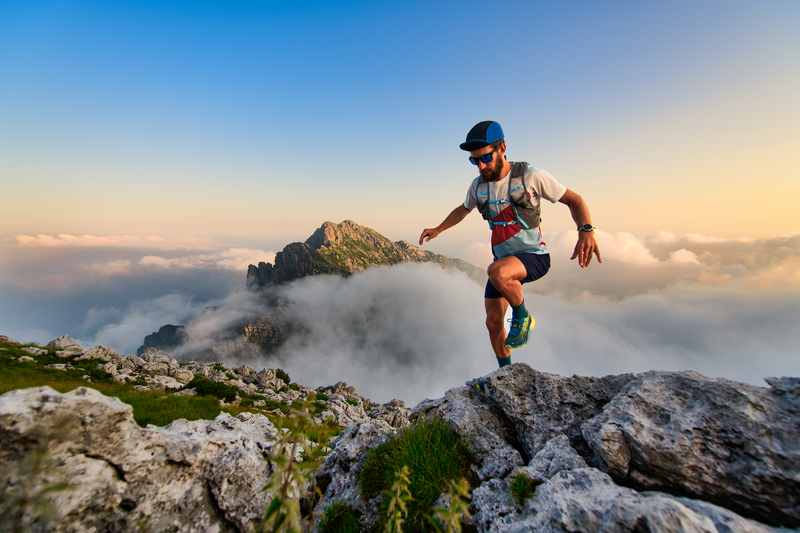
95% of researchers rate our articles as excellent or good
Learn more about the work of our research integrity team to safeguard the quality of each article we publish.
Find out more
REVIEW article
Front. Environ. Sci. , 13 February 2023
Sec. Soil Processes
Volume 10 - 2022 | https://doi.org/10.3389/fenvs.2022.962581
By affecting 10% of the world’s total arable land, soil salinity has become a potential threat to feeding the exploding population. As per the current scenario, among 1,125 million hectares of salt-affected land, nearly 76 million hectares are seriously affected due to human-induced salinization. Due to soil salinization, crop productivity is being hampered. In order to enhance productivity, there is an urgent need to shift from traditional methods to advanced 3E (efficient, economic, and environmentally sound) technology for soil salinity reclamation and management to achieve better soil health and sustainable crop production. The detailed mechanism of salt interference with various pathways involved in plant growth and development needs to be understood. This article critically reviews the mechanism of harmful salt interference with nutrient dynamics in soil and various physiological pathways involved in crop growth to apply various soil-oriented (crop residue management, biochar application, and agroforestry system) and plant-oriented [plant growth-promoting microbes (PGPMs), plant growth regulators, and nanotechnology] promising reclamation and rehabilitation approaches to mitigate its hazardous effect on soil salinity. The monitoring and assessment of salt-affected soils through remote sensing (RS) and geographical information systems (GISs) are pivotal in the management and framing of long-term policies to confront alarming threats to crop productivity and sustainability. This study provides an insight into recent developments in soil salinity management and proposes futuristic solutions that could ameliorate soil salinity to attain crop sustainability under adverse environmental conditions.
Plants are encountering several environmental adversities caused both by living and non-living factors, with the former known as biotic stress and the latter as abiotic stress. Various stress factors have been found in almost all environments and climatic conditions, but salinity stress seen in plants growing under arid and semi-arid conditions should be given priority. Salinity stress is a form of abiotic stress, which is caused due to salt accumulation in the root zones of plants, making them physiologically flaccid and unfit for production (Munns, 2005; Manchanda and Garg, 2008). Currently, approximately 1.1 × 109 ha of land is salt-affected globally, and saline soils are increasing at an alarming rate of 1.5 million hectares annually (Hossain, 2019). The FAO estimated that over 6% of the world’s land area and about 20% of irrigated croplands are affected by salinity (Parihar et al., 2015). Nearly 95% of the total degraded land in India (7.5 million hectares) is accounted for by soil salinity/alkalinity, especially found in the states in the north western part (Western Uttar Pradesh, Punjab, Haryana, northern Rajasthan, and Delhi), the central part (Gujarat, central and western Rajasthan, Maharashtra, and Madhya Pradesh), and the north eastern part (West Bengal, central and eastern Uttar Pradesh, Bihar, and eastern Odisha) of India (Choudhary et al., 2018; Kumar and Sharma, 2020). Meanwhile, the human population will reach approximately 9.7 billion in 2050 and, to feed this population, the target is to bring about a 1.7-fold increase in crop yields (Tilman et al., 2011; Yadav, 2020), for which sustainable agriculture with maintained food quality is highly desired.
Containing profuse neutral soluble salts (chlorides and sulfates of sodium, magnesium, and calcium), saline soils inhibit the growth of plants growing in them (Panhwar et al., 2019; Ismayilov et al., 2021). These soils are characterized mainly by a highly elevated water table, the escalated salinity level of underground water, and poor drainage conditions, leading to ceased aeration of the affected soil. Soil salinity affects soil microbial activity and diversity, which in turn disturbs various vital activities in soil, viz., residue decomposition, soil respiration, and nitrogen (N) transformations (Schirawski and Perlin, 2018). The combinations of these problems have rendered large tracts of land unproductive, with direct economic losses. Salinity is developed naturally through primary or human-induced secondary salinity (Shrivastava and Kumar 2015). In a plant system, salt can have an effect in three ways, viz., reducing water potential, which results in osmotic stress (Setia et al., 2013), induced ion imbalance or disturbing ion homeostasis, and ion toxicity due to the excess accumulation of ions in plant cells (Bharti et al., 2016). The majority of physiological processes in plants, including seedling germination, growth, photosynthesis in leaves, and nutrient and water uptake by vascular bundles, are affected by salt stress leading to a decrease in yield (Bartels and Sunkar 2005; Bano and Fatima 2009; Qin et al., 2016), although the type and degree of these effects vary with crops, their growth stages, and their sensitivity to a saline environment. The extent of adverse impacts due to salinity stress is more prominent in dicotyledons ranging from Arabidopsis thaliana, which is very sensitive toward salinity, to halophytes such as Salicornia bigelovii and Panicum virgatum (Pang et al., 2010). Plants have the inherent ability to cope with fluctuations in their ambiance and adversity due to environmental extremities (Simontacchi et al., 2015). The principal defensive mechanisms utilized by plants when exposed to salinity stress can be attributed to ion exclusion by the roots and controlling the uptake and transport of specific ions (Sairam and Tyagi, 2004; Hanin et al., 2016) through carrier proteins, channel proteins, antiporters, and symporters (Gupta and Huang, 2014), as well as tissue tolerance to high ion concentration (Munns and Tester 2008). Plants synthesize and accumulate compatible solutes, such as proline, glycine betaine, sugar (Kerepesi and Galiba, 2000; Ashraf and Foolad, 2007; Hossain et al., 2011), and polyols, that have been reported to maintain cell homeostasis in plants under stress. Furthermore, microbial populations present in soil help boost plants’ tolerance mechanisms by activating several internal processes within plants known as plant growth-promoting rhizobacteria (PGPR). Through the activation of the required genes, enzymes, metabolites, and hormones, these beneficial microbes have been reported to have a direct or indirect effect on plant growth and metabolism under various stressful environmental conditions (Bloemberg and Lugtenberg 2001; Yang et al., 2009; Egamberdieva 2012; Panhwar et al., 2014; Naher et al., 2018; Yadav, 2020), such as the solubilization of complex phosphate (Hamdali et al., 2008), siderophore production (Carrillo-Castaneda et al., 2002; Etesami and Beattie, 2017), improving photosynthesis and water use efficiency (Porcel et al., 2012), helping in nitrogen fixation (Bloemberg and Lugtenberg 2001; Etesami, 2018), maintaining ionic homeostasis (Na+/K+ ratio) within plant cells (Islam et al., 2016), the alleviation of biotic (Toklikishvili et al., 2010) and abiotic stresses (Dimkpa et al., 2009) through the production of antioxidant enzymes [such as catalase, superoxide dismutase (SOD), and peroxidase (Islam et al., 2016)], volatile substances (Yang et al., 2009; Timmusk et al., 2014), and the regulation of the stress-responsive genes (Gond et al., 2015).
The unwise and random use of fertilizers also results in soil salinity, leading to a decrease in crop productivity (Rütting et al., 2018). Therefore, it is necessary to adopt technologies that could improve crop production, crop residue utilization, and soil fertility. Due to its more significant potential in enhancing soil physico-chemical properties, organic matter could be a game changer in modifying the saline environment into a stable and healthy crop environment (Mahmoud et al., 2009). Improving soil structure, aeration, and aggregation increases the probability of a higher crop yield and healthier soil (Sparks, 1995). Understanding the impacts of salinity both on soil health and plant physiology is necessary to mitigate their adverse effects on crop production. However, there are a few age-old soil salinity remediations, such as leaching or flushing excess salt with quality irrigation water up to the required root zone and regularly scraping the surface salts. In contrast, considering that water scarcity will be a huge problem that will affect crop production worldwide, mostly in arid and semi-arid regions, in the next few decades, sustainable ways to remediate the adverse effects of soil salinity on crop growth and ultimately crop production need to be emphasized. Therefore, this review article is focused on discussing the responses of plants under salinity stress and improving plant performance under a saline environment through various sustainable approaches.
Saline soils are categorized as one of the most problematic soils, unsuitable for crop cultivation, due to the accumulation of high levels of adsorbed Na+ in the soil matrix. Irrigated agriculture usually leads to secondary soil salinization, which affects almost 20% of irrigated lands worldwide as most of the dry regions are devoid of rainfall and depend entirely on irrigation for agricultural practices (Pang et al., 2010; Kumar and Sharma, 2020; Mirlas et al., 2022). Due to insufficient precipitation in arid and semi-arid regions, there is a problem regarding the leaching of salts from surface soil. Their accumulation leads to soil salinization, which is further aggravated due to improper irrigation systems followed for crop production (Shrivastava and Kumar, 2015). There are various measures to categorize saline soils based on the values of the electrical conductivity of saturation extract (ECe), sodium absorption ratio (SAR), or exchangeable sodium percentage (ESP) as saline, sodic, or saline-sodic soil (Table 1). There are many severe effects of salt accumulation on soil health and crop productivity as salts, particularly Na+ in excess, deteriorate physical soil conditions by clogging the soil pores, causing impermeability, which further leads to crusting and hardpan formation in various layers of the soil matrix (Kumar and Sharma, 2020). Saline soil is defined as one in which the electrical conductivity (EC) of the saturation extract (ECe) in the root zone exceeds 4 dS m−1 (approximately 40 mM NaCl) at 25°C and has exchangeable sodium of 15% (Jamil et al., 2011). Values above this level lead to a depreciation in crop production, along with ecological imbalance and deteriorated soil physicochemical properties. Understanding the deleterious effect of soil salinity on various processes in plants represents the first step toward the remediation of salinity-related stress in crops for sustainable crop production.
TABLE 1. Classification of soil salinity based on the reaction (pH), electrical conductivity (EC), exchangeable sodium percentage (ESP), and sodium absorption ratio (SAR) of the affected soil.
Soil salinity is hazardous to intrinsic soil permeability and infiltration, especially in saline soil (ECe >4.0 dS m−1). Studies have revealed that excessive salt deposition in the plant rhizosphere makes water entry to the plant system difficult due to high osmotic pressure, which further reduces the soil water potential in the context of crop uptake (Singh et al., 2011; Kumar et al., 2014). However, when it comes to sodic soil, due to excess sodium ion (Na+) deposition in the soil matrix, the deflocculation of soil particles leads to disturbance in the soil structure and aggregate stability. As a result, a reduced macroporosity is responsible for infiltration, and drainage further aggravates the unavailability of water and nutrients for stress-affected plants (Singh et al., 2016; Mishra et al., 2018a). Salinity, when it persists, gradually reduces the water influx into the vascular bundles present in plant’s roots, making the cells flaccid, and the plant gradually suffers from physiological drought. Tang et al. (2020) revealed the signaling mechanism for stomatal closure due to osmotic stress by highlighting cellular Ca2+ signaling, which plays a vital role in signal transduction in a plant cell. Here, Ca2+ signaling is responsible for activating the CBL1/9-CIPK 23 pathway, which causes stomatal closure in response to salinity stress. Calcineurin B-like proteins (CBLs) are a specific group of Ca2+ sensors that decode all Ca2+ signals by activating CBL-interacting protein kinases (CIPKs) (DeFalco et al., 2009; Luan, 2009). In the present context, the CBL-CIPK pathway responsible for stomatal closure under abiotic stress has been focused on (CBL1/9-CIPK 23). In response to ionic stress due to the accumulation of Na+ dominating all other cells in the apoplast and outside the cell membrane, there is the production of hydrogen peroxide (H2O2), which is a reactive oxygen species produced in response to salt stress from singlet oxygen (O2 -) (Zhu, 2016). The reactive oxygen species (ROS) molecules enter the plasma membrane and activate Ca2+ molecules in response to stress and, again, this signals the CBL-CIPK pathway to close the stomata by losing water molecules (Tang et al., 2020). Stomatal closure leads to stopping the entry of carbon dioxide (CO2), which has an impact on the Calvin cycle (C3 cycle) within the stroma of the chloroplast and leads to an increased rate of photorespiration, which leads to loss of energy from plant cells, and the process continues to peroxisomes and mitochondria. Increased photorespiration during the conversion of glycolate to glyoxylate by the enzyme glyoxylate oxidase leads to a rise in H2O2 accumulation in the peroxisomes (Zhu, 2016; Ma et al., 2020). Such an increase may lead to the activation of an antioxidant enzyme complex [alternative oxidase (AOX)], such as ascorbate peroxidase (APX) or catalase (CAT), which breaks down H2O2 to produce water (H2O) molecules, but another enzyme, namely, indole acetic acid oxidase (IAA oxidase), oxidizes indole-3-acetic acid (IAA) to produce inactive molecules in the cytoplasm (oxidized indole acetic acid) (Moller and Sweetlove, 2010; Wu et al., 2020). On the other hand, the H2O2 molecules in the peroxisomes are converted at the same time into hydroxyl radicals (OH) by Fenton’s reaction and enter different cell organelles, including mitochondria, which are the powerhouse of the cell (Cruz de Carvalho, 2008; Deinlein et al., 2014). Some studies have revealed an increase in ATPase activity due to ROS accumulation in mitochondria, leading to an increase in the electron transport system (Cruz de Carvalho, 2008; Asthir et al., 2020). Due to the fast electron transport system (ETS), sometimes there is a loss of electrons (e−) from the complex I, III, and IV, and the skipped electron is often accepted by free oxygen (O2−) and is either converted to superoxide radicals (O2.-) by Mehler’s reaction or to H2O2 and OH.by Fenton’s reaction, and there is ROS accumulation in mitochondria (Cruz de Carvalho, 2008; Asthir et al., 2020). The ROS radicals degrade the membranes of the vital cell organelles (chloroplasts and mitochondria), cause damage to deoxyribonucleic acid (DNA) inside the nucleus, and often destructure the essential amino acids (methionine and thiol group of cysteines) present in the rough endoplasmic reticulum (RER) (Van Aken and Van Breusegem, 2015; Zhao et al., 2020). This might be due to their accumulation in the organelles, hence imposing severe stress on plant cells known as oxidative stress. Malondialdehyde (MDA) production is often considered the measure of oxidative stress imposed on plant cells, which is produced due to the oxidation of polyunsaturated fatty acids (PUFAs) present in cell lipid bilayers of the cell membrane during the entry of H2O2 inside the cell, causing lipid membrane peroxidation (Marino et al., 2012). As there is an accumulation of Na+ inside the cell by suppressing other essential ions (K+ and Ca2+), the vacuole takes the excessive Na+ inside through tonoplast via membrane compartmentalization, which develops ionic stress in the plant cell (Tang et al., 2020; Zhao et al., 2020). As discussed previously, a prolonged saline environment can cause protein degradation, membrane lipid peroxidation, cell organelle degradation in plant cells, and physiological drought in plants due to osmotic, ionic, and oxidative stress alone or in combination (Figure 1). A larger part of physiological cycles in plants, including seedling germination, development, photosynthesis in leaves, and supplement and water take-up by vascular groups, is impacted by salt pressure leading to a decline in yield (Qin et al., 2016), and the level of these impacts shifts with crops, their development stages, and their aversion to a saline climate. The effect of excess salt concentration in soil solution on various physiological and morphological traits, which ultimately hampers crop yield and productivity, is summarized in Table 2.
FIGURE 1. Increased salt concentration in soil results in the elevated production of ABA in plant roots, which enters the cell through the ABA receptor and activates the RBOH receptor via PLD and PA at the N-terminal of the RBOH receptor. The RBOH receptor stimulates H2O production from O2 and O2.-, which leads to oxidative injury via malondialdehyde (MDA) production and supports the increased concentration of cytosolic calcium ion production. Increased cytosolic calcium ion production further initiates stomatal closing via the induction of CBL1/9-CIPK23 complex formation. Stomatal closing contributes toward reduced CO2 formation in the chloroplast, resulting in an increased Fenton’s reaction in thylakoid (leading to ROS accumulation) and a reduced rate of the C3 cycle, as well as an increased rate of photorespiration in the stroma, which accelerates H2O2 accumulation in peroxisome affecting the mitochondria (increased Mehler’s reaction and Fenton’s reaction leading to ROS accumulation and increased ATPase activity), endoplasmic reticulum (methionine and cytosine destruction), and nucleus (DNA damage). The effect on sub-cellular organelles may finally lead to apoptosis via oxidative stress. Increased salt concentration may further give rise to an ionic imbalance in the vacuole, leading to apoptosis via ionic stress. Increased sodium ion accumulation in the root may also contribute to osmotic stress. ABA, abscisic acid; RBOH, respiratory burst oxidase homolog; PLD, phospholipase D; PA, phosphatidic acid; CBL, calcineurin B-like protein; CIPK, CBL-interacting protein kinase. The concept of the figure was inspired by Mao et al. (2016) and Zhang et al. (2009). The figure template was inspired by Berru (2007), LadyofHats (2013), Rifqi (2017), Fvasconcellos (2007), Manske (2009), Smartse (2010), and Zifan (2016).
Understanding the effect of excess salt on the plant environment, plants, and plant cells can lead to the development of technologies to mitigate the negative effects of salinity stress on crop growth and physiology.
Due to poor irrigation practices and ecologically harmful cropping practices, soil salinity is expected to affect more than 50% of arable land by 2050 (Srivastava and Saxena, 2004). Crop production in saline soil declines due to prolonged salt interference with the plant–soil environment, or productivity sometimes ultimately declines due to extreme salinity. Due to the decrease in production, salinity directly impacts our food security. Therefore, proper and efficient methods for the identification and mapping of saline areas are unavoidable as a precautionary measure and a step toward salinity remediation. Due to the ever-growing population and the limited arable land for cultivation, it will likely not be possible to leave the salt-affected soil devoid of crop production, which requires various advances and innovations in salinity remediation for a sustainable crop production system.
Crop production nowadays is becoming more intensive, thereby significantly reducing the interval between two crops to manage the residue of the previous crop (Hou et al., 2019). There are different ways of handling crop residues, but farmers are more likely to utilize in situ management. Therefore, it is necessary to take into account the proper management of in situ residues that sustain soil health and air pollution (Perkins-Kirkpatrick et al., 2017). Over time, adopting crop residue burning as the simplest and most economical way to treat residues has been observed (Ravindra et al., 2019). However, this process has many adverse effects, and soil salinization is known to be one of them. Organic amendment improves plant growth and yield by modifying ionic homeostasis, photosynthetic apparatus, and antioxidant machineries, and by reducing oxidative damages (Hoque et al., 2022). However, the beneficial regulatory role of organic amendments in plants and their stress mitigation mechanisms has received insufficient attention. The emphasis in this section is, therefore, on addressing the adverse effects of residue burning on soil salinization, along with the follow-up action required for an eco-friendly approach to residue management.
Among the various options for managing crop residues, crop residue burning is a prevalent economical method practiced by most farmers, leading to secondary salinity. Burning on the soil surface interferes with its nutrient cycling and physical, chemical, and biological properties (Certini, 2005). The impact of residue burning is expressed more on the top layer of soil having leaf litter as organic matter and although the availability of some nutrients (K, Ca, and Mg) being enhanced due to its combustion, while several major nutrients become volatilized (N, P, and S). The cation exchange capacity (CEC), aggregate stability, aeration, infiltration, and microbial diversity of soil are affected due to the loss of its organic matter (Bhuvaneshwari et al., 2019). Compared to natural decomposition in the soil environment, residue burning on the surface of soil often leads to the rapid mineralization and volatilization of many essential nutrients (Bhuvaneshwari et al., 2019). An increased soil temperature (34–42°C) resulting from crop stubble burning results in a significant loss of an essential nutrient from the topsoil, namely, nitrogen. Residue burning in a long-term manner has resulted in a reduction of total nitrogen in the topsoil layer (0–15 cm) (Gupta et al., 2003). In a laboratory experiment, a temperature of 250°C lasting 15 min was enough to reduce OM (Jain et al., 2014; Thomaz and Fachin, 2014). Furthermore, the burning of crop residues also influences the acidity of soil as it increases the soil’s electrical conductivity (EC) and pH (Huang et al., 2012). Generally, due to the presence of soluble salts, soil salinity hinders water extraction by plant roots due to elevated osmotic pressure on the former, which leads to physiological drought. An increase in soil CEC has been reported, along with an elevated level of other nutrients on residue-treated soils, compared to control groups (Ogbodo, 2011). Thus, it can be concluded that crop residue burning may lead to an increase in soil salinity. The long-term continuous use of this practice can result in a considerable reduction in soil organic C and N. It may lead to detrimental effects on some soil properties and a loss of productivity.
Crop residue recycling improves soil physical (e.g., structure, infiltration rate, plant available water capacity, and soil strength), chemical (e.g., nutrient cycling, cation exchange capacity, and soil reaction), and biological (e.g., SOC sequestration, microbial biomass C, and activity and species diversity of soil biota) quality (Singh et al., 2010; Singh, 2018; Goswami et al., 2020). Long-term residue management also seems promising in reducing soil salinity (Mishra et al., 2018b). Therefore, strategic research on mixed residue recycling needs to be conducted in salt-affected soils to precisely capture the evidence and generate recommendations for management.
As a finely grained and highly porous soil amendment, biochar is often distinguished from other charcoal-based soil amendments (Ali et al., 2017). Biochar has been found to be produced under specific heat treatment for the optimization of its specific characteristics, such as a high specific surface area (SSA) and tenacity in soils, circumventing its biological decay (Ali et al., 2017). Its ability to adsorb and retain soil moisture and essential nutrients is mainly based on the porous nature of the amendment (Warnock et al., 2007; Zhang et al., 2014). Due to the presence of surface functional groups (acidic, i.e., carboxylic, phenolic, and lactones; basic-ketones, pyrones, and chromes) produced during pyrolysis (Roy et al., 2022), biochar retains an essential property of adsorption, and this property makes it a suitable and economical option in soil salinity amelioration through the removal and sorption of harmful organic compounds (Ali et al., 2017). The application of biochar in saline soil amelioration, in many ways, is discussed further in this section.
Increased stomatal conductance and reduced abscisic acid (ABA) are often correlated with biochar-mediated plant growth enhancement under salt stress. Many recent studies have concluded that biochar application is the reason behind improved stomatal conductance in crops (viz., tomato and wheat) (Akhtar et al., 2015b). Studies have reported decreased ABA levels with the application of biochar (Akhtar 2015a), but this is not applicable to all soil types. Many researchers have witnessed a decrease in ABA content due to the synergistic effect of biochar and PGPR under salinity stress (Akhtar, 2015b; Hafez et al., 2019), hence supporting plant growth and development under salinity stress.
By interfering with the ionic balance, excess salt causes toxicity in plants. In particular, sodium has been seen to lead to the deterioration of soil physical properties, such as poor soil structure, reduced hydraulic conductivity, and surface permeability, which have been found to have a direct or indirect effect on soil erosion (Sun et al., 2020). It has been reported that sodium ion toxicity negatively impacts soil aggregate stability due to replacement of calcium ions from cation exchange sites (Lashari et al., 2015; Sun et al., 2020). Biochar may represent a cost-effective solution to soil salinity mitigation in agricultural as well as contaminated soils due to its salt sorption capacity, thereby enhancing the CEC of soil when applied with proper doses and combinations (Liang et al., 2010; Kim et al., 2016). Biochar has also been seen to ameliorate salinity stress by reducing Na+ uptake in plants (Thomas et al., 2013; Lashari et al., 2015).
Biochar application can act as an effective ameliorant against hazardous abiotic stress, such as salinity stress, by nourishing overall soil properties directly related to Na removal, as mentioned in Section 5.2 (Sun et al., 2016). According to reports, biochar application in its various forms (composted biochars or pyroligneous solutions) can affect the enzymatic activities of salt-affected soils, which also depends on the rate and time of application of the biochar, along with the group of enzymes under study (Rizwan et al., 2016a; Guanming et al., 2017; Luo et al., 2017). When applied with organic matter, biochar shows the significant enhancement of soil organic matter, as well as CEC, and reduces the exchangeable Na and pH of saline soils (Bhaduri et al., 2016; Rizwan et al., 2016b; Ali et al., 2017). Biochar and fulvic acid have been found to improve the overall soil physical and chemical properties, which further adds to the multiple benefits of salt reduction, nutrient supply, water retention, and crop growth (Sun et al., 2020). Biochar, used alone or in various combinations, has been found to enhance the overall physicochemical and biological properties of soil, as summarized in Table 3.
TABLE 3. Role of biochar in improving crop tolerance to salinity stress when applied alone or in combinations.
Crop production in many parts of the world has declined as a result of soil salinization due to the negative impacts of excessive salts on the water-absorbing capacity of plant roots, which is primarily due to very high concentrations of ions and total dissolved solids (TSSs) in the soil solution (Lashari et al., 2015; Xu et al., 2016). Additionally, salinity causes several nutritional disorders and yield losses by limiting essential nutrient uptake by crop plants (Grattan and Grieve, 1998; Sun et al., 2020). Due to its mineral and water sorption capacity, biochar has been considered an effective amendment in enhancing crop tolerance to salt toxicity (Lashari et al., 2015; Sun et al., 2020). It has been reported that biochar application improves available soil phosphorus in less fertile soils (Subedi et al., 2016). According to Sun et al. (2020), biochar and fulvic acid can release nutrients to the soil for plant utilization under salinity stress. Biochar application has been considered a potential tool for plant nutrient availability, even under salt stress (Table 3). According to reports, the application of biochar has been seen to raise soil pH to some extent, which could hinder crop growth in alkaline as well as calcareous soils (Zhang et al., 2014). Therefore, complete laboratory evaluation of the biochar amendment and the targeted soil should be performed before its application to crop fields.
Physical methods, such as surface and subsurface drainage and the application of chemical amendments, are viable remedies to reclaim these soils, but they are either costly or sometimes comparatively inefficient. The agroforestry system has been identified as one of the most suitable environment-friendly soil remediation strategies against land degradation problems as the former has been found to be comprised of multiple components, such as plantation, crops, and horticultural crops, as well as animal husbandry and aquaculture (Dagar and Minhas, 2016). The main environmental benefits of agroforestry systems are carbon sequestration and water quality enhancement, which often lead to an improved soil across all environments (Dagar, 2014; Kumar et al., 2015). Many successful experiments have been performed in India on ameliorating salt-affected soils through the practice of tree plantations and agroforestry on those soils (Kumar et al., 2020). A 20-year-old tree plantation (Prosopis julifera, Acacia nilotica, Terminalia arjuna, Albizzia lebbeck, and Eucalyptus tereticornis) in alkali soil was found to be effective in the amelioration of salt in a salt-affected soil (Dagar, 2014). Significant decreases in soil pH and EC levels have been reported under different plantations of varying ages. It has been reported that silvopastoral and tree plantation systems have improved soil organic carbon and, thereby, microbial activity when adopted in sodic soils (Dagar, 2014; Arya and Lohara, 2016; Gupta and Dagar, 2016). Reported a considerable carbon sequestration potential of 157.26 Mg C ha−1 for the plantation of Grevillea robusta raised on sodic soil over 25 years. A decrease of 3.39 dS/m in the EC level and a 23% increase in SOC have reported an 8-year-old intercropped Eucalyptus plantation at 3 m × 3 m spacing (Kumar et al., 2019). Basavaraja et al. (2010) estimated a decrease of 1.3 in soil pH in a 10-year-old Acacia nilotica plantation. A decrease in the range of 0.90–2.45 in pH and 1.05–1.41 dS m−1 in the soil EC level for agroforestry systems of Prosopis juliflora, Terminalia arjuna, and Tectona grandis with rice-berseem cropping pattern being observed by Kaur et al. (2020a) and Kaur et al. (2020b). These studies demonstrate that tree plantations and agroforestry systems can efficiently be applied as remediation and restoration strategies for degraded and marginal lands (Table 4).
Excess salt interferes with plant biology, viz., photosynthesis, protein synthesis, and lipid metabolism (Kumar and Verma, 2018). Because microorganisms communicate with plants and support the living environment, they are considered to constitute an essential part of plant defense to deter numerous stresses. Microorganisms can live in various conditions and thus have an immense capacity to minimize stress through their metabolism, such as salinity (Meena et al., 2017; Kerry et al., 2018; Das et al., 2020a). As an important indicator of soil health, soil microbial biomass (SMBC) plays a major role in recycling organic matter and enhancing soil fertility (Xu et al., 2020). Salinity has adverse effects on microbial structure, composition, and functions. The application of microorganisms as bio-fertilizer under saline soil promotes agriculture yield and soil status (Daliakopoulos et al., 2016; Tiwari et al., 2016). Owing to their versatility, ubiquity, and unmatchable capacity, microbes offer an innovative and feasible option in saline agricultural technology (Singh et al., 2011; Singh et al., 2016). Such microbes are often termed plant growth-promoting microbes (PGPMs). Various microbes have been reported for their tremendous properties under saline conditions.
The saline stress environment exceeds plant hormone ethylene levels, known as stress ethylene (Kumar A. et al., 2020). Stress ethylene is generally detrimental to plant growth and is frequently involved in introducing chlorosis, senescence, and abscission. As an instant precursor of ethylene biosynthesis, aminocyclopropane-1-carboxylate (ACC) is found to be hydrolyzed to microbes utilizing α-ketobutyrate and ammonium ions (NH4+) due to the activity of an enzyme present in microbes known as 1-aminocyclopropane-1-carboxylate deaminase (ACCD) (Glick, 2014; Ansari et al., 2019). Various ACCD-producing PGPMs have been reported in recent years for their beneficial effects and minimizing stress ethylene concentration under different abiotic and biotic stress environments by colonizing plant roots under saline environments (Vimal et al., 2017; Ansari et al., 2019). Plant–microbe interactions under saline stress exert beneficial effects both on the plants and microbes involved as microorganisms containing ACC deaminase activity minimize ethylene concentration due to ACCD activity and the plants provide a source of nutrition to the microbes via the intermediates produced due the enzymatic activity (α-ketobutyrate and NH4+) (Figure 2). Many PGPMs have been reported to produce exo-polysaccharides (EPSs), which form a biofilm and protect plants from hydric stress, enhance water retention potential, and maintain the mass of carbon sources (Upadhyay et al., 2011; Mukherjee et al., 2019). The microbial EPSs stabilize soil aggregates by binding Na+ and Cl− ions and hence improve nutrient and water transmission movement across plant roots (Figure 2). The microbial production of IAA induces the root architecture to enhance nutrient uptake and improves plant health under salt stress (Mukherjee et al., 2019; Bhat et al., 2020). Some microbes possess antioxidant enzyme complexes (AOX), such as CAT, monodehydroascorbate reductase (MDHAR), dehydroascorbate reductase (DHAR), glutathione S-transferases (GST), and APX, resulting in the hydrolysis of ROS, such as catalase and peroxidases, thus reducing salinity toxicity (Figure 2). Microbes enhance osmolyte accumulation, such as proline and sugars, in plants and increase the K+ level and maintain the Na+/K+ ratio (Ruppel et al., 2013; Bhat et al., 2020). Microbe-mediated saline stress management has been reported in many crops in various geographical locations (Table 5). These microbes show potential biological solutions to promote plant architecture under saline stresses. The commercial application of microbial formulations in saline agro-ecosystems is a latent approach for saline agriculture (Mukherjee et al., 2019; Kumar A. et al., 2020).
FIGURE 2. Plant growth-promoting bacteria (PGPB) mediate the salinity stress mitigation mechanism in plants via exopolysaccharide (EPS) secretion and the production of an antioxidant enzyme complex (AOX) to reduce reactive oxygen species (ROS) in cell organelles and ACC deaminase (ACCD), which reduces the stress ethylene in salt-stressed cells. The concept of the figure was inspired by Egamberdieva et al. (2019), Narsing Rao et al. (2019), and Soni et al. (2018).
Plant hormones are organic substances produced by plants that stimulate a physiological response. When synthesized chemically, they are called plant growth regulators (PGRs). Ethylene, auxin (IAA), abscisic acid (ABA), jasmonic acid (JA), and salicylic acid (SA) are just a few of these. Survival under biotic and abiotic stress conditions involves sophisticated crosstalk between phytohormones (Koo et al., 2020). Cell ion homeostasis disturbed due to salt stress increases cytosolic the free Ca2+ ion concentration. ABA signals a secondary messenger, Ca2+, to be released from membrane channels or vacuoles into the cytoplasm, which helps cope with high Na+ concentrations (Quan et al., 2007). ROS, essential signaling molecules, together with ABA and Ca2+, exhibit complex signaling crosstalk to control plant growth responses to salt stress. Indole acetic acid improves the number of photosynthetic pigments that promote carbohydrate formation and thus increases stem diameter, an essential parameter in the case of fodder crops (Waheed et al., 2014). Salt stress induces physiological stress and reduces water absorption by roots, the partial closure of stomata, restricted photosynthesis, and the accumulation of ROS (Wang et al., 2016). It has been suggested that, to enhance plant tolerance to soil salinity, antioxidants such as ascorbic acid and glutathione promote the homeostasis of Na+/K+ and ROS (Tao et al., 2015). Sulfur is an integral part of proteins and hormones necessary for oilseeds and the active metabolism of nitrogen, which is improved by salicylic acid spray (Rajabi Dehnavi et al., 2019).
According to the activation of non-selective cation channels (NSCCs) through ROS, which causes K+ leakage, can be controlled by SA application to plants. It has been reported that silicon (Si) application can directly or indirectly mitigate oxidative stress in plant cells via the regulation of the antioxidant defense system and the metabolism of polyamines (Liang et al., 2010; Zhu et al., 2019). The addition of Si in chickpeas has resulted in the reduced absorption and translocation of Na+ due to enhanced H+-ATPase activities in the vacuolar membrane of the plant cells. Studies over the decades have shown a beneficial effect of several natural and synthetic PGRs on plant growth and regulation; some of these are listed in Supplementary Table S1.
The uncontrolled and excess use of agrochemical fertilizers has become the primary threat to soil health, leading to extreme salinity in the soil. Currently, there is a paucity of information on the impact of nano-materials on soil salinity management. Nanoparticles are widely agreed to be a cluster of atoms in the size range of 1–100 nm (Pan and Xing., 2012; Kerry et al., 2017; Kerry et al., 2019). It is also known that nanoparticles have a high surface-to-volume ratio, and their bioavailability could be significantly greater than larger particles (Shalaby et al., 2016). Their large surface area enhances the reactivity and easier delivery of particles to porous soils, making nano-materials significant amendments for soil reclamation (Liu and Lal, 2012). Researchers have used nanoparticles as a nutrient carrier, but there is also a concern that they may be lethal, depending upon the quantity of application and the nature of the nanoparticles. Furthermore, the abundance and diversity may shift in response to foreign nano-materials in the soil. The idea of nanoparticles in the soil can be attributed to nano-fertilizers and is one of the most promising methods to reduce environmental pollution (DeRosa et al., 2010).
Nano-iron oxide treatment in saline soil has also improved the mean weight diameter and infiltration rate (Ghodsi et al., 2011) and has facilitated the formation of soil macroaggregates and microaggregates (Liu and Lal, 2012). A study concluded that the controlled application of nano-materials in the rhizosphere significantly enhances the microbial population; it was observed that, through the application of 100 mg kg−1 nano-Fe2O3 and nano-ZnO treatments at an elevated CO2 level, the microbial biomass carbon was highest. In contrast, a sharp decrease was noted at a higher concentration compared to the ambient CO2 level (Yadav et al., 2014). Mimicking the saline environment by applying NaCl with varying concentrations, various researchers have studied the impact of no fertilizer, typical silica fertilizer, and silica nanoparticles on soil health. According to reports, sodium chloride (NaCl) induces soil salinity and reduces plant growth, but when soil salinity is combined with Si nanoparticles, enhancement in chlorophyll content is observed, along with the fresh and dry weight of the shoots (Kalteh et al., 2014; Tantawy et al., 2015).
The availability of micronutrients in saline soil is the most common problem that arises due to abundant calcium salt, which negatively impacts cop production. Higher alkaline pH reduces zinc availability in the soil. In contrast, ZnO application in the form of nanoparticles can solve the problem due to the provision of a more soluble form of Zn with comparatively higher reactivity than bulk with higher calcium incidence (Duhana et al., 2017). Recently, Hussein and Abou-Baker (2018) investigated the influence of nano-zinc fertilizer application on the mineral status as well as the growth and yield of Gossypium barbadense L. under salinity stress. The results corroborated that the negative impact of adverse salinity on crop production could be mitigated by nano-Zn (Hussein and Abou-Baker, 2018). An experiment by Avestan et al. (2019) showed that the treatment of nano-silicon dioxide could effectively alleviate tolerance to salt stress in Fragaria anansa. Under salinity stress, an acute ionic imbalance or ionic toxicity is observed in the plant rhizosphere, which hinders balanced nutrition in the plant, thereby restricting its growth and yield as described previously. In this section, we describe how nanoparticles can be used as a carrier of many vital nutrients needed for plants that can be carried quickly due to their small molecular size inside the plant cells, thereby maintaining the ionic homeostasis in many of the cell organelles, such as chloroplasts, mitochondria, endoplasmic reticulum, vacuoles, and cytoplasm (Figure 3). Researchers are trying to fill the gaps regarding nano application and interaction with plants owing to the fact that their fate must be conceived on the geosphere (pedosphere)–biosphere–hydrosphere–atmosphere continuum; the time lag of emerging technologies reaching farmers’ fields, especially given that many emerging economies are unwilling to spend on innovation; and the lack of foresight due to agricultural education not attracting a sufficient number of brilliant minds from around the world, while personnel from related disciplines may lack the required understanding. Recently, the United States Environmental Protection Agency (USEPA), the Food and Drug Administration (FDA), and the Institute for Food and Agricultural Standards (IFAS) in the United States have developed protocols and procedures for dealing with nano-material production and use, as well as potential threats to people, animals, and the environment. The product concentration, particle size, shape, surface area, crystal structure, dose, exposure time, pre-exposure, and crystal structure effect are the factors used to assess the toxicity of nano-materials. It is necessary to ascertain the tolerable safety limits and toxicity of nanoparticles toward the soil.
FIGURE 3. Higher salt concentration in plant cells results in a salt deposition in the leaf, leading to reactive oxygen species (ROS) accumulation in the chloroplasts, mitochondria, and nucleus. ROS accumulation in sub-cellular organelles may further lead to DNA damage and lipid peroxidation, which can be neutralized by applying nanotechnology, specifically nano-delivery systems via the induction of antioxidant production and/or the direct inhibition of ROS production. The concept of the figure was inspired by Avestan et al. (2019) and Hussein and Abou-Baker (2018). The figure template was inspired by Berru (2007), Forluvoft (2008), LadyofHats (2013), LadyofHats (2006), McKenna (2006), Qef (2009), and Rifqi (2017).
For mapping wasteland areas, RS and GIS data have been extensively used (Allbed and Kumar, 2013) and have led to the successful reduction of soil salinity through various recovering activities, from site selection to soil monitoring and soil monitoring to groundwater monitoring. The National Remote Sensing Centre (NRSC) has prepared wasteland maps of most of the Indian states based on visual interpretation of IRS P6 LISS-III and LISS-IV geocoded satellite imagery on a 1:50,000 scale since 1985 (Sahu et al., 2016). RS, GISs, modeling, geo-statistics, and advanced electromagnetic induction are the preeminently discussed tools for assessing, mapping, and monitoring soil salinity (Srivastava and Saxena, 2004). Various kinds of spatial and non-spatial data for wasteland management in a particular district or region can be captured, stored, manipulated, and analyzed via RS and GISs. RS technology is time-saving, faster than ground methods, and gives comprehensive coverage facilitating long-term monitoring. Multispectral and hyperspectral sensors have been used for mapping and ascertaining soil salinity (Allbed and Kumar, 2013).
The conceptual basis for RS monitoring has been fabricated upon the spectral characteristics of saline soils. Spectral analysis denotes a detailed examination of the electromagnetic radiation reflected by the target to determine its properties (Harti et al., 2016). The spectral analysis of salt-affected soils serves as a non-destructive, less laborious, and time-saving technique for monitoring the salt-affected soils on a large area’s spatial and temporal basis, which may further be used for preparing maps and providing early warnings, as well as for determining the feasibility of any reclamation measures. The spectral analysis of bare soils has revealed that salt-affected soils have higher reflectance in the wavelength range of 0.4–0.9 μm compared to average soils (Castaldi et al., 2016). Salt-affected soils having a surface crust show the highest reflectance, followed by salt-affected soils without a crust, whereas ploughed soil shows the most negligible reflectance (Harti et al., 2016). The reflectance from the saline soil increases with an increase in the severity of the salinity (Kahaer and Tashpolat, 2019). The l-band of the microwave radiation can distinguish between saline and sodic soils at varying moisture levels because the imaginary part of the complex permittivity increases with an increase in electrical conductivity (EC). At the same time, the sodium adsorption ratio (SAR) does not affect either the real or imaginary parts (Sahu et al., 2016). Vegetation indirectly indicates the characteristics of salt-affected soils due to its retarded growth (Masimbye et al., 2012) and increased reflectance in the wavelength ranging from 0.9 to 2.5 μm caused by the increased thickness of the leaves (Sidike et al., 2014). Methods in soil salinity mapping involve ground truthing with field observations from the visual interpretation of satellite imageries collected from RS data (Nguyen et al., 2020). The ground truth observations point to a way forward toward soil sampling and analysis (soil characterization, and physical and chemical analyses) in affected regions by interpreting which soil salinity map is being prepared (Figure 4).
FIGURE 4. Methodology for soil salinity mapping (Abuelgasim and Ammad, 2019; Taghadosi et al., 2019).
Soil degradation, particularly through salinity, impedes the productivity of croplands and thereby poses challenges in securing livelihoods. A multidimensional approach has shown promising results in reducing soil salinity in different Indian agroecological situations. Strategic and adaptive research on the incorporation of modern technologies, salt-tolerant varieties, biochar, PGPR, and nanoparticles will be critical in amending salt-affected soil and increasing the productivity of degraded land. Because of diversification into other sectors, cultivable land in India is shrinking; the restoration, amelioration, and management of salt-affected soils appear promising for land expansion and production enhancement to ensure food and livelihoods.
Soil quality characteristics are commonly used to assess sustainable land management in agro-ecosystems. Over the last two decades, several land management studies have been conducted in India and elsewhere regarding reducing the salinity and improving the productivity of the land. Unfortunately, there is insufficient data to provide critical remarks on the best working and ecofriendly techniques that should be practiced for salinity remediation in the enhancement of crop production. Plant-associated microorganisms as biofertilizers can promote agriculture yield and soil status under saline soil (Das et al., 2020). These organisms constitute endophytic microbes and symbiotic fungi in the rhizoplane and rhizosphere and they act in many ways, such as initiating the osmotic reaction, growth hormones, and nutritional elements, working as biocontrol agents by inducing specific genes in crops. Biochar and crop residues could be used to ameliorate salinity stress by improving the physical and thereby biological status of the soil and reducing the uptake of Na+ by plants. At present, nanotechnology is being adapted for specific nutrient availability purposes, and it is also being used for conserving soil fertility and reducing the impacts of salinity from the soil. Many studies have revealed that tree plantations and agroforestry systems could efficiently be applied as remediation and restoration strategies for degraded and marginal lands. Many salt-tolerant species or halophytes with potential agricultural or industrial value can be grown in degraded saline areas as cash crops or commercial crops. Proper land evaluation is considered an essential component for managing any degraded lands, including salt-affected ones, to model the best suitable cropping practices to achieve a sustainable agricultural system. In the field of salinity, research is needed, particularly on land evaluation and opportunities for salinity management in the agro-ecosystem to enhance land productivity. Globally, soil salinity poses a severe menace to food security and threatens millions of small and marginal farmers’ livelihoods. Low-cost, need-based, site-specific restoration and amelioration programs should be strategically planned and implemented in order to counter soil salinity. Developmental research on integrating modern technologies, salt-tolerant varieties, biochar, PGPR, and nano-gypsum will be of great significance in reducing salt-affected soil and increasing the productivity of degraded lands. In addition to technological solutions, socio-economic and political considerations are critical in mitigating soil salinization. Farmers need to be provided with various incentives rather than subsidies to undertake corrective salinity measures.
AM: conceptualization, writing and supervision of the manuscript, and critical revision. RD: data collection, interpretation, writing original draft, and graphics. RG: data curation, and graphics and editing. BB: table incorporation and editing. TS: tabular information and editing. SS: review and editing. PA: review and editing. MK: critical review.
The first author is thankful to the Ministry of Education, Culture, Sports, Science and Technology (MEXT) Scholarship for providing them with the opportunity to pursue doctoral studies in Japan. The authors would also like to thank their institutes and heads for the encouragement to write this review on the burning issue of salinity management in the era of climate change.
The authors declare that the research was conducted in the absence of any commercial or financial relationships that could be construed as a potential conflict of interest.
All claims expressed in this article are solely those of the authors and do not necessarily represent those of their affiliated organizations, or those of the publisher, the editors, and the reviewers. Any product that may be evaluated in this article, or claim that may be made by its manufacturer, is not guaranteed or endorsed by the publisher.
The Supplementary Material for this article can be found online at: https://www.frontiersin.org/articles/10.3389/fenvs.2022.962581/full#supplementary-material
123RF, 2020123RF (2020). 3d structure of Kinetin, a type of cytokinin, a class of plant. Available at: https://www.123rf.com/photo_69368699_3d-structure-of-kinetin-a-type-of-cytokinin-a-class-of-plant-hormone-that-promotes-cell-division-oft.html (accessed October 14, 2020).
Aamir, M., Aslam, A., Khan, M. Y., and Usman, M. (2013). Co-inoculation with rhizobium and plant growth promoting rhizobacteria (PGPR) for inducing salinity tolerance in mung bean under field conditions of a semi-arid climate. Asian J. agri. Biol. 1, 7.
Abuelgasim, A., and Ammad, R. (2019). Mapping soil salinity in arid and semi-arid regions using landsat 8 OLI satellite data. Remote Sens. Appl. Soc. Environ. 13, 415–425. doi:10.1016/j.rsase.2018.12.010
ACS. Brassinolide (2011). American chemical society (ACS). Chem. Life. Am. Chem. Soc. Available at: https://www.acs.org/content/acs/en/molecule-of-the-week/archive/b/brassinolide.html (accessed October 14, 2020).
Ahmed, S., Ahmed, S., Roy, S., Woo, S., Sonawane, K., and Shohael, A. (2019). Effect of salinity on the morphological, physiological and biochemical properties of lettuce (Lactuca sativa L.) in Bangladesh. Open Agric. 4 (1), 361–373. doi:10.1515/opag-2019-0033
Akhtar, S. S., Andersen, M. N., and Liu, F. (2015a). Biochar mitigates salinity stress in potato. J. Agron. Crop Sci. 201, 368–378. doi:10.1111/jac.12132
Akhtar, S. S., Andersen, M. N., Naveed, M., Zahir, Z. A., and Liu, F. (2015b). Interactive effect of biochar and plant growth-promoting bacterial endophytes on ameliorating salinity stress in maize. Funct. Plant Biol. 42, 770–781. doi:10.1071/fp15054
Akladious, S. A., and Mohamed, H. I. (2018). Ameliorative effects of calcium nitrate and humic acid on the growth, yield component and biochemical attribute of pepper (Capsicum annuum) plants grown under salt stress. Sci. Hortic. 236, 244–250. doi:10.1016/j.scienta.2018.03.047
Ali, S., Rizwan, M., Qayyum, M. F., Ok, Y. S., Ibrahim, M., Riaz, M., et al. (2017). Biochar soil amendment on alleviation of drought and salt stress in plants: A critical review. Environ. Sci. Pollut. Res. 24, 12700–12712. doi:10.1007/s11356-017-8904-x
Allbed, A., and Kumar, L. (2013). Soil salinity mapping and monitoring in arid and semi-arid regions using remote sensing technology: A review. Adv. remote Sens. 2 (4), 13. doi:10.4236/ars.2013.24040
Ansari, F. A., Ahmad, I., and Pichtel, J. (2019). Growth stimulation and alleviation of salinity stress to wheat by the biofilm forming Bacillus pumilus strain FAB10. Appl. Soil Ecol. 143, 45–54. doi:10.1016/j.apsoil.2019.05.023
Arthur, J. S. (2006). Fijałkowskipl wiki: WarXcommonsWarXmail: [1]jabber: WarX@jabber orgirc: [2]consultations: Masur. Lipid raft organisation scheme.
Arya, R., and Lohara, R. R. (2016). Enhancing forest productivity through afforestation on arid salt affected sandy soils in Rajasthan. J. Agri. Ecol. 1, 59–70. doi:10.53911/jae.2016.1107
Ashraf, M., and Foolad, M. R. (2007). Roles of glycine betaine and proline in improving plant abiotic stress resistance. Environ. Exp. Bot. 59 (2), 206–216. doi:10.1016/j.envexpbot.2005.12.006
Aslam, F., and Ali, B. (2018). Halotolerant bacterial diversity associated with Suaeda fruticosa (L.) forssk. improved growth of maize under salinity stress. Agron 8, 131. doi:10.3390/agronomy8080131
Asthir, B., Kaur, G., and Kaur, B. (2020). Convergence of pathways towards ascorbate–glutathione for stress mitigation. J. Plant Biol. 63, 243–257. doi:10.1007/s12374-020-09253-7
Avestan, S., Ghasemnezhad, M., Esfahani, M., and Byrt, C. S. (2019). Application of nano-silicon dioxide improves salt stress tolerance in strawberry plants. Agron 9, 246. doi:10.3390/agronomy9050246
Bano, A., and Fatima, M. (2009). Salt tolerance in Zea mays (L). following inoculation with Rhizobium and Pseudomonas. Biol. Fertil. Soils 45, 405–413. doi:10.1007/s00374-008-0344-9
Banyal, R., Kumar, M., Yadav, R. K., and Dagar, J. C. (2017). Agroforestry for rehabilitation and sustenance of saline ecologies. Agrofor, 413–454.
Barassi, C. A., Ayrault, G., Creus, C. M., Sueldo, R. J., and Sobrero, M. T. (2006). Seed inoculation with Azospirillum mitigates NaCl effects on lettuce. Sci. Hortic. 109, 8–14. doi:10.1016/j.scienta.2006.02.025
Barnawal, D., Bharti, N., Maji, D., Chanotiya, C. S., and Kalra, A. (2014). ACC deaminase-containing Arthrobacter protophormiae induces NaCl stress tolerance through reduced ACC oxidase activity and ethylene production resulting in improved nodulation and mycorrhization in Pisum sativum. J. Plant Physiol. 171, 884–894. doi:10.1016/j.jplph.2014.03.007
Bartels, D., and Sunkar, R. (2005). Drought and salt tolerance in plants. Crit. Rev. Plant Sci. 24, 23–58. doi:10.1080/07352680590910410
Basavaraja, P. K., Sharma, S. D., Dhananjaya, B. N., and Badrinath, M. S. (2010). “Acacia nilotica: A tree species for amelioration of sodic soils in central dry zone of Karnataka, India,” in Proceedings of the 19th world congress of soil science (Brisbane, Australia: Soil Solutions for a Changing World), 73–76.
Berru, M. R. L. (2007). Translation by. Français: Diagramme du système endomembranaire d’une cellule eukaryote.
Bhaduri, D., Saha, A., Desai, D., and Meena, H. N. (2016). Restoration of carbon and microbial activity in salt-induced soil by application of peanut shell biochar during short-term incubation study. Chemosphere 148, 86–98. doi:10.1016/j.chemosphere.2015.12.130
Bharti, N., Pandey, S. S., Barnawal, D., Patel, V. K., and Kalra, A. (2016). Plant growth promoting rhizobacteria Dietzia natronolimnaea modulates the expression of stress responsive genes providing protection of wheat from salinity stress. Sci. Rep. 6, 1–16. doi:10.1038/srep34768
Bhat, M. A., Kumar, V., Bhat, M. A., Wani, I. A., Dar, F. L., Farooq, I., et al. (2020). Mechanistic insights of the interaction of plant growth-promoting rhizobacteria (PGPR) with plant roots toward enhancing plant productivity by alleviating salinity stress. Front. Microbiol. 11, 1952. doi:10.3389/fmicb.2020.01952
Bhuvaneshwari, S., Hettiarachchi, H., and Meegoda, J. N. (2019). Crop residue burning in India: Policy challenges and potential solutions. Int. J. Environ. Res. Public Heal. 16 (5), 832. doi:10.3390/ijerph16050832
Bloemberg, G. V., and Lugtenberg, B. J. J. (2001). Molecular basis of plant growth promotion and biocontrol by rhizobacteria. Curr. Opin. Plant Biol. 4, 343–350. doi:10.1016/s1369-5266(00)00183-7
Carrillo-Castaneda, G., Munos, J. J., Peralta-Videa, J. R., Gomez, E., Tiemannb, K. J., Duarte-Gardea, M., et al. (2002). Alfalfa growth promotion by bacteria grown under iron limiting conditions. Adv. Environ. Res. 6, 391–399. doi:10.1016/s1093-0191(02)00054-0
Castaldi, F., Palombo, A., Santini, F., Pascucci, S., Pignatti, S., and Casa, R. (2016). Evaluation of the potential of the current and forth coming multispectral and hyperspectral imagers to estimate soil texture and organic carbon. Remote Sens. Environ. 179, 54–65. doi:10.1016/j.rse.2016.03.025
Certini, G. (2005). Effects of fire on properties of forest soils: A review. Oecol 143, 1–10. doi:10.1007/s00442-004-1788-8
Chang, P., Gerhardt, K. E., Xiao-Dong, H., Xiao-Ming, Y., Glick, B. R., Gerwing, P. D., et al. (2014). Plant growth promoting bacteria facilitate the growth of barley and oats in saltimpacted soil: Implications for phytoremediation of saline soils. Int. J. Phytorem. 16, 1133–1147. doi:10.1080/15226514.2013.821447
Chen, J., Zou, X., Liu, Q., Wang, F., Feng, W., and Wan, N. (2014). Combination effect of chitosan and methyl jasmonate on controlling Alternaria alternata and enhancing activity of cherry tomato fruit defense mechanisms. Crop Prot. 56, 31–36. doi:10.1016/j.cropro.2013.10.007
Choudhary, A. K., Sultana, R., Vales, M. I., Saxena, K. M., Kumar, R. R., and Ratnakumar, P. (2018). Integrated physiological and molecular approaches to improvement of abiotic stress tolerance in two pulse crops of the semi-arid tropics. Crop J. 6, 99–114. doi:10.1016/j.cj.2017.11.002
Cook, S. D. (2019). An historical review of phenylacetic acid. Plant Cell. Physiol. 60 (2), 243–254. doi:10.1093/pcp/pcz004
Cruz de Carvalho, M. H. (2008). Drought stress and reactive oxygen species: Production, scavenging and signaling. Plant signal. behave 3 (3), 156–165. doi:10.4161/psb.3.3.5536
Dagar, J. C., and Minhas, P. S. (2016). “Introduction,” in Agroforestry for the management of waterlogged saline soils and poor-quality waters. Advances in agroforestry. Editors J. Dagar, and P. Minhas (New Delhi: Springer), 13.
Dagar, J. C. (2014). “Greening salty and waterlogged lands through agroforestry systems for livelihood security and better environment,” in Agroforestry systems in India: Livelihood security & ecosystem services (New Delhi: Springer), 273–332.
Daliakopoulos, I. N., Tsanis, I. K., Koutroulis, A., Kourgialas, N. N., Varouchakis, A. E., Karatzas, G. P., et al. (2016). The threat of soil salinity: A European scale review. Sci. Total Environ. 573, 727–739. doi:10.1016/j.scitotenv.2016.08.177
Dardanelli, M. S., Fernandez de Cordoba, F. J., Espuny, M. R., Carvajal, M. A. R., Diaz, M. E. S., Senero, A. M. G., et al. (2008). Effect of Azospirillum brasilense coinoculated with rhizobium on Phaseolus vulgaris flavonoids and nod factor production under salt stress. Soil Biol. biochem. 40, 2713–2721. doi:10.1016/j.soilbio.2008.06.016
Das, R., Mishra, S., Kumar, M., Singh, C., Tiwari, S., and Singh, J. S. (2020a). “Plant–microbe association leading to a sustainable agroecosystem,” in Microbes in agriculture and environmental development. Editor A. Nath (Boca Raton, London, Newyork: CRC Press, Taylor and Francis Group), 1–24.
Das, R., Pradhan, M., Sahoo, R. K., Mohanty, S., and Kumar, M. (2020b). Isolation, identification and role of novel endosymbiotic bacterium rhizobium pusence in root nodule of green gram cv.OUM-11-15 (vigna radiata L.) under salinity stress. Legum. Res. doi:10.18805/LR-4239
DeFalco, T. A., Bender, K. W., and Snedden, W. A. (2009). Breaking the code: Ca2+ sensors in plant signalling. Biochem. J. 425, 27–40. doi:10.1042/bj20091147
Deinlein, U., Stephan, A. B., Horie, T., Luo, W., Xu, G., and Schroeder, J. I. (2014). Plant salt-tolerance mechanisms. Trends Plant Sci. 19 (6), 371–379. doi:10.1016/j.tplants.2014.02.001
del Amor, F., and Cuadra-Crespo, P. (2012). Plant growth-promoting bacteria as a tool to improve salinity tolerance in sweet pepper. Funct. Plant Biol. 39, 82–90. doi:10.1071/FP11173
DeRosa, M. C., Monreal, C., Schnitzer, M., Walsh, R., and Sultan, Y. (2010). Nanotechnology in fertilizers. Nat. Nanotechnol. 5, 91. doi:10.1038/nnano.2010.2
Dimkpa, C., Weinand, T., and Asch, F. (2009). Plant– rhizobacteria interactions alleviate abiotic stress conditions. Plant Cell. Environ. 32, 1682–1694. doi:10.1111/j.1365-3040.2009.02028.x
Duhana, J. S., Kumar, R., Kumar, N., Kaur, P., Nehra, K., and Duhan, S. (2017). Nanotechnology: The new perspective in precision agriculture. Biotechnol. Rep. 15, 11–23. doi:10.1016/j.btre.2017.03.002
Egamberdieva, D., Jabborova, D., and Hashem, A. (2015). Pseudomonas induces salinity tolerance in cotton (Gossypium hirsutum) and resistance to Fusarium root rot through the modulation of indole-3-acetic acid. Saudi J. Biol. Sci. 22, 773–779. doi:10.1016/j.sjbs.2015.04.019
Egamberdieva, D., Wirth, S., Bellingrath-Kimura, S. D., Mishra, J., and Arora, N. K. (2019). Salt-tolerant plant growth promoting rhizobacteria for enhancing crop productivity of saline soils. Front. Microbiol. 10, 2791. doi:10.3389/fmicb.2019.02791
Egamberdieva, D. (2012). Pseudomonas chlororaphis: A salt-tolerant bacterial inoculant for plant growth stimulation under saline soil conditions. Acta Physiol. Plant. 34, 751–756. doi:10.1007/s11738-011-0875-9
Etesami, H., and Beattie, G. A. (2017). “Plant-microbe interactions in adaptation of agricultural crops to abiotic stress conditions,” in Probiotics and plant health, 163–200. doi:10.1007/978-981-10-3473-2_7
Etesami, H. (2018). Can interaction between silicon and plant growth promoting rhizobacteria benefit in alleviating abiotic and biotic stresses in crop plants? Agric. Ecosyst. Environ. 253, 98–112. doi:10.1016/j.agee.2017.11.007
Farahat, M. G., Mahmoud, M. K., Youseif, S. H., Saleh, S. A., and Kamel, Z. (2020). Alleviation of salinity stress in wheat by ACC deaminase-producing Bacillus aryabhatti EWR29 with multifarious plant growth-promoting attributes. Plant Arch. 20 (1), 417–429.
Fariduddin, Q., Yusuf, M., Ahmad, I., and Ahmad, A. (2014). Brassinosteroids and their role in response of plants to abiotic stresses. Biol. Plant. 58 (1), 9–17. doi:10.1007/s10535-013-0374-5
Farman, S., Mushtaq, A., and Azeem, M. W. (2019). Plant growth regulators (PGRs) and their applications: A review. Int. J. Chem. Biochem. Sci. 15, 94–103.
Farooq, M., Asif, S., Jang, Y-H., Park, J-R., Zhao, D-D., Kim, E-G., et al. (2022). Effect of different salts on nutrients uptake, gene expression, antioxidant, and growth pattern of selected rice genotypes. Front. Plant Sci. 13, 895282. doi:10.3389/fpls.2022.895282
Fathalla, A., and Abd el-mageed, A. (2020). Salt tolerance enhancement of wheat (Triticum aestivum L.) genotypes by selected plant growth promoting bacteria. AIMS Microbiol. 6 (3), 250–271. doi:10.3934/microbiol.2020016
Garg, N., and Chandel, S. (2011). Effect of mycorrhizal inoculation on growth, nitrogen fixation, and nutrient uptake in Cicer arietinum (L.) under salt stress. Turk. J. Agric. For. 35, 205–214.
Ghodsi, A., Astaraei, A. R., and Emami, H. (2011). “Effect of different amounts of nano iron oxide powder and urban solid waste compost coated sulfur on some physical properties of saline-sodic soil,” in 2nd conference on applications of nanotechnology in Sciences, engineering and medicine (NTC2011) (Mashhad-Iran. 16-17 May.
Gianfagna, T. (1995). “Natural and synthetic growth regulators and their use in horticultural and agronomic crops,” in Plant hormones. Editor P. J. Davies (Dordrecht: Springer). doi:10.1007/978-94-011-0473-9_34
Glick, B. R. (2014). Bacteria with ACC deaminase can promote plant growth and help to feed the world. Microbiol. Res. 169 (1), 30–39. doi:10.1016/j.micres.2013.09.009
Gond, S. K., Torres, M. S., Bergen, M. S., Helsel, Z., and White, J. F. (2015). Induction of salt tolerance and up-regulation of aquaporin genes in tropical corn by rhizobacterium Pantoea agglomerans. Lett. Appl. Microbiol. 60, 392–399. doi:10.1111/lam.12385
Goswami, S. B., Mondal, R., and Mandi, S. K. (2020). Crop–residue management options in rice–rice systems. Arch. Agron. Soil Sci. 66 (91), 234.
Grattan, S. R., and Grieve, C. M. (1998). Salinity–mineral nutrient relations in horticultural crops. Sci. Hort. 78, 127–157. doi:10.1016/s0304-4238(98)00192-7
Guangming, L., Xuechen, Z., Xiuping, W., Hongbo, S., Jingsong, Y., and Xiangping, W. (2017). Soil enzymes as indicators of saline soil fertility under various soil amendments. Agric. Ecosyst. Environ. 237, 274–279. doi:10.1016/j.agee.2017.01.004
Gupta, S. R., and Dagar, J. C. (2016). “Agroforestry for ecological restoration of salt-affected lands,” in Innovative saline agriculture (New Delhi: Springer), 161–182.
Gupta, B., and Huang, B. (2014). Mechanism of salinity tolerance in plants: Physiological, biochemical, and molecular characterization. Int. J. Genom. 2014, 1–18. Article ID 701596. doi:10.1155/2014/701596
Gupta, S., and Pandey, S. (2019). ACC deaminase producing bacteria with multifarious plant growth promoting traits alleviates salinity stress in French Bean (Phaseolus vulgaris) Plants. Front. Microbiol. 10, 1506. doi:10.3389/fmicb.2019.01506
Gupta, R. K., Naresh, R. K., Hobbs, P. R., Jiaguo, Z., and Ladha, J. K. (2003). Improving the Productivity and Sustainability of Rice–Wheat Systems: Issues and Impacts, 65. Wisconsin,USA: ASA Special Publication.Sustainability of post-green revolution agriculture: The rice–wheat cropping systems of the indo-gangetic plains and China
Gurmani, A. R., Bano, A., Khan, S. U., Din, J., and Zhang, J. L. (2011). Alleviation of salt stress by seed treatment with abscisic acid (ABA), 6-benzylaminopurine (BA) and chlormequat chloride (CCC) optimizes ion and organic matter accumulation and increases yield of rice ('Oryza sativa'L.). Aust. J. Crop Sci. 5 (10), 1278.
Hafez, E. M., Alsohim, A. S., Farig, M., Omara, A. E., Rashwan, E., and Kamara, M. M. (2019). Synergistic effect of biochar and plant growth promoting rhizobacteria on alleviation of water deficit in rice plants under salt-affected soil. Agron 9 (12), 847. doi:10.3390/agronomy9120847
Hamdali, H., Bouizgarne, B., Hafidi, M., Lebrihi, A., Virolle, M. J., and Ouhdouch, Y. (2008). Screening for rock phosphate solubilizing Actinomycetes from Moroccan phosphate mines. Appl. Soil Ecol. 38, 12–19. doi:10.1016/j.apsoil.2007.08.007
Hammer, E. C., Forstreuter, M., Rillig, M. C., and Kohler, J. (2015). Biochar increases arbuscular mycorrhizal plant growth enhancement and ameliorates salinity stress. Appl. Soil Ecol. 96, 114–121. doi:10.1016/j.apsoil.2015.07.014
Hanin, M., Ebel, C., Ngom, M., Laplaze, L., and Masmoudi, K. (2016). New insights on plant salt tolerance mechanisms and their potential use for breeding. Front. Plant Sci. 7, 1787. doi:10.3389/fpls.2016.01787
Harti, A. E., Lhissou, R., Chokmani, K., Ouzemou, J., Hassouna, M., Bachaoui, E. M., et al. (2016). Spatiotemporal monitoring of soil salinization in irrigated Tadla Plain (Morocco) using satellite spectral indices. Int. J. Appl. Earth Obs. Geoinf. 50, 64–73. doi:10.1016/j.jag.2016.03.008
Hoque, M. N., Imran, S., Hannan, A., Paul, N. C., Mahamud, M. A., Chakrobortty, J., et al. (2022). Organic amendments for mitigation of salinity stress in plants: A review. Life 12, 1632. doi:10.3390/life12101632
Hossain, M. A., Munemasa, S., Uraji, M., Nakamura, Y., Mori, I. C., and Murata, Y. (2011). Involvement of endogenous abscisic acid in methyl jasmonate-induced stomatal closure in Arabidopsis. Plant Physiol. 156 (1), 430–438. doi:10.1104/pp.111.172254
Hossain, M. S. (2019). Present scenario of global salt affected soils, its management and importance of salinity research. Int. Res. J. Biol. Sci. 1, 1–3.
Hou, L., Chen, X., Kuhn, L., and Huang, J. (2019). The effectiveness of regulations and technologies on sustainable use of crop residue in Northeast China. Energy Econ. 81, 519–527. doi:10.1016/j.eneco.2019.04.015
Huang, G. B., Luo, Z. Z., Li, L. L., Zhang, R. Z., Li, G. D., Cai, L. Q., et al. (2012). Effects of stubble management on soil fertility and crop yield of rainfed area in western loess plateau, China. Appl. Environ. Soil Sci. 2012, 1–9. doi:10.1155/2012/256312
Hussein, M. M., and Abou-Baker, N. H. (2018). The contribution of nano-zinc to alleviate salinity stress on cotton plants. R. Soc. Open Sci. 5, 171809. doi:10.1098/rsos.171809
Ilangumaran, G., and Smith, D. L. (2017). Plant growth promoting rhizobacteria in amelioration of salinity stress: A systems biology perspective. Front. Plant Sci. 8, 1768. doi:10.3389/fpls.2017.01768
Irizarry, I., and White, J. (2017). Application of bacteria from non-cultivated plants to promote growth, alter root architecture and alleviate salt stress of cotton. J. Appl. Microbiol. 122, 1110–1120. doi:10.1111/jam.13414
Islam, F., Yasmeen, T., Arif, M. S., Ali, S., Ali, B., Hameed, S., et al. (2016). Plant growth promoting bacteria confer salt tolerance in Vigna radiata by upregulating antioxidant defense and biological soil fertility. Plant Growth Regul. 80, 23–36. doi:10.1007/s10725-015-0142-y
Ismayilov, A. I., Mamedov, A. I., Fujimaki, H., Tsunekawa, A., and Levy, G. J. (2021). Soil salinity type effects on the relationship between the electrical conductivity and salt content for 1:5 soil-to-water extract. Sustainability 13, 3395. doi:10.3390/su13063395
Jain, N., Bhatia, A., and Pathak, H. (2014). Emission of air pollutants from crop residue burning in India. Aerosol Air Qual. Res. 14, 422–430. doi:10.4209/aaqr.2013.01.0031
Jamil, A., Riaz, S., Ashraf, M., and Foolad, M. R. (2011). Gene expression profiling of plants under salt stress. Crit. Rev. Plant Sci. 30, 435–458. doi:10.1080/07352689.2011.605739
Kahaer, Y., and Tashpolat, N. (2019). Estimating salt concentrations based on optimized spectral indices in soils with regional heterogeneity. J. Spectrosc. 2019, 1–15. doi:10.1155/2019/2402749
Kalteh, M., Alipour, Z. T., Ashraf, S., Aliabadi, M. M., and Nosratabadi, A. F. (2014). Effect of silica nanoparticles on Basil (Ocimum basilicum) under salinity stress. J. Chem. Heal. Risks. 4 (3), 49–55.
Kang, S. M., Shahzad, R., Bilal, S., Khan, L., Park, Y. G., Lee, K. E., et al. (2019). Indole-3-acetic-acid and ACC deaminase producing Leclercia adecarboxylata MO1 improves Solanum lycopersicum L. growth and salinity stress tolerance by endogenous secondary metabolites regulation. BMC Microbiol. 19, 80. doi:10.1186/s12866-019-1450-6
Kasim, W. A., Gaafar, R. M., Abou-Ali, R. M., Omar, M. N., and Hewait, H. M. (2016). Effect of biofilm forming plant growth promoting rhizobacteria on salinity tolerance in barley. Ann. Aric. Sci. 61, 217–227. doi:10.1016/j.aoas.2016.07.003
Kasotia, A., Varma, A., and Choudhary, D. K. (2015). Pseudomonas-mediated mitigation of salt stress and growth promotion in Glycine max. Agric. Res. 4, 31–41. doi:10.1007/s40003-014-0139-1
Kaur, B., Gupta, S. R., and Singh, G. (2002a). Carbon storage and nitrogen cycling in silvopastoral systems on a sodic soil in northwestern India. Agrofor. Syst. 54, 21–29. doi:10.1023/a:1014269221934
Kaur, B., Gupta, S. R., and Singh, G. (2002b). Bioamelioration of a sodic soil by silvopastoral systems in northwestern India. Agrofor. Syst. 54, 13–20. doi:10.1023/a:1014221306004
Kerepesi, I., and Galiba, G. (2000). Osmotic and salt stress-induced alteration in soluble carbohydrate content in wheat seedlings. Crop Sci. 40 (2), 482–487. doi:10.2135/cropsci2000.402482x
Kerry, R. G., Gouda, S., Das, G., Vishnuprasad, C. N., and Patra, J. K. (2017). “Agricultural nanotechnologies: Current applications and future prospects,” in Microbial biotechnology: Volume 1. Applications in agriculture and environment. Editors J. K. Patra, C. N. Vishnuprasad, and G. Das (Singapore: Springer), 3–28. doi:10.1007/978-981-10-6847-8_1
Kerry, R. G., Patra, S., Gouda, S., Patra, J. K., and Das, G. (2018). “Microbes and their role in drought tolerance of agricultural food crops,” in Microbial biotechnology: Volume 2. Application in food and pharmacology. Editors J. K. Patra, G. Das, and H. S. Shin (Singapore: Springer), 253–273. doi:10.1007/978-981-10-7140-9_12
Kerry, R. G., Rout, J. R., Das, G., Fraceto, L. F., Paramithiotis, S., and Patra, J. K. (2019). “Applications of nanotechnology in food and agriculture,” in Food molecular microbiology. Editors S. Paramithiotis, and J. K. Patra First Edition (London, SW1P 1WG, UK: Routledge Taylor & Francis, CRC Press), 262.
Khan, N., Zandi, P., Ali, S., Mehmood, A., Shahid, M. A., and Yang, J. (2018). Impact of salicylic acid and PGPR on the drought tolerance and phytoremediation potential of helianthus annus. Front. Microbiol. 9, 2507. doi:10.3389/fmicb.2018.02507
Khan, M. A., Asaf, S., Khan, A. L., Adhikari, A., Jan, R., Ali, S., et al. (2019). Halotolerant rhizobacterial strains mitigate the adverse effects of NaCl stress in soybean seedlings. Biomed. Res. Int. 2019, 9530963. Article ID 9530963. doi:10.1155/2019/9530963
Khan, I., Muhammad, A., Chattha, M. U., Skalicky, M., Bilal Chattha, M., Ahsin Ayub, M., et al. (2022). Mitigation of salinity-induced oxidative damage, growth, and yield reduction in fine rice by sugarcane press mud application. Front. Plant Sci. 13, 840900. doi:10.3389/fpls.2022.840900
Kim, H. S., Kim, K. R., Yang, J. E., Ok, Y. S., Owens, G., Nehls, T., et al. (2016). Effect of biochar on reclaimed tidal land soil properties and maize (Zea mays L.) response. Chemosphere 142, 153–159. doi:10.1016/j.chemosphere.2015.06.041
Kohler, J., Caravaca, F., and Roldan, A. (2010). An AM fungus and a PGPR intensify the adverse effects of salinity on the stability of rhizosphere soil aggregates of Lactuca sativa. Soil Biol. biochem. 42, 429–434. doi:10.1016/j.soilbio.2009.11.021
Koo, Y. M., Heo, A. Y., and Choi, H. W. (2020). Salicylic acid as a safe plant protector and growth regulator. Plant Pathol. J. 36 (1), 1–10. doi:10.5423/ppj.rw.12.2019.0295
Kumar, P., and Sharma, P. K. (2020). Soil salinity and food security in India. Front. Sustain. Food Syst. 4, 533781. doi:10.3389/fsufs.2020.533781
Kumar, P., and Sharma, P. K. (2020). Soil salinity and food security in India. Front. Sustain. Food Syst. 4, 533781. doi:10.3389/fsufs.2020.533781
Kumar, A., and Verma, J. P. (2018). Does plant—microbe interaction confer stress tolerance in plants: A review? Microbiol. Res. 207, 41–52. doi:10.1016/j.micres.2017.11.004
Kumar, P., Chaudhari, S. K., Mishra, A. K., Singh, K., Rai, P., Singh, R., et al. (2014). Labile carbon dynamics and soil amelioration in six-year old Eucalyptus tereticornis plantation in sodic soils. J. Soil Salin. Water Qual. 6, 91–95.
Kumar, P., Singh, K., Mishra, A. K., Kumar, A., Singh, R., Rai, P., et al. (2015). Effect of temporal changes and plantation age on gas exchange characteristics of Populus deltoides in the Indo-Gangetic plains of India. Indian J. Agrofor. 17, 22–26.
Kumar, P., Mishra, A. K., Kumar, M., Chaudhari, S. K., Singh, R., Singh, K., et al. (2019). Biomass production and carbon sequestration of Eucalyptus tereticornis plantation in reclaimed sodic soils of north-west India. Indian J. Agric. Sci. 89, 1091–1095.
Kumar, P., Mishra, A. K., Chaudhari, S. K., Sharma, D. K., Rai, A. K., Singh, K., et al. (2020). Carbon sequestration and soil carbon build-up under Eucalyptus plantation in semi-arid regions of north-west India. J. Sustain. 40, 319–331. doi:10.1080/10549811.2020.1749856
Kumar, A., Singh, S., Gaurav, A. K., Srivastava, S., and Verma, J. P. (2020a). Plant growth-promoting bacteria: Biological tools for the mitigation of salinity stress in plants. Front. Microbiol. 11, 1216. doi:10.3389/fmicb.2020.01216
Kvasnica, M., Buchtova, K., Budesinsky, M., Beres, T., Rarova, L., and Strnad, M. (2019). Synthesis, characterization and antiproliferative activity of seco analogues of brassinosteroids. Steroids 146, 1–13. doi:10.1016/j.steroids.2019.03.004
LadyofHats (2006). English: The image is a corrected version of an image I made sometime ago. The original quote on the image was "the image describes the parts on a typical plant cell. the image i made myself as resources i used the simple structure here, also the one i found hereand must of the text i could get from here.
LadyofHats (2013). English: A diagram showing a mitochondrion of the eukaryotic cell. Mitochondria are organelles surrounded by membranes, distributed in the cytosol of most eukaryotic cells. Its main function is the conversion of potential energy of pyruvate molecules into atp.
Lashari, M. S., Ye, Y., Ji, H., Li, L., Kibue, G. W., Lu, H., et al. (2015). Biochar–manure compost in conjunction with pyroligneous solution alleviated salt stress and improved leaf bioactivity of maize in a saline soil from central China: A 2-year field experiment. J. Sci. Food Agric. 95, 1321–1327. doi:10.1002/jsfa.6825
Li, H. Q., and Jiang, X. W. (2017). Inoculation with plant growth-promoting bacteria (PGPB) improves salt tolerance of maize seedling. Russ. J. Plant Physiol. 64, 235–241. doi:10.1134/s1021443717020078
Liang, B., Lehmann, J., Sohi, S., Thies, J. E., O’Neill, B., Trujillo, L., et al. (2010). Black carbon affects the cycling of non-black carbon in soil. Org. Geochem. 41, 206–213. doi:10.1016/j.orggeochem.2009.09.007
Lin, H. R., Shu, H. Y., and Lin, G. H. (2018). Biological roles of indole-3-acetic acid in Acinetobacter baumannii. Microbiol. Res. 216, 30–39. doi:10.1016/j.micres.2018.08.004
Liu, R., and Lal, R. (2012). Nanoenhanced materials for reclamation of mine lands and other degraded soils: A review. J. Nanotechnol. Article ID 461468.
Lu, H., Lashari, M. S., Liu, X., Ji, H., Li, L., Zheng, J., et al. (2015). Changes in soil microbial community structure and enzyme activity with amendment of biochar-manure compost and pyroligneous solution in a saline soil from Central China. Euro. J. Soil Biol. 70, 67–76. doi:10.1016/j.ejsobi.2015.07.005
Luan, S. (2009). The CBL-CIPK network in plant calcium signaling. Trends Plant Sci. 14, 37–42. doi:10.1016/j.tplants.2008.10.005
Luo, X., Liu, G., Xia, Y., Chen, L., Jiang, Z., Zheng, H., et al. (2017). Use of biochar-compost to improve properties and productivity of the degraded coastal soil in the Yellow River Delta, China. J. Soils Sedim. 17, 780–789. doi:10.1007/s11368-016-1361-1
Ma, M., Ayaz, P., Jin, W., Hussain, M., and Zhou, W. (2019). Analysis of the dissipation behavior of defoliants in cotton fiber during field and scouring process using liquid and gas chromatography. Int. J. Anal. Chem. 2019, 1–5. doi:10.1155/2019/2879074
Ma, Y., Dias, M. C., and Freitas, H. (2020). Drought and salinity stress responses and microbe-induced tolerance in plants. Front. Plant Sci. 11, 591911. doi:10.3389/fpls.2020.591911
Mahmoud, E., Ibrahim, M., Robin, P., Akkal-Corfini, N., and El-Saka, M. (2009). Rice straw composting and its effect on soil properties. Compost Sci. Util. 17, 146–150. doi:10.1080/1065657x.2009.10702415
Malik, J. A., AlQarawi, A. A., AlZain, M. N., Dar, B. A., Habib, M. M., and Ibrahim, S. N. S. (2022). Effect of salinity and temperature on the seed germination and seedling growth of desert forage grass lasiurus scindicus henr. Sustainability 14, 8387. doi:10.3390/su14148387
Manchanda, G., and Garg, N. (2008). Salinity and its effects on the functional biology of legumes. Acta Physiol. Plant. 30, 595–618. doi:10.1007/s11738-008-0173-3
Manske, M. (2009). Secretory pathway diagram, including nucleus, endoplasmic reticulum and golgi apparatus.
Mao, J., Manik, S. M. N., Shi, S., Chao, J., Jin, Y., Wang, Q., et al. (2016). Mechanisms and physiological roles of the CBL-CIPK networking system in Arabidopsis thaliana. Genes. 7, 62. doi:10.3390/genes7090062
Marino, D., Dunand, C., Puppo, A., and Pauly, N. (2012). A burst of plant NADPH oxidases. Trends Plant Sci. 17, 9–15. doi:10.1016/j.tplants.2011.10.001
Mashimbye, Z. E., Cho, M. A., Nell, J. P., De Clercq, W. P., Van Niekerk, A., and Turner, D. P. (2012). Model-based integrated methods for quantitative estimation of soil salinity from hyperspectral remote sensing data: A case study of selected South African soils. Pedosphere 22, 640–649. doi:10.1016/s1002-0160(12)60049-6
Mayak, S., Tirosh, T., and Glick, B. R. (2004). Plant growth-promoting bacteria confer resistance in tomato plants to salt stress. Plant Physiol. biochem. 42, 565–572. doi:10.1016/j.plaphy.2004.05.009
Meena, K. K., Sorty, A. M., Bitla, U. M., Choudhary, K., Gupta, P., Pareek, A., et al. (2017). Abiotic stress responses and microbe-mediated mitigation in plants: The omics strategies. Front. Plant Sci. 8, 172. doi:10.3389/fpls.2017.00172
Mirlas, V., Anker, Y., Aizenk, A., and Goldshleger, N. (2022). Irrigation quality and management determine salinization in Israeli olive orchards. Model. Dev. 15, 129–143. doi:10.5194/gmd-15-129-2022
Mishra, A. K., Das, R., Sahoo, S., and Biswal, B ( (2018a). “Global regulations and legislations on nanoparticles usage and application in diverse horizons,” in Environmental nanotechnology: Implications and impact, comprehensive analytical chemistry. Editors N. B. Turan, G. O. Engine, and M. S. Bilgili (Elsevier), 261–290.
Mishra, A. K., Mahinda, A. J., Shinjo, H., Jat, M. L., Singh, A., and Funakawa, S. (2018b). Role of conservation agriculture in mitigating soil salinity in indo-gangetic plains of India, Eng. Pract. Manag. Soil Salin. Apple Academic Press, 129–147.
Misra, S., and Chauhan, P. S. (2020). ACC deaminase-producing rhizosphere competent Bacillus spp. mitigate salt stress and promote Zea mays growth by modulating ethylene metabolism. 3 Biotech. 10, 119. doi:10.1007/s13205-020-2104-y
Mol-Instincts (2020). Humic acid high-quality images - C9H9NO6. Mol-Instincts. https://www.molinstincts.com/image/Humic-acid-img-CT1100402435.html (accessed October 14, 2020).
Møller, I. M., and Sweetlove, L. J. (2010). ROS signalling – specificity is required. Trends Plant Sci. 15, 370–374. doi:10.1016/j.tplants.2010.04.008
Mukherjee, P., Mitra, A., and Roy, M. (2019). Halomonas rhizobacteria of Avicennia marina of Indian sundarbans promote rice growth under saline and heavy metal stresses through Exopolysaccharide production. Front. Microbiol. 10, 1207. doi:10.3389/fmicb.2019.01207
Munns, R., and Tester, M. (2008). Mechanisms of salinity tolerance. Annu. Rev. Plant Biol. 59, 651–681. doi:10.1146/annurev.arplant.59.032607.092911
Munns, R. (2005). Genes and salt tolerance: Bringing them together. New Phytol. 167, 645–663. doi:10.1111/j.1469-8137.2005.01487.x
Nadeem, S. M., Zahir, Z. A., Naveed, M., and Arshad, M. (2007). Preliminary investigations on inducing salt tolerance in maize through inoculation with rhizobacteria containing ACC deaminase activity. Can. J. Microbiol. 53, 1141–1149. doi:10.1139/w07-081
Nadeem, S. M., Zahir, Z. A., Naveed, M., and Nawaz, S. (2013). Mitigation of salinity induced negative impact on the growth and yield of wheat by plant growth-promoting rhizobacteria in naturally saline conditions. Ann. Microbiol. 63, 225–232. doi:10.1007/s13213-012-0465-0
Naher, U. A., Panhwar, Q. A., Othman, R., Shamshuddin, J., Ismail, M. R., and Zhou, E. (2018). Proteomic study on growth promotion of PGPR inoculated aerobic rice (Oryza sativa L.) cultivar MR219-9. Pak. J. Bot. 50 (5), 1843–1852.
Narsing Rao, M. P., Dong, Z. Y., Xiao, M., and Li, W. J. (2019). “Effect of salt stress on plants and role of microbes in promoting plant growth under salt stress,” in Microorganisms in saline environments: Strategies and functions. Soil biology. Editors B. Giri, and A. Varma (Cham: Springer), 56. doi:10.1007/978-3-030-18975-4_18
Nguyen, K., Liou, Y., Tran, H., Hoang, P., and Nguyen, H. (2020). Soil salinity assessment by using near-infrared channel and vegetation soil salinity index derived from landsat 8 OLI data: A case study in the tra vinh province, mekong delta, vietnam. Prog. Earth Planet Sci. 7, 1. doi:10.1186/s40645-019-0311-0
O'Brien, J. A., and Benková, E. (2013). Cytokinin crosstalking during biotic and abiotic stress responses. Front. Plant Sci. 4, 451. doi:10.3389/fpls.2013.00451
Ogbodo, E. N. (2011). Effect of crop residue on soil chemical properties and rice yield on an ultisol at abakaliki, southeastern Nigeria. World J. Agric. Sci. 7, 13–18.
Pan, B., and Xing, B. (2012). Applications and implications of manufactured nanoparticles in soils: A review. Eur. J. Soil Sci. 63, 437–456. doi:10.1111/j.1365-2389.2012.01475.x
Pang, Q., Chen, S., Dai, S., Chen, Y., Wang, Y., and Yan, X. (2010). Comparative proteomics of salt tolerance inArabidopsis thaliana and Thellungiella halophila. J. Proteome Res. 9, 2584–2599. doi:10.1021/pr100034f
Panhwar, Q. A., Shamshuddin, J., Naher, U. A., Radziah, O., and Mohd Razi, I. (2014). Bio-fertilizer, ground magnesium limestone and basalt applications may improve chemical properties of Malaysian acid sulfate soils and rice growth. Pedosphere 24, 827–835. doi:10.1016/s1002-0160(14)60070-9
Panhwar, Q. A., Ali, A., Naher, U. A., Depar, N., and Memon, M. Y. (2019). “Ameliorating plant salt stress through bacterial inoculation: Prospects and challenges,” in Salt stress, microbes, and plant interactions: Mechanisms and molecular approaches. Editor M. S. Akhtar (Springer Singapore), 253–268.
Parihar, P., Singh, S., Singh, R., Singh, V. P., and Prasad, S. M. (2015). Effect of salinity stress on plants and its tolerance strategies: A review. Environ. Sci. Pollut. Res. 22, 4056–4075. doi:10.1007/s11356-014-3739-1
Peng, J., Biswas, A., Jiang, Q., Zhao, R., Hu, J., Hu, B., et al. (2018). Estimating soil salinity from remote sensing and terrain data in southern Xinjiang Province, China. Geoderma 337, 1309–1319. doi:10.1016/j.geoderma.2018.08.006
Perkins-Kirkpatrick, S. E., Fischer, E. M., Angélil, O., and Gibson, P. B. (2017). The influence of internal climate variability on heatwave frequency trends. Environ. Res. Lett. 12, 044005. doi:10.1088/1748-9326/aa63fe
Poonam, R. K., Bhardwaj, R., and Sirhindi, G. (2015). Castasterone regulated polyphenolic metabolism and photosynthetic system in Brassica juncea plants under copper stress. J. Pharmacogn. Phytochem. 4, 282–289.
Porcel, P., Aroca, R., and Ruiz-Lozano, J. M. (2012). Salinity stress alleviation using arbuscular mycorrhizal fungi. A review. A Rev. Agron. Sustain. Dev. 32, 181–200. doi:10.1007/s13593-011-0029-x
Qamar, M., and Muneer, M. (2005). Comparative photocatalytic study of two selected pesticide derivatives, indole-3-acetic acid and indole-3- butyric acid in aqueous suspensions of titanium dioxide. J. Hazard. Mat. 120, 219–227. doi:10.1016/j.jhazmat.2005.01.005
Qef (2009). English: Basic structure of a peroxisome, showing the crystalized enzyme core as found in rat liver cells.
Qin, Y., Druzhinina, I. S., Pan, X., and Yuan, Z. (2016). Microbially mediated plant salt tolerance and microbiome-based solutions for saline agriculture. Biotechnol. Adv. 34, 1245–1259. doi:10.1016/j.biotechadv.2016.08.005
Quan, R., Lin, H., Mendoza, I., Zhang, Y., Cao, W., Yang, Y., et al. (2007). SCABP8/CBL10, a putative calcium sensor, interacts with the protein kinase SOS2 to protect Arabidopsis shoots from salt stress. Plant Cell. 19, 1415–1431. doi:10.1105/tpc.106.042291
Rajabi Dehnavi, A., Zahedi, M., Razmjoo, J., and Eshghizadeh, H. (2019). Effect of exogenous application of salicylic acid on salt-stressed sorghum growth and nutrient contents. J. Plant Nutr. 42, 1333–1349. doi:10.1080/01904167.2019.1617307
Ravindra, K., Singh, T., and Mor, S. (2019). Emissions of air pollutants from primary crop residue burning in India and their mitigation strategies for cleaner emissions. J. Clean. Prod. 208, 261–273. doi:10.1016/j.jclepro.2018.10.031
Rifqi, S. (2017). Bahasa Indonesia: Diagram kloroplas tanpa bingkai, diterjemahkan Dari versi bahasa Inggris (File:Chloroplast (borderless version)-en.svg).
Rima, F. S., Biswas, S., Kumer, P., Islam, S. Md. R., and Seraj, Z. I. (2018). Bacteria endemic to saline coastal belt and their ability to mitigate the effects of salt stress on rice growth and yields. Ann. Microbiol. 68, 525–535. doi:10.1007/s13213-018-1358-7
Rizwan, M., Meunier, J. D., Davidian, J. C., Pokrovsky, O. S., Bovet, N., and Keller, C. (2016b). Silicon alleviates Cd stress of wheat seedlings (Triticum turgidum L. cv. Claudio) grown in hydroponics. Environ. Sci. Pollut. Res. 23, 1414–1427. doi:10.1007/s11356-015-5351-4
Roy, H., Islam, M. S., Arifin, M. T., and Firoz, S. H. (2022). Synthesis, characterization and sorption properties of biochar, chitosan and ZnO-based binary composites towards a cationic dye. Sustainability 14, 14571. doi:10.3390/su142114571
Ruppel, S., Franken, P., and Witzel, K. (2013). Properties of the halophyte microbiome and their implications for plant salt tolerance. Funct. Plant Biol. 40, 940–951. doi:10.1071/fp12355
Rütting, T., Aronsson, H., and Delin, S. (2018). Efficient use of nitrogen in agriculture. Nutr. Cycl. Agroecosys. 110, 1–5. doi:10.1007/s10705-017-9900-8
Sahu, N., Singh, S. K., Reddy, G. P. O., Kumar, N., Nagaraju, M. S. S., and Srivastava, R. (2016). Large-scale soil resource mapping using IRS-P6 LISS-IV and cartosat-1 DEM in basaltic terrain of central India. J. Indian Soc. Remote Sens. 44, 811–819. doi:10.1007/s12524-015-0540-7
Sairam, R. K., and Tyagi, A. (2004). Physiology and molecular biology of salinity stress tolerance in plants. Curr. Sci. 86, 407–421.
Saleem, S., Iqbal, A., Ahmed, F., and Ahmad, M. (2021). Phytobeneficial and salt stress mitigating efficacy of IAA producing salt tolerant strains in Gossypium hirsutum. Saudi J. Biol. Sci. 28 (9), 5317–5324. doi:10.1016/j.sjbs.2021.05.056
Saravanakumar, D., and Samiyappan, R. (2007). ACC deaminase from Pseudomonas fluorescens mediated saline resistance in groundnut (Arachis hypogea) plants. J. Appl. Microbiol. 102, 1283–1292. doi:10.1111/j.1365-2672.2006.03179.x
Schirawski, J., and Perlin, M. H. (2018). Plant− microbe interaction 2017-the good, the bad and the diverse. Int. J. Mol. Sci. 19, 1374. doi:10.3390/ijms19051374
Setia, R., Gottschalk, P., Smith, P., Marschner, P., Baldock, J., Setia, D., et al. (2013). Soil salinity decreases global soil organic carbon stocks. Sci. Total. Environ. 465, 267–272. doi:10.1016/j.scitotenv.2012.08.028
Shalaby, T. A., Bayoumi, Y., Abdalla, N., Taha, H., Alshaal, T., Shehata, S., et al. (2016). Nanoparticles, soils, plants and sustainable agriculture. Springer International Publishing Switzerland.
Shi, Q., Bao, Z., Zhu, Z., Ying, Q., and Qian, Q. (2006). Effects of different treatments of salicylic acid on heat tolerance, chlorophyll fluorescence, and antioxidant enzyme activity in seedlings of Cucumis sativa L. Plant Growth Reg. 48, 127–135. doi:10.1007/s10725-005-5482-6
Shrivastava, P., and Kumar, R. (2015). Soil salinity: A serious environmental issue and plant growth promoting bacteria as one of the tools for its alleviation. Saudi J. Biol. Sci. 22, 123–131. doi:10.1016/j.sjbs.2014.12.001
Sidike, A., Zhao, S., and Wen, Y. (2014). Estimating soil salinity in Pingluo County of China using QuickBird data and soil reflectance spectra. Int. J. Appl. Earth Obs. Geoinf. 26, 156–175. doi:10.1016/j.jag.2013.06.002
Simontacchi, M., Galatro, A., Ramos-Artuso, F., and Santa-Maria, G. E. (2015). Plant survival in a changing environment: The role of nitric oxide in plant responses to abiotic stress. Front. Plant Sci. 6, 977. doi:10.3389/fpls.2015.00977
Singh, Y., Singh, M., Sidhu, H. S., Khanna, P. K., Kapoor, S., Jain, A. K., et al. (2010). Options for effective utilization of crop residues. Ludhiana, India: Directorate of Research, Punjab Agricultural University, 32p.
Singh, J. S., Pandey, V. C., and Singh, D. P. (2011). Efficient soil microorganisms: A new dimension for sustainable agriculture and environmental development. Agric. Ecosyst. Environ. 140, 339–353. doi:10.1016/j.agee.2011.01.017
Singh, Y. P., Singh, G., and Sharma, D. K. (2011). Ameliorative effect of multipurpose tree species grown on sodic soils of Indo-Gangetic alluvial plains of India. Arid. Land Res. Manag. 25, 55–74. doi:10.1080/15324982.2010.528150
Singh, J. S., Kaushal, S., Kumar, A., Vimal, S. R., and Gupta, V. K. (2016). Book review: Microbial inoculants in sustainable agricultural productivity- vol. II: Functional application. Front. Microbiol. 7, 923. doi:10.3389/fmicb.2016.00923
Singh, G. (2018). Climate change and sustainable management of salinity in agriculture. Res. Med. Eng. Sci. 6, 1–7. doi:10.31031/rmes.2018.06.000635
Smartse, J. (2010). Vectorised by. English: A diagram showing the different routes through which water can travel through a plant.
Soni, R., Yadav, S. K., and Rajput, A. S. (2018). “ACC-deaminase producing rhizobacteria: Prospects and application as stress busters for stressed agriculture,” in Microorganisms for green revolution. Microorganisms for sustainability. Editors D. Panpatte, Y. Jhala, H. Shelat, and R. Vyas (Singapore: Springer), 7. doi:10.1007/978-981-10-7146-1_9
Srivastava, R., and Saxena, A. K. (2004). Technique of large-scale soil mapping in basaltic terrain using satellite remote sensing data. Int. J. Remote Sens. 25, 679–688. doi:10.1080/0143116031000068448
Stock, A. (2018). Photo — methyl jasmonate plant stress signal molecule. 3D rendering. Atoms are represented as spheres with conventional color coding: Hydrogen (white), carbon. Alamy. Available at: https://www.alamy.com/stock-photo-methyl-jasmonate-plant-stress-signal-molecule-3d-rendering-atoms-are-177320312.html (accessed October 14, 2020).
Subedi, R., Taupe, N., Ikoyi, I., Bertora, C., Zavattaro, L., Schmalenberger, A., et al. (2016). Chemically and biologically-mediated fertilizing value of manure-derived biochar. Sci. Total. Env. 550, 924–933. doi:10.1016/j.scitotenv.2016.01.160
Sultana, S., Paul, S. C., Parveen, S., Alam, S., Rahman, N., Jannat, B., et al. (2020). Isolation and identification of salt-tolerant plant-growthpromoting rhizobacteria and their application for rice cultivation under salt stress. Can. J. Microbiol. 66, 144–160. doi:10.1139/cjm-2019-0323
Sun, J., He, F., Shao, H., Zhang, Z., and Xu, G. (2016). Effects of biochar application on Suaedasalsa growth and saline soil properties. Environ. Earth Sci. 75, 630–636. doi:10.1007/s12665-016-5440-9
Sun, Y., Yang, J., Yao, R., Chen, X., and Wang, X. (2020). Biochar and fulvic acid amendments mitigate negative effects of coastal saline soil and improve crop yields in a three year field trial. Sci. Rep. Artic. No 10, 8946. doi:10.1038/s41598-020-65730-6
Taghadosi, M. M., Hasanlou, M., and Eftekhari, K. (2019). Soil salinity mapping using dual-polarized SAR Sentinel-1 imagery. Int. J. Remote Sens. 40, 237–252. doi:10.1080/01431161.2018.1512767
Tang, R., Wang, C., Li, K., and Luan, S. (2020). The CBL–CIPK calcium signaling network: Unified paradigm from 20 Years of discoveries. Trends Plant Sci. 25, 604–617. doi:10.1016/j.tplants.2020.01.009
Tank, N., and Saraf, M. (2010). Salinity-resistant plant growth promoting rhizobacteria ameliorates sodium chloride stress on tomato plants. J. Plant Interact. 5, 51–58. doi:10.1080/17429140903125848
Tantawy, A. S., Salama, Y. A. M., El-Nemr, M. A., and Abdel-Mawgoud, A. M. R. (2015). Nano silicon application improves salinity tolerance of sweet pepper plants. Int. J. Chemtech Res. 8, 11–17.
Tao, J. J., Chen, H. W., Ma, B., Zhang, W. K., Chen, S. Y., and Zhang, J. S. (2015). The role of ethylene in plants under salinity stress. Front. Plant Sci. 6, 1059. doi:10.3389/fpls.2015.01059
Tewari, S., and Arora, N. K. (2014). Talc based exopolysaccharides formulation enhancing growth and production of Hellianthus annuus under saline conditions. Cell. Mol. Biol. 60, 73–81.
Tewari, S., and Arora, N. K. (2018). Role of salicylic acid from Pseudomonas aeruginosa PF23EPS+ in growth promotion of sunflower in saline soils infested with phytopathogen Macrophomina phaseolina. Env. Sust. 1, 49–59. doi:10.1007/s42398-018-0002-6
Thomas, S. C., Frye, S., Gale, N., Garmon, M., Launchbury, R., Machado, N., et al. (2013). Biochar mitigates negative effects of salt additions on two herbaceous plant species. J. Environ. Manag. 129, 62–68. doi:10.1016/j.jenvman.2013.05.057
Thomaz, E. L., and Fachin, P. A. (2014). Effects of heating on soil physical properties by using realistic peak temperature gradients. Geoderma 230-231, 243–249. doi:10.1016/j.geoderma.2014.04.025
Tilman, D., Balzer, C., Hill, J., and Befort, B. L. (2011). Global food demand and the sustainable intensification of agriculture. Proc. Natl. Acad. Sci. U. S. A. 108, 20260–20264. doi:10.1073/pnas.1116437108
Timmusk, S., El-Daim, I. A. A., Copolovici, L., Tanilas, T., Kännaste, A., Behers, L., et al. (2014). Drought-tolerance of wheat improved by rhizosphere bacteria from harsh environments: Enhanced biomass production and reduced emissions of stress volatiles. PloS One 9, e96086. doi:10.1371/journal.pone.0096086
Tiwari, S., Lata, C., Chauhan, P. S., and Nautiyal, C. S. (2016). Pseudomonas putida attunes morphophysiological, biochemical and molecular responses in Cicer arietinum L. during drought stress and recovery. Plant Physiol. Biochem. 99, 108–117. doi:10.1016/j.plaphy.2015.11.001
Toklikishvili, N., Dandurishvili, N., Vainstein, A., Tediashvili, M., Giorgobiani, N., Lurie, S., et al. (2010). Inhibitory effect of ACC deaminase-producing bacteria on crown gall formation in tomato plants infected by Agrobacterium tumefaciens or A. vitis. Plant Pathol. 59, 1023–1030. doi:10.1111/j.1365-3059.2010.02326.x
Tuna, A. L., Kaya, C., Dikilitas, M., and Higgs, D. (2008). The combined effects of gibberellic acid and salinity on some antioxidant enzyme activities, plant growth parameters and nutritional status in maize plants. Environ. Exp. Bot. 62, 1–9. doi:10.1016/j.envexpbot.2007.06.007
TurboSquid (2020). 3d model abscisic acid 2020. Available at: https://www.turbosquid.com/3d-models/3d-model-abscisic-acid/871889 (accessed October 14, 2020).
Tuteja, N. (2007). Abscisic acid and abiotic stress signaling. Plant Signal. Behav. 2, 135–138. doi:10.4161/psb.2.3.4156
Upadhyay, S. K., Singh, J. S., and Singh, D. P. (2011). Exopolysaccharide-producing plant growth-promoting rhizobacteria under salinity condition. Pedosphere 21, 214–222. doi:10.1016/s1002-0160(11)60120-3
Van Aken, O., and Van Breusegem, F. (2015). Licensed to kill: Mitochondria, chloroplasts, and cell death. Trends Plant Sci. 20, 754–766. doi:10.1016/j.tplants.2015.08.002
Venu, V. K. P., Mahmoud, S., Hollenberg, M. D., and Hirota, S. A. (2018). Gut derived indole 3-propionic acid regulates endothelial function in A pxr dependent mechanism. Atheroscler. Suppl. 32, 125. doi:10.1016/j.atherosclerosissup.2018.04.385
Vimal, S. R., Singh, J. S., Arora, N. K., and Singh, S. (2017). Soil-plant-microbe interactions in stressed agriculture management: A review. Pedosphere 27, 177–192. doi:10.1016/s1002-0160(17)60309-6
Vreugdenhil, D., Bradshaw, J., Gebhardt, C., Govers, F., Taylor, M. A., MacKerron, D. K., et al. (2011). Potato biology and biotechnology: Advances and perspectives. Elsevier Science.
Waheed, A., Hamid, F. S., Ahmad, H., Abbassi, F. M., Aslam, S., Shah, A. H., et al. (2014). Effect of Indole Butyric acid (IBA) on early root formation (tomato “Sahil” hybrid) cuttings. J. Mat. Env. Sci. 6, 272–279.
Wang, Y., Wu, J., Kim, S. G., Tsuda, K., Gupta, R., Park, S. Y., et al. (2016). Magnaporthe oryzae-secreted protein MSP1 induces cell death and elicits defense responses in rice. Mol. Plant-Microbe Interact. 29, 299–312. doi:10.1094/mpmi-12-15-0266-r
Warnock, D. D., Lehmann, J., Kuyper, T. W., and Rillig, M. C. (2007). Mycorrhizal responses to biochar in soil — concepts and mechanisms. Plant Soil 300, 9–20. doi:10.1007/s11104-007-9391-5
Wu, F., Chi, Y., Jiang, Z., Xu, Y., Xie, L., Huang, F., et al. (2020). Hydrogen peroxide sensor HPCA1 is an LRR receptor kinase in Arabidopsis. Nature 578, 577–581. doi:10.1038/s41586-020-2032-3
Xu, G., Zhang, Y., Sun, J., and Shao, H. (2016). Negative interactive effects between biochar and phosphorus fertilization on phosphorus availability and plant yield in saline sodic soil. Sci. Total. Environ. 568, 910–915. doi:10.1016/j.scitotenv.2016.06.079
Xu, Z., Shao, T., Lv, Z., Yue, Y., Liu, A., Long, X., et al. (2020). The mechanisms of improving coastal saline soils by planting rice. Sci. Total. Environ. 703, 135529. doi:10.1016/j.scitotenv.2019.135529
Yadav, R. C., Patra, A. K., Purakayastha, T. J., Singh, R., and Kumar, C. (2014). Effect of engineered nanoparticles of Fe and Zn oxides on enzyme activity and bacterial abundance in soil at ambient and elevated atmospheric CO2. Proc. Natl. Acad. Sci. India Sect. B Biol. Sci. 84, 649–656. doi:10.1007/s40011-014-0316-9
Yadav, A. N. (2020). “Plant microbiomes for sustainable agriculture: Current research and future challenges,” in Plant microbiomes for sustainable agriculture. Editor A. N. Yadav (Springer International Publishing), 475–482.
Yan, Y. H., Li, J. L., Zhang, X. Q., Yang, W. Y., Wan, Y., Ma, Y. M., et al. (2014). Effect of naphthalene acetic acid on adventitious root development and associated physiological changes in stem cutting of Hemarthria compressa. PloS One 9 (3), e90700. doi:10.1371/journal.pone.0090700
Yang, J., Kloepper, J. W., and Ryu, C. M. (2009). Rhizosphere bacteria help plants tolerate abiotic stress. Trends Plant Sci. 14, 1–4. doi:10.1016/j.tplants.2008.10.004
Yanni, Y., Zidan, M., Dazzo, F., Rizk, R., Mehesen, A., Abdelfattah, F., et al. (2016). Enhanced symbiotic performance and productivity of drought stressed common bean after inoculation with tolerant native rhizobia in extensive fields. Agric. Ecosyst. Environ. 232, 119–128. doi:10.1016/j.agee.2016.07.006
Yasin, N. A., Khan, W. U., Ahmad, S. R., Ali, A., Ahmad, A., and Akram, W. (2018). Imperative roles of halotolerant plant growth-promoting rhizobacteria and kinetin in improving salt tolerance and growth of black gram (Phaseolus mungo). Environ. Sci. Pollut. Res. 25, 4491–4505. doi:10.1007/s11356-017-0761-0
Yasmin, H., Naeem, S., Bakhtawar, M., Jabeen, Z., Nosheen, A., Naz, R., et al. (2020). Halotolerant rhizobacteria Pseudomonas pseudoalcaligenes and Bacillus subtilis mediate systemic tolerance in hydroponically grown soybean (Glycine max L.) against salinity stress. PloS ONE 15 (4), e0231348. doi:10.1371/journal.pone.0231348
ACS. Zeatin (2014). American chemical society (ACS). Chem. Life. Am. Chem. Soc. Available at: https://www.acs.org/content/acs/en/molecule-of-the-week/archive/z/zeatin.html (accessed October 14, 2020).
Zhang, Y., Zhu, H., Zhang, Q., Li, M., Yan, M., Wang, R., et al. (2009). Phospholipase Dα1 and phosphatidic acid regulate NADPH oxidase activity and production of reactive oxygen species in ABA-mediated stomatal closure in Arabidopsis. Plant Cell. 21, 2357–2377. doi:10.1105/tpc.108.062992
Zhang, T., Shao, Y., Gong, H., Li, L., and Wang, L. (2014). Salt content distribution and paleoclimatic significance of the lop nur “Ear” feature: Results from analysis of EO-1 hyperion imagery. Remote Sens. 6, 7783–7799. doi:10.3390/rs6087783
Zhao, Y., Yu, H., Zhou, J. M., Smith, S. M., and Li, J. (2020). Malate circulation: Linking chloroplast metabolism to mitochondrial ROS. Trends Plant. Sci. 25, 446–454. doi:10.1016/j.tplants.2020.01.010
Zhu, Y. X., Gong, H. J., and Yin, J. L. (2019). Role of silicon in mediating salt tolerance in plants: A review. Plants 8, 147. doi:10.3390/plants8060147
Zhu, J. K. (2016). Abiotic stress signaling and responses in plants. Cell. 167, 313–324. doi:10.1016/j.cell.2016.08.029
Keywords: PGPM, nanotechnology, agroforestry, ROS, ethylene, biochar, residue retention
Citation: Mishra AK, Das R, George Kerry R, Biswal B, Sinha T, Sharma S, Arora P and Kumar M (2023) Promising management strategies to improve crop sustainability and to amend soil salinity. Front. Environ. Sci. 10:962581. doi: 10.3389/fenvs.2022.962581
Received: 06 June 2022; Accepted: 30 December 2022;
Published: 13 February 2023.
Edited by:
Ilan Stavi, Dead Sea and Arava Science Center, IsraelReviewed by:
Fasih Ullah Haider, South China Botanical Garden (CAS), ChinaCopyright © 2023 Mishra, Das, George Kerry, Biswal, Sinha, Sharma, Arora and Kumar. This is an open-access article distributed under the terms of the Creative Commons Attribution License (CC BY). The use, distribution or reproduction in other forums is permitted, provided the original author(s) and the copyright owner(s) are credited and that the original publication in this journal is cited, in accordance with accepted academic practice. No use, distribution or reproduction is permitted which does not comply with these terms.
*Correspondence: Ajay Kumar Mishra, YS5rLm1pc2hyYUBpcnJpLm9yZw==; Rajeswari Das, ZGFzLnJhamVzd2FyaTIwMDhAZ21haWwuY29t
Disclaimer: All claims expressed in this article are solely those of the authors and do not necessarily represent those of their affiliated organizations, or those of the publisher, the editors and the reviewers. Any product that may be evaluated in this article or claim that may be made by its manufacturer is not guaranteed or endorsed by the publisher.
Research integrity at Frontiers
Learn more about the work of our research integrity team to safeguard the quality of each article we publish.