- 1Institute for Climate and Atmospheric Science, School of Earth and Environment, University of Leeds, Leeds, United Kingdom
- 2Bangladesh Agricultural Research Institute, Gazipur, Bangladesh
- 3Rothamsted Research, Sustainable Soils and Crops, Harpenden, United Kingdom
- 4School of Agriculture and Food Sciences, the University of Queensland, Brisbane, QLD, Australia
- 5Division of Agronomy, Bangladesh Wheat and Maize Research Institute, Dinajpur, Bangladesh
The rice-maize (R-M) system is rapidly expanding in Bangladesh due to its greater suitability for diverse soil types and environments. The present conventional method of cultivating puddled transplanted rice and maize is input-intensive, decreases soil health through intense ploughing, and ultimately reduces farm profitability. There is a need to investigate alternatives. Accordingly, we conducted a replicated 2-year (2020–2021) field study to investigate the effects of conservation agriculture (CA) based tillage and crop establishment (TCE) techniques and residue management practices on the physical, chemical, and biological properties of soil along with crop productivity and the profitability of rice-maize systems in the sandy loam soil of Northwest Bangladesh. Two TCE techniques Puddled transplanted rice (PTR) followed by Conventional tillage maize (CTM) and strip tillage direct-seeded rice (STDSR) followed by strip-tilled maize (STM) were assigned to the main plots and different percentages of crop residue retention (0, 25, and 50% by height) were allocated to the subplots. Results showed that a reduction in bulk density (BD), soil penetration resistance (SPR), and increased soil porosity were associated with STDSR/STM-based scenarios (strip tillage coupled with 25 and 50% residue retention). The soil organic carbon (SOC) fractions, such as dissolved organic C (DOC), light and heavy particulate organic matter C (POM-C), MAOM, and microbial biomass C (MBC) levels in the 0–10 cm layer under ST based treatments were 95, 8, 6, 2 and 45% greater, respectively, compared to CT with no residue treatment. When compared to the CT treatment, the DOC, light POM-C, heavy POM-C, and MAOM in the 10–20 cm layer with ST treatment were 8, 34, 25, 4 and 37% higher, respectively. Residue retention in ST increased average rice, maize, and system yields by 9.2, 14.0, and 14.12%, respectively, when compared to CT. The system gross margin and benefit-cost ratio (BCR) were $1,515 ha−1 and 1.90 under conventional tillage to $1,696 ha−1 and 2.15 under strip-tillage practices. Thus, our study suggests that CA could be an appropriate practice for sustaining soil fertility and crop yield under R-M systems in light-textured soils or other similar soils in Bangladesh.
1 Introduction
The main rice-based cropping system in Bangladesh, termed rice-rice (R-R) is practiced through a monsoon (T. aman) crop in the rainy Kharif season, followed by a winter (Boro) crop during the winter season when irrigation water is available. The area covers about 2306 M ha of land in 2014, 2015 (Nasim et al., 2017). When water is scarce, maize, wheat, potato, vegetables, or other crops are grown instead of Boro to increase profits. Among the cropping systems practiced, rice-wheat (R-W) system are predominant in tropical to subtropical climate areas of the Indo-Gangetic plains (IGP) of Bangladesh, Nepal, India and Pakistan because they serve a significant role in achieving food security and income for rural and urban populations (Chaki A. K. et al., 2021). During the 2000s, the maize area increased considerably, changing from 50 M ha in 2000 to 401 M ha in 2017, 2018 (DAE, 2019). This change is mainly because of the rising demand for maize grain for poultry and fisheries and also for the human diet (Ali et al., 2008; Timsina et al., 2010). This rice-maize (R-M) system occupies approximately 1.31 M ha in Bangladesh, India, and Nepal, explaining their importance in the region (Gathala et al., 2015).
In the north-western part of Bangladesh, farmers experience a delay in maize planting when excessive soil moisture has caused a delay in harvesting the previous rice crop. This happens frequently and any kind of tillage operation is inadvisable until the soil moisture has reduced sufficiently to allow traffic without compaction or slippage. Usually, farmers use conventional tillage, which involves up to 3–5 passes of slow-speed rotary tillage with a two-wheeled, tractor (2WT) driven power tiller. This is the reason why farmers need an additional 2,3 weeks after the rice harvest to carry out tillage operations before planting maize, which significantly delays planting. (Gathala et al., 2015). Therefore, the maize crop is affected by heat stress during the reproductive stage if sown late (Timsina et al., 2010), which may cause a 12–22% yield loss (Ali, 2006). The literature suggests that to minimize the yield gap and achieve the potential yield, maize crops should be planted as soon as possible after the rice harvest (Timsina et al., 2010).
In Bangladesh, a significant amount of soil organic carbon (SOC) has been lost over the last decade (BBS, 2017; Uddin et al., 2019. This is due to a decrease in inherent soil fertility, and poor soil and irrigation management, along with the adoption of inappropriate intensive farming practices such as intensive tillage by a two-wheel tractor driven power tiller for land preparation (Krupnik et al., 2013), use cow dung as a fuel, residue removal and burning practices, which accelerate the physical disruption of soil aggregate and decrease soil organic carbon (SOC) (Gupta Choudhury et al., 2014; Lenka et al., 2015), and microbial activities (Curaqueo et al., 2011).
In the context of delayed planting, heat stress and soil health deterioration, the application of climate-smart agriculture (CSA), for example, conservation agriculture (CA) techniques, which involve minimum disturbance of soil, residue retention/cover crops (Blair et al., 2006), and crop rotation (Parihar et al., 2016), may be especially relevant. With no-till practices or minimum tillage in CA systems, there is little need to prepare the land for planting (FAO, 2001). This could allow early sowing, avoid heat stress, and keep soil moisture (Kucharik, 2006; Marongwe et al., 2012). According to previous research, no-till with crop residue retention has a significant impact on soil erosion control, enhanced soil structure by maintaining soil aggregates (Galdos et al., 2009), minimum oxidation of soil organic matter, reduced runoff and increasing crop productivity (Roose and Barthes, 2001; Erenstein, 2002; Chaki A. K. et al., 2021). The agronomic productivity is increased when 25–50% (1.3–2.5 Mg ha−1) of the entire crop residues are incorporated with a chisel plough (Bahrani et al., 2007). Another finding from Kumawat et al. (2022) who conducted a field experiment with varying amounts of residue retention under CA based maize-chickpea cropping system. They found that the lowest bulk density, higher soil moisture content and soil available nitrogen, phosphorus and organic carbon was recorded in 60 and 90% of crop residue plots compared to no residue retained plots. Vasconcelos et al. (2018) suggested that 6 Mg ha−1 of crop residue would be a good way to prevent soil C loss and keep the soil covered. Furthermore, several studies have suggested that retaining a moderate quantity (50%) of crop residues can increase crop productivity. Under irrigated conditions, a short-term evaluation of applying crop residue at different rates (ranging from 25 to 100%) and with varied tillage techniques showed that applying residues at R100, followed by R75 and R50, significantly enhanced soil organic carbon and wheat grain production (Mirzaei et al., 2021. The benefits of reduced tillage practices can be more productive if optimally combined with crop residue management and mixed-cropping systems (crop rotation diversification). In this context, future research is needed to investigate the effect of crop residue management and different cropping systems on changes in soil parameters, and crop productivity (Asargew et al., 2022).
Crop residue returning, both aboveground or belowground biomass, to the field after harvesting a crop is a globally accepted good practice for improving soil health parameters. To maintain soil quality and ensure sustainability, residue returning must be implemented scientifically. This is because tillage practises, how residue is returned to the soil, and how long it takes, and weather conditions, can have an effect on achieving the maximum benefit from residue retention (Naresh et al., 2021). Examples include Chalise et al. (2019) who reported that mulch retention had a positive impact on soybean yield. Another study was conducted by Krupnik et al. (2014) at two locations in Bangladesh and found inconsistent results; at one location, there was no difference in the tillage system in either year, whereas, in another location, conventional tillage gave a higher yield in the first year but strip-tillage gave higher yields in the second year. So future research is needed to understand the performance of various tillage techniques, such as conventional tillage and no tillage under equal residue retention, in a range of crop, soil, and climatic conditions (Singh et al., 2020). Clearly, given the lack of understanding of these issues, investigation of appropriate tillage with crop establishment methods and straw return in R-M systems is therefore critical for rice and maize production, ensuring food security, and fulfilling the feed demand from livestock, poultry and fish industries in Bangladesh.
Many studies have been conducted separately on rice and maize production systems such as R-R, and R-W systems in Asia, and tillage and nutrient management (Timsina and Connor, 2001), although studies on the R-M systems in South Asia, especially in Bangladesh are still limited (Timsina et al., 2018). To cover this information gap, it is important to investigate the long-term sustainability of R-M system production in Bangladesh using various tillage alternatives. It is hypothesized that Conservation Agriculture (CA) techniques which considered zero, strip, and reduced tillage, crop residue retention, and diversified maize-based crop rotations, improve soil health parameters such as physical, chemical and biological, compared to conventional tillage and the existing dominant R-R, R-W cropping system of the region. Hence, in response to this knowledge gap and to test the hypothesis, the objectives of the present study were to investigate the short-term effects of different tillage practices with residue return on the physical and chemical properties, and biological activity under a rice-maize rotation in sandy loam soil in Bangladesh.
2 Materials and methods
2.1 Site and soil characteristics
The field experiment was conducted at the Agricultural Research Station (ARS), Bangladesh Agricultural Research Institute, Rajbari, Dinajpur during the 2019–20 and 2020–21 seasons of Aman rice (rainy season) and maize in the North-Western part of Bangladesh (Figure 1). The experimental site is located in the Old Himalayan piedmont plain (AEZ 1) (BARC, 2015; FAO/UNDP 1988). The soil of the experimental site is a well-drained sandy loam with pH 6.7, and the initial physical, chemical and biological properties of the soil are given in Table 1.
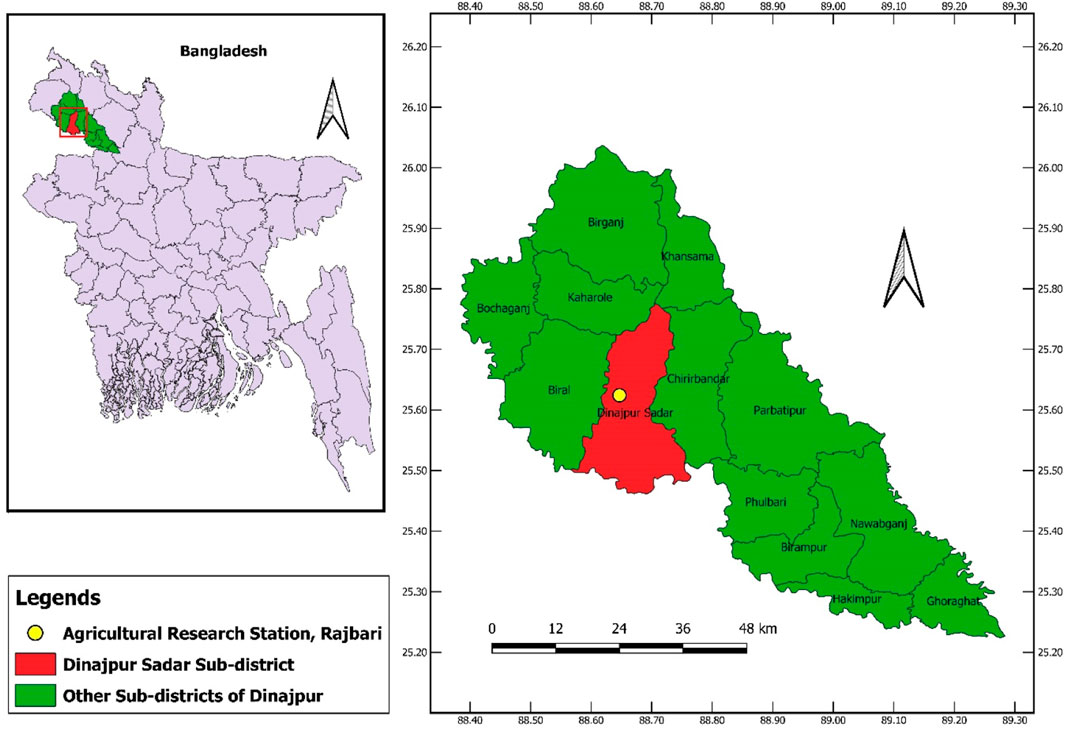
FIGURE 1. The whole green highlighted areas represent the experimental district and the red areas represent the Dinajpur Sadar Sub-district on the map of Bangladesh. The yellow point is the location of the experimental site.
2.2 Climatic characteristics
Figure 2 highlights that during the experimental period the monthly maximum temperatures varied from 22 to 34°C and the minimum temperature from 10 to 27°C at the study site. The average 2 years (2019–21) annual rainfall was 1796 mm and overall, 80% of this fell during the May to October period.
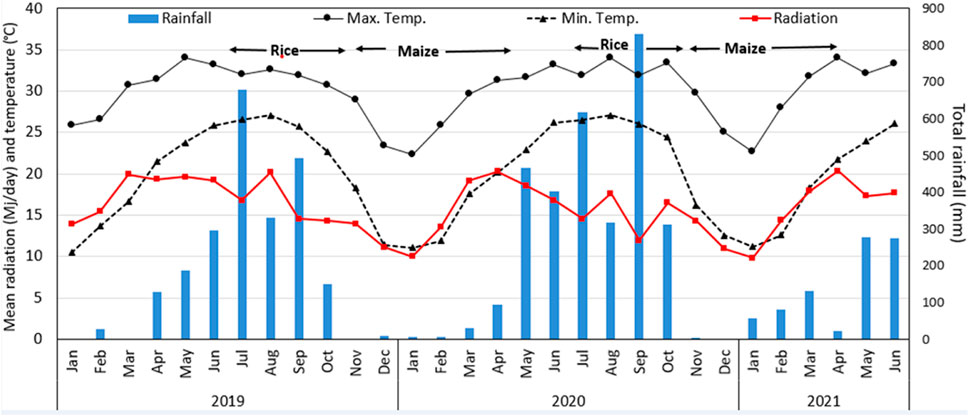
FIGURE 2. Observed rainfall, solar radiation, and maximum and minimum temperatures in the study area.
2.2.1 Rice season
The total rainfall during the rice season (June-November) was 1950 mm in 2019 whereas 2,486 mm in 2020. Total monthly rainfall during June was 297 in 2019 whereas it was 403 mm in 2020 and the total rainfall in July ranged from 618 mm in 2019 to about 680 mm in 2020. June and July rainfall are very important for sowing direct-seeded rice (DSR), whereas rainfall during July is crucial for transplanted rice. The average maximum temperatures from June to November were 29–34°C while the minimum temperatures were 16–27°C.
2.2.2 Maize season
The weather pattern fluctuated across the 2 years. The total amount of rainfall in the winter maize growing season (November-May) was higher in 2020–2021 (743.7 mm) than in 2019–2020 (601.3 mm). Maize is grown during the cool (11–22°C) winter period (Mid-November to the first week of May) and at that time rainfall is very limited. The monthly mean daily maximum temperatures from November to May were 223–340°C while the minimum temperatures were 11.10–20.14°C, respectively.
2.3 Experimental details
The experiment was laid out in a 2-factor split-plot design with three replications. Main plot treatments were puddled transplanted rice (PTR) followed by conventional tillage maize (CTM) and strip tillage direct-seeded rice (STDSR) followed by strip-tilled maize (STM) and the sub-plot treatments were three rice residue management options (0, 25 and 50%) either retained on the soil surface in strip tillage plots or incorporated into the soil in conventional tillage plots. The maize stalks were cut and chopped into 5–10 cm lengths and spread uniformly over the whole plot across the treatments. The treatments in the current study have been discussed details in Table 2.
2.4 Crop management
Twenty-two-day old seedlings were manually transplanted with a spacing of 20 cm × 15 cm and 2,3 seedlings per hill. All DSR plots were sown with zero-till maize/multi-crop planter having an inclined plate seed metering system (model, BMWRI-ZT) with 20 cm row using a 30 kg seeds ha−1. The sowing of DSR and wet bed rice nursery for PTR was done at the same time in the third week of July each year. In CTM plots, maize (BARI Hybrid maize-9) dibbled manually at 20 kg ha−1 maintaining 60 cm × 20 cm plant spacing in the third week of November whereas STM plots were sown by the zero-till maize/multi-crop planter (model, BMWRI-ZT). In the experiment field, rice was fertilized with 54 kg N + 12 kg P + 60 kg K + 9 kg S + 1.2 kg Zn ha−1, while maize received 218 kg N + 76 kg P + 80 kg K + 37 kg S + 11 kg Mg+ 2.1 kg Zn ha−1. No pre-planting herbicides were used in the CT plots, but pyrazosulfuron, a broad-spectrum post-emergence herbicide, was used in the STDSR plots and glyphosate was used in the CTM plots to control weeds.
2.5 Harvesting and yield measurements
Both crops, rice and maize, were harvested at the physiological maturity stage. The rice crop was harvested in DSR plots during the first week of November, whereas PTR plots were harvested in the second week of November in both years. Rice was harvested manually in an area of 3.7 m2 within a field of each plot, following a zig-zag pattern to avoid border effects. For the maize crop, a net plot area of 35 m2 was harvested and the biomass was dried in the field for 3–5 days under the Sun. The rice grain yields were adjusted to a 12% moisture content whereas for the maize grain it was 14%. The dry weight of stubble/straw was recorded after drying at 70°C to a constant weight.
Annual system productivity was determined as rice equivalent yield (REY) by converting the yield of maize crops into rice equivalent yield
The prices of rice and maize used for the calculation were US$ 0.21, and US$ 0.24 kg−1, respectively. The grain prices of all the component crops were determined based on local market prices in BDT and later converted to US$ (1 US$ = 85.00 BDT, the average exchange rate in the experimental period.
2.6 Leaf chlorophyll
For the rice crop, the chlorophyll content was determined by using a chlorophyll meter (SPAD-502, Minolta Camera Co. Ltd., Osaka, Japan) during the vegetative and reproductive phases, a mature leaf being taken from the top of the plant to measure the SPAD values.
2.7 Soil sampling and processing
The soil was collected from the experimental fields before establishing the treatments in 2019, and in 2021 after the harvest of the maize crop in the second year. Briefly, nine representative soil samples were randomly taken from the experimental field at 0–10 and 10–20 cm depths and subsequently composited based on depth for the analysis of soil chemical properties. In addition, another soil sample was collected (from 0 to 10, 10–20, 20–30, 30–40, 40–50, 50–60 and 60–70 cm soil profile) by digging a 100 cm deep soil pit in the experimental site to determine the initial physical and chemical properties of the soil layers. After 2 years of the rice-maize cropping systems, in May 2021 (after the maize harvest), three representative soil samples were collected from each plot at 0–10 and 10–20 cm depths and composited according to depth for the analysis of carbon fraction in each depth. For microbial biomass carbon, soil samples were collected from each plot at 0–5 and 5–10 cm depths. The soil samples were gently sieved through a 4 mm mesh sieve to remove large organic substances. After sieving, the soil samples were passed through a 2 mm sieve and stored in plastic zipper bags at 4°C before microbial biomass carbon analysis. Soil penetration resistance (SPR) was calculated at 0–10 and 10–20 cm depths before starting the experiments and after the end of the experiment in the second year using a Hand Penetrometer (Eijkelkamp Equipment, Model 06.01, and Serial No. 11911698/11, Giesbeek, Netherlands).
2.8 Analytical methods
2.8.1 Soil physical and chemical properties
Organic matter (OM), nitrogen (N), phosphorus (P), potassium (K) and zinc (Zn) were measured following standard procedures (Page et al., 1989). Soil pH was measured with a glass electrode pH meter (WTW pH 522) at a soil-water ratio of 1:2.5 as described by (Page et al., 1982), soil organic C was determined by Walkley and Black’s wet oxidation method as described by Jackson. (1973) and total N was determined by micro-Kjeldahl method (Page et al., 1989); available P was measured following the Olsen method (Jackson, 1973), exchangeable K was quantified following the NH4OAc extraction method (Black, 1965), S was determined by the turbidimetric method through a spectrophotometer using a wavelength of 420 nm (Page et al., 1989). Ca was measured by the complexometric method of titration using Na2-EDTA (Disodium ethylenediaminetetraacetic acid) as a complexing agent (Page et al., 1989), Mg was estimated by using the NH4OAc extraction method (Black, 1965), and available Zn, Cu, Fe, and Mn were measured by using the diethylenetriamine Penta acetic acid (DTPA) extraction method (Lindsay, 1978). Particle size distribution was assessed by the hydrometer method (Bouyoucos, 1962), and the soil textural class was calculated using the USDA textural triangle. Bulk density and particle density of the soil samples were determined by the core sampler method and Pycnometer method, respectively (Karim et al., 1988). The soil porosity was calculated from the relationship between bulk density and particle density
where, BD is bulk density (Mg m−3), PD is particle density (Mg m−3).
2.8.2 Carbon fractionation by size and density
We determined the TOC contents of composite soil samples by the size-density fractionation technique proposed by Robertson et al. (2019). The main goal was to figure out how SOM changes in each of the different soil fractionations. With this approach, four soil fractions were made: DOM (dissolved organic matter), Light POM (particulate organic matter, density, < 1.85 Mg m−3), Heavy POM (heavy particulate organic matter, size >53 µm), and MAOM (mineral-associated organic matter, < 53 µm) (Figure 3). To assess DOM, 10 g of air-dried soil was passed through a 2 mm sieve and placed in a 50 ml centrifuge tube, then 30 ml of deionized water was added and the sample was shaken for 15 min at 95 rpm. After that, the sample was centrifuged for 15 min at 1874 g (calculate rpm for 19.2 cm SoG rotor = 2,876 rpm), and the soil solution was filtered using 20 µm Whatman No 1 filter paper. After that, the sample was analyzed within 48 h by elemental analyser (Vario micro cube CHNOS Elemental Analyzer; EuroEA3000).
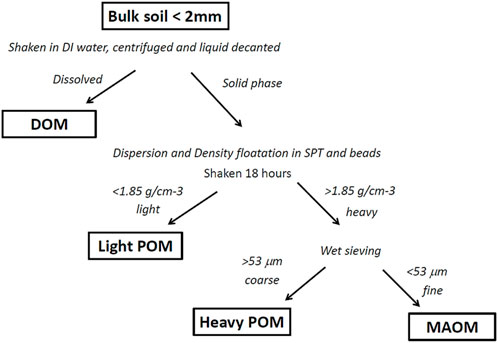
FIGURE 3. Schematic diagram showing SOC fractionation DOM = Dissolved Organic Matter, Light POM = Particulate Organic Matter, density, Heavy POM = Heavy Particulate Organic Matter, and MAOM = Mineral-Associated Organic Matter. (Modified from Robertson et al., 2019).
The light POM technique begins with the first step, following that, the solid material was retained on the filter in the pre-weighed aluminium pan in order to measure the weight of the light fraction. Besides this, 20 ml of sodium polytungstate (SPT) 1.85 Mg m−3was added to a centrifuge tube containing the centrifuged 10 g soil, and shaken for 18 h on a reciprocal shaker at 95 rpm to disperse the sample and then centrifuged for 30 min at 1874 g. It was then collected in the previously weighed aluminium pan and dried at 60°C in the oven. The light POM was then recorded.
For the heavy POM, the procedure was firstly to remove the SPT by repeatedly rinsing the soil with deionized water: deionized water was added (to the 40 ml mark), the sample was shaken to mix it, it was centrifuged, and the water discarded and finally passed onto a 53 µm sieve. To assess MAOM, we collected the sample that has passed through the sieve into a pre-weighed aluminium pan–this was the silt and clay-sized organic matter fraction (MAOM). Finally, we put 10 mg of each of the ground solid fractions (Light POM, Heavy POM, and MAOM) into 9 × 5 mm silver capsules, added 30 µl of 15% hydrochloric acid, and oven-dried them at 60°C, and the samples were analyzed in the elemental analyser (Vario micro cube CHNOS Elemental Analyzer; EuroEA3000). The soil organic carbon (SOC) stock was calculated according to the following equation (Batjes, 1996):
SOC stock (Mg ha−1) = SOC concentrations (%)
The carbon stock computed in different fractions considering amount of visible piece of degraded plant material in every fraction as well as % of SOC concentrations.
2.8.3 Microbial biomass carbon (MBC)
The chloroform fumigation extraction method was adopted to estimate the amount of microbial biomass C in soils. Fumigated and non-fumigated soils were extracted with 0.5 M K2SO4 (soil: K2SO4 solution = 1:4) and shaken for 30 min and then, filtered. From the extract, the amount of biomass C was determined according to the method described by Vance et al. (1987).
2.9 Economic analysis
Partial economic analysis under a range of tillage practices and residue retention levels was computed based on the production costs and income from the sale of rice and maize grain, and rice stubble and maize stover. The production costs involved input costs, machinery costs, and labour used for the experiment. The cost of seed, growing the seedlings, fertilizers, insecticides, herbicides, and irrigation was considered as input costs; whereas machinery costs included a multi-crop planter and power tiller hired for tillage and seed sowing. The labour costs involved different operations, e.g., tillage, seedbed preparation, sowing/transplanting, irrigation management, thinning, weeding, harvesting and threshing. Gross returns (GR) were estimated by multiplying grain and straw yield by the price of grain and straw per hectare each year. The net income was calculated by subtracting the total input costs from the gross return and the gross margin was estimated by subtracting the total production cost from the gross return. The benefit-cost ratio (BCR) was computed as the gross return divided by the cost of production. All the prices were converted to US$ based on a conversion rate of 85BDT = 1 US$ (www.xe.com).
2.10 Statistical analysis
All data were analyzed statistically using a two-way factorial model based on a split-plot design (Popat and Banakara, 2020). In our study, as all the data were normally distributed (p > 0.05), they were exposed to parametric tests. The variables of the effects of different treatments were tested by analysis of variance (ANOVA), and comparisons between the treatments based on the least significant difference at p ≤ 0.05. Before doing statistical analysis, the normality assumption of analysis of variance (ANOVA) was tested by Shapiro and Wilk (1965) by R Core Team (2020) and STAR statistical software (Biometrics and Breeding Informatics, PBGB Division, International Rice Research Institute, Los Baños, Laguna). In addition, the Conformity of homogeneity of variance was also tested by Bartlett’s test (Snedecor and Cochran, 1989). Since the normality assumption of ANOVA was met, there was no need for data transformation. The effect of the treatment PTR/CTM vs. STDSR/STM was compared using t-test for independent samples (using STAR software).
3 Results
3.1 Effect on soil physical properties
3.1.1 Soil bulk density
The effects of TCE and crop residue management practices on soil bulk density (BD) were significant at 0–10 and 10–20 cm profile depths (Table 3). The ANOVA showed that, at 0–10 cm soil depth, the effects of TCE techniques on bulk density was lower by 2.73% in STDSR/STM compared to PTR/CTM. At the same depth, irrespective of residue management practices, the soil bulk density under TCE was lower than with no crop residue retention by 2% (4%) in 25% (50%) crop residue retention plots. On the other hand, in sub-surface soil (10–20 cm), PTR/CTM had a higher value (1.53) than STDSR/STM (1.49) considering TCE techniques. A similar trend was also found concerning residue management practices and a lower value was obtained in 50% crop residue retention treatment (1.49), followed by 25% crop residue (1.51) and no residue retention treatments (1.54). In addition, BD in soils under TCE and crop residue management practices increased with increasing soil profile depths. However, the ANOVA showed no significant interaction effect on TCE and residue management practices on BD in the 0–10 and 10–20 cm soil depths but the value of STDSR/STM with residue incorporation/retention plots declined at both depths.
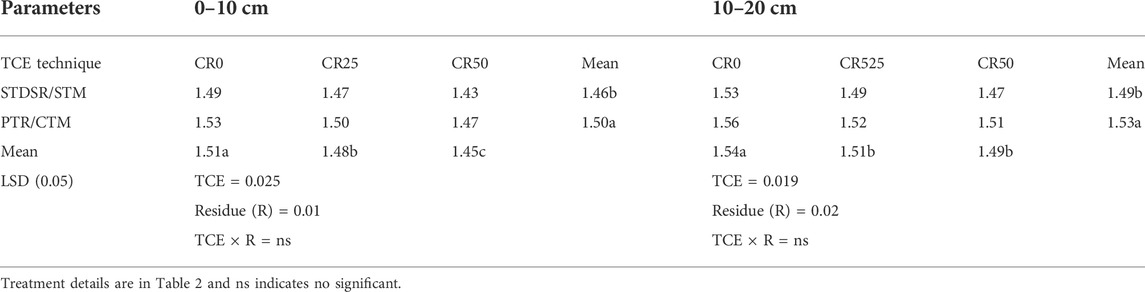
TABLE 3. Soil bulk density (Mg m−3) at two soil depths under different tillage and crop establishment (TCE) techniques and residue management (R) options at the end of 2 years of the rice-maize system.
3.1.2 Soil penetration resistance (SPR)
The main effects of TCE techniques and residue management were significant on SPR at 0–10 and 10–20 cm soil depths (Figure 4). SPR showed a tendency to increase at a depth of 0–10 cm and was always higher in PTR (333 kpa) than in DSR (ST) systems considering tillage practices. Furthermore, retention of residue caused a significant reduction in SPR compared to the residue removal plots, and the maximum SPR (366.01 kpa) was obtained in no crop residue retention followed by 25% crop residue retention (283.50 kpa). The lowest SPR (243.33 kpa) was recorded in 50% crop residue retention. At the same depth, irrespective of conventional and strip tillage with residue management practices, a significant effect on SPR was found in no and 50% crop residue retention, respectively. At a 10–20 cm depth, TCE techniques showed no significant effect on SPR, whereas a 16% reduction in SPR was recorded in STDSR/STM compared to PTR/CTM. At the same depth, mean SPR under 25 and 50% crop residue retention in STDSR/STM plots compared to no crop residue retention in PTR/CTM plot was reduced by 24 and 29%, respectively. In addition, at the same depth SPR values were 13 and 17% lower in 25 and 50% crop residue retention plots compared to no crop residue retention plots. The changes in SPR were positively correlated with BD at both depths. In our study, there was no significant differences between conventional and strip tillage at a 10–20 cm soil depth. Our study also found that 61% variation in SPR could be explained through BD; SPR = 1,452.3BD - 1854.2, R2 = 0.61***, p ≤ 0.001.
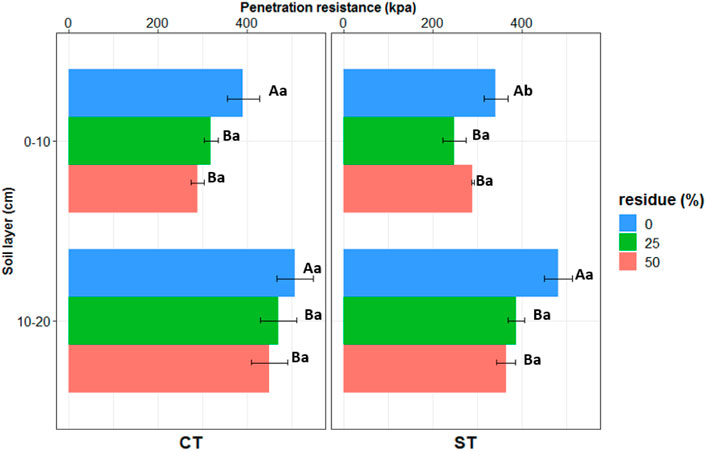
FIGURE 4. Effect of tillage and crop establishment (TCE) techniques and residue management (R) options on soil penetration resistance (KPa) at 0–10 and 10–20 cm profile depths at maize harvest in 2020–21. For treatment, details refer to Table 2. Different upper-case letters represent significant intragroup statistical difference at p ≤ 0.05; Different lower-case letters represent significant intergroup statistical difference at p ≤ 0.05; p values calculated by the ANOVA test and t-test.
In comparison to two tillage methods with the same amount of crop residue retention, the mean SPR values in PTR/CTM plots were 14–46% higher under 0, 25, and 50% crop residue retention compared to STDSR/STM plots at 0–10 cm soil depth and by 23–26% at 10–20 cm soil depth (Figure 4).
3.1.3 Soil moisture content (SMC)
The ANOVA showed no significant interaction effect of TCE and residue management practices on soil moisture content (SMC) in the 0–10 and 10–20 cm soil depths. But the effects of residue management on SMC were, however significant at 0–10 cm and 10–20 cm profile depths. At a 0–10 cm depth, SMC increased by 7 and 17% under 25 and 50% crop residue retention plots compared to no residue plots. Besides, the effects of TCE techniques on SMC were not significant but it was increased by 10% in STDSR/STM compared to PTR/CTM (Supplementary Table S1). At a 10–20 cm depth, SMC was higher by 34% in STDSR/STM compared to PTR/CTM plots. At the same depth, SMC values were 8 and 15% higher in 25 and 50% crop residue retention plots compared to no crop residue retention plots. There was a significant effect on SMC in the STDSR/STM and PTR/CTM plots, irrespective of residue management at 10–20 cm depth only (Figure 5). In our study, SMC was inversely correlated to SPR in both soil depths (r = −0.51, p ≤ 0.01). When comparing two tillage systems with the same level of crop residue retention, the mean SMC values were 6–11% higher in STDSR/STM plots than in PTR/CTM plots at 0–10 cm soil depth and 32–35% higher at 10–20 cm soil depth (Figure 5).
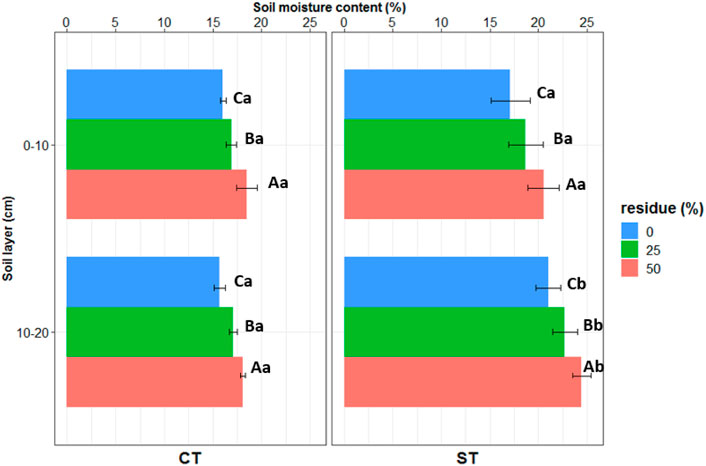
FIGURE 5. Effect of tillage and crop establishment (TCE) techniques and residue management (R) options on soil moisture content (SMC) at 0–10 and 10–20 cm profile depths at maize harvest in 2020–21. For treatment, details refer to Table 2. Different upper-case letters represent significant intragroup statistical difference at p ≤ 0.05; Different lower-case letters represent significant intergroup statistical difference at p ≤ 0.05; p values calculated by the ANOVA test and t-test.
3.1.4 Soil porosity
The effect of residue management practices was significant at both depths. At the 0–10 cm depth, the porosity value was higher by 9 and 18 in 25 and 50% crop residue retention plots compared to no crop residue retention plots. On the other hand, in sub-surface soil (10–20 cm) a similar trend was also observed and the value was 8 and 17% greater under 25 and 50% crop residue retention treatments compared to no residue retained/incorporation treatments. As compared to the conventional and strip tillage with residue management practices, there was a significant effect on soil porosity in the 50% crop residue retention plots at a 10–20 cm depth only (Figure 6). There was no interaction effect of TCE and residue management practices on soil porosity at 0–10 and 10–20 cm profile depths but the value was higher in the SDSR/STM plots (Supplementary Table S2). Mean soil porosity values were 11–45% higher under no 25 and 50% crop residue retention in STDSR/STM plots compared to PTR/CTM plots at 0–10 cm soil depth and by 11–12% at 10–20 cm soil depth.
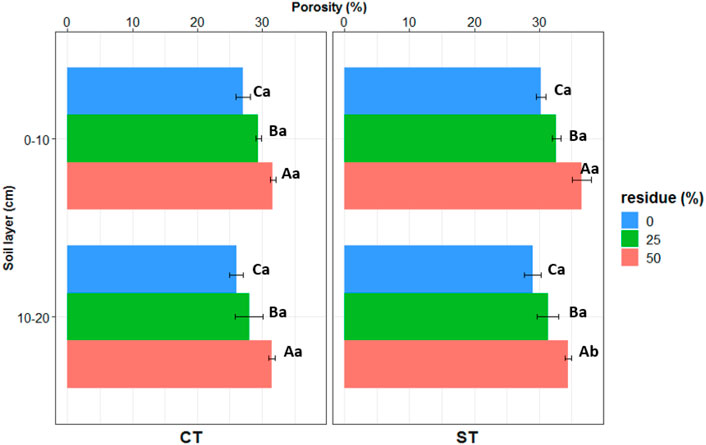
FIGURE 6. Effect of tillage and crop establishment (TCE) techniques and residue management (R) options on soil porosity at 0–10 and 10–20 cm profile depths. For treatment, details refer to Table 2. Different upper-case letters represent significant intragroup statistical difference at p ≤ 0.05; Different lower-case letters represent significant intergroup statistical difference at p ≤ 0.05; p values calculated by the ANOVA test and t-test.
3.2 Carbon fractionation
3.2.1. Dissolved organic carbon
In the present research, tillage management practises had the greatest influence on dissolved organic carbon (DOC) content (Table 4). The amounts of DOC were significantly higher in STDSR/STM management plots (38.73 mg kg−1) compared to no residue retention plots (19.69 mg kg−1) at a 0–10 cm depth. Irrespective of residue and tillage interaction plots, the value was higher in STDSR/STM practices under 25 and 50% residue retention/incorporation plots. Similarly, at 10–20 cm depth, a higher value (64%) was obtained in STDSR/STM practices compared to no residue retention practices. Considering, residue management practices, in both of the years, a higher value was observed in 25 and 50% residue retention/incorporation plots than no residue retention plots (Table 4).
3.2.2 Carbon stock in fractionation contributed by light, heavy POM and MAOM
The amounts of carbon in light POM present in the 50% residue retention/incorporation plots was 25% higher compared to no residue retained plots However, irrespective of residue level, the trend was higher in STDSR/STM plots at both depths. In sub-surface soil at a depth of 10–20 cm, the proportion of carbon provided by light POM was 28.7% higher in 50% residue plots than in residue removal plots (Figure 7).
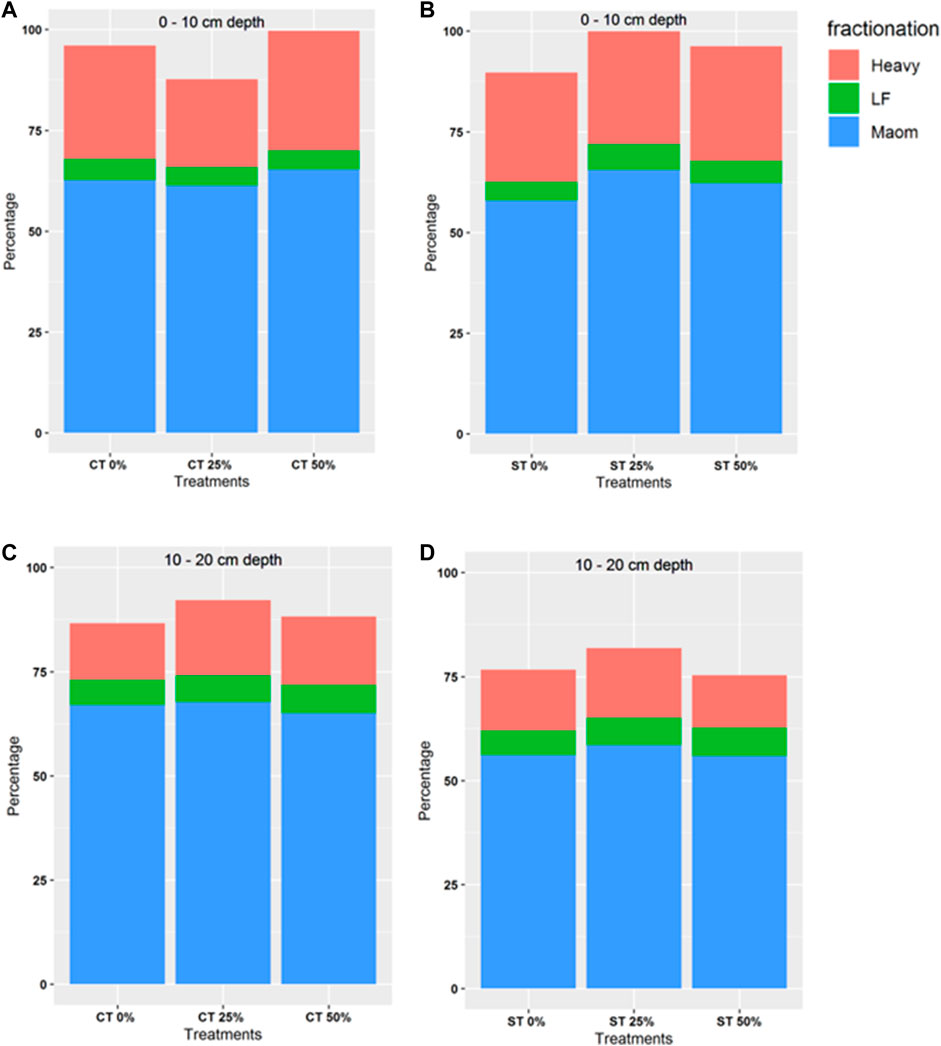
FIGURE 7. The proportion of carbon contributed by light POM, heavy POM and MAOM at 0–10 (A,B) and 10–20 (C,D) cm soil depths.
Although no significant variation was observed in tillage practices, the value was 10% higher in strip tillage compared to conventional tillage practices. For the carbon stock in light POM, 50% crop residue gave better results compared to other treatments, as demonstrated by the highest amounts of SOC compared with the other residue management practices at a 10–20 cm depth (p ≤ 0.05) (Figure 8). However, irrespective of residue levels, there was no significant difference but the value was 10% higher in strip-tillage than in conventional tillage. The interaction effect between tillage and residue management practices was not significant but greater values were observed in the STDSR/STM plots (Supplementary Table S3).
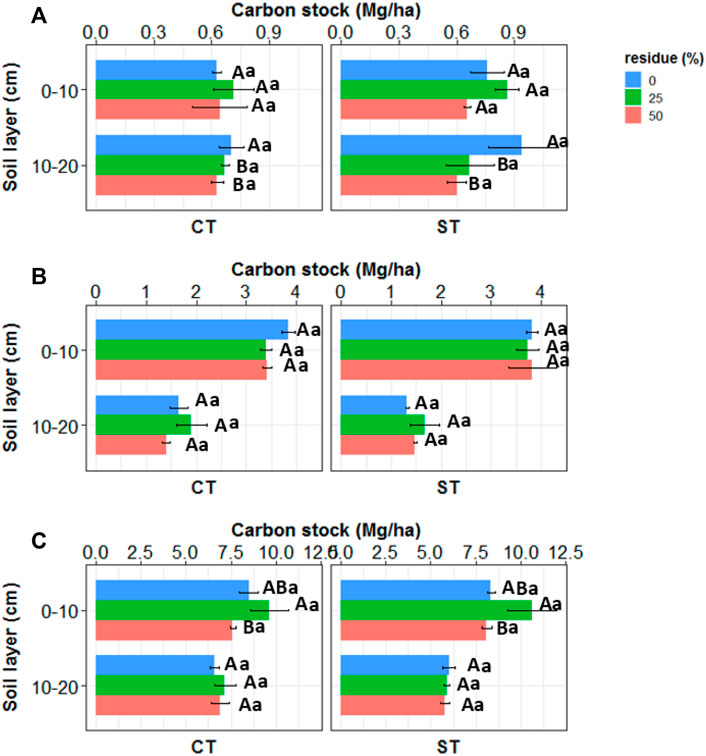
FIGURE 8. Carbon stock in fractionation contributed by light POM (A), heavy POM (B) and MAOM (C) at 0–10 and 10–20 cm profile depths at maize harvest in 2020–21. For treatment, details refer to Table 2. Different upper-case letters represent significant intragroup statistical difference at p ≤ 0.05; Different lower-case letters represent significant intergroup statistical difference at p ≤ 0.05; p values calculated by the ANOVA test and t-test.
Our study showed that the SOC was higher in surface soil compared to the subsurface soil (Figure 8 and Supplementary Table S4) and a higher value was found in 25 and 50% residue plots than in no residue plots. The proportion of carbon contributed by heavy POM was 29% in surface soil and 14% in subsurface soil and the value was higher in 50% residue plots than in residue removal plots (Figure 7). We did not find any significant difference between tillage and residue interaction effect (Supplementary Table S4).
The effect of residue management practices was significant in surface soil. At the 0–10 cm depth, the carbon stock was higher by 27 and 8% in 25 and 50% crop residue retention plots compared to no crop residue retention plots (Figure 8). On the other hand, a similar trend was also observed in sub-surface soil (10–20 cm). Irrespective of tillage management practices, the higher carbon stock value was found in STDSR/STM plots (9.03 Mg ha−1) compared to PTR/CTM plots (8.59 Mg ha-1). The percentage of SOC stock that resided in the MAOM fraction and the value was 64 and 71% in 25 and 50% residue retention plots compared to the no residue plots (60%) (Figure 7). The ANOVA showed that there were no significant interaction effects between tillage and crop establishment (TCE) technique with residue management practices on carbon stock at 0–10 and 10–20 cm profile depths. But the value was higher in strip tillage with 50% residue retention plots compared to conventional tillage with no residue plots (Supplementary Table S5).
3.3 Effect of tillage and residue management on microbial biomass carbon (MBC)
3.3.1 Microbial biomass carbon (MBC)
The MBC was significantly higher under residue retention compared to no residue retention at both of the depths. At the depth of 0–5 cm, retention of 25 and 50% residues resulted in 45–54% higher MBC than no residue retention, whereas 37–41% was found at the 5–10 cm depth. Overall, there was a tendency for the values to be lower at the 5–10 cm depth. Moreover, we did not get an interaction effect (p ≤ 0.05) with tillage and residue practices on microbial biomass carbon (Supplementary Table S6). The result showed that there was no significant difference between two tillage systems with the same level of crop residue retention on MBC but the mean MBC values were 4–17% higher in STDSR/STM plots than in PTR/CTM plots for 25 and 50% crop residue retention at 0–5 cm soil depth (Figure 9).
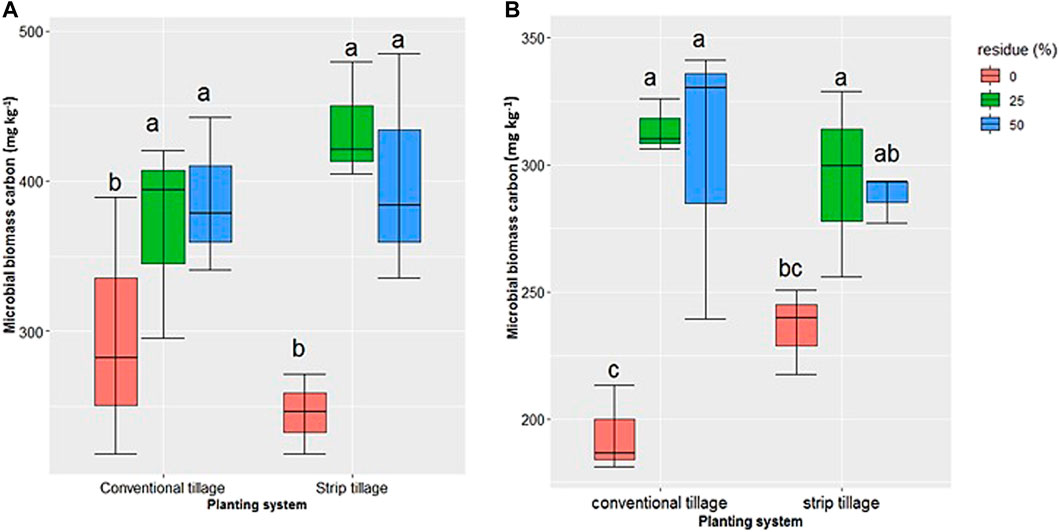
FIGURE 9. Allocation of microbial biomass at 0–5 cm (A) and 5–10 cm (B) depths as influenced by residue retention, and tillage-based crop establishment practices after 2-year of rice-maize system.
3.4 Physiological parameters
3.4.1 Chlorophyll concentration
3.4.1.1 Rice
In the first year, there was no significant variation in SPAD under crop establishment (TCE) techniques and residue management practices in different rice stages; 50, 60, 70, and 80 days after sowing (DAS) as shown in (Supplementary Table S7). However, higher SPAD values were observed in 60 DAS and the value was lower in 80 DAS. In the second year, the value was higher in 25 and 50% crop residue retention plots than in the 0% residue retention plots (Supplementary Table S8). The SPAD values decreased and varied from 38.7 to 31.4 (relative content of chlorophyll) in the no residue retention practices.
3.4.1.2 Maize
In the first year of our study, there was significant variation in the effects of residue management practices in 90 DAS as shown in Supplementary Table S9. The maximum SPAD value (61.20) was recorded in 50% crop residue retention plots followed by 25% crop residue retention (59.39). On the other hand, the lowest values were found in no residue retention practice plots (57.56). Similarly, in the second year, the effects of TCE techniques and residue management practices were significant in 90 DAS and the maximum values were obtained in ST (57.26) rather than in PTR systems (56.30), as shown in (Supplementary Table S10). Irrespective of residue management practices, 50%, and 25% of crop residue retention plots showed higher values (57.38 and 56.03) compared to the no residue plots. However, in both the years, the interaction effects of crop establishment (TCE) techniques and residue management practices were non-significant (p ≤ 0.05) during the growing period of maize but STM gave a higher value than residue removal plots.
3.5 The effect of tillage and residue management on yield component and cropping system
3.5.1 Yield and yield parameter of rice and maize
In the first year, there was no significant TCE × residue management interaction effect on the yield and contributing characters of rice. In our study (tiller/hill and panicle length) of rice were significantly affected by TCE techniques. However, PTR (CT) had 8 and 2% higher values in relation to panicle density and panicle length (cm) than DSR (ST) plots. Considering the yield, the trend was higher in PTR plots than in ST plots (Supplementary Table S11). Similarly, in the second year ANOVA also showed no significant interaction effects residue × TCE techniques on rice yield. The main effects were observed in tillage management practices, the yield being 14% higher in PTR compared to DSR (ST) plots. In both seasons, the biomass yield followed a similar trend to grain yield (Supplementary Table S12).
For maize, during 2019, 2020, the main effects of TCE practices were plant height and thousand-grain weight while other effects from residue management were recorded from grains/cob, 1,000 grain weight, cob length, cob line, and cob round. A decrease in plant height and weight of 1,000 grain weight in the order of 3 and 5% were observed in CTM after PTR compared to STM after STDSR. Retention of crop residues increases the grains cob−1 by 3 and 4%, 1,000 grain weight by 5 and 9%, cob length (cm) by 9 and 21%, in the case of 25 and 50% crop residue retention rather than no residue retention plots. The highest maize yield was recorded from 50% crop residue retention (9.14 Mg ha−1) followed by 25% residue retention (8.85 Mg ha−1), and the lowest was found from no residue treatment (8.37 Mg ha−1), although there was no significant effect of the TCE practices on maize yield (Supplementary Table S13). In 2020, 2021, the trend of cobs plant−1, grains/cob, weight of 1,000 grains, cob length, cob line, and cob round were similar to the previous maize crop. However, there was an increase in grains/cob (3 and 6%), the weight of 1,000 grains (4 and 8%), cob length (7 and 19%), cob round (2 and 6%) in relation to 25 and 50% crop residue retention compared to no residue retention plots. The trend in maize yield was similar to the previous season for TCE and residue management practices, and there was also a higher yield of 10 and 14% under 25 and 50% residue retention compared to no residue management practices (Supplementary Table S14). In both seasons, the biomass yields also followed a similar trend to grain yield. The present study did not find any significant interaction effect between tillage and residue practice but the yield was higher in STM plots compared to CTM plots.
3.5.2 Rice-maize system productivity
The total rice equivalent yield (REY) of the R-M system ranged from 9.56 to 10.46 Mg ha−1 in the first year, while in the second year, it was 9.91–11.31 Mg ha−1. Residue retention/incorporation practices resulted in consistently higher REY yields across the years. However, the highest system productivity was obtained from 50% crop residue retention practices (10.45 Mg ha−1in year 1, 11.31 Mg ha−1 in year 2) followed by 25% crop residue retention management (10.16, 10.92 Mg ha−1). The lowest REY was recorded from no residue retention practices in year 1 and year 2 (9.56 and 9.91 Mg ha−1). The incremental decline in REY was significant when crop residue was removed, with no significant difference when compared with the TCE technique. Overall, in year 1, the REY was higher at 5 and 9% under 25 and 50% residue retention compared to no residue management practices while it was 10 and 14% in year 2 (Table 5).
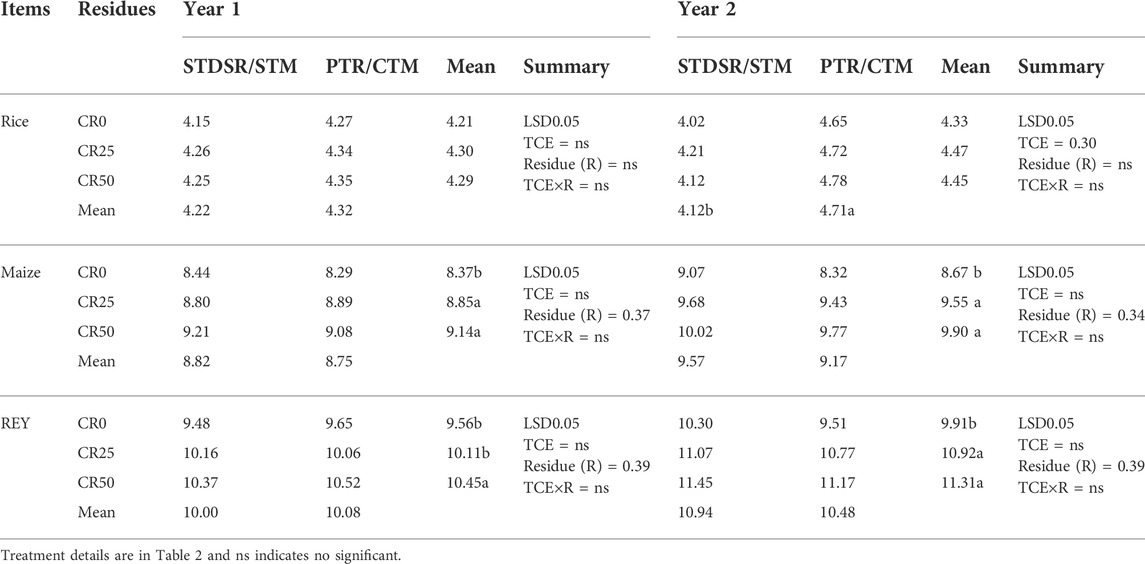
TABLE 5. Rice and maize grain yields and rice equivalent yield (REY) (Mg ha−1) in Rice-Maize cropping systems during 2019–2020 and 20–21.
3.6 Gross margin analysis
In 2019, 2020, the effect of residue management was significant in relation to production costs, gross return, gross margin, and BCR but in the TCE technique (tillage practices) only production costs were significant in the RM system. The production costs were US$216 higher for PTR/CTM compared to STDSR/STM (Figure 10). Irrespective of residue retention, the production cost was highest (US$1,557) in CR0 while it was lowest (US$1,527) in CR50. Total gross return, gross margin, and benefit-cost ratio (BCR) are also highest in 50% crop residue retention treatments and lowest for no crop residue retention plots.
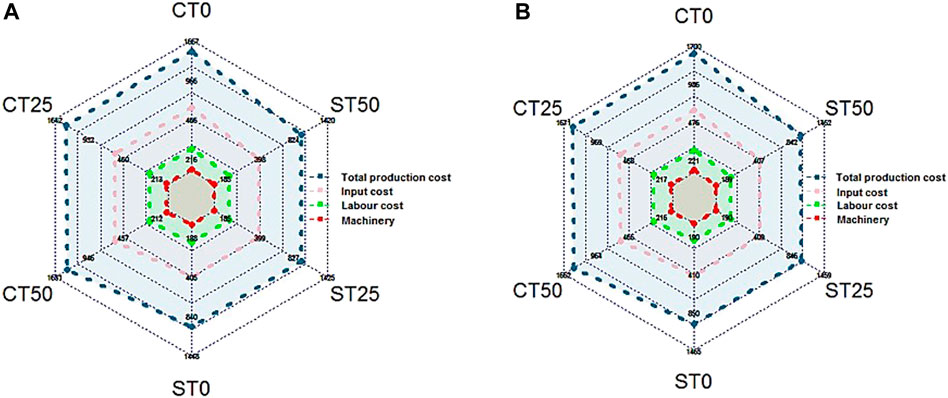
FIGURE 10. Cost of production under TCE techniques with residue management options for rice and maize system in northwest Bangladesh (CT0 = conventional tillage with no residue; CT25 = conventional tillage with 25% residue; CT50 = conventional tillage with 50% residue; ST0 = Strip tillage with no residue; ST25 = Strip tillage with 25% residue; ST50 = Strip tillage with 50% residue during 2019–2020 (A) and 2020–2021(B). Numbers in each spider diagram mention amount in US$.
In 2021, 2022, there was significant variation in the main effects of TCE techniques and residue management practices relating to production costs, gross margin, and BCR in the RM system. The highest production cost (US$1,678) was found from PTR/CTM and the STDSR/STM gave the lowest production cost (US$1,462), as shown in (Figure 10). In the R-M system, the highest gross margin (US$1,696) and BCR (2.15) were also recorded from STDSR/STM and the lowest was from the PTR/CT system (Table 6). The main effect of residue retention refers to gross return, gross margin, and BCR following the same trend in the previous year.
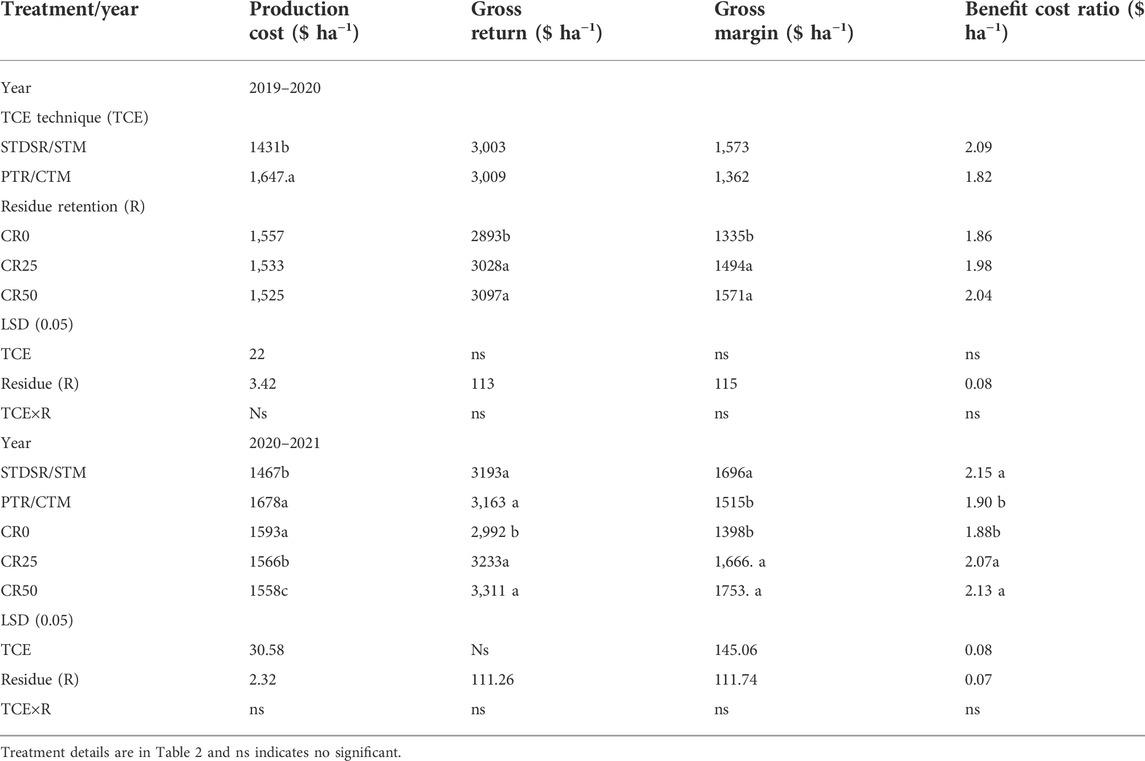
TABLE 6. Economics of different crop establishment techniques and residue management options in rice-maize cropping system during 2019–2020 and 2020–21.
4 Discussion
4.1 The effect of tillage and residue management on soil physical properties
Soil penetration resistance (SPR) values were significantly lower in DSR (ST) than under PTR (CT) systems. Further, our results also showed an increase in SPR with an increase in soil depth. The key interpretation is that a higher SPR under PTR was related with a higher bulk density (especially at 10–20 cm depth) in these plots. This agrees with the results reported by Singh et al. (2016), who conducted an experiment in sandy loam soil with three tillage and two residue management options in the R-M system in north-west India and found that SPR showed an increasing trend with an increase in soil depth and was always higher in PTR (CT) compared to DSR (ZT) systems. Salahin et al. (2021) carried out a 3-year study in the Gangetic Plains of Bangladesh to evaluate the effects of zero tillage (ZT), strip tillage (ST), bed planting (BP), and conventional tillage (CT) with two residue retention levels. They found that the soil penetration resistance of the ST system was lower than that of the CT system. Lampurlanés and Cantero-Martínez (2003) also reported that increasing trend in SPR with an increase in soil depth. The higher SPR value in CT plots may be also associated with the development of plough pan at a 10–20 cm depth (Singh et al., 2013).
Irrespective of residue management practices, SPR was consistently lower in residue incorporation/retention plots compared to removal residue plots CT at 0–10, and 10–20 cm soil depths. The beneficial effect of incorporation/retention residue on PR is supported by Saha et al. (2010), who found that increased residue incorporation/retention reduced soil PR at a 0–15 cm depth on sandy loam soil. In a study in Uttar Pradesh, India, the continuous application of 5 Mg ha−1 crop residues for 5 years in R-M systems decreased the SPR value by 23–31% over no residue plots (Singh et al., 2016). However, it is important to bear in mind that SPR is directly correlated to BD and inversely related to soil water content (Sharma and De Datta, 1986) and in our study, SPR also closely followed BD and soil moisture content trends. Jat et al. (2009) also reported that SPR had a greater value under puddling compared to ZT/conservation tillage.
The interaction effect of TCE techniques and residue on SPR was not significant in the present study. Similar observations were also reported by Singh et al. (2013), who conducted a long-term experiment to assess the effects of three tillage systems, no tillage (NT), ridge-tillage (RT) and plough tillage (PT), and three mulch rates (no residue, 8, and 16 Mg ha−1 yr−1 and reported that the interaction between tillage, mulch, and soil depth was not significant on SPR.
The present study demonstrated that the TCE technique’s influence on bulk density and the value were lower in STDSR/STM compared to PTR/CTM at both depths. The main explanation is that puddling in rice crops is known to destroy soil aggregates and increase compaction of the soil (Gathala et al., 2011a). Our research also observed that strip tillage in DSR had a lower value compared to PTR (CT) treatment plots. This study is also in line with another researcher Singh et al. (2016) who observed that crop residue retention under DSR plots decreased soil bulk density compared to conventional tillage. In order to assess the impacts of zero tillage (ZT), strip tillage (ST), bed planting (BP), and conventional tillage (CT) with two residue retention levels, Salahin et al. (2021) conducted a 3-year research in the Gangetic Plains of Bangladesh. They discovered that the CT system had a larger bulk density near the soil surface than the ST system. According to a 4-year study in India by Gathala et al. (2011a), the CT-based system tends to have higher soil bulk density near the soil surface (10–20 cm soil depths) than the CA system.
In contrast, other researchers have found that higher bulk density under ZT at a 0–5 cm depth compared to CT in different cropping systems than R-M system (Wu et al., 1992; Gathala et al., 2011a; Huang et al., 2012; Jat et al., 2013). In our study, soil bulk density varied significantly due to residue management practices which is similar to the findings of He et al. (2009) who observed that crop residue retention under no-tillage practices decreased soil bulk density. The decreasing trend is strongly correlated with the deposition of organic matter and greater soil biological activity in ST practice (Alam et al., 2014). Moreover, Lal (2000) found that the incorporation of 16 Mg ha−1 of rice residue for 3 years decreased BD from 1.20 to 0.98 Mg m−3 on sandy loam soil. Coinciding with this result, Salahin et al. (2021) observed that soil BD did not significantly vary due to crop residue incorporation/retention practices. Sokolowski et al. (2020) observed that no-tillage practices increased soil BD compared to tillage systems (mouldboard plough) although the study was conducted in clay loam soil in an area with heavy rainfall. The present study also indicated that the interaction effects of TCE and crop residue management had no significant effect on soil BD. The findings is also confirmed by Singh et al. (2013) which reported that the interactive effect of tillage and mulch practices on soil BD was not significant in wheat crops. Our findings also differ from the results of Singh et al. (2016), who found interaction effects on the BD value at 0–15 cm and 15–30 cm depths.
Our study showed that strip tillage with residue retention/incorporation generated higher soil moisture content at all depths than in no residue plots. This is because residue retention in strip tillage maintaining favourable soil temperature by changing soil energy balances and heat fluxes (Kozak et al., 2007; Abdullah, 2014). In addition to this, another argument is that no residue with conventional tillage often creates the land unprotected from extreme temperature which in turn leads to a decrease in the amount of moisture contained in the soil (Ward et al., 2013). These findings are similar to Zhao et al. (2020) who conducted a meta-analysis to evaluate changes in SMC (soil moisture content), looking at CR retention in China (considering 278 publications), and observed that CRR (crop residue retention) led to an increase in SMC by 5.9% compared to CR removal. The present study also showed that higher SMC was found in strip tillage with residue retention compared to convention tillage with residue incorporation. The positive effect of retention residue on SMC is substantiated by Bhattacharyya et al. (2013), who performed a 6-years field experiment on sandy loam soil comparing various tillage methods with residues incorporated or retained on the soil surface. They discovered that areas where agricultural residues were retained on the surface included much more water-stable macroaggregates, which contributed to a higher SMC. Another example from Verhulst et al. (2011) who found that SMC was higher in residue retained plot due to less evaporation, which has an effect on SMC. A global meta-analysis by Li et al. (2019) indicated that CA-based management strategies increased accessible water by 10.2% higher than conventional practises. Many researchers agree that CA methods, such as strip tillage with the mulching effect of CR, are advantageous (Stewart et al., 2018; Lu, 2020). Other researchers Kader et al. (2017) also found that straw mulching helped to conserve soil moisture at a 0–30 cm depth and reduced soil temperature. The main explanation might be linked to lower soil temperatures and lower evaporation from residue retention plots (Busari et al., 2013). However, the trend of increased SMC owing to CA management practices is highly dependent on the regional climate (Abdallah et al., 2021). For example, Gathala et al. (2020) conducted a study in Bangladesh, Nepal and India and observed that water productivity was increased by 19% by adopting CA practices in the subtropical region. Consequently, negative outcomes were also found in cold -humid and tropical humid climates, where waterlogging was observed (Abdallah et al., 2021). The current study indicated that the retention of crop residue together with strip-tillage increased SMC compared to CT with the removal of residues. These results are similar to (Song et al., 2016) who found that removal of crop residue through conventional tillage causes soil water loss, thus affecting soil moisture content.
Soil porosity under CT was significantly lower than ST at two soil depths. The main explanation is that puddling in rice crops is known to increase compaction of the soil and ultimately reduces the porosity (Singh et al., 2016). Higher soil porosity under residue retention/incorporation in the plots than in soil with residue removal has been also reported by others (Alam et al., 2014; Alam et al., 2019). Another example from Patra et al. (2019) found that soil porosity was higher in zero tillage than in conventional tillage. These findings also in line with Liu et al. (2005), who observed that the retention of crop residue increased soil porosity when there was minimal tillage in the sub-surface layer. The increase of soil porosity in ST might be due to the addition of organic matter and crop residues which was the result of minimum soil disturbance (Alam et al., 2014). In contrast, Sasal et al. (2006) observed that total porosity was 3.5% higher in conventional tillage practices than in ZT-based practices in the surface soil layer (0–15 cm). Another example from Tangyuan et al. (2009) found that the total soil porosity was most affected in the surface layer rather than the sub-surface layer.
4.2 The effect of tillage and residue management on soil organic carbon fraction
The SOC fractions, like dissolved organic C, microbial biomass C and particulate organic matter C are known as a soil quality indicator parameter (Liu et al., 2014; Dong et al., 2009; Saviozzi et al., 2001; Lenka et al., 2015; Yang et al., 2005). As a relatively mobile fraction of the SOC, DOC plays an important role in the transport of nutrients, such as nitrogen and phosphorus (Kaiser, 2003). The current study revealed that the DOC in the 10–20 cm depth was higher in the TCE technique (ST) as compared to the CT soil. The strong stratification of the DOC at the 10–20 cm layer of the ST soil, due to receiving higher rainfall during the crop growing periods that may increase the downward movement of DOC to the deeper layer of soil. This is in line with Roy et al. (2022), who observed that surface drip irrigation increased the moist soil environment which is closely associated with a downward movement of DOC to a deeper layer. In coarse texture soil, a lower amount of clay content also contributed to this process (Gmach et al., 2019). Our results also showed that strip-tillage with direct-seeded rice plots have higher DOC, as compared to CT practices. The fundamental reason is that CT methods expose SOC to air, leading to increased organic carbon oxidation, whereas reducing tillage management practices favour organic carbon build-up under zero or reduced tillage (Zhao et al., 2015).
The study also observed a higher SOC stock in light POM in higher residue retention plots. It might be due to the mixing of crop stubbles and roots with soil which ultimately results in higher SOC stock. Our findings corroborate those of many other researchers (Blanco-Canqui and Lal, 2007; Liang et al., 2007; Nobuhisa and Hiroyuki, 2009). Liu et al. (2014) reported that improved crop management practices, such as no-tillage and residue retention/incorporation practices, often lead to an increase in SOC and SOC fractions compared to CT. Another example from Chivenge et al. (2007), found that higher SOC was obtained from sandy soils in plots where mulch ripping with residue retention was practiced compared with plots where clean ripping was carried out with no residue retention.
In heavy POM, the beneficial effect of strip tillage and crop residue retention/incorporation on SOC stock was recorded only in the 0–10 cm soil layer, but not in lower layers (Figure 8). These findings are also similar to Luo et al. (2010) who observed that SOC stock was higher in ZT plots only in the upper surface layer (0–10 cm), but decreased by 3.30 ± 1.61 Mg ha−1 at a lower depth (20–40 cm) over CT practices. Roy et al. (2022) also reported that higher SOC stock was observed at the surface layer followed by lower SOC stock in the subsurface soil layer in CA-based practices compared to conventional practices. Based on a short-term study (2-year trial) in Bangladesh, Chaki AK. et al. (2021) discovered that the CA-based system tended to have greater soil TOC near the soil surface (0–5 cm and 5–15 cm soil depths) than the CT system. Moreover, This was also well supported by Zeng et al. (2021) who recorded a higher SOC in the top layer of soils than in the sub-layer soils. The key management difference across the treatments that could explain the greater SOC stock in the STDSR/CMT was the addition of residue of 4.2 Mg ha−1 year−1 (Figure 8 and Supplementary Table S15). According to Bhattacharyya et al. (2015), CA practices boosted both SOC content and stock as compared to CT. Similarly, the residue retention plot produced a much larger SOC stock (6 Mg/ha/year), according to (Ranaivoson et al., 2017).
Crop residue can play an important role to increasing and/or maintaining SOC levels in the soil profile although its effect may be influenced by how residue is kept in the soil, e.g., residue surface retention vs incorporation (Turmel et al., 2015). When comparing two tillage systems with the same level of crop residue retention, the study observed that SOC stock was higher under strip tillage with residue retention compared to conventional tillage with the incorporation of residue in the plot at the surface layer. The main explanation is that crop residue on the surface under strip tillage involves less interaction with soil microorganisms (Salinas-Garcia et al., 2001), and therefore decomposition is more gradual than CT, where residue comes into close contact with microorganisms when mixed in the soil (Reicosky et al., 1997). This finding is in line with Kuswaha et al. (2001) who conducted an experiment in India between residue retention vs. incorporation, and found that SOC is higher under minimal tillage with the residue retained in plots compared to incorporation in plots. Our findings are in agreement with other researchers who found higher SOC content in no tillage than reduced tillage (Singh et al., 2020). In contrast, Dong et al. (2009) conducted an experiment in Northern China and found that SOC content was higher in CT with residue incorporation treatment but the study was conducted in silt loamy soil. Moreover, Turmel et al. (2015) reported that there was a significant increase in SOC content in the CT with residue treatment plots. However, it is well understood that incorporating crop residues into the soil improves soil aeration, and temperature, and creates favourable conditions for microorganisms, resulting in higher decomposition rates and ultimately SOC loss (Coppens et al., 2007; Fontaine et al., 2007) particularly in sub-humid temperate to sub-humid tropical regions (Turmel et al., 2015). At 30°C, the rate of SOC mineralization can increase by up to 72–177%, according to Ghimire et al. (2019). In addition, Moldboard ploughing is generally shown to decrease C stocks in the soil (Turmel et al., 2015). However, our study confirmed that combining strip tillage with residue retention, either complete or partial on the surface, is more helpful than removing the residue entirely.
Because of the inconsistency of the findings of SOC in the soil under tillage and residue management practices, it is, therefore, recommended that the whole soil profile should be studied rather than shallow sampling (Vanden Bygaart and Angers, 2006). This will help to provide more accurate information on the effects of residue management practices on SOC in the soil (Baker et al., 2007).
4.3 The effect of tillage and residue management on microbial biomass carbon (MBC)
Soil microbiological indicators like MBC are influenced by land management practices and environmental changes (Zhao et al., 2018)Our study showed that strip tillage with residue retention/incorporation generated higher microbial biomass carbon (MBC) at all depths than in no residue retention plots. This is because the addition of residue increased soil organic carbon (Saurabh et al., 2021) and this gradually increased with increasing quantities of residue return, which ultimately promote soil MBC (Zhao et al., 2018). Another explanation for higher MBC could be that the residue provides readily mineralisable and hydrolysable carbon for better microbial growth (Samal et al., 2017). Moreover, the incorporation of crop residues into the soil may have a beneficial effect on endogeic (horizontal-burrowing) earthworms because it will act as a food source (Wuest et al., 2005). This is consistent with our observation that it influences the total organic C pool, due to changes in C supplied by crop residues, and ultimately that is reflected in the microbial biomass (Franzluebbers et al., 1999). The present study also observed that higher MBC were found on the surface than in the subsurface layers. The possible reason for this may be the lesser availability of crop residue at a lower soil depth. Moreover, another reason is that zero tillage with residue retention on the topsoil makes the soil cooler and wetter, resulting in lower fluctuations in moisture and temperature (Kaldivgo, 2001) and ultimately encouraging microbial substrates as well as higher MBC (Luna-guidoet al, 2007). Our findings are in agreement with many other researchers (Zhao et al., 2018; Chen et al., 2020). In addition, a reduction in the loss of SOC and a uniform supply of carbon from crop residues act as a source of energy for microorganisms (Kumar and Babalad, 2018). Our study also suggested that there was no significant effect between TCE techniques in relation to MBC. This result was confirmed by other research (Luna-guido et al., 2007) which found that zero tillage on its own does not provide higher MBC compared to zero tillage with residue retained. When comparing two tillage systems with the same level of crop residue retention, the study observed that MBC was higher under strip tillage with residue retention compared to conventional tillage with the incorporation of residue at the surface layer. This may be because microbial biomass was closely connected to the distribution of SOC and the amount of moisture in the soil (Doran, 1987; Salinas-Garcia et al., 2001).
4.4 The effect of tillage and residue management on physiological properties
The concentration of chlorophyll in the leaf is an important indicator that can be used to determine soil N supply to growing plants during the growing season (Mupangwa et al., 2020).
Our study showed that strip tillage with residue retention/incorporation generated higher SPAD values than in no residue retention plots. A higher SPAD value in the second year might be partly due to higher rainfall and the residue conserving the moisture. This finding is in line with other researchers (Liu and Wiatrak, 2012) who concluded that there was no significant difference between different tillage systems but found that the value was higher in the season with the highest rainfall. Other findings, for example, Najafinezhad et al. (2015) reported that drought stress reduced the concentration of leaf chlorophyll (SPAD value) by 5.21% compared to normal irrigation. Moreover, Shefazadeh et al. (2012) also found that the chlorophyll concentration in wheat leaves was highly correlated with the soil’s moisture status. Reductions in chlorophyll might be due to the production of ROS (reactive oxygen species) under oxidative stress which ultimately leads to the degradation of chlorophyll pigments (Sairam and Srivastava, 2002). A decrease in the chlorophyll content was also reported in other crops when the supply of water and nitrogen was limited (Lauer and Boyer 1992; Paknejad et al., 2007; Massacci et al., 2008). In maize, our study showed that the retention of residue caused a significant increase in SPAD values compared to the removal of residue from the plots. These findings are also similar to Najafinezhad et al. (2015) who found that drought stress decreased total chlorophyll by 12.46% in the corn crop. The increase in SPAD value in the residue retention plots may be associated with the increase of moisture retention in the soil, resulting in the prevention of oxidative stress effects, and ultimately, it helps to overcome the harmful influences of drought stress on chlorophyll (Najafinezhad et al., 2015). An increase in chlorophyll content in barley crops by using 4.5 t ha−1 residue has been reported by Najafinezhad et al. (2015) as well as in ridge tillage with mulching in winter wheat crops (Li et al., 2018).
4.5 The effect of tillage and residue recycling on crop yields and the cropping system
The results of the study demonstrated that STDSR gave lower yield compared to PTR. The lower yield of STDSR was associated with a lower number of panicles and reduced panicle length compared to PTR. Other possible reasons for a lower yield in DSR compared to PTR could be, 1) micronutrient deficiency (Fe and Zn) due to aerobic conditions (Singh et al., 2016) 2) increased weed and insect infestation (Gathala et al., 2011a) 3) moisture deficiency due to higher infiltration rates (Singh et al., 2016) and 4) the high plant density of DSR needs more mineral nutrients than PTR (Schnier et al., 1990). These findings are similar to Chaki AK. et al. (2021) who found that the mean decrease in rice yield was 0.83 t ha−1 in zero tillage in comparison with PTR in light-textured soil. Similarly, Rashid et al. (2018), who conducted an experiment in light-textured soil in southern Bangladesh, also recorded a 3, 4% lower yield in ZT compared to PTR. In contrast, other studies conducted by Haque et al. (2016); Islam et al. (2019) and Saharawat et al. (2010) also compared the performance of PTR and ZT UPTR with fully irrigated conditions, where the ZT UPTR produced a higher yield than or similar yield to PTR. Therefore, there is a need to assess the dynamics of macro-and micronutrients in the soil to achieve an optimum rice yield when PTR is replaced by DSR. Although the DSR plots gave a lower yield, the shorter time the crops spent in the field in DSR (as DSR plots were harvested 7–10 days earlier than puddled transplanted rice (Saharawat et al., 2010) might create an opportunity for the timely planting of successional maize crops. However, despite the lower yields in DSR than in PTR, an aerobic rice system requires low input (water, labour, and fuel) (Farooq et al., 2011; Kumar and Ladha, 2011).
In our study, the TCE techniques with rice residue retention/incorporation of either 25% or 50% gave a higher maize yield compared to the removal of all residue from the plots. Our findings agree with Rashid et al. (2019) who concluded that compared with full straw removal, 50% straw retention increased the grain yield of maize by 5%, and Singh et al. (2016) who also reported that the maize yield under a ZTDSR/ZTM + R system was higher by 4.0 and 14.2% than CTDSR/CTM and PTR/CTM. The higher maize yield under residue retention/incorporation practices might be due to the utilization of mineral N by microorganisms, and in later seasons, increased the efficiency of available N uptake by nutrient recycling (Jat et al., 2012; Alam et al., 2020). Moreover, the higher soil moisture concentrations under ST with residue retention practices are also likely to have contributed to the higher grain and biomass yield compared to conventional tillage practices (Asargew et al., 2022). In addition, the yield was increased in STM after DSR possibly due to avoiding puddling in rice (Hobbs et al., 2002; Gathala et al., 2011b; Jat et al., 2014), and the fact that the role of crop residues correlated with reducing the adverse impact of terminal heat stress during the reproductive phase (April and May), and provided an optimum soil thermal regime (STR), coupled with better root growth (Singh et al., 2016). Puddling (wet tillage) in rice forms a hard plough pan, increases the bulk density, disturbs the soil structure, fills the macropores with finer soil particles as well as reducing porosity and increasing soil compaction, which adversely affects upland crops (Sharma et al., 2003; Gathala et al., 2011a). These results are also consistent with the findings of another researcher, Singh et al. (2016) who recorded a higher yield in ZT (zero tilled) maize compared to that in CT plots. However, the meta-analysis conducted by Sun et al. (2020) found that semi-arid to humid regions, with 40 ≤ HI < 100, are good for CA-practices and have the potential to enhance SOC in soil (humidity index, “HI” (average rainfall/mean air temperature). The R-M system productivity (rice equivalent yields) generally followed the increasing trend with time, ranging from 5 to 9% in year 1 to 10–14% in year 2, when crop residue was retained/recycled. These findings are similar to Rashid et al. (2019) who concluded that the highest REY was found from residue retention compared with no retention plots.
4.6 The effect of tillage and residue management on profitability
The current study showed that the cost of production for R-M was higher in CTPTR/CTM compared to STDSR/STM practices due to the high labour and fuel costs of land preparation for maize, the high cost for transplanting rice seedlings and manually seeding maize crops. In our study, CTPTR practices required the highest ($1,647 ha−1) and STDSR/STM required the lowest input costs (∼$1,431 ha−1) in the rice maize system. There could be several explanations for higher production costs in CTPTR including 1) higher labour costs associated with activities such as land preparation, transplanting rice, sowing maize, irrigation etc., under CTPTR/CTM practices, 2) machinery costs, especially for puddling which typically required tilling 4–6 times before transplanting rice and 3, 4 times before for sowing maize. Our findings are in agreement with others (Singh et al., 2014; Gathala et al., 2015; Rashid et al., 2019), who compared input costs, e.g., labour and the machinery required for PTR, and STDSR practices, where STDSR involved lower costs compared to PTR. In the current study, regardless of residue retention, the production cost in residue retention plots was lower than in no residue plots. This may be because crop residue cleanup and transportation on the farm needed more labour and fuel. These findings are similar to those of Sarkar et al. (2020), who observed that removal of crop residues required more labour, effort, and capital. The present study also showed a higher gross return, gross margin, and BCR under strip tillage with residue incorporation/retention than conventional tillage with no residue retention in the plots. This finding is similar to that of other researchers (Gathala et al., 2011b; Laik et al., 2014; Parihar et al., 2016; Rashid et al., 2019). Although such clear benefits were observed from TCE techniques with residue retention/incorporation, but the present study has some limitations for implementing these research findings in farmers’ fields as our analysis is based on data from a research station experiment on a small plot of 35 m2. Our study suggests that economic analysis in the future could be conducted by research station experiments on larger plots.
4.7 Practical applications for climate change mitigation and future research
Given the current climate change issues, the implementation of a CA-based agricultural system is one of the most essential ways to decrease the expected increase of GHG emissions in the atmosphere. Since the CA-based systems improve soil health and organic carbon stocks by fostering soil carbon sequestration by incorporating crop residue and also minimum disturbance of soils. However, CA may not absorb more carbon over time than conventional systems if all CA principles, such as minimum soil disturbance, permanent soil organic cover with crop residues and/or cover crops and crops diversification are not followed properly. The current study revealed that crop residue retention is essential for enhancing soil organic carbon in R-M rotation. Providing incentives to farmers based on carbon footprint/storage and other ecosystem services through residue retention is a viable technique for encouraging the adoption of CA technology in tropical and temperate climatic regions. However, it is recognized that these results represent only 2 years of a field experiment, and a longer period of the study is needed to assess the performance of ZTDSR/ZTM with varying rates of crop residue mulch in R-M systems in diverse soil, climatic, and socio-economic conditions. Besides longer experiments, cropping system simulation studies accounting for the impact of climate change on soil and crop variables might be needed to give greater insights into the long-term impacts of tillage and residue management on sandy soil under R-M systems in Bangladesh.
5 Conclusion
Adoption of conservation agricultural techniques in the study areas has a tremendous effect on the crop profitability of farmers, particularly on sandy loam soils in North-Western Bangladesh. The sustainable intensification practices assessed in this study address the issue of declining soil fertility, especially the decline in organic carbon, microbial biomass carbon and increased soil compaction, etc. We found that strip tillage direct-seeded rice (STDSR), followed by strip-tilled maize (STM) with partial residue retention/incorporation (+R) from both the crops, improved SOC content and the soil’s physical properties, namely soil bulk density, porosity, soil penetration resistance, soil moisture, and other soil and crop parameters, especially microbial biomass carbon and chlorophyll content. Our results showed a decrease in bulk density (4.3–6.9%) and penetration resistance (15.9–30.7%), and an increase in organic carbon (23.6–35.3%), soil moisture content (11.1–21.3%), and porosity (16.1–32.5%) compared to a conventional tillage-based rice-maize rotation in sandy soil. It was also observed that soil biological health, i.e., microbial biomass carbon (4–9%), and physiological parameters like leaf chlorophyll concentration, had significantly improved in STDSR/STM compared to PTR/CTM. Furthermore, puddling in rice with residue removal practices showed a negative impact on soil properties for maize production. The overall improvement in soil conditions resulted in gradually enhanced crop productivity, particularly for maize in ST plots, and improved farm profitability compared to conventionally tilled rice and maize crops. Therefore, to maintain soil health and high crop productivity, residue inputs should be combined with the use of appropriate tillage techniques. However, for organic matter to build up in sandy soil, more emphasis should be put on the addition of organic resources, such as keeping at least 25–50% of crop residues and incorporating them into the soil.
Data availability statement
The original contributions presented in the study are included in the article/Supplementary Material, further inquiries can be directed to the corresponding author.
Author contributions
MS: Conceptualization, Methodology, Analysis, Data curation, Writing- original draft; MG: Supervision, Formal analysis, and editing; AnC: Supervision, Formal analysis, and editing and MH: Conducted experiment and data collection, ApC: Data collection and Visualization and AH: Visualization, and editing.
Funding
The manuscript is a part of a PhD—Research of first author MS; thesis title: Trade-off analysis of crop residue management for improving conservation agriculture practices under a changing climate in Bangladesh. The research was financially supported by the National Agricultural Technology Program (NATP-Phase II), Bangladesh Agricultural Research Council (BARC), Bangladesh and Climate Research Bursary Fund, Priestley International Centre for Climate, University of Leeds, United Kingdom.
Acknowledgments
The authors express their appreciation to the staff of BARI, BWMRI for their cordial help and support during conducting the experiment, data collection, and analysis. We are also thankful to SRDI, Dinajpur for their help in analysing soil samples. We are also grateful to Dr. Md. Khairul Alam, PSO, BARC for his help and support during conducting the experiment, data collection, and analysis. We wish to thank Rachel Gasior, David Ashley, and Holly Armitage for the analytical help in the University of Leeds Geography lab.
Conflict of interest
The authors declare that the research was conducted in the absence of any commercial or financial relationships that could be construed as a potential conflict of interest.
Publisher’s note
All claims expressed in this article are solely those of the authors and do not necessarily represent those of their affiliated organizations, or those of the publisher, the editors and the reviewers. Any product that may be evaluated in this article, or claim that may be made by its manufacturer, is not guaranteed or endorsed by the publisher.
Supplementary material
The Supplementary Material for this article can be found online at: https://www.frontiersin.org/articles/10.3389/fenvs.2022.969819/full#supplementary-material
References
Abdallah, A. M., Jat, H. S., Choudhary, M., Abdelaty, E. F., Sharma, P. C., and Jat, M. L. (2021). Conservation agriculture effects on soil water holding capacity and water-saving varied with management practices and agroecological conditions: A review. Agronomy 11, 1681. doi:10.3390/agronomy11091681
Abdullah, A. S. (2014). Minimum tillage and residue management increase soil water content, soil organic matter and canola seed yield and seed oil content in the semiarid areas of Northern Iraq. Soil Tillage Res. 144, 150–155. doi:10.1016/j.still.2014.07.017
Alam, M. K., Bell, R. W., and Biswas, W. K. (2019). Increases in soil sequestered carbon under conservation agriculture cropping decrease the estimated greenhouse gas emissions of wetland rice using life cycle assessment. J. Clean. Produc. 224, 72–87.
Alam, M. K., Islam, M. M., Salahin, N., and Hasanuzzaman, M. (2014). Effect of tillage practices on soil properties and crop productivity in wheat-mungbean-rice cropping system under subtropical climatic conditions. Sci. World J. 2014, 1–15. doi:10.1155/2014/437283
Alam, M. K., Bell, R. W., Haque, M. E., Islam, M. A., and Kader, M. A. (2020). Soil nitrogen storage and availability to crops are increased by conservation agriculture practices in rice–based cropping systems in the Eastern Gangetic Plains. Field Crops Res. 250, 107764. doi:10.1016/j.fcr.2020.107764
Ali, M. Y. (2006). “Rice-maize systems in Bangladesh,” in Invited Oral Presentation in the Workshop on Assessing the Potential of Rice-Maize Systems in Asia. IRRI-CIMMYT Alliance Program for Intensive Production Systems in Asia, IRRI, Los Baños, Philippines, December 4-8.
Ali, M. Y., Waddington, S. R., Hodson, D., Timsina, J., and Dixon, J. (2008). Maize-rice crop-ping systems in Bangladesh: Status and research opportunities. Mexico: CIMMYT–IRRI Joint Publication, 36.
Asargew, F., Tsunekawa, A., Haregeweyn, N., Adgo, E., Tsubo, M., Ebabu, K., et al. (2022). Tillage and crop management impacts on soil loss and crop yields in northwestern Ethiopia. Int. Soil Water Conservation Res. 10 (1), 75–85. doi:10.1016/j.iswcr.2021.04.006
Bahrani, M. J., Raufat, M. H., and Ghadiri, H. (2007). Influence of wheat residue management on irrigated corn grain production in a reduced tillage system. Soil Tillage Res. 94 (2), 305–309. doi:10.1016/j.still.2006.08.004
Baker, J. M., Ochsner, T. E., Venterea, R. T., and Griffis, T. J. (2007). Tillage and soil carbon sequestration – what do we really know? Agric. Ecosyst. Environ. 118, 1–5. doi:10.1016/j.agee.2006.05.014
BARC (2015). Fertilizer recommendation guide for Bangladesh. Farm Gate, Dhaka, Bangladesh: Bangladesh Agricultural Research Council.
Batjes, N. H. (1996). Total carbon and nitrogen in the soils of the world. Eur. J. Soil Sci. 47, 151–163. doi:10.1111/j.1365-2389.1996.tb01386.x
Bhattacharyya, R., Das, T. K., Sudhishri, S., Dudwal, B., Sharma, A. R., Bhatia, A., et al. (2015). Conservation agriculture effects on soil organic carbon accumulation and crop productivity under a rice–wheat cropping system in the Western Indo-Gangetic Plains. Eur. J. Agron. 70, 11–21. doi:10.1016/j.eja.2015.06.006
Bhattacharyya, R., Tuti, M., Kundu, S., Bisht, J., and Bhatt, J. C. (2013). Conservation tillage impacts on soil aggregation and carbon pools in a sandy clay loam soil of the Indian himalayas. Soil Sci. Soc. Am. J. 76, 617–627. doi:10.2136/sssaj2011.0320
Black, C. A. (1965). Method of soil analysis part-I and II. Madison, Wis, USA: American Society of Agronomy.
Blair, N., Faulkner, R. D., Till, A. R., and Poulton, P. R. (2006). Long-term management impacts on soil C, N and physical fertility. Soil Tillage Res. 91, 30–38. doi:10.1016/j.still.2005.11.002
Blanco-Canqui, H., and Lal, R. (2007). No-Tillage and soil-profile carbon sequestration: An on- farm assessment. Soil Sci. Soc. Am. J. 72, 693–701. doi:10.2136/sssaj2007.0233
Bouyoucos, G. J. (1962). Hydrometer method improved for making particle size analyses of soils 1. Agron. J. 54, 464–465. doi:10.2134/agronj1962.00021962005400050028x
Busari, M. A., Salako, F. K., Tuniz, C., Zuppi, G. M., Stenni, B., Adetunji, M. T., et al. (2013). Estimation of soil water evaporative loss after tillage operation using the stable isotope technique. Int. Agrophys. 27, 257–264. doi:10.2478/v10247-012-0093-8
Chaki, A. K., Gaydon, D. S., Dalal, R. C., Bellotti, W. D., Gathala, M. K., Hossain, A., et al. (2021a). Conservation agriculture enhances the rice-wheat system of the Eastern Gangetic Plains in some environments, but not in others. Field Crops Res. 265, 108109. doi:10.1016/j.fcr.2021.108109
Chaki, A. K., Gaydon, D. S., Dalal, R. C., Bellotti, W. D., Gathala, M. K., Hossain, A., et al. (2021b). Puddled and zero-till unpuddled transplanted rice are each best suited to different environments – an example from two diverse locations in the Eastern Gangetic Plains of Bangladesh. Field Crops Res. 262, 108031. doi:10.1016/j.fcr.2020.108031
Chalise, K. S., Singh, S., Wegner, B. R., Kumar, S., Pérez-Gutiérrez, J. D., Osborne, S. L., et al. (2019). Cover crops and returning residue impact on soil organic carbon, bulk density, penetration resistance, water retention, infiltration, and soybean yield. Agron. J. 111 (1), 99–108. doi:10.2134/agronj2018.03.0213
Chen, H., Dai, Z., Veach, A. M., Zheng, J., Xu, J., and Schadt, C. W. (2020). Global meta-analyses show that conservation tillage practices promote soil fungal and bacterial biomass. Agric. Ecosyst. Environ. 293, 106841. doi:10.1016/j.agee.2020.106841
Chivenge, P., Murwira, H., Giller, K., Mapfumo, P., and Six, J. (2007). Long-term impact of reduced tillage and residue management on soil carbon stabilization: Implications for conservation agriculture on contrasting soils. Soil Tillage Res. 94, 328–337. doi:10.1016/j.still.2006.08.006
Coppens, F., Garnier, P., Findeling, A., Merckx, R., and Recous, S. (2007). Decomposition of mulched versus incorporated crop residues: Modelling with PASTIS clarifies interactions between residue quality and location. Soil Biol. Biochem. 39, 2339–2350. doi:10.1016/j.soilbio.2007.04.005
Curaqueo, G., Barea, J. M., Acevedo, E., Rubio, R., Cornejo, P., and Borie, F. (2011). Effects of different tillage system on arbuscular mycorrhizal fungal propagules and physical properties in a Mediterranean agroecosystem in central Chile. Soil Tillage Res. 113 (1), 11–18. doi:10.1016/j.still.2011.02.004
DAE (2019). Krishi diary. Agricultural information services. Khamar Bari, Dhaka: Ministry of Agriculture, 13.
Dong, W., Hu, C., Chen, S., and Zhang, Y. (2009). Tillage and residue management effects on soil carbon and CO2 emission in a wheat–corn double-cropping system. Nutr. Cycl. Agroecosyst. 83, 27–37. doi:10.1007/s10705-008-9195-x
Doran, W. (1987). Microbial biomass and mineralizable nitrogen distribution in no tillage and plowed soils. Biol. Fertil. Sols 5, 68–75.
Erenstein, O. (2002). Crop residue mulching in tropical and semi tropical countries. An evaluation of residue availability and other technological implications. Soil Tillage Res. 67 (2), 115–133. doi:10.1016/s0167-1987(02)00062-4
FAO/UNDP (1988). Food and agricultural organization/united nations development programme, land resources appraisals of Bangladesh for agricultural development. Rome, FAO: Agro-ecological regions of Bangladesh.
Farooq, M., Siddique, K. H. M., Rehman, H., Aziz, T., Lee, D. J., and Wahid, A. (2011). Rice direct seeding: Experiences, challenges and opportunities. Soil Tillage Res. 111 (2), 87–98. doi:10.1016/j.still.2010.10.008
Fontaine, S., Barot, S., Barre, P., Bdioui, N., Mary, B., and Rumpel, C. (2007). Stability of organic carbon in deep soil layers controlled by fresh carbon supply. Nature 450, 277–280. doi:10.1038/nature06275
Franzluebbers, A., Haney, R., Hons, F., and Zuberer, D. (1999). Assessing biological soil quality with chloroform. Can. J. Soil Sci. 79, 521–528. doi:10.4141/s99-010
Galdos, M., Cerri, C. C., Cerri, E. P., Paustian, K., and Antwerpen, R. (2009). Simulation of sugarcane residue decomposition and aboveground growth. Plant Soil 326, 243–259. doi:10.1007/s11104-009-0004-3
Gathala, M. K., Alison, M. Laing., Tiwari, T. P., Timsina., J., Islam., Md. S., Chowdhury, A. K., et al. (2020). Enabling smallholder farmers to sustainably improve their food, energy and water nexus while achieving environmental and economic benefits. Renew. Sustain. Energy Rev. 120, 109645. doi:10.1016/j.rser.2019.109645
Gathala, M. K., Ladha, J. K., Kumar, V., Saharawat, Y. S., Kumar, V., Kumar, V., et al. (2011a). Effect of tillage and crop establishment methods on physical properties of a medium-textured soil under a seven-year rice-wheat rotation. Soil Sci. Soc. Am. J. 75, 1851–1862. doi:10.2136/sssaj2010.0362
Gathala, M. K., Ladha, J. K., Kumar, V., Saharawat, Y. S., Kumar, V., Sharma, P. K., et al. (2011b). Tillage and crop establishment affects sustainability of South Asian rice-wheat system. Agron. J. 103, 961–971. doi:10.2134/agronj2010.0394
Gathala, M. K., Timsina, J., Islam, M. S., Rahman, M. M., Hossain, M. I., Harun-Ar-Rashid, M., et al. (2015). Conservation agriculture-based tillage and crop establishment options can maintain farmers’ yields and increase profits in South Asia’s rice-maize systems: Evidence from Bangladesh. Field Crops Res. 172, 85–98. doi:10.1016/j.fcr.2014.12.003
Ghimire, R., Bista, P., and Machado, S. (2019). Long-term management effects and temperature sensitivity of soil organic carbon in grassland and agricultural soils. Sci. Rep. 9, 12151. doi:10.1038/s41598-019-48237-7
Gmach, M. R., Cherubin, M. R., Kaiser, K., and Cerri, C. E. P. (2019). Processes that influence dissolved organic matter in the soil: A review. Sci. Agric. 77. doi:10.1590/1678-992X-2018-0164
Gupta Choudhury, S., Srivastava, S., Singh, R., Chaudhari, S. K., Sharma, D. K., Singh, S. K., et al. (2014). Tillage and residue management effects on soil aggregation, organic carbon dynamics and yield attribute in rice-wheat cropping system under reclaimed sodic soil. Soil Tillage Res. 136, 76–83. doi:10.1016/j.still.2013.10.001
Haque, M. E., Bell, R. W., Islam, M. A., and Rahman, M. A. (2016). Minimum tillage unpuddled transplanting: An alternative crop establishment strategy for rice in conservation agriculture cropping systems. Field Crops Res. 185, 31–39. doi:10.1016/j.fcr.2015.10.018
He, J., Wang., Q., Li, H., Tullberg, J. N., McHugh, A. D., Bai, Y., et al. (2009). Soil physical properties and infiltra- tion after long-term no-tillage and ploughing on the Chinese Loess Plateau. N. Z. J. Crop Hortic. Sci. 37 (3), 157–166. doi:10.1080/01140670909510261
Hobbs, P. R., Singh, Y., Giri, G. S., Lauren, J. G., and Duxbury, J. M. (2002). “Direct seeding and reduced tillage options in the rice-wheat systems of the IndoHi -Gangetic Plains of South Asia,” in Direct seeding: Research strategies and opportunities. Editors S. Pandey, M. Mortimer, L. Wade, T. P. Tuong, K. Lopez, and B. Hardy (Los Ba˜nos: IRRI), 201–215.
Huang, M., Yingbin, Z., Peng, J., Bing, X., Feng, Y., Cheng, Z., et al. (2012). Effect of tillage on soil and crop properties of wet-seeded flooded rice. Field Crops Res. 129, 28–38. doi:10.1016/j.fcr.2012.01.013
Islam, S., Gathala, M. K., Tiwari, T. P., Timsina, J., Laing, A. M., Maharjan, S., et al. (2019). Conservation agriculture based sustainable intensification: Increasing yields and water productivity for smallholders of the eastern gangetic plains. Field Crops Res. 238, 1–17. doi:10.1016/j.fcr.2019.04.005
Jackson, M. L. (1973). Soil chemical analysis. New Delhi, India: Constable and Co. Ltd. Prentice Hall of India Pvt. Ltd.
Jat, M. L., Gathala, M. K., Ladha, J. K., Saharawat, Y. S., Jat, A. S., Kumar, V., et al. (2009). Evaluation of precision land leveling and double zero-till systems in the rice-wheat rotation: Water use, productivity, profitability and soil physical properties. Soil Tillage Res. 105, 112–121. doi:10.1016/j.still.2009.06.003
Jat, M. L., Gathala, M. K., Saharawat, Y. S., Tetarwal, J. P., Gupta, R., and Yadvinder, Singh. (2013). Double no-till and permanent raised beds in maize–wheat rotation of north-Western Indo-Gangetic plains of India: Effects on crop yields, water productivity, profitability and soil physical properties. Field Crops Res. 149, 291–299. doi:10.1016/j.fcr.2013.04.024
Jat, R. A., Wani, S. P., and Sahrawat, K. L. (2012). Conservation agriculture in the semi-arid tropics: Prospects and problems. Adv. Agron. 117, 191–273. doi:10.1016/b978-0-12-394278-4.00004-0
Jat, R. K., Sapkota, T. B., Singh, R. G., Jat, M. L., Kumar, M., and Gupta, R. K. (2014). Seven years of conservation agriculture in a rice-wheat rotation of eastern gangetic plains of south Asia: Yield trends and economic profitability. Field Crops Res. 164, 199–210. doi:10.1016/j.fcr.2014.04.015
Kader, M. A., Senge, M., Mojid, M. A., and Nakamura, K. (2017). Mulching type-induced soil moisture and temperature regimes and water use efficiency of soybean under rain-fed condition in central Japan. Int. Soil Water Conservation Res. 5 (4), 302–308. doi:10.1016/j.iswcr.2017.08.001
Kaiser, K. (2003). Dissolved organic phosphorus and sulphur as influenced by sorptive interactions with mineral subsoil horizons. Eur. J. Soil Sci. 52, 489–493. doi:10.1046/j.1365-2389.2001.00396.x
Kaldivgo, E. J. (2001). Tillage systems and soil ecology. Soil Tillage Res. 61, 61–76. doi:10.1016/s0167-1987(01)00179-9
Karim, Z., Rahman, S. M., Ali, M. I., and Karim, A. J. (1988). A manual for determination of soil physical parameters. BARC: Soils and Irrigation Division. Soil bulk density.
Kozak, J. A., Aiken, R. M., Flerchinger, G. N., Nielsen, D. C., Ma, L., and L, . (2007). Comparison of modeling approaches to quantify residue architecture effects on soil temperature and water. Soil Tillage Res. 95, 84–96. doi:10.1016/j.still.2006.11.006
Krupnik, T. J., Santos, V. S., McDonald, A. J., Justice, S., Hossain, I., and Gathala, M. K. (2013). Made in Bangladesh: Scale-appropriate machinery for agricultural resource conservation. Mexico: CIMMYT, 126.
Krupnik, T. J., Yasmin, S., Pandit, D., Asaduzzaman, M., Khan, S. I., Majumdar, K., et al. (2014). “Yield performance and agronomic N efficiency of a maize-rice rotation under strip and conventional tillage in con- trasting environments in Bangladesh,” in World Congress 6 on Conservation Agriculture, Winnipeg, Canada, 22–25 June, 2014.
Kucharik, C. J. (2006). A multidecadal trend of earlier corn planting in the central USA. Agron. J. 98, 1544–1550. doi:10.2134/agronj2006.0156
Kumar, B. T. N., and Babalad, H. B. (2018). Soil organic carbon, carbon sequestration, soil microbial biomass carbon and nitrogen and soil enzymatic activity as influenced by conservation agriculture in pigeonpea and soybean intercropping system. Int. J. Curr. Microbiol. Appl. Sci. 7 (3), 323–333. doi:10.20546/ijcmas.2018.703.038
Kumar, V., and Ladha, V. (2011). Direct-seeding of rice: Recent developments and future research needs. Adv. Agron. 111, 297–413. doi:10.1016/B978-0-12-387689-8.00001-1
Kumawat, A., Vishwakarma, A. K., Wanjari, R. H., Sharma, N. K., Kumar, D., Biswas, A. K., et al. (2022). Impact of levels of residue retention on soil properties under conservation agriculture in Vertisols of central India. Archives Agron. Soil Sci. 68 (3), 368–382. doi:10.1080/03650340.2020.1836345
Kushwaha, C. P., Tripathi, S. K., and Singh, K. P. (2001). Soil organic matter and water-stable aggregates under different tillage and residue conditions in a tropical dryland agroecosystem. Appl. Soil Ecol. 16, 229–241. doi:10.1016/s0929-1393(00)00121-9
Laik, R., Sharma, S., Idris, M., Singh, A. K., Singh, S. S., Bhatt, B. P., et al. (2014). Integration of conservation agriculture with best management practices for improving system performance of the rice– wheat rotation in the Eastern Indo-Gangetic Plains of India. Agric. Ecosyst. Environ. 195, 68–82. doi:10.1016/j.agee.2014.06.001
Lal, R. (2000). Mulching effects on soil physical quality of an alfisol in Western Nigeria. Land Degrad. Dev. 11, 383–392. doi:10.1002/1099-145x(200007/08)11:4<383::aid-ldr393>3.0.co;2-6
Lampurlanés, J., and Cantero-Martínez, C. (2003). Soil bulk density and penetration resistance under different tillage and crop management systems and their relationship with barley root growth. Agron. J. 95 (3), 526–536. doi:10.2134/agronj2003.5260
Lauer, M. J., and Boyer, J. S. (1992). Internal CO2 measured directly in leaves: Abscisic acid and low leaf water potential cause opposing effects. Plant Physiol. 98, 1310–1316. doi:10.1104/pp.98.4.1310
Lenka, S., Lenka, N. K., Singh, R. C., Manna, M. C., Misra, A. K., and Rautaray, S. K. (2015). Tillage and manure induced changes in carbon storage and carbon management index in soybean–wheat cropping system in the vertisols of central India. Natl. Acad. Sci. Lett. 38, 461–464. doi:10.1007/s40009-015-0384-2
Li, N., Zhou, C., Sun, X., Jing, J., Tian, X., and Wang, L. (2018). Effects of ridge tillage and mulching on water availability, grain yield, and water use efficiency in rain-fed winter wheat under different rainfall and nitrogen conditions. Soil Tillage Res. 179, 86–95. doi:10.1016/j.still.2018.01.003
Li, Y., Li, Z., Cui, S., Jagadamma, S., and Zhang, Q. (2019). Residue retention and minimum tillage improve physical environment of the soil in croplands: A global meta-analysis. Soil Tillage Res. 194, 104292. doi:10.1016/j.still.2019.06.009
Liang, A. Z., Zhang, X. P., Fang, H. J., Yang, X. M., and Drury, C. F. (2007). Short-term effects of tillage practices on organic carbon in clay loam soil of northeast China. Pedosphere 17, 619–623. doi:10.1016/s1002-0160(07)60073-3
Lindsay, W. L. (1978). Development of a DTPA soil test for zinc, iron, manganese, and copper. Soil Sci. Soc. Am. J. 42, 421–428. doi:10.2136/sssaj1978.03615995004200030009x
Liu, E., Ghirmai, S., Yan, C., Yu, J., Gu, R., Liu, S., et al. (2014). Long-term effects of no-tillage management practice on soil organic carbon and its fractions in the northern China. Geoderma 213, 379–384. doi:10.1016/j.geoderma.2013.08.021
Liu, K., and Wiatrak, P. (2012). Corn production response to tillage and nitrogen application in dry-land environment. Soil Tillage Res. 124, 138–143. doi:10.1016/j.still.2012.05.017
Liu, S. P., Zhang, H. C., Dai, Q. G., Huo, Z. Y., Xu, K., and Ruan, H. F. (2005). Effects of no-tillage plus interplanting and remaining straw on the field on crop land eco environment and wheat growth. Chin. J. Appl. Ecol. 16, 393–396.
Lu, X. (2020). A meta-analysis of the effects of crop residue return on crop yields and water use efficiency. PLoS ONE 15, e0231740. doi:10.1371/journal.pone.0231740
Luna-guido, M., Crossa, J., Ceja, J., and Jat, R. A. (2007). Influence of tillage, residue management, and crop rotation on soil microbial biomass and catabolic diversity. doi:10.1016/j.apsoil.2007.03.006
Luo, Z., Wang, E., and Sun, O. J. (2010). Can no-tillage stimulate carbon sequestration in agricultural soils? A meta-analysis of paired experiments. Agric. Ecosyst. Environ. 139 (1–2), 224–231. doi:10.1016/j.agee.2010.08.006
Marongwe, L. S., Nyagumbo, I., Kwazira, K., Kassam, A., and Friedrich, T. (2012). Conservation agriculture and sustainable crop intensification: A Zimbabwe case study. Rome: Integrated Crop Management. Plant Production and Protection Division, Food and Agriculture Organization of the United Nations.
Massacci, A., Nabiev, S. M., Pietrosanti, L., Nematov, S. K., Chernikova, T. N., Thor, K., et al. (2008). Response of the photosynthetic apparatus of cotton (Gossypium hirsutum) to the onset of drought stress under field conditions studied by gas-exchange analysis and chlorophyll fluor- escence imaging. Plant Physiol. biochem. 46, 189–195. doi:10.1016/j.plaphy.2007.10.006
Mirzaei, M., Anari, M. G., Razavy-toosi, E., Asadi, H., Moghiseh, E., Saronjic, N., et al. (2021). Preliminary effects of crop residue management on soil quality and crop production under different soil management regimes in corn-wheat rotation systems. Agronomy 11, 302. doi:10.3390/agronomy11020302
Mupangwa, W., Thierfelder, C., Cheesman, S., Nyagumbo, I., Muoni, T., MhlangaNgwira, A., et al. (2020). Effects of maize residue and mineral nitrogen applications on maize yield in conservation-agriculture-based cropping systems of Southern Africa. Renew. Agric. Food Syst. 35 (3), 322–335. doi:10.1017/S174217051900005X
Najafinezhad, H., Sarvestani, Z. T., Ali, S., and Naghavi, H. (2015). Evaluation of yield and some physiological changes in corn and sorghum under irrigation regimes and application of barley residue, zeolite and superabsorbent polymer. Archives Agron. Soil Sci. 61 (7), 891–906. doi:10.1080/03650340.2014.959938
Naresh, R. K., Chandra, M. S., Baliyan, A., Pathak, S. O., Kanaujiya, P. K., Kumar, B. N., et al. (2021). Impact of residue incorporation on soil carbon storage, soil organic fractions, microbial community composition and carbon mineralization in rice-wheat rotation – a review. Int. J. Environ. Clim. Change 11 (4), 42–59. doi:10.9734/ijecc/2021/v11i430390
Nasim, M. S. M., Shahidullah, A., Saha, M. A., Muttaleb, T. L., Aditya, M. A., and Kabir, M. S. (2017). Distribution of crops and cropping patterns in Bangladesh. Bangladesh Rice J. 21 (2), 1–55. doi:10.3329/brj.v21i2.38195
Nobuhisa, K., and Hiroyuki, T. (2009). Effects of reduced tillage, crop residue management and manure application practices on crop yields and soil carbon sequestration on an Andisol in northern Japan. Soil Sci. Plant Nutr. 55, 546–557. doi:10.1111/j.1747-0765.2009.00385.x
Page, A. L., Miller, R. H., and Dr, Kuny. (1989). “Methods of soil analysis. Part 2,” in American society of agronomy, soil science society of America, madison, wis, USA. 2nd edition.
Page, A. L., Miller, R. H., and Kuny, D. R. (1982). “Methods of soil analysis. Part 2,” in American soc. Agron., inc., soil sci. Soc. American inc. Madison, Wisconsin, USA. 2nd edn., 403–430.
Paknejad, F., Nasri, M., Tohidi, M. H. R., Zahedi, H., and Jami, A. M. (2007). Effects of drought stress on chlorophyll fluorescence parameters, chlorophyll content and grain yield of wheat cultivars. J. Biol. Sci. 7, 841–847. doi:10.3923/jbs.2007.841.847
Parihar, C. M., Jat, S. L., Singh, A. K., Kumar, B., Pradhan, S., Pooniya, S., et al. (2016). Conservation agriculture in irrigated intensive maize-based systems of north-Western India. Effects on crop yields, water productivity and economic profitability. Field Crops Res. 193, 104–116. doi:10.1016/j.fcr.2016.03.013
Patra, S., Julich, S., Feger, K. H., Jat, M. L., Jat, H., Sharma, P. C., et al. (2019). Soil hydraulic response to conservation agriculture under irrigated intensive cereal-based cropping systems in a semiarid climate. Soil Tillage Res. 192, 151–163. doi:10.1016/j.still.2019.05.003
Popat, R., and Banakara, K. (2020). DoE bioresearch: Analysis of design of experiments for biological research. R package version 0.1.0.
Ranaivoson, L., Naudin, K., Ripoche, A., Affholder, F., Rabeharisoa, L., and Corbeels, M. (2017). Agro-ecological functions of crop residues under conservation agriculture. A review. Agron. Sustain. Dev. 37 (4), 26. doi:10.1007/s13593-017-0432-z
Rashid, M. H., Goswami, P. C., Hossain, M. F., Mahalder, D., Rony, M. K. I., Shirazy, B. J., et al. (2018). Mechanised non-puddled transplanting of boro rice following mustard conserves resources and enhances productivity. Field Crops Res. 225, 83–91. doi:10.1016/j.fcr.2018.06.006
Rashid, M. H., Timsina, J., Islam, N., and Islam, S. (2019). Tillage and residue-management effects on productivity, profitability and soil properties in a rice-maize-mungbean system in the Eastern Gangetic Plains. J. Crop Improv. 33 (5), 683–710. doi:10.1080/15427528.2019.1661056
R Core Team (2020). R: a language and environment for statistical computing. Vienna, Austria: R Foundation for Statistical Computing. Available at: https://www.R-project.org/.
Reicosky, D. C., Dugas, W., and Torbert, H. (1997). Tillage-induced soil carbon dioxide loss from different cropping systems. Soil Tillage Res. 41, 105–118. doi:10.1016/s0167-1987(96)01080-x
Robertson, A. D., Paustian, K., Ogle, S., Wallenstein, M. D., Lugato, E., and Cotrufo, M. F. (2019). Unifying soil organic matter formation and persistence frameworks : The MEMS model, 1225–1248.
Roose, E., and Barthes, B. (2001). Organic matter management for soil conservation and productivity restoration in africa: A contribution from francophone research. Nutr. Cycl. Agroecosyst. 61, 159–170. doi:10.1023/A:1013349731671
Roy, D., Datta, A., and Choudhary, M. (2022). Impact of long term conservation agriculture on soil quality under cereal based systems of North West India Geoderma Impact of long term conservation agriculture on soil quality under cereal based systems of North West India, (January). doi:10.1016/j.geoderma.2021.115391
Saha, S., Chakraborty, D., Sharma, A. R., Tomar, R. K., Bhadraray, S., Sen, U., et al. (2010). Effect of tillage and residue management on soil physical properties and crop productivity in maize (Zea mays)-Indian mustard (Brassica juncea) system. Indian J. Agric. Sci. 80 (8), 679–685.
Saharawat, Y. S., Singh, B., Malik, R. K., Ladha, J. K., Gathala, M., Jat, M. L., et al. (2010). Evaluation of alternative tillage and crop establishment methods in a rice-wheat rotation in North Western IGP. Field Crops Res. 116 (3), 260–267. doi:10.1016/j.fcr.2010.01.003
Sairam, R. K., and Srivastava, G. C. (2002). Changes in antioxidant activity in sub-cellular fractions of tolerant and susceptible wheat genotypes in response to long-term salt stress. Plant Sci. 162, 897–904. doi:10.1016/S0168-9452(02)00037-7
Salahin, N., JahirUddin, M., Islam, M. R., Alam, M., K., Haque, M. E., Ahmed, S., et al. (2021). Establishment of crops under minimal soil disturbance and crop residue retention in rice-based cropping system: Yield advantage, soil health improvement, and economic benefit. Land 10, 581. doi:10.3390/land10060581
Salinas-Garcia, J. R., Baez-Gonzalez, A. D., Tiscareno-Lopez, M., and Rosales-Robles, E. (2001). Residue removal and tillage interaction effects on soil properties under rain-fed corn production in central Mexico. Soil Tillage Res. 59, 67–79. doi:10.1016/s0167-1987(00)00187-2
Samal, S. K., Rao, K. K., Poonia, S. P., Kumar, R., Mishra, J. S., Prakash, V., et al. (2017). Evaluation of long-term conservation agriculture and crop intensification in rice-wheat rotation of Indo-Gangetic Plains of South Asia: Carbon dynamics and productivity. Eur. J. Agron. 90, 198–208. doi:10.1016/j.eja.2017.08.006
Sarkar, S., Skalicky, M., Hossain, A., Brestic, M., Saha, S., Garai, S., et al. (2020). Management of crop residues for improving input use efficiency and agricultural sustainability. Sustain. Switz. 12 (23), 9808–9824. doi:10.3390/su12239808
Sasal, M. C., Andriulo, A. E., and Taboada, M. A. (2006). Soil porosity characteristics and water movement under zero tillage in silty soils in Argentinian Pampas. Soil Tillage Res. 87, 9–18. doi:10.1016/j.still.2005.02.025
Saurabh, K., Rao, K. K., Mishra, J. S., Kumar, R., Poonia, S. P., Samal, S. K., et al. (2021). Influence of tillage-based crop establishment and residue management practices on soil quality indices and yield sustainability in rice-wheat cropping system of Eastern Indo-Gangetic Plains. Soil Tillage Res. 206, 104841. doi:10.1016/j.still.2020.104841
Saviozzi, A., Levi-Minzi, R., Cardelli, R., and Riffaldi, R. (2001). A comparison of soil quality in adjacent cultivated, forest and native grassland soils. Plant Soil 233, 251–259.
Schnier, H. F., Dingkuhn, M., De Datta, S. K., Mengel, K., and Faronilo, J. E. (1990). Nitrogen fertilization of direct-seeded flooded vs. transplanted rice: I. Nitrogen uptake, photosynthesis, growth, and yield. Crop Sci. 30, 1276–1284. doi:10.2135/cropsci1990.0011183x003000060024x
Shapiro, S. S., and Wilk, M. B. (1965). An analysis of variance test for normality (complete samples). Biometrika 52 (3/4), 591–611. doi:10.2307/2333709
Sharma, P. K., and De Datta, S. K. (1986). Physical properties and processes of puddled rice soils. Adv. Soil Sci. 5, 139–178.
Sharma, P. K., Ladha, J. K., and Bhushan, L. (2003). “Soil physical effects of puddling in rice– wheat cropping system,” in Improving the productivity and sustainability of rice–wheat systems: Issues and impacts. Editor J. K. Ladhaet al. 97–114.
Shefazadeh, M. K., Karimizadeh, R., Mohammadi, M., and Suq, H. S. (2012). Using flag leaf chlorophyll content and canopy temperature depression for determining drought resistant durum wheat genotypes. J. Food Agric. Environ. 10, 509–515.
Singh, D., Lenka, S., Lenka, N. K., Trivedi, S. K., Bhattacharjya, S., Sahoo, S., et al. (2020). Effect of reversal of conservation tillage on soil nutrient availability and crop nutrient uptake in soybean in the vertisols of central India. Sustain. Switz. 12 (16), 6608. doi:10.3390/su12166608
Singh, M., Lal, R., and Ann-varughese, M. (2013). Soil & Tillage Research Twenty-two years of tillage and mulching impacts on soil physical characteristics and carbon sequestration in Central Ohio. Soil & Tillage Res. 126, 151–158. doi:10.1016/j.still.2012.08.001
Singh, R. C., Lenka, S., and Singh, C. D. (2014). Conservation tillage and manure effect on soil aggregation, yield and energy requirement for wheat (Triticum aestivum) in vertisols. Indian J. Agric. Sci. 84, 267–271.
Singh, V. K., Yadvinder, S., Dwivedi, B. S., Singh, S. K., Majumdar, K., JatMishra, P. R., et al. (2016). Soil physical properties, yield trends and economics after five years of conservation agriculture-based rice-maize system in north-Western India. Soil Tillage Res. 155, 133–148. doi:10.1016/j.still.2015.08.001
Snedecor, G. W., and Cochran, W. G. (1989). Statistical methods. 8th Edn. Ames: Iowa State University Press.
Sokolowski, A. C., Prack McCormick, B., De Grazia, J., Wolski, J. E., Rodríguez, H. A., Rodríguez-Frers, E. P., et al. (2020). Tillage and no-tillage effects on physical and chemical properties of an Argiaquoll soil under long-term crop rotation in Buenos Aires, Argentina. Int. Soil Water Conservation Res. 8 (2), 185–194. doi:10.1016/j.iswcr.2020.02.002
Song, K., Yang, J., Xue, Y., Lv, W., Zheng, X., and Pan, J. (2016). Influence of tillage practices and straw incorporation on soil aggregates, organic carbon, and crop yields in a rice-wheat rotation system. Sci. Rep. 6, 36602. doi:10.1038/srep36602
Stewart, P. R., Dougill, A. J., Thierfelder, C., Pittelkow, C. M., Stringer, L. C., Kudzala, M., et al. (2018). The adaptive capacity of maize-based conservation agriculture systems to climate stress in tropical and subtropical environments: A meta-regression of yields. Agric. Ecosyst. Environ. 251, 194–202. doi:10.1016/j.agee.2017.09.019
Sun, W., Canadell, J. G., Yu, L., Yu, L., Zhang, W., Smith, P., et al. (2020). Climate drives global soil carbon sequestration and crop yield changes under conservation agriculture. Glob. Chang. Biol. 26, 3325–3335. doi:10.1111/gcb.15001
Tangyuan, N., Bin, H., Nianyuan, J., Shenzhong, T., and Zengjia, L. (2009). Effects of conservation tillage on soil porosity in maize-wheat cropping system. Plant Soil Environ. 55, 327–333. doi:10.17221/25/2009-pse
Timsina, J., and Connor, D. J. (2001). Productivity and management of rice–wheat cropping systems: Issues and challenges. Field Crops Res. 69, 93–132. doi:10.1016/s0378-4290(00)00143-x
Timsina, J., Jat, M. l., and Majumdar, K. (2010). Rice-maize systems of South Asia: Current status, future prospects and research priorities for nutrient management. Plant Soil 335, 65–82. doi:10.1007/s11104-010-0418-y
Timsina, J., Wolf, J., Guilpart, N., Van Bussel, L. G. J., Grassini, P., Van Wart, J., et al. (2018). Can Bangladesh produce enough cereals to meet future demand? Agric. Syst. 163, 36–44. doi:10.1016/j.agsy.2016.11.003
Turmel, M. S., Speratti, A., Baudron, F., Verhulst, N., and Govaerts, B. (2015). Crop residue management and soil health: A systems analysis. Agric. Syst. 134, 6–16. doi:10.1016/j.agsy.2014.05.009
Uddin, M. J., Hooda, P. S., Mohiuddin, A. S. M., Smith, M., and Waller, M. (2019). Land inundation and cropping intensity influences on organic carbon in the agricultural soils of Bangladesh. Catena 178, 11–19. doi:10.1016/j.catena.2019.03.002
Vance, E. D., Brookes, P. C., and Jenkinson, D. S. (1987). An extraction method for measuring soil microbial biomass C. Soil Biol. Biochem. 19 (6), 703–707. doi:10.1016/0038-0717(87)90052-6
Vanden Bygaart, A. J., and Angers, D. A. (2006). Towards accurate measurements of soil organic carbon stock change in agroecosystems. Can. J. Soil Sci. 86, 465–471. doi:10.4141/s05-106
Vasconcelos, A. L. S., Cherubin, M. R., Feigl, B. J., Cerri, C. E., Gmach, M. R., and Siqueira-Neto, M. (2018). Greenhouse gas emission responses to sugarcane straw removal. Biomass Bioenergy 113, 15–21. doi:10.1016/j.biombioe.2018.03.002
Verhulst, N., Nelissen, V., Jespers, N., Haven, H., Sayre, K., Deckers, J., et al. (2011). Soil water content, maize yield and its stability as affected by tillage and crop residue management in rainfed semi-arid highlands. Plant Soil 344, 73–85. doi:10.1007/s11104-011-0728-8
Ward, P. R., Roper, M. M., Jongepier, R., and Fernandez, M. M. A. (2013). Consistent plant residue removal causes decrease in minimum soil water content in a Mediterranean environment. Biologia 68, 1128–1131.
Wu, L., Swan, J. B., Paulson, W. H., and Randall, G. W. (1992). Tillage effects on measured soil hydraulic properties. Soil Tillage Res. 25, 17–33. doi:10.1016/0167-1987(92)90059-k
Wuest, S., Caesar, T., Wright, S. F., and Williams, J. (2005). Organic matter addition, N, and residue burning effects on infiltration, biological, and physical properties of an intensively tilled silt-loam soil. Soil Tillage Res. 84, 154–167. doi:10.1016/j.still.2004.11.008
Yang, C. M., Yang, L. Z., and Zhu, O. Y. (2005). Organic carbon and its fractions in paddy soil as affected by different nutrient and water regimes. Geoderma 124, 133–142. doi:10.1016/j.geoderma.2004.04.008
Zeng, R., Wei, Y., Huang, J., Chen, X., and Cai, C. (2021). Soil organic carbon stock and fractional distribution across central-south China. Int. Soil Water Conservation Res. 9 (4), 620–630. doi:10.1016/j.iswcr.2021.04.004
Zhao, S. C., Huang, S. W., Qiu, S. J., and He, P. (2018). Response of soil organic carbon fractions to increasing rates of crop residue return in a wheat-maize cropping system in north-central China. Soil Res. 56 (8), 856–864. doi:10.1071/SR18123
Zhao, X., Liu, B. Y., Liu, S. L., Qi, J. Y., Wang, X., Pu, C., et al. (2020). Sustaining crop production in China’s cropland by crop residue retention: A meta-analysis. Land Degrad. Dev. 31, 694–709. doi:10.1002/ldr.3492
Keywords: direct seeded rice, strip tillage, residue management, conservation agriculture, system productivity, carbon fractions
Citation: Sarker MR, Galdos MV, Challinor AJ, Huda MS, Chaki AK and Hossain A (2022) Conservation tillage and residue management improve soil health and crop productivity—Evidence from a rice-maize cropping system in Bangladesh. Front. Environ. Sci. 10:969819. doi: 10.3389/fenvs.2022.969819
Received: 15 June 2022; Accepted: 14 September 2022;
Published: 07 October 2022.
Edited by:
Mike Rivington, The James Hutton Institute, United KingdomReviewed by:
Nirmalendu Basak, Central Soil Salinity Research Institute (ICAR), IndiaSangeeta Lenka, Indian Institute of Soil Science (ICAR), India
Copyright © 2022 Sarker, Galdos, Challinor, Huda, Chaki and Hossain. This is an open-access article distributed under the terms of the Creative Commons Attribution License (CC BY). The use, distribution or reproduction in other forums is permitted, provided the original author(s) and the copyright owner(s) are credited and that the original publication in this journal is cited, in accordance with accepted academic practice. No use, distribution or reproduction is permitted which does not comply with these terms.
*Correspondence: Mamunur Rashid Sarker, ZWVtbXJzQGxlZWRzLmFjLnVr
†ORCID: Mamunur Rashid Sarker, orcid.org/0000-0002-4001-340X; Marcelo Valadares Galdos, orcid.org/0000-0002-6080-0726; Andrew J. Challinor, orcid.org/0000-0002-8551-6617; Apurbo K. Chaki, orcid.org/0000-0001-8555-7438; Akbar Hossain, orcid.org/0000-0003-0264-2712