R. subcapitata. However, the Nd EC50nominal they obtained was much higher (57 mg L−1) than the one we determined in the current study (≈1 mg L−1). Such toxicity difference might be explained by the different chemical form of Nd they used: oxides compared to chloride and nitrate forms used in the current study. Indeed, Joonas et al. (2017) showed that REE oxides were much less toxic (EC50 ranged from 1 to 98 mg L−1) towards R. subcapitata than REE nitrates (EC50 around 1.3 mg L−1).
4.3.4 DOM influence on REE toxicity
The presence of DOM significantly reduced the toxicity of REE, individually and in mixture, towards R. subcapitata and D. magna probably because REE-DOM complexation decreased REE bioavailability and the subsequent bioaccumulation, which led to a decreased toxicity. Our results are in accordance with previous works demonstrating, at 9 mg C L−1, a reduction of Tm (Loveridge et al., 2021) and Dy (Vukov et al., 2016) toxicity to the crustacean Hyalella azteca. Similar results were observed with Chlorella fusca, by a decrease of Ce and La uptake and toxicity in the presence of organic ligands (Aharchaou et al., 2020). This DOM protective effect was mitigated for Yb in the crustacean test probably because of Yb precipitation (Lachaux et al., 2022).
4.3.5 Comparison between the different REE
The absence of toxicity in the fish test and the narrow interval including the EC50 values of Nd, Gd and Yb obtained in the algal and crustacean tests indicate that the different REE have similar biological effects. The three REE have the same classification of hazardous substances to the aquatic environment (United Nations, 2021): category 2 (EC50 > 1 to ≤10 mg L−1) or category 1 (EC50 < 1 mg L−1) if considering EC50[REEdiss]mean obtained in the algal test without DOM. A similar toxicity was observed among 13 REE on the alga Skeletonema costatum (Tai et al., 2010), between Ce, Tm and Y on C. reinhardtii (Morel et al., 2021), among La, Ce, Pr, Nd and Gd on D. magna (Blinova et al., 2018) and between La, Ce and Nd on D. rerio (Huang et al., 2022). This toxicity uniformity among the different REE could be explained by their similar physicochemical properties but there is no consensus in the literature. Some studies claim a higher toxicity of HREE compared to LREE (González et al., 2015; Manusadžianas et al., 2020) while Blaise et al. (2018) found that LREE were more toxic than HREE. Dubé et al. (2019) found a positive correlation between REE toxicity and electronegativity.
4.3.6 REE mixture effects
Beyond the characterization of the potential toxicity differences between REE, it is important to determine if REE should be considered individually or as a group for their ERA. We showed in the algal and crustacean tests that REE mixture effects were additive (Figure 2) and that mixture toxicity was relatively close to REE individual toxicity (Table 3). Our results demonstrate that REE can be considered as a uniform group when assessing their environmental risk, as suggested in the most recent and exhaustive review on REE toxicity in freshwater systems (Blinova et al., 2020). We assume that a mixture including the 17 REE would also induce additive effects because of their similar physicochemical properties and because additivity probability increases with the number of compounds in the mixture (Olwenn Martin et al., 2021). Tai et al. (2010) demonstrated with S. costatum that the toxicity of 13 REE, individually and in mixture, were similar, which suggests additive effects. However, data on REE mixture toxicity is scarce and results differ. Antagonistic interactions were found in C. reinhardtii based on transcriptomic data (Morel et al., 2021). Different mixture effects were described depending on the studied species (Romero-Freire et al., 2019; Bergsten-Torralba et al., 2020), mixture composition (Bergsten-Torralba et al., 2020) or exposure duration (Gong et al., 2021). In the current study, mixture effects were constant regardless of the studied species and medium composition. Here we provide new consistent data about REE mixture toxicity obtained using a reliable methodology (Olwenn Martin et al., 2021), which should help to characterize REE mixture effects. However, complementary studies investigating the toxicity of all REE, individually and in mixture would be useful to confirm the uniformity and additivity of REE toxic effects. In addition, it would be helpful to determine a method normalizing the different data sets in order to compare the results from different studies based on a common basis.
4.4 Environmental risk assessment of REE in freshwater systems
4.4.1 Hazard assessment
In the current study, we assessed REE hazard by performing standard ecotoxicological tests on three species belonging to different trophic levels. We determined REE PNEC based on several criteria:
1) The alga was the most sensitive species towards REE.
2) Contrary to previous ERA based on individual REE (Sneller et al., 2000; González et al., 2015), we addressed REE as a uniform group. We consider that it is more suitable to take into account the mixture for REE hazard assessment because REE occur together in the environment and because different REE have a similar toxicity inducing additive effects in mixture. Risk of combined REE has been assessed in sediment using REE mixture effects probability (Gu et al., 2020). In the current study, we assessed for the first time the risk of REE in the aquatic environment using measured REE mixture effects.
3) Dissolved concentration should be used because it is more reliable as explained in Section 4.1.
4) REE PNEC determination should include DOM because it is ubiquitous and influences REE toxicity. Using mixture EC50[REEdiss]mean obtained in the algal test with DOM (1.468 mg L−1) is less protective than using the one obtained without DOM (0.097 mg L−1). However, we think that it is more reliable because it is likely that the EC50 obtained without DOM does not reflect direct REE toxicity because of the possible REE-phosphate precipitation.
5) Consequently, Min (EC50) corresponds to the mixture EC50[REEdiss]mean obtained in the algal test in the presence of DOM referring to 1.468 mg L−1 (Table 3).
6) We divided Min (EC50) by an AF of 1,000 because the uncertainties in extrapolation from laboratory data to the “real” environment (cf. Section 2.6) are increased when using short-term toxicity data (European Commission, 2003). The resulting PNEC is equal to 1.468 µg L−1. This value is close to the maximum permissible concentration of 1.8 µg L−1 determined for Nd in Dutch freshwaters (Sneller et al., 2000).
4.4.2 Environmental exposure assessment
Concerning REE exposure, we selected in the literature several REE concentrations measured in natural freshwaters (Table 4). Scenarios without identified REE contamination (close to natural background) are represented at different scales: world (Noack et al., 2014), Europe (Salminen et al., 2005) and local (Louis et al., 2020). Remaining scenarios include anthropogenic REE with releases from agricultural (Loic A Martin et al., 2021), urban (Hissler et al., 2016), WWTP (Verplanck et al., 2010; Hissler et al., 2016), mining (Liang et al., 2014) and industrial (Kulaksız and Bau, 2011) activities. Different hydrological conditions were also considered (Louis et al., 2020; Loic A Martin et al., 2021). We used the sum of the concentrations of all REE because they occur altogether in the environment and because we consider REE as a uniform group, as explained before. In addition, we chose measured dissolved (<0.45 or <0.22 µm) concentration because the PNEC is also based on dissolved concentration.
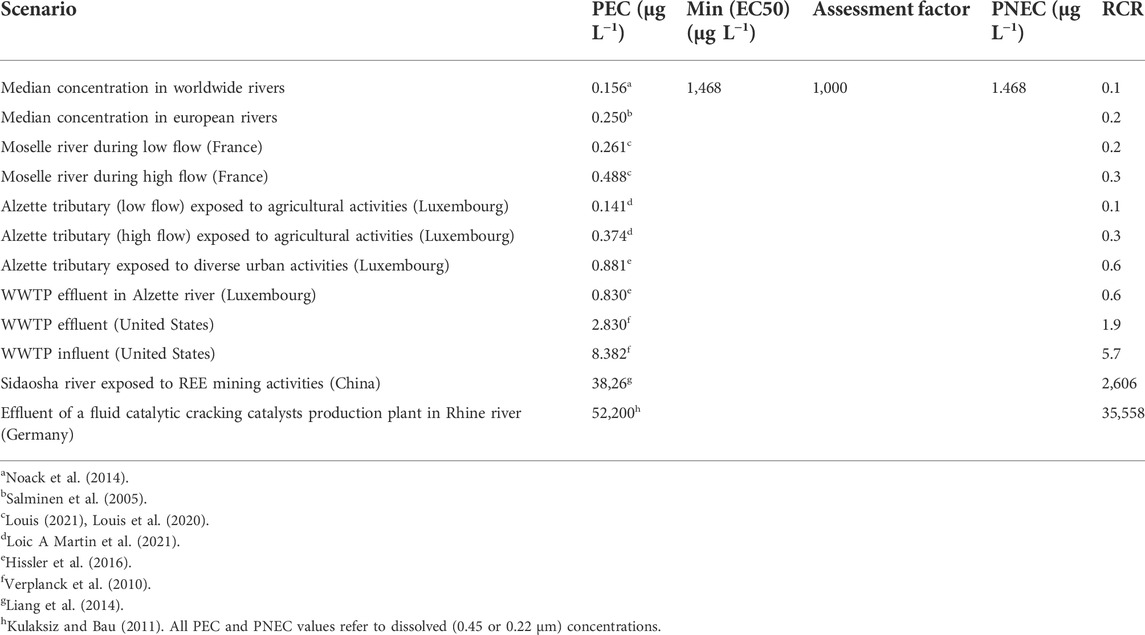
TABLE 4. Environmental risk assessment of REE under different environmental scenarios. PEC, predicted environmental concentration; Min (EC50), minimum EC50 value obtained between algal, crustacean and fish tests; PNEC, predicted no effect concentration = Min (EC50)/assessment factor; RCR, risk characterisation ratio = PEC/PNEC. If RCR <1, the risk is controlled; if RCR >1, the risk is not controlled.
4.4.3 Risk characterisation
We calculated RCR in order to compare REE hazard and REE exposure concentrations (Table 4). The results show that REE do not represent a risk (RCR = 0.1–0.3) in the absence of anthropogenic REE. This was expected because in these scenarios REE concentrations is supposed to be close to the natural background, which is probably tolerated by adapted autochthonous species. Agricultural activities can release REE into aquatic environments via runoff of cultures using REE as fertilizer (Tommasi et al., 2020) or sewage sludge containing REE (most likely source of REE in our scenarios). Risk is controlled for agricultural activities (RCR = 0.1–0.3). It can be explained by the low leaching of REE from sewage sludge (<1%) (Louis, 2021). The risk of REE releases from WWTP, steel plants, waste incinerators and domestic sewage (Hissler et al., 2016) is also considered to be controlled (RCR = 0.6). However, if considering the effluents of another WWTP (Verplanck et al., 2010), there is a potential risk (RCR = 1.9). This shows that the risk should be preferentially assessed at the local scale to avoid misleading global conclusions concerning a source of contamination. It is tricky to say that WWTP influents represent a risk (RCR = 5.7) because aquatic organisms are not directly exposed to them. However, it shows that WWTP treatments can remove a certain amount of REE (a part goes to sewage sludge later used in agriculture) but not enough to avoid the risk due to the effluents. It is important to note here that Gd from MRI contrast-agents represents the main part of REE released by WWTP. It is likely that Gd from MRI should be considered differently in ERA of REE because 1) its specific chemical form can lead to different bioavailability and toxicity and 2) data on Gd contrast–agent ecotoxicology are still limited (Trapasso et al., 2021). The risk is very high (RCR = 2,855) in REE mining areas where REE concentrations commonly exceed the PNEC (Liang et al., 2014; Liu et al., 2019), which can lead to severe damage to exposed freshwater ecosystems. The risk can be also extremely high (RCR = 35,558) for ecosystems exposed to specific industrial effluents (fluid catalytic cracking catalysts production plant) (Kulaksız and Bau, 2011), which represent in this case an extraordinary REE hotspot. Anthropogenic REE releases are the main factor that influence the risk but also natural events such as some hydrological conditions (high flow) can increase, although in a lesser extent, the exposure and the subsequent risk of REE (Table 4).
5 Conclusion
In this study, we aimed to assess REE hazard in close to environmental conditions with the perspective to perform an integrated ERA. For that, we applied ECHA procedures by assessing REE ecotoxicity on the primary producer R. subcapitata (ISO 8692, 2012), the primary consumer D. magna (ISO 6341, 2012) and the secondary consumer D. rerio (OECD, 2010). We tested three representative REE: Nd (LREE), Gd (MREE) and Yb (HREE). In addition to standard test recommendations, we enhanced environmental realism by testing the three REE in mixture and in the presence of DOM because REE occur altogether and DOM is ubiquitous in the environment. REE concentration measurements in the different test media revealed that the real exposure concentrations were significantly lower than the nominal concentrations. We took this difference into account by calculating geometric mean concentrations in order to analyse more properly REE bioaccumulation and toxicity. Bioaccumulation measurements showed that D. magna accumulated more REE than D. rerio. The presence of DOM reduced REE accumulation in both species, probably because of REE-DOM complexation that decreased REE bioavailability. Consequently, REE toxicity to the crustaceans was lower in the presence of DOM. In the algal and crustacean tests, REE exhibited a similar toxicity and additive effects in mixture. Thus, we assumed that REE could be addressed as a uniform group. For the first time, we assessed the risk of REE in aquatic environments in an integrative way, including a REE mixture instead of the more usual and restricted way considering individual REE. EC50 values revealed that the algal species was the most sensitive to REE. Thus, to calculate a PNEC value, we used REE mixture EC50 (based on dissolved concentration) determined in the algal test in the presence of DOM because we considered it is more realistic and reliable. Comparison of PNEC with several PEC corresponding to different exposure scenarios suggest that REE do not represent a risk for freshwater ecosystems in the absence of anthropogenic REE. Currently, the risk induced by REE is limited to the freshwater ecosystems exposed to WWTP, industrial and mining releases. However, the scenarios exhibiting a RCR close to 1 should be monitored with caution because anthropogenic REE releases are expected to increase in the future (Balaram, 2019), which could lead to increased PEC values and the subsequent risk.
It is still tricky to assess the environmental risk of REE considering literature data because of the variability of ecotoxicity results. Potential sources of variability are test medium composition, tested REE chemical form and speciation (Lachaux et al., 2022), among others. In order to take into account these factors when assessing REE ecotoxicity, it would be consistent to express their toxicity values according to their bioavailability as already applied for lead and nickel (European Parliament, 2013). It would allow a homogenisation of REE ecotoxicity data and a more accurate and reliable ERA. Further studies on REE toxic mechanisms in the long-term would be useful to better understand REE toxicity and could help to explain mixture additive effects, which suggest a common mode of action (Borgmann et al., 2005; Crémazy et al., 2013).
Data availability statement
The raw data supporting the conclusion of this article will be made available by the authors, without undue reservation.
Ethics statement
The animal study was reviewed and approved by CELMEA n°66 (Comité Lorrain en Matiére d’Expérimentation Animale) Authorization number: APAFIS#19386-2019031912194883v9.
Author contributions
LG, E-MG, and NL contributed to conception and design of the study. NL performed the experiments, collected and analysed the samples and data and wrote the first draft of the manuscript. CC-L handled and supervised manipulations with fish. LP performed and supervised REE concentration measurements. E-MG supervised and validated the study. LG obtained the funding, administrated the project, supervised and validated the study. All authors contributed to manuscript revision, reading, editing and approved the submitted version.
Funding
This work was supported by the French National Research Agency through the ECOTREE project (ANR-16-CE34-0012) and the national program “Investissements d’avenir” (ANR-10-LABX-21-01/RESSOURCES21) with financial support by the ZAM LTSER Moselle for the thesis of NL.
Acknowledgments
We would like to thank also the Pôle de compétences en biologie environnementale (LIEC–ANATELo–Université de Lorraine–CNRS—http://liec.univ-lorraine.fr) for the technical assistance. We thank Bert-Jan Groenenberg for providing the DOM stock solution.
Conflict of interest
The authors declare that the research was conducted in the absence of any commercial or financial relationships that could be construed as a potential conflict of interest.
Publisher’s note
All claims expressed in this article are solely those of the authors and do not necessarily represent those of their affiliated organizations, or those of the publisher, the editors and the reviewers. Any product that may be evaluated in this article, or claim that may be made by its manufacturer, is not guaranteed or endorsed by the publisher.
Supplementary material
The Supplementary Material for this article can be found online at: https://www.frontiersin.org/articles/10.3389/fenvs.2022.974191/full#supplementary-material
References
Aharchaou, I., Beaubien, C., Campbell, P. G. C., and Fortin, C. (2020). Lanthanum and cerium toxicity to the freshwater green alga Chlorella fusca: Applicability of the biotic ligand model. Environ. Toxicol. Chem. 39, 996–1005. doi:10.1002/etc.4707
Amyot, M., Clayden, M. G., MacMillan, G. A., Perron, T., and Arscott-Gauvin, A. (2017). Fate and trophic transfer of rare earth elements in temperate lake food webs. Environ. Sci. Technol. 51, 6009–6017. doi:10.1021/acs.est.7b00739
Arienzo, M., Ferrara, L., Trifuoggi, M., and Toscanesi, M. (2022). Advances in the fate of rare earth elements, REE, in transitional environments: Coasts and estuaries. Water 14, 401. doi:10.3390/w14030401
Balaram, V. (2019). Rare earth elements: A review of applications, occurrence, exploration, analysis, recycling, and environmental impact. Geosci. Front. 10, 1285–1303. doi:10.1016/j.gsf.2018.12.005
Bau, M., and Dulski, P. (1996). Anthropogenic origin of positive gadolinium anomalies in river waters. Earth Planet. Sci. Lett. 143, 245–255. doi:10.1016/0012-821X(96)00127-6
Bednarska, A. J., Jevtić, D. M., and Laskowski, R. (2013). More ecological ERA: Incorporating natural environmental factors and animal behavior: Environmental Factors and Animal Behavior in ERA. Integr. Environ. Assess. Manag. 9, e39–e46. doi:10.1002/ieam.1444
Belden, J. B., Gilliom, R. J., and Lydy, M. J. (2007). How well can we predict the toxicity of pesticide mixtures to aquatic life? Integr. Environ. Assess. Manag. 3, 364–372. doi:10.1002/ieam.5630030307
Bergsten-Torralba, L. R., Magalhães, D. P., Giese, E. C., Nascimento, C. R. S., Pinho, J. V. A., and Buss, D. F. (2020). Toxicity of three rare earth elements, and their combinations to algae, microcrustaceans, and fungi. Ecotoxicol. Environ. Saf. 201, 110795. doi:10.1016/j.ecoenv.2020.110795
Blaise, C., Gagné, F., Harwood, M., Quinn, B., and Hanana, H. (2018). Ecotoxicity responses of the freshwater cnidarian Hydra attenuata to 11 rare earth elements. Ecotoxicol. Environ. Saf. 163, 486–491. doi:10.1016/j.ecoenv.2018.07.033
Blinova, I., Lukjanova, A., Muna, M., Vija, H., and Kahru, A. (2018). Evaluation of the potential hazard of lanthanides to freshwater microcrustaceans. Sci. Total Environ. 642, 1100–1107. doi:10.1016/j.scitotenv.2018.06.155
Blinova, I., Muna, M., Heinlaan, M., Lukjanova, A., and Kahru, A. (2020). Potential hazard of lanthanides and lanthanide-based nanoparticles to aquatic ecosystems: Data gaps, challenges and future research needs derived from bibliometric analysis. Nanomaterials 10, 328. doi:10.3390/nano10020328
Borgmann, U., Couillard, Y., Doyle, P., and Dixon, D. G. (2005). Toxicity of sixty-three metals and metalloids to Hyalella azteca at two levels of water hardness. Environ. Toxicol. Chem. 24, 641. doi:10.1897/04-177R.1
Broderius, S. J., Kahl, M. D., and Hoglund, M. D. (1995). Use of joint toxic response to define the primary mode of toxic action for diverse industrial organic chemicals. Environ. Toxicol. Chem. 14, 1591–1605. doi:10.1002/etc.5620140920
Cardon, P.-Y., Triffault-Bouchet, G., Caron, A., Rosabal, M., Fortin, C., and Amyot, M. (2019). Toxicity and subcellular fractionation of yttrium in three freshwater organisms: Daphnia magna , Chironomus riparius, and Oncorhynchus mykiss. ACS Omega 4, 13747–13755. doi:10.1021/acsomega.9b01238
Crémazy, A., Campbell, P. G. C., and Fortin, C. (2013). The biotic ligand model can successfully predict the uptake of a trivalent ion by a unicellular alga below pH 6.50 but not above: Possible role of hydroxo-species. Environ. Sci. Technol. 47, 2408–2415. doi:10.1021/es3038388
Cui, J., Zhang, Z., Bai, W., Zhang, L., He, X., Ma, Y., et al. (2012). Effects of rare earth elements La and Yb on the morphological and functional development of zebrafish embryos. J. Environ. Sci. 24, 209–213. doi:10.1016/S1001-0742(11)60755-9
Davranche, M., Gruau, G., Dia, A., Bouhnik-Le Coz, M., Marsac, R., Pédrot, M., et al. (2017). “Rare earth elements in wetlands,” in Trace elements in waterlogged soils and sediments, 135–162.
Dubé, M., Auclair, J., Hanana, H., Turcotte, P., Gagnon, C., and Gagné, F. (2019). Gene expression changes and toxicity of selected rare earth elements in rainbow trout juveniles. Comp. Biochem. Physiology Part C Toxicol. Pharmacol. 223, 88–95. doi:10.1016/j.cbpc.2019.05.009
El-Akl, P., Smith, S., and Wilkinson, K. J. (2015). Linking the chemical speciation of cerium to its bioavailability in water for a freshwater alga: Bioavailability of Ce in water. Environ. Toxicol. Chem. 34, 1711–1719. doi:10.1002/etc.2991
European Chemicals Agency (2016a). Guidance on information requirements and chemical safety assessment Chapter R.16. Helsinki: environmental exposure assessment. ECHA.
European Chemicals Agency (2016b). Guidance on information requirements and chemical safety assessment Part E: Risk characterisation. Helsinki: ECHA.
European Commission (2010). Directive 2010/63/EU of the European Parliament and of the Council of 22 September 2010 on the protection of animals used for scientific purposes (Text with EEA relevance).
European Commission (2011). Communication from the commission to the European parliament, the council, the European economic and social committee and the committee of the regions tackling the challenges in commodity markets and on raw materials (communication No. 52011DC0025). Brussels.
European Parliament (2013). Directive 2013/39/EU of the European Parliament and of the Council of 12 August 2013 amending Directives 2000/60/EC and 2008/105/EC as regards priority substances in the field of water policyText with EEA relevance.
Fujiwara, K., Matsumoto, Y., Kawakami, H., Aoki, M., and Tuzuki, M. (2008). Evaluation of metal toxicity in Chlorella kessleri from the perspective of the periodic table. Bull. Chem. Soc. Jpn. 81, 478–488. doi:10.1246/bcsj.81.478
Gong, B., He, E., Van Gestel, C. A. M., Tang, Y., Yang, W., Yang, J., et al. (2021). Dynamic interaction processes of rare earth metal mixtures in terrestrial organisms interpreted by toxicokinetic and toxicodynamic model. J. Hazard. Mater. 418, 126281. doi:10.1016/j.jhazmat.2021.126281
González, V., Vignati, D. A. L., Pons, M.-N., Montarges-Pelletier, E., Bojic, C., and Giamberini, L. (2015). Lanthanide ecotoxicity: First attempt to measure environmental risk for aquatic organisms. Environ. Pollut. 199, 139–147. doi:10.1016/j.envpol.2015.01.020
Gu, Y.-G., Gao, Y.-P., Huang, H.-H., and Wu, F.-X. (2020). First attempt to assess ecotoxicological risk of fifteen rare earth elements and their mixtures in sediments with diffusive gradients in thin films. Water Res. 185, 116254. doi:10.1016/j.watres.2020.116254
Guida, M., Siciliano, A., and Pagano, G. (2016). “Chapter 6 rare earth element toxicity to marine and freshwater algae,” in Rare earth elements in human and environmental health. Editor G. Pagano (Singapore: Pan Stanford Publishing), 143–154. Penthouse Level, Suntec Tower 3, 8 Temasek Boulevard. doi:10.1201/9781315364735-8
Guo, G., Song, B., Lei, M., and Wang, Y. (2019). Rare earth elements (REEs) in PM 10 and associated health risk from the polymetallic mining region of Nandan County, China. Hum. Ecol. Risk Assess. Int. J. 25, 672–687. doi:10.1080/10807039.2018.1447362
Gwenzi, W., Mangori, L., Danha, C., Chaukura, N., Dunjana, N., and Sanganyado, E. (2018). Sources, behaviour, and environmental and human health risks of high-technology rare earth elements as emerging contaminants. Sci. Total Environ. 636, 299–313. doi:10.1016/j.scitotenv.2018.04.235
Hao, X., Wang, D., Wang, P., Wang, Y., and Zhou, D. (2016). Evaluation of water quality in surface water and shallow groundwater: A case study of a rare earth mining area in southern jiangxi province, China. Environ. Monit. Assess. 188, 24. doi:10.1007/s10661-015-5025-1
Hissler, C., Stille, P., Iffly, J. F., Guignard, C., Chabaux, F., and Pfister, L. (2016). Origin and dynamics of rare earth elements during flood events in contaminated river basins: Sr–Nd–Pb isotopic evidence. Environ. Sci. Technol. 50, 4624–4631. doi:10.1021/acs.est.5b03660
Huang, Z., Gao, N., Zhang, S., Xing, J., and Hou, J. (2022). Investigating the toxically homogenous effects of three lanthanides on zebrafish. Comp. Biochem. Physiology Part C Toxicol. Pharmacol. 253, 109251. doi:10.1016/j.cbpc.2021.109251
International Organization for Standardization (2012a). Qualité de l’eau - essai d’inhibition de la croissance des algues d’eau douce avec des vertes unicellulaires. ISO 8692:2012).
International Organization for Standardization (2012b). Qualité de l’eau - Détermination de l’inhibition de la mobilité de Daphnia magna Straus (Cladocera, Crustacea) - essai de toxicité aiguë. (ISO 6341:2012).
Johannesson, K. H., Lyons, W. B., Stetzenbach, K. J., and Byrne, R. H. (1995). The solubility control of rare earth elements in natural terrestrial waters and the significance of PO43−and CO32−in limiting dissolved rare earth concentrations: A review of recent information. Aquat. Geochem. 1, 157–173. doi:10.1007/BF00702889
Johannesson, K. H., Tang, J., Daniels, J. M., Bounds, W. J., and Burdige, D. J. (2004). Rare earth element concentrations and speciation in organic-rich blackwaters of the Great Dismal Swamp, Virginia, USA. Chem. Geol. 209, 271–294. doi:10.1016/j.chemgeo.2004.06.012
Joonas, E., Aruoja, V., Olli, K., Syvertsen-Wiig, G., Vija, H., and Kahru, A. (2017). Potency of (doped) rare earth oxide particles and their constituent metals to inhibit algal growth and induce direct toxic effects. Sci. Total Environ. 593–594, 478–486. doi:10.1016/j.scitotenv.2017.03.184
Klaver, G., Verheul, M., Bakker, I., Petelet-Giraud, E., and Négrel, P. (2014). Anthropogenic rare earth element in rivers: Gadolinium and lanthanum. Partitioning between the dissolved and particulate phases in the rhine river and spatial propagation through the rhine-meuse delta (The Netherlands). Appl. Geochem. 47, 186–197. doi:10.1016/j.apgeochem.2014.05.020
Kulaksız, S., and Bau, M. (2011). Rare earth elements in the Rhine River, Germany: First case of anthropogenic lanthanum as a dissolved microcontaminant in the hydrosphere. Environ. Int. 37, 973–979. doi:10.1016/j.envint.2011.02.018
Kulaksız, S., and Bau, M. (2013). Anthropogenic dissolved and colloid/nanoparticle-bound samarium, lanthanum and gadolinium in the Rhine River and the impending destruction of the natural rare earth element distribution in rivers. Earth Planet. Sci. Lett. 362, 43–50. doi:10.1016/j.epsl.2012.11.033
Kulkarni, P., Chellam, S., and Fraser, M. P. (2006). Lanthanum and lanthanides in atmospheric fine particles and their apportionment to refinery and petrochemical operations in Houston, TX. Atmos. Environ. 40, 508–520. doi:10.1016/j.atmosenv.2005.09.063
Lachaux, N., Catrouillet, C., Marsac, R., Poirier, L., Pain-Devin, S., Gross, E. M., et al. (2022). Implications of speciation on rare earth element toxicity: A focus on organic matter influence in Daphnia magna standard test. Environ. Pollut. 307, 119554. doi:10.1016/j.envpol.2022.119554
Li, J.-X., Zheng, L., Sun, C.-J., Jiang, F.-H., Yin, X.-F., Chen, J.-H., et al. (2016). Study on ecological and chemical properties of rare earth elements in tropical marine organisms. Chin. J. Anal. Chem. 44, 1539–1546. doi:10.1016/S1872-2040(16)60963-5
Liang, T., Li, K., and Wang, L. (2014). State of rare earth elements in different environmental components in mining areas of China. Environ. Monit. Assess. 186, 1499–1513. doi:10.1007/s10661-013-3469-8
Liu, W.-S., Guo, M.-N., Liu, C., Yuan, M., Chen, X.-T., Huot, H., et al. (2019). Water, sediment and agricultural soil contamination from an ion-adsorption rare earth mining area. Chemosphere 216, 75–83. doi:10.1016/j.chemosphere.2018.10.109
Loewe, S., and Muischnek, H. (1926). Über kombinationswirkungen. Arch. F. Exp. Pathol. U. Pharmakol. 114, 313–326. doi:10.1007/BF01952257
Loic A Martin, L. A., Vignati, D. A. L., and Hissler, C. (2021). Contrasting distribution of REE and yttrium among particulate, colloidal and dissolved fractions during low and high flows in peri-urban and agricultural river systems. Sci. Total Environ. 790, 148207. doi:10.1016/j.scitotenv.2021.148207
Louis, P., Messaoudene, A., Jrad, H., Abdoul-Hamid, B. A., Vignati, D. A. L., and Pons, M.-N. (2020). Understanding Rare Earth Elements concentrations, anomalies and fluxes at the river basin scale: The Moselle River (France) as a case study. Sci. Total Environ. 742, 140619. doi:10.1016/j.scitotenv.2020.140619
Louis, P. (2021). Origine, comportement et devenir des Terres Rares dans les installations de traitements des eaux résiduaires urbaines et les milieux récepteurs associés. Nancy: Université de Lorraine.
Loveridge, A., Smith, D. S., and McGeer, J. C. (2021). Dissolved organic matter mitigates the acute toxicity of thulium to Hyalella azteca but Ca, Mg and Na do not. Arch. Environ. Contam. Toxicol. 81, 637–647. doi:10.1007/s00244-021-00898-0
Lürling, M., and Tolman, Y. (2010). Effects of lanthanum and lanthanum-modified clay on growth, survival and reproduction of Daphnia magna. Water Res. 44, 309–319. doi:10.1016/j.watres.2009.09.034
MacMillan, G. A., Chételat, J., Heath, J. P., Mickpegak, R., and Amyot, M. (2017). Rare earth elements in freshwater, marine, and terrestrial ecosystems in the eastern Canadian Arctic. Environ. Sci. Process. Impacts 19, 1336–1345. doi:10.1039/C7EM00082K
MacMillan, G. A., Clayden, M. G., Chételat, J., Richardson, M. C., Ponton, D. E., Perron, T., et al. (2019). Environmental drivers of rare earth element bioaccumulation in freshwater zooplankton. Environ. Sci. Technol. 53, 1650–1660. doi:10.1021/acs.est.8b05547
Manusadžianas, L., Vitkus, R., Gylytė, B., Cimmperman, R., Džiugelis, M., Karitonas, R., et al. (2020). Ecotoxicity responses of the macrophyte algae nitellopsis obtusa and freshwater Crustacean thamnocephalus platyurus to 12 rare earth elements. Sustainability 12, 7130. doi:10.3390/su12177130
Marsac, R., Davranche, M., Gruau, G., and Dia, A. (2010). Metal loading effect on rare earth element binding to humic acid: Experimental and modelling evidence. Geochimica Cosmochimica Acta 74, 1749–1761. doi:10.1016/j.gca.2009.12.006
Marsac, R., Davranche, M., Gruau, G., Bouhnik-Le Coz, M., and Dia, A. (2011). An improved description of the interactions between rare earth elements and humic acids by modeling: PHREEQC-model VI coupling. Geochimica Cosmochimica Acta 75, 5625–5637. doi:10.1016/j.gca.2011.07.009
Minguez, L., Bureau, R., and Halm-Lemeille, M.-P. (2018). Joint effects of nine antidepressants on Raphidocelis subcapitata and Skeletonema marinoi : A matter of amine functional groups. Aquat. Toxicol. 196, 117–123. doi:10.1016/j.aquatox.2018.01.015
Moermond, C. T. A., Tijink, J., van Wezel, A. P., and Koelmans, A. A. (2001). Distribution, speciation, and bioavailability of lanthanides in the Rhine-Meuse estuary, The Netherlands. Environ. Toxicol. Chem. 20, 1916–1926. doi:10.1002/etc.5620200909
Möller, P., Knappe, A., and Dulski, P. (2014). Seasonal variations of rare earths and yttrium distribution in the lowland Havel River, Germany, by agricultural fertilization and effluents of sewage treatment plants. Appl. Geochem. 41, 62–72. doi:10.1016/j.apgeochem.2013.11.011
Morel, E., Cui, L., Zerges, W., and Wilkinson, K. J. (2021). Mixtures of rare earth elements show antagonistic interactions in Chlamydomonas reinhardtii. Environ. Pollut. 287, 117594. doi:10.1016/j.envpol.2021.117594
Noack, C. W., Dzombak, D. A., and Karamalidis, A. K. (2014). Rare earth element distributions and trends in natural waters with a focus on groundwater. Environ. Sci. Technol. 48, 4317–4326. doi:10.1021/es4053895
Olwenn Martin, O., Scholze, M., Ermler, S., McPhie, J., Bopp, S. K., Kienzler, A., et al. (2021). Ten years of research on synergisms and antagonisms in chemical mixtures: A systematic review and quantitative reappraisal of mixture studies. Environ. Int. 146, 106206. doi:10.1016/j.envint.2020.106206
Organisation for Economic Co-operation and Development (2000). Guidance document on aquatic toxicity testing of difficult substances and mixtures.
Organisation for Economic Co-operation and Development (2010). Short guidance on the threshold approach for acute fish toxicity. Series on testing and assessment No. 126.
Organisation for Economic Co-operation and Development (2019b). Guidance document on aqueous-phase aquatic toxicity testing of difficult test chemicals. Series on testing and assessment No. 23. second edition. ENV/JM/MONO(2000)6/REV1.
Organisation for Economic Co-operation and Development (2019a). OECD guideline for testing of chemicals - fish acute toxicity test (OECD 203:2019).
Pourret, O., Davranche, M., Gruau, G., and Dia, A. (2007). Rare earth elements complexation with humic acid. Chem. Geol. 243, 128–141. doi:10.1016/j.chemgeo.2007.05.018
Qiang, T., Xiao-rong, W., Li-qing, T., and Le-mei, D. (1994). Bioaccumulation of the rare earth elements lanthanum, gadolinium and yttrium in carp (Cyprinus carpio). Environ. Pollut. 85, 345–350. doi:10.1016/0269-7491(94)90057-4
Ritz, C., Baty, F., Streibig, J. C., and Gerhard, D. (2015). Dose-response analysis using R. PLOS ONE 10, e0146021. doi:10.1371/journal.pone.0146021
Rogowska, J., Olkowska, E., Ratajczyk, W., and Wolska, L. (2018). Gadolinium as a new emerging contaminant of aquatic environments: Gadolinium as a new emerging contaminant. Environ. Toxicol. Chem. 37, 1523–1534. doi:10.1002/etc.4116
Romero-Freire, A., Joonas, E., Muna, M., Cossu-Leguille, C., Vignati, D. A. L., and Giamberini, L. (2019). Assessment of the toxic effects of mixtures of three lanthanides (Ce, Gd, Lu) to aquatic biota. Sci. Total Environ. 661, 276–284. doi:10.1016/j.scitotenv.2019.01.155
Rowell, J.-A., Fillion, M.-A., Smith, S., and Wilkinson, K. J. (2018). Determination of the speciation and bioavailability of samarium to Chlamydomonas reinhardtii in the presence of natural organic matter: Speciation and bioavailability of Sm. Environ. Toxicol. Chem. 37, 1623–1631. doi:10.1002/etc.4106
Sadeghi, M., Petrosino, P., Ladenberger, A., Albanese, S., Andersson, M., Morris, G., et al. (2013). Ce, La and Y concentrations in agricultural and grazing-land soils of Europe. J. Geochem. Explor. 133, 202–213. doi:10.1016/j.gexplo.2012.12.007
Salminen, R., Batista, M. J., Bidovec, M., Demetriades, A., De Vivo, B., De Vos, W., et al. (2005). Geochemical atlas of Europe. Part 1 - background information, methodology and maps. Espoo: Geological Survey of Finland.
Siciliano, A., Guida, M., Pagano, G., Trifuoggi, M., Tommasi, F., Lofrano, G., et al. (2021). Cerium, gadolinium, lanthanum, and neodymium effects in simplified acid mine discharges to Raphidocelis subcapitata, Lepidium sativum, and Vicia faba. Sci. Total Environ. 787, 147527. doi:10.1016/j.scitotenv.2021.147527
Sneller, F. E. C., Kalf, D. F., Weltje, L., and Van Wezel, A. P. (2000). Maximum permissible concentrations and negligible concentrations for rare earth elements (REEs). (RIVM report No. 601501 011).
Song, H., Shin, W.-J., Ryu, J.-S., Shin, H. S., Chung, H., and Lee, K.-S. (2017). Anthropogenic rare earth elements and their spatial distributions in the Han River, South Korea. Chemosphere 172, 155–165. doi:10.1016/j.chemosphere.2016.12.135
Sonke, J. E. (2006). Lanthanide−Humic substances complexation. II. Calibration of humic ion-binding model V †. Environ. Sci. Technol. 40, 7481–7487. doi:10.1021/es060490g
Squadrone, S., Brizio, P., Stella, C., Prearo, M., Pastorino, P., Serracca, L., et al. (2016). Presence of trace metals in aquaculture marine ecosystems of the northwestern Mediterranean Sea (Italy). Environ. Pollut. 215, 77–83. doi:10.1016/j.envpol.2016.04.096
Tai, P., Zhao, Q., Su, D., Li, P., and Stagnitti, F. (2010). Biological toxicity of lanthanide elements on algae. Chemosphere 80, 1031–1035. doi:10.1016/j.chemosphere.2010.05.030
Tan, Q.-G., Yang, G., and Wilkinson, K. J. (2017). Biotic ligand model explains the effects of competition but not complexation for Sm biouptake by Chlamydomonas reinhardtii. Chemosphere 168, 426–434. doi:10.1016/j.chemosphere.2016.10.051
Tang, J., and Johannesson, K. H. (2003). Speciation of rare earth elements in natural terrestrial waters: Assessing the role of dissolved organic matter from the modeling approach. Geochimica Cosmochimica Acta 67, 2321–2339. doi:10.1016/S0016-7037(02)01413-8
Thurman, E. M. (1985). Organic geochemistry of natural waters. Dordrecht: Springer Netherlands. doi:10.1007/978-94-009-5095-5
Tommasi, F., Thomas, P. J., Pagano, G., Perono, G. A., Oral, R., Lyons, D. M., et al. (2020). Review of rare earth elements as fertilizers and feed additives: A knowledge gap analysis. Arch. Environ. Contam. Toxicol. 81, 531–540. doi:10.1007/s00244-020-00773-4
Trapasso, G., Chiesa, S., Freitas, R., and Pereira, E. (2021). What do we know about the ecotoxicological implications of the rare earth element gadolinium in aquatic ecosystems? Sci. Total Environ. 781, 146273. doi:10.1016/j.scitotenv.2021.146273
United Nations (2021). in Globally harmonized system of classification and labelling of chemicals (GHS) (New York Geneva: United Nations publication).
van Zomeren, A., and Comans, R. N. J. (2007). Measurement of humic and fulvic acid concentrations and dissolution properties by a rapid batch procedure. Environ. Sci. Technol. 41, 6755–6761. doi:10.1021/es0709223
Verplanck, P. L., Furlong, E. T., Gray, J. L., Phillips, P. J., Wolf, R. E., and Esposito, K. (2010). Evaluating the behavior of gadolinium and other rare earth elements through large metropolitan sewage treatment plants. Environ. Sci. Technol. 44, 3876–3882. doi:10.1021/es903888t
Vukov, O., Smith, D. S., and McGeer, J. C. (2016). Acute dysprosium toxicity to Daphnia pulex and Hyalella azteca and development of the biotic ligand approach. Aquat. Toxicol. 170, 142–151. doi:10.1016/j.aquatox.2015.10.016
Wallace, W., Lee, B., and Luoma, S. (2003). Subcellular compartmentalization of Cd and Zn in two bivalves. I. Significance of metal-sensitive fractions (MSF) and biologically detoxified metal (BDM). Mar. Ecol. Prog. Ser. 249, 183–197. doi:10.3354/meps249183
Weltje, L., Heidenreich, H., Zhu, W., Wolterbeek, H. Th., Korhammer, S., de Goeij, J. J. M., et al. (2002). Lanthanide concentrations in freshwater plants and molluscs, related to those in surface water, pore water and sediment. A case study in The Netherlands. Sci. Total Environ. 286, 191–214. doi:10.1016/S0048-9697(01)00978-0
Yang, X., Yin, D., Sun, H., Wang, X., Dai, L., Chen, Y., et al. (1999). Distribution and bioavailability of rare earth elements in aquatic microcosm. Chemosphere 39, 2443–2450. doi:10.1016/S0045-6535(99)00172-1
Yang, G., Tan, Q.-G., Zhu, L., and Wilkinson, K. J. (2014). The role of complexation and competition in the biouptake of europium by a unicellular alga: Biouptake of rare earth elements. Environ. Toxicol. Chem. 33, 2609–2615. doi:10.1002/etc.2722
Yang, G., and Wilkinson, K. J. (2018). Biouptake of a rare earth metal (Nd) by Chlamydomonas reinhardtii – bioavailability of small organic complexes and role of hardness ions. Environ. Pollut. 243, 263–269. doi:10.1016/j.envpol.2018.08.066
Keywords: rare earth elements (REE), mixture, aquatic ecotoxicology, environmental risk assessment (ERA), dissolved organic matter (DOM), bioaccumulation
Citation: Lachaux N, Cossu-Leguille C, Poirier L, Gross EM and Giamberini L (2022) Integrated environmental risk assessment of rare earth elements mixture on aquatic ecosystems. Front. Environ. Sci. 10:974191. doi: 10.3389/fenvs.2022.974191
Received: 20 June 2022; Accepted: 11 August 2022;
Published: 06 September 2022.
Edited by:
Karen H. Johannesson, University of Massachusetts Boston, United StatesReviewed by:
Helen Poynton, University of Massachusetts Boston, United StatesCamilla Della Torre, University of Milan, Italy
Copyright © 2022 Lachaux, Cossu-Leguille, Poirier, Gross and Giamberini. This is an open-access article distributed under the terms of the Creative Commons Attribution License (CC BY). The use, distribution or reproduction in other forums is permitted, provided the original author(s) and the copyright owner(s) are credited and that the original publication in this journal is cited, in accordance with accepted academic practice. No use, distribution or reproduction is permitted which does not comply with these terms.
*Correspondence: Nicolas Lachaux, bmljb2xhcy5sYWNoYXV4QHVuaXYtbG9ycmFpbmUuZnI=