- 1Department of Environmental Science/Interdisciplinary Centre for Climate Change, Aarhus University, Roskilde, Denmark
- 2Finnish Meteorological Institute, Helsinki, Finland
- 3Centre for Atmospheric and Climate Physics Research, Centre for Climate Change Research, University of Hertfordshire, Hatfield, United Kingdom
- 4Norwegian Institute of Public Health, Oslo, Norway
- 5Swiss National Science Foundation, Bern, Switzerland
- 6Center for International Climate Research (CICERO), Oslo, Norway
- 7Center for Earth System Research and Sustainability, University of Hamburg, Hamburg, Germany
- 8Ludwig-Maximilians-Universität München, München, Germany
- 9National Meteorological Administration, Bucharest, Romania
Feedbacks between air pollutants and meteorology play a crucial role in the direction of the response of future climate and air pollution. These feedbacks are important to understand and quantify the potential impact of adaptation and mitigation policies setup for protecting the population against air pollution and heat stress. We review the interactions between climate and air pollution, with special focus on the projections of air pollution under different future climate scenarios and time horizons, based on a literature review of research articles and reports from the last decade. The assessment focuses on 1) the specific impacts of climate change on air pollution and natural particle and precursor emissions in Europe in the near future (2030), by mid-century (2050) and by end of the century (2100), 2) impacts on air pollution due to changes in emissions vs. changes in climate, 3) feedbacks from air pollution on climate, 4) impacts of climate change on wildland fires and air pollutant levels, and 5) the role of adaptation and mitigation policies on climate change and air pollution. Available literature to a large extent suggests that ozone concentrations will likely increase in the second half of the century by up to 9 ppb [−4 + 9.3], while in the first half of the century, changes are much smaller and are up to ±1.5 ppb. These changes are mainly attributed to increased temperatures and emissions of biogenic volatile organic compounds, but also depends on the models and scenarios used in these studies. On the other hand, the predicted changes in particle concentrations and chemical composition are uncertain and much smaller. Similar to ozone, larger changes in the particle concentrations are projected in the second half of the century. The main conclusion from this review is that the estimated changes in pollutant levels in the future vary significantly depending on the applied model systems, as well as the different emission or meteorological scenarios used in the different studies. Nevertheless, studies generally agree on the overall trend of the changes in pollutant levels due to climate change, in particular in the second half of the century.
1 Introduction
Climate change can affect air pollution both physically, by modifying transport and mixing of pollutants as well as emissions of natural origin, and chemically by affecting chemical reactions. Such changes can lead to a “climate change penalty” which can be defined as the deterioration of air quality due to a warming climate, in the absence of changes in anthropogenic polluting activities (Fu and Tian, 2019). Changes in climate also influence the frequency and intensity of natural wildland fires (Lozano et al., 2017). Vice versa, pollutants in the atmosphere and their precursor emissions can influence climate by modifying clouds properties, precipitation, and radiation (Myhre et al., 2013). Feedbacks between climate change and air pollution should thus be taken into account in developing mitigation and adaptation strategies. However, the existing knowledge gaps on the involved mechanisms result in large uncertainties in the understanding and quantification of these feedbacks. For instance, although the main cause of wildfires currently is anthropogenic, i.e., caused by agriculture and forest management practices (Sofiev et al., 2013), projections suggest that the risk of wildland fires will increase due to higher temperatures and droughts (IPCC, 2022; McCarty et al., 2021; Dupuy et al., 2020). Recent findings from the latest Coupled Model Intercomparison Project Phase 6 (CMIP6: e.g., Turnock et al., 2020), the sixth assessment report from the IPCC (AR6), as well las the latest assessment on the short lived climate forcers (SLCFs) from the Arctic Monitoring and Assessment Programme (AMAP 2021) shows that future anthropogenic pollutant source strengths and levels decrease under all scenarios over Europe, but also in some other regions globally. This suggests that natural sources such as mineral dust, sea-salt, and wildland fires can be important drivers of air pollution and climate over Europe, where the feedback mechanisms and climate adaptation/mitigation scenarios will be particularly important.
Due to the above reasons, the aim of this study is to review the latest literature on the interactions between climate change and air pollution and assess the effects of climate change on surface ozone (O3) and fine particulate matter (PM2.5) levels over Europe, and how they will change in the future. Section 1 introduces the major mechanisms and pathways of meteorology-air pollution interactions and summarizes the current knowledge and findings regarding the different physical and chemical processes in relation to climate change and air pollution; Section 2 summarizes the recent findings on the specific impacts of climate change on ozone and particulate matter concentrations, respectively; Section 3 compares the relative impact of future emissions on air quality versus the impacts on air quality caused by climate change alone; Section 4 summarizes recent findings on the impacts of atmospheric composition on climate change; Section 5 summarizes the impacts of climate change on wildland fires and consequently on air pollution and climate change; Section 6 summarizes the co-benefits of climate adaptation and mitigation on air pollution; and finally, Section 7 offers some recommendations regarding needs for future research. Therefore, the current review paper attempts to give a more holistic picture of future air pollution and climate change, as well as feedbacks, impacts of emerging sources such as wildland fires, and climate adaptation and mitigation, compared to the existing reviews.
1.1 Mechanisms by which climate affects air pollution
Meteorology is a major driver of the fate of all chemically active species within the atmosphere, including climate-relevant compounds like carbon dioxide (CO2), methane (CH4), ozone (O3) and secondary inorganic (e.g., sulfate and nitrate) and organic aerosols. Changes in the climate and thereby in meteorological factors can affect atmospheric composition in several ways: 1) Changes in temperatures, precipitation and wind patterns may affect the natural sources of aerosols in the atmosphere, including mineral dust, sea salt, and biogenic volatile organic compounds (BVOCs). 2) This is also the case for anthropogenic sources, where e.g., increasing temperatures will increase NH3 emissions from agricultural activities and VOC emissions from loading of petroleum products. 3) Changes in temperature, humidity, solar radiation, and other meteorological factors can affect the rate of photochemical, oxidative, and other chemical reactions, speeding up or slowing down the chemical transformation of emitted species. 4) Changes in wind patterns, ambient temperatures and precipitation affect transport and mixing of atmospheric components, as well as deposition processes. 5) Changes in large-scale weather patterns, which control the dispersion and transport of air pollutants, can result in changes of the frequency and intensity of air pollution events. In summary, to understand how climate change may affect air pollution, one needs to consider how climate affects the full range of biological, chemical, and physical processes. Figure 1 summarizes how climate affects air quality and the two-way interactions between emissions and climate.
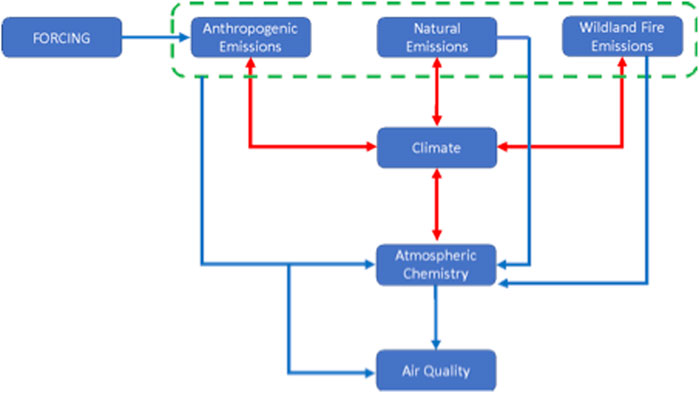
Figure 1. Interactions between climate, emissions and air quality (adopted from Jacob and Winner, 2009). Forcing term refers to the changes in the socioeconomic factors. The green dashed box shows the different emissions, while the red and the blue arrows show two-way and one-way interactions, respectively.
Certain types of synoptic and meteorological drivers favor higher concentrations of ozone (Otero et al., 2016). In particular, atmospheric blocking favors the occurrence of heatwaves and high ozone concentrations in the summer (Hodnebrog et al., 2012; Pfahl and Wernli, 2012). Pfahl and Wernli (2012) found that up to 80% of summer warm extremes are associated with blocking. Although climate models have notoriously had problems representing atmospheric blocking, large model ensembles can reproduce observed correlations between heat waves and these events, and results suggest that the frequency of blocking events in Europe will not change dramatically in the future, and neither will the relationship between blocking events and heatwaves (Schaller et al., 2018). However, with global warming, heatwaves will get more intense, more frequent, and last longer (Russo et al., 2014), increasing the likelihood for periods with higher ozone concentrations and high temperatures, especially during blocking events.
1.2 Climate change impacts on natural emissions
Wind speed and sea-surface temperatures also modify the production of sea-salt aerosols (SSA) emissions (Soares et al., 2016). The two main mechanisms responsible for sea spray formation are air bubble-bursting during whitecap formation, and decay and direct tearing of droplets from the top of breaking waves. Therefore, the formation of primary SSA is mainly dependent on wind speed (Monahan et al., 1986). In addition, laboratory (Mårtensson et al., 2003) and in situ measurements (Nilsson et al., 2007) showed that for nano-sized particles, the aerosol number emission in nano sizes decreases with increasing seawater temperature, while number emissions of particles larger than 100 nm increase with increasing seawater temperature.
Wind speed and precipitation also impacts the mineral dust emissions and their transport. Changes in wind can modify both the emissions of mineral dust particles, as well as their transport pathways. The dust emissions depend on the availability of fine crustal material that can be lifted from the ground when surface wind velocity exceeds a certain threshold. This threshold depends on surface roughness (e.g., rocks and vegetation), grain size and soil moisture. Mineral dust is an important natural source of atmospheric aerosols, and interferes with the atmosphere’s radiation, impacting climate (Klingmuller et al., 2019). However, there are large variations among projections of the future global mineral dust burden (Boucher et al., 2013). While some studies show decreases by up to 60% globally, mainly due to the effect of CO2 fertilization on vegetation (Mahowald et al., 2006), others project moderate (10%–20%: Tegen et al., 2004; Jacobson and Streets, 2009; Liao et al., 2009) to large increases (tripling) due to large increases in bare soil fraction (Woodward et al., 2005). The large range reflects different responses of the atmosphere and vegetation cover to climate change, and results in low confidence in these model predictions. The frequency of dust events might also change with climate. By analyzing historical high-resolution glacio-chemical data from the Swiss-Italian Alps in combination with meteorological parameters, Clifford et al. (2019), e.g., predict that a future warming will result in less frequent, but more intense events, where dust is transported from Sahara to Europe.
Increasing temperatures can lead to increased emissions of biogenic VOCs such as isoprene and terpenes, which are emitted by the vegetation, and are important precursors for O3 and secondary organic aerosols (SOA) (Guenther et al., 2006). The future development in the emission of BVOCs is also depending on the atmospheric CO2 level, where a tendency of a CO2 inhibition is seen for isoprene emissions (Arneth et al., 2007), while the impact on monoterpenes is more unclear (Arneth et al., 2007). Although isoprene is the largest biogenic contributor to SOA formation globally, over Europe aerosol formation is enhanced in the presence of mainly monoterpenes (D’Andrea et al., 2015). In contrast, the importance of isoprene for O3 formation is higher than that of other terpenoids. In addition to the type of the biogenic species and the availability of nitrogen oxides as reaction partners, recent investigations show that positive feedbacks exist between biogenic and anthropogenic VOCs for aerosol formation as well as ozone formation (Grote, 2019). Finally, recent studies highlighted the importance for extreme weather conditions such as droughts on the emissions of biogenic precursors such as isoprene (e.g., Pegoraro et al., 2004; Jiang et al., 2018), which is important due to the projected increase in the intensity of these drought periods (IPCC, 2013; Hoegh-Guldberg et al., 2018). Jiang et al. (2018) estimated a 17% reduction in the global isoprene emissions when the drought stress on the plants is taken into account, translating to a decrease of surface O3 mixing ratios by 3 ppb over Europe.
1.3 Climate change impacts on anthropogenic emissions
Meteorological parameters like surface wetness, wind and especially temperature have also been shown to have an important impact on emissions related to human activities. The ammonia emissions (NH3) from agricultural sources have been projected to increase significantly in Europe in a future climate (Skjøth and Geels, 2013) and at the global scale an empirical estimate proposes that a 5°C temperature increase would increase NH3 emission by 42% [28%–67%] (Sutton et al., 2013; Fowler et al., 2015). This suggested climate penalty on NH3 can through the conversion to ammonium (NH4+) also affect the PM2.5 levels in Europe. In addition, changes in temperatures also impact the use of ventilation systems, thus modifying energy production and related emissions; warmer temperatures increase the energy demand for cooling in the summer, while decreasing the demand for heating in the winter (EEA, 2019a). In addition, changes to the hydrological cycle have an impact on hydropower (EEA, 2019a). An important source for PM in Europe is residential wood combustion, e.g., small-scale combustion units, where the ambient temperature influences the extent of the use.
1.4 Climate change impacts on chemical processes
The role of meteorological factors such as temperature and relative humidity for atmospheric chemistry is to a large extent through its influence on the hydroxyl radical (OH), which is the major component for the oxidizing capacity of the troposphere and thus crucial for the chemical removal of pollutants. The primary sources of OH are the photolysis of O3, and reaction with water vapor, the latter increasing with a warming climate (Seinfeld and Pandis, 2016). Increase in temperatures results in an increase in summertime ozone over polluted areas due to increased photochemical activity, and a reduction of background ozone due to an expected increase in water vapor (Jacob and Winner, 2009). In addition, increasing temperatures also increase sulfate aerosol (SO42−) formation due to faster sulfur dioxide (SO2) oxidation, while nitrate (NO3−) particles decrease as ammonium nitrate evaporates and nitrate shifts from the particle phase to the gas phase (Dawson et al., 2007). On the other hand, increasing temperatures can also reduce SO4= aerosols due to less available OH to react with SO2 in order to produce SO42−, as increased biogenic emissions also react with the available OH, thus competing with SO2 (Im et al., 2012). SOA can both be reduced due to the shift from particle to gas phase and increase due to the increase of biogenic SOA precursors (Sheehan and Bowman, 2001; Kanakidou et al., 2005; Tsigaridis and Kanakidou, 2007).
1.5 Climate change impacts on production, dispersal, and removal rates
In addition to temperature, there are other meteorological factors affecting the production, dispersion, and removal of air pollutants. Climate change can modify large-scale circulations (Otero et al., 2018), thus ventilation (wind speed/direction, mixing height). Lower wind speeds in polluted regions can cause O3 concentrations to increase due to longer residence/reaction time and due to increased aerodynamic resistance to dry deposition (Dawson et al., 2007). Mixing heights have a more complicated effect on O3 concentrations. Increasing mixing height increases surface O3 levels under low-surface O3 conditions due to the downwards transport of tropospheric O3 lying above the mixing layer, while it decreases O3 under high-surface O3 conditions due to the increased vertical dispersion of surface O3 (Liu et al., 1987; Kleeman, 2007). Changes in ventilation have stronger effects on particulate matter (PM) than on O3 because of the lower PM background concentrations (Jacob and Winner, 2009). Increasing ventilation rates can have a diluting effect on PM, as well as a compensating effect that is associated with a decrease in precipitation, leading to reduction in removal via wet deposition. Dry deposition of both gasses and PM are also dependent on the surface conditions, where e.g., a decrease in the snow cover can lead to an increase in the deposition over land. Opposite will a decrease in the sea ice extent result in a decreased removal over marine areas as O3 deposits slower to water surfaces than to sea-ice.
PM concentrations decrease with increasing precipitation as wet deposition is the main removal process for PM, in addition to sedimentation that affects coarse particles. Summertime PM levels are highly sensitive to precipitation frequency, when events tend to be convective and small in scale, while they show a low sensitivity in winter when synoptic-scale storms dominate. Changes in humidity and cloudiness also affect PM concentration and properties. Increasing relative humidity increases the PM water content and thus, the uptake of semi-volatile components, mainly for nitrate and organics. Sulfate can be produced by the aqueous-phase oxidation of SO2 by hydrogen peroxide (H2O2) in the cloud. However, due to the short time scale of this process (i.e., minutes), it is very challenging to simulate aqueous-phase sulfate formation in clouds in mesoscale or global models (Koch et al., 2003).
2 Climate change impacts on surface level air pollution
There are several approaches that are used in the literature in order to understand how O3 and particulate matter (PM) are influenced by changes in climate or emissions (Fiore et al., 2019). These include 1) sensitivity studies in which individual meteorological parameters are perturbed, 2) statistical downscaling of future changes in meteorology, using correlations between observed changes in air quality and meteorological variables from climate models, 3) the direct calculation of air quality by using fully coupled global or regional chemistry–climate models (CCM), global-to-urban CCMs, or off-line global or regional chemical transport models (CTMs) forced by either projections of meteorological fields from separate atmosphere–ocean general circulation models, or dynamically downscaled meteorology. In addition, there is also large literature available on the statistical relationships between observed meteorological parameters and air pollution concentrations (Im et al., 2006, Im et al. 2008, Jacob and Winner, 2009). Such studies aid in understanding the short-term changes in air pollution concentrations with changes in meteorological factors, which can be used to extrapolate for future conditions (e.g., Cheng et al., 2007; Lin et al., 2007).
2.1 Climate change impacts on surface ozone
Atmospheric O3 and its sensitivity to climate change has gained increasing attention throughout the last decades. First in simplified sensitivity studies where one parameter (e.g., temperature) was changed in chemistry transport models (CTM) and then in more complex studies using chemistry-climate models directly or by using CTMs driven by meteorological 3-D fields projected by climate models (e.g., Hedegaard et al., 2008). Some studies have looked at the global scale, while more studies have focused on projections of the chemistry-climate impacts over polluted regions in mainly the United States and Europe. In order to isolate the effect of climate change, the anthropogenic emissions have typically been kept constant in these studies. In more recent years a number of reviews have gone through the literature summarizing the latest findings regarding the climate change penalty on surface O3. We will in the following focus on recent findings for Europe and give an overview of the related uncertainties and knowledge gaps.
Figure 2 summarizes the recent findings on the impacts of climate on the future levels of European O3, adopted from Fu and Tian (2019). The potential future developments in air pollution levels in Europe were studied in a multi-model setup including four state-of-the art chemistry-transport models (Lacressonnière et al., 2016; Watson et al., 2016). Assuming +2°C global warming, the model simulations pointed towards a modest increase in the average O3 levels over Europe during summer (−0.1 to +0.8 ppb relative to present day), but the results were not statistically significant across the four models (Watson et al., 2016). When analyzing the SOMO35 (sum of daily maximum O3 exceeding 35 ppm) in more details, Lacressonnière et al. (2016) showed that the majority of the models simulate an increase in summertime O3 across Europe, and for parts of central Europe, Eastern Europe and in Spain all the models agree on higher surface O3 levels during the summer. All models indicate smaller changes during winter. Similarly, Collette et al. (2015), using a meta-analysis of 25 model projections, found a summertime penalty in the continental Europe surface O3 levels of [0.44–0.64] and [0.99–1.50] ppb for the periods 2041–2070 and 2071–2100, respectively. However, these numbers include a latitudinal tendency in the penalty. In Southern and Central Europe, the climate penalty is more pronounced and by the middle of the century the summertime change in O3 can exceed + 1 ppb. In contrast, a small decrease in the summertime O3 level was projected at more northern latitudes (Scandinavia and British Isles).
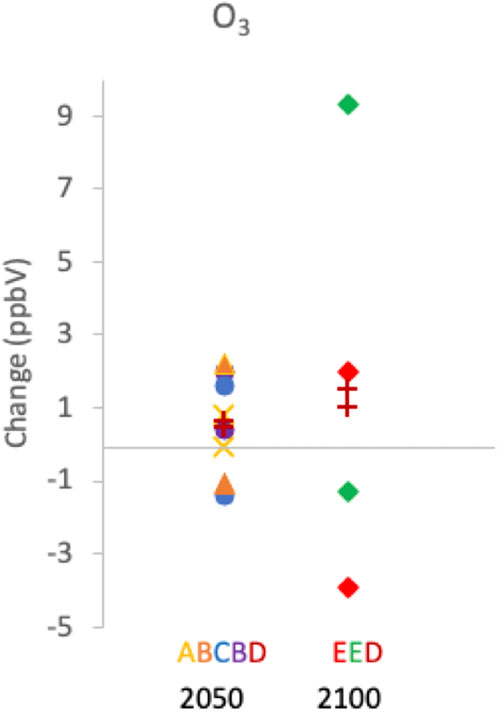
Figure 2. Model projections of O3 as calculated by the studies included in this review, based on Fu and Tian. (2019). The changes are color- and symbol-coded by the studies; (A) Watson et al. (2016), (B) Fortems-Cheiney et al. (2019), (C) Glotfelty et al. (2016), (D) Colette et al. (2015), and (E) Schnell et al. (2016). Each study is represented by the lower and upper estimates of the projections if available.
In a more recent study, Fortems-Cheiney et al. (2019) used the CHIMERE CTM in combination with the RCP8.5 and RCP4.5 climate scenarios and scenarios for anthropogenic emissions to investigate the impact of a +3°C and +2°C temperature change. Based on simulations including emissions for 2050 (the ECLIPSE-v4a CLE) the differences in the annual mean European O3 levels between a +2°C (31.99 ± 1.01 ppb) and +3°C (34.41 ± 1.07 ppb) scenario are statistically significant. On average the levels are 8% higher in the +3°C scenarios, illustrating the impact of the higher temperature alone (the impact from emissions will be discussed below). In relation to health issues, it is important to note that this study also finds that the frequency of days with high O3 levels are increasing in the +3°C scenario leading to e.g., a higher number of days where the WHO limit value for ozone is exceeded in especially the South-eastern part of Europe. Fortems-Cheiney et al. (2019) concluded this was linked to a lower boundary layer height and a larger number of very hot summer days in this region in the warmer scenario.
Using simulations with five CMIP6 models considering future climate (ssp370SST) and present-day climate (ssp370pdSST) under the same future emissions trajectory (SSP3-7.0), Zanis et al. (2022) estimated a non-robust slight ozone benefit over Europe on an annual basis and in winter, which becomes slightly positive but non-robust among the models (an ozone penalty of 0.2 ppb °C−1) during summer. These slightly negative or positive (in summer) signals Europe are attributed: 1) to the generally much lower NOx emissions, 2) to contributions from enhanced BVOC emissions and lightning NOx emissions, and 3) the competitive role of the large-scale background ozone chemical destruction linked to more water vapor under global warming.
I emission of biogenic VOCs (BVOCs) and the driving feedback processes is one of the key knowledge gaps (Fu and Tian, 2019). The terrestrial biosphere emits BVOCs as part of photosynthetic activity and the emission is a function of e.g., plant species as well as a number of environmental parameters like temperature and sunlight (Hantson et al., 2017). Natural BVOCs (e.g., isoprene and monoterpene) are therefore by nature highly variable in space and time. A warming climate can directly influence the emissions of biogenic VOCs and thereby O3 levels (Im et al., 2011). The BVOC emissions are typically calculated on-line in the chemistry-transport models and several of the studies referred to above include a significant increase in the BVOC emissions in the projections for the future. However, the sensitivity to temperature can differ substantially between chemistry-transport models (Langner et al., 2012). Newer studies use more complex and dynamic vegetation models in order to assess the future developments in biogenic VOC emissions, when taking other drivers than temperature into account (e.g., Hantson et al., 2017; Bauwens et al., 2018). They show that increasing atmospheric CO2 concentrations can lead to increased biogenic VOC emissions (the greening effect), but similarly can the inhibitory CO2 effect work against this increase. The overall impact of processes is still not well understood. Likewise, natural, and anthropogenic induced vegetation changes can lead to changes in the distribution and strength of the biogenic VOCs emissions across Europe in the future. These studies indicate that the projections for future developments in biogenic VOCs still are very uncertain.
The large variability in the future surface O3 levels in the different studies stem from several reasons including the uncertainty in biogenic emissions, the differences in models and their spatial/temporal resolution, and finally, the different emissions/climate scenarios used in the different studies.
2.2 Climate change impacts on surface particulate matter and components
2.2.1 Impacts on particulate matter mass
Impacts of climate on aerosol levels have been extensively studied over the last few decades. However, different studies focused on different time horizons (e.g., mid-century or end of the century), using different climate scenarios, and different chemistry and transport models (CTMs) that are either coupled to global/regional climate models, or CTMs driven by global climate projections downscaled to European scale. This section focuses on estimates of the isolated impacts of climate on the particulate matter (PM) levels and their chemical composition over Europe (i.e., model studies where emissions of PM precursors are kept constant). Figure 3 summarizes the changes in PM and its components as calculated in the different studies. In general, studies agree in the direction of the response, regardless which climate scenario has been implemented, with business-as-usual scenarios giving the largest changes for the anthropogenic species. Dust concentrations stay relatively constant throughout the century, while sea-salt is projected to increase. It can also be seen that most studies calculated a weak impact of climate change on the chemical composition, while the level of confidence varies from low to medium as discussed in Jacob and Winner (2009), Fiore et al. (2012), and Isaksen et al. (2009), as summarized in Table 1.
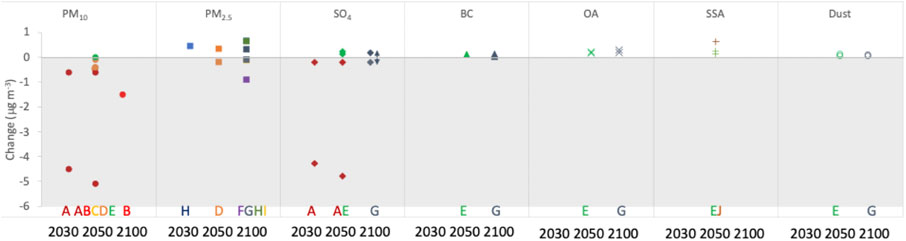
Figure 3. Model projections of PM and its components as calculated by the studies included in this assessment report. The changes are color- and symbol-coded by the studies; (A) Lacressonniere et al. (2014), (B) Juda et al. (2012), (C) Manders et al. (2012), (D) Lacressonniere et al. (2017), (E) Cholakian et al. (2019), (F) Lemaire et al. (2016), (G) Westerveld et al. (2016), (H) Silva et al. (2017), (I) Park et al. (2020), (J) Soares et al. (2016). Each study is represented by the lower and upper estimates of the projections if available.
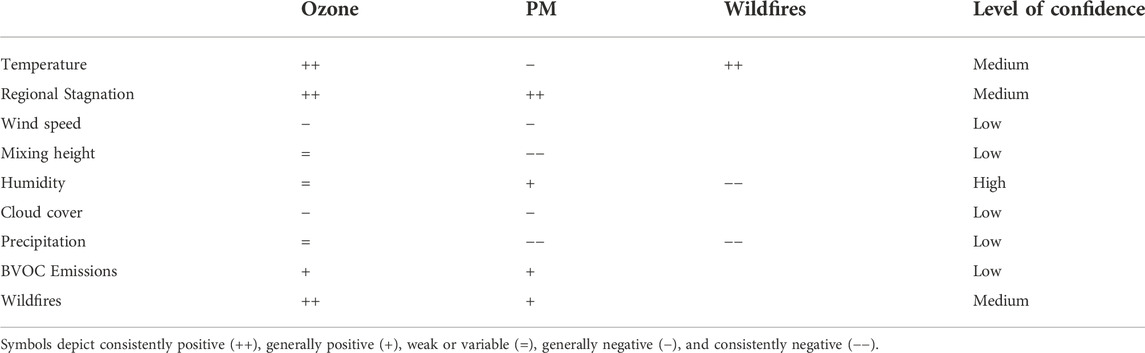
Table 1. Impacts of temperature-driven pathways on surface O3 and PM, adapted from Jacob and Winner (2009), Isaksen et al. (2009) and Fiore et al. (2012).
While some studies performed sensitivity simulations to quantify the impact of various meteorological parameters on surface PM2.5 levels, others performed climate simulations in order to study the evolution of future PM2.5 concentrations. For example, Megaritis et al. (2014) investigated the impact of different meteorological variables on PM2.5 concentrations, perturbing temperature, humidity, precipitation, and wind speeds individually, in prescribed increments. They showed that in Europe, PM2.5 concentrations change by −0.7 to 0.3 μg m−3 per 1 K increase in temperatures, while they change by −0.13 to 0.16 μg m−3 per 1% change in humidity, by −0.11 μg m−3 per 1% increase of precipitation and by 0.14 μg m−3 per 1% increase of wind speed. They concluded that precipitation is expected to have the largest impact on PM2.5 concentrations under a changed future climate, driven mainly by the accelerated wet deposition of PM2.5 species and their gas precursors. They showed that PM2.5 concentrations can change by up to ±2 μg m−3 in the future due to changes in precipitation. In addition, they projected that changes in wind speed over Europe in the future can change PM2.5 levels up to ±1.4 μg m−3.
Surface PM10 concentrations over Europe are projected to decrease in many studies due to meteorological factors. In the near-future (2030), PM10 (PM with a size below 10 μm) concentrations are projected to decrease by 0.6 μg m−3 (summer) to 4.5 μg m−3 (winter) (Lacressonnière et al., 2014), while in 2050, PM10 concentrations are projected to decrease by 5.1 to 0.1 μg m−3 (Juda et al., 2012: Lacressonnière et al., 2014, Lacressonnière et al., 2017, Cholakian et al., 2019). These differences are attributed to increases in winter precipitation (Juda et al., 2012: Lacressonnière et al., 2014, 2017), increases in humidity (Lacressonnière et al., 2014), or changes in wind patterns and mineral dust emissions (Lacressonnière et al., 2017). Very recently, Cholakian et al. (2019) projected a decrease of PM10 concentrations by 0.01, 0.01, and 0.42 μg m−3 under the RCP2.6, RCP4.5, and RCP8.5 climate scenarios, respectively. Their analyses showed that these changes can vary seasonally and by the different aerosol species comprising the PM mass. They also showed that when the impacts of climate change are isolated, nitrate decrease governs the decrease of PM10 and PM2.5 in RCP4.5 and RCP8.5; however, in RCP2.6, the increase in dust, sea-salt and biogenic SOA particles outweigh the decrease in nitrates.
Opposite to PM10, meteorological conditions are projected to lead to an increase in surface PM2.5 concentrations over Europe by mid-century. PM2.5 (PM with a size below 2.5 μm) concentrations are projected to increase slightly in most studies. There are, however, also studies showing decreases, but in smaller magnitudes compared to the increases. Silva et al. (2017) showed that population-weighted PM2.5 concentrations will increase by 0.3 μg m−3 in 2030 under the RCP8.5 scenario. By the mid-century, PM2.5 levels are projected to change by −1.6 to 2.47 μg m−3 (Lacressonnière et al., 2016; Lemarie et al., 2016; Silva et al., 2017). Lacressonnière et al. (2016), using three regional CTMs coupled with climate models, calculated an ensemble mean change in PM2.5 concentrations of −0.5 to 1.3 μg m−3. They have found an increase of PM2.5 over southwestern Europe, while a decrease is projected over some parts of eastern and central Europe. They showed that the largest differences due to changes only in climate are due to dust and sea salt, which are largely dependent on meteorological fields, as described above.
By the end of the century, studies do not agree on the direction of the changes in surface PM2.5 concentrations over Europe. Lemarie et al. (2016) projected a decrease of area-weighted PM2.5 concentrations by 0.98 μg m−3 by the end of the century, mainly attributed to changes in surface temperature. They suggested a strong influence of high vertical stability events, where low surface temperature and planetary boundary layer (PBL) height leads to an increase in PM2.5 concentrations, while for high temperature ranges, the PBL height becomes a less discriminating factor. However, they suggest that even though the PBL depth constitutes the most important meteorological driver for PM2.5, it does not evolve notably compared to the surface temperature in the future. Recently, Park et al. (2020), using a set of ACCMIP global models, showed that in 2100, PM2.5 concentrations over Europe will increase on average by 0.13 μg m−3 (5.1%), ranging from −0.23 to 0.50 μg m−3, under the RCP8.5 scenario, in agreement with Westervelt et al. (2016) who calculated an increase of 0.19 μg m−3 in PM2.5 concentrations. Westervelt et al. (2016) also showed a decrease of PM2.5 concentrations by 0.2 μg m−3 in 2100 if RCP2.6 is used instead of RCP8.5.
2.2.2 Impacts on chemical composition
In addition to the direct impacts of meteorology on PM levels and composition, impacts due to change in emissions are also important. Im et al. (2012) calculated that higher temperatures increase biogenic emissions, thereby enhancing biogenic SOA by 0.01 ± 0.00 μg m−3 K−1 and NO3− aerosol concentrations by 0.02 ± 0.02 μg m−3 K−1. They also showed that increased temperatures reduce anthropogenic SO42− by 0.04 ± 0.07 μg m−3 K−1, induced by significant reduction in the cloud cover (90% K−1) and subsequent aqueous-phase production. The PM2.5 concentrations show a very small positive response to temperature changes, increasing by 0.003 ± 0.042 μg m−3 K−1 (0.04% K−1) due to the compensation of organic carbon increases by nss-SO42− reductions. They also showed that locally, larger changes can take place, with anthropogenic SO42− and NO3− in fine aerosols reduced by up to 0.62 and 0.80 μg m−3 K−1, respectively. Increases as high as 0.097 and 0.034 μg m−3 K−1 are calculated for organic and elemental carbon, respectively. Results show that changes in temperature modify not only the aerosol mass but also its chemical composition.
Regarding SO42− aerosols, studies are not consistent in the direction of the change in concentrations in response to changes in climate. Lacressonniere et al. (2014) projected a decrease of SO42− concentrations by 4.2 to 0.3 μg m−3 in 2030, while in 2050, they projected a slightly larger decrease, which they attributed to higher precipitation and humidity in 2050 compared to 2030, enhancing the transformation of SO2 into SO42− aerosols. Cholakian et al. (2019), on the other hand, projected an increase of SO42− by 0.02 μg m−3 (RCP8.5) to 0.11 μg m−3 (RCP4.5) in 2050, based on the different RCP scenarios they used, attributed to higher humidity in winter and higher OH levels in summer due to increased temperatures. They also showed that decreased mixing heights contributed to these increases.
All the different studies agreed on a likely increase of organic aerosols (OA), due to increased temperatures, and thus increase in biogenic emissions and OH levels. While increased temperatures lead to increased biogenic emissions, which in return consume the available OH, it also leads to increased OH by regeneration chemistry by increasing O3 in areas with sufficient levels of NOx. Cholakian et al. (2019) projected an increase of 0.06 to 0.12 μg m−3 in 2050 under the different RCP scenarios. Westervelt et al. (2016) projected end of the century increases of 0.09 to 0.19 μg m−3 under RCP2.6 and RCP8.5 scenarios, respectively. Regarding sea-salt concentrations, these slightly increase by 0.01–0.11 μg m−3, due to increases in wind speeds (Cholakian et al., 2019) and sea-surface temperatures (Soares et al., 2016), leading to increased sea-salt emissions. Regarding dust concentrations, Westervelt et al. (2016) shows a slight increase in 2100 according to the RCP2.6 scenario due to an increase in wind speeds, while in RCP8.5, a decrease is projected due to a decrease in wind speeds.
The variability in the future surface aerosol levels and composition in the different studies stem from several reasons including the uncertainty in biogenic emissions, which impacts the biogenic SOA formation, the differences in models in terms of aerosol representations, in particular how they treat SOA and their spatial/temporal resolution, and finally, the different emissions/climate scenarios used in the different studies.
3 Impacts on air pollution due to changes in emissions vs. changes in climate
Several of the model studies included in this review have also analyzed the combined effect of future changes in both climate and air pollutant and precursor emissions such as NOx, CO, SO2, NMVOCs, and primary particles. For Europe, the anthropogenic emissions of the main air quality components (e.g., SOx, NOx, and PM2.5) are projected to decrease in the future posing the central question whether the benefits of these emission reductions are counteracted by a possible climate penalty.
The latest CMIP6 models under different future scenarios, shared socio-economic pathways (SSPs) showed that O3 concentrations over Europe can increase by up to 4 ppb compared to the 2005–2014 mean under low mitigation scenarios (e.g., SSP3-7.0 and SSP5-8.5), while high mitigation scenarios lead to decreases by up to 16 ppb (in SSP1-2.6) (Turnock et al., 2020). Surface PM2.5 concentrations over Europe, on the other hand, are projected to decrease consistently by all models by 1–4 ug m−3. The recent studies conclude that expected emission changes in comparison to impacts of climate will have the largest impact on European O3 levels (e.g., Colette et al., 2015; Westervelt et al., 2016). This conclusion is, however, very dependent on the scenarios used for Europe, but also for the developments outside Europe. In the study by Fortems-Cheiney et al. (2019), the high emission scenario RCP8.5 and the related higher European O3 concentrations, would to a large degree be caused by inflow from outside Europe. In the more detailed study by Lacressonniere et al. (2014), it is also shown that long-range transport from outside Europe can increase the O3 levels in Northern Europe in spite of decreasing European emissions. The impact of the European emissions was studied directly by Fortems-Cheiney et al. (2019) as they were running a CTM with both current day (2005) and future (2050) European emissions and future climate data. The applied Current Legislation emissions for 2050 projects a significant decrease (more than 40%) in most components across Europe (except for the Turkish area). When emissions are fixed to 2005 under RCP8.5 for 2050, the mean summer O3 levels increase by up to +12% (+4.30 ppb), except in urban areas with high NOx emissions. Studies also showed that the anthropogenic emissions within the European region have the largest impact on the European PM concentrations towards the end of the century, compared to developments in both climate and long-range transport (Cholakian et al., 2019).
The future developments in health impacts related to air pollution have also been assessed in a number of studies (see e.g., the review by Doherty et al., 2017 and the global study by Silva et al., 2017). Geels et al. (2015) combined two CTMs with a health assessment model and focused on the development in the number of premature deaths related to PM2.5 and O3 in Europe in the 2050s and 2080s. Climate change alone in the RCP4.5 scenario, where emissions were kept constant, had only minor impacts (±2%–4%) on the PM2.5 related number of cases, while the number of cases related to O3 increased by up to 10% (relative to the 2000s). Including the emissions from the same RCP4.5 scenario leads, however, to significant decreases in the health effects especially for PM2.5 with a significant decline in the number of premature deaths (∼65% in 2050s and ∼80% in 2080s). But, as pointed out by Doherty et al. (2017), the health assessments for future conditions are related to additional uncertainties as e.g., the temperature effects on applied pollutant-response relationships are not well known.
4 Feedbacks from air pollution on climate
Air pollutants like O3 and different types of aerosols [e.g., black carbon (BC), SO42-, NO3− and organic aerosols (OA)] have important radiative effects on climate, while other pollutants such as carbon monoxide (CO), VOCs, nitrogen oxides (NOx) and SO2, although being negligible greenhouse compounds themselves, have an important indirect effect on climate by altering the abundances of radiatively active gasses such as O3 and CH4, a well as by acting as precursors for secondary inorganic and organic particles (Stohl et al., 2015).
Tropospheric climate–chemistry interactions are to a large extent related to chemical and physical processes and to compounds that show large variability in global distribution and trends, mainly driven by precursor emissions that also show large spatial variability. Atmospheric composition influences climate by regulating the radiation budget and cloud properties (Boucher et al., 2013; Stohl et al., 2015; Bellouin et al., 2020), through the presence of water vapor (H2O), CO2, CH4, O3, and aerosols. The main radiative effect of the gasses is through the greenhouse effect (i.e., through interference with long-wave radiation), thus heating the atmosphere, while aerosols may either heat or cool the surface (through interference with short-wave radiation), depending on their optical properties (Table 2: Bond et al., 2013; Stohl et al., 2015).
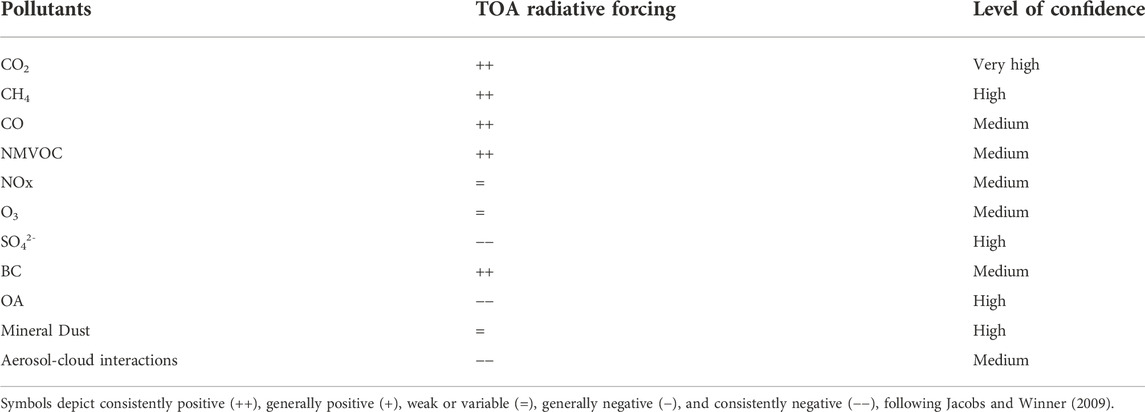
Table 2. Impacts of gasses and aerosols on the top of the atmosphere (TOA) radiative forcing due to aerosol-radiation interactions (ARI), adapted from Myhre et al. (2013).
Aerosol–cloud interactions represent an area with potential for strong interactions in the climate system (Myhre et al., 2013). The largest uncertainty in the climate projections caused by the interaction of aerosols with clouds (Lee et al., 2016; Mülmenstädt and Feingold, 2018). The representation of aerosol-cloud interactions in ESMs remains a challenge in the Sixth Assessment Report (AR6) from IPCC, due to the limited representation of important sub-grid scale processes, from the emissions of aerosols and their precursors to precipitation formation and lack of certain processes such as contribution from anthropogenic aerosols acting as ice nucleating particles (INP) and aerosol effects on deep convective clouds. Aerosols determine the cloud radiative properties and thus the radiative heating/cooling and participate in the precipitation process. Although considerable progress has been made in recent years to include parameterization of aerosol–cloud droplet interaction and explicit microphysics for cloud water/ice content in climate models, inadequate understanding of the processes contributes to significant uncertainties in model simulated future climate changes (Table 2: Malavelle et al., 2017; Toll et al., 2019). According to IPCC AR6, effective radiative forcing due to aerosol-cloud interactions (ERFaci) based on observational evidence alone (–1.0 ± 0.7 W m−2) is very similar to the one based on model-evidence alone (–1.0 ± 0.8 W m−2), with a medium confidence (Table 2), compared to the low confidence in AR5.
Regarding the trends in emissions of air pollutants affecting radiative forcing, large differences are observed in the temporal and geographical distributions of human-related (anthropogenic) emissions and natural emissions (Shindell and Faluvegi, 2009; Flanner, 2013). Anthropogenic emissions respond to regulations and to different growth rates in energy use, transportation, and industry. For example, emissions from Europe generally show reductions thanks to the regulatory actions taken over the last decades (EEA, 2019b), while emissions in regions like Southeast Asia, and in other developing countries show large increases during recent years (IEA, 2020). In addition to geographical differences, there are also sectoral differences, such as the large increases observed in aircraft and shipping (Isaksen et al., 2009). On the other hand, natural emissions of key climate precursors (NOx, CO, biogenic VOCs and sulfur compounds) are difficult to regulate and exhibit large year-to-year variations (e.g., in the case of biomass burning).
5 Climate change impacts on wildland fires
Climate change causes an increasing number of wildfires in some regions (Lozano et al., 2017) because it increases wildfire risk by raising the average temperature of the atmosphere, intensifying droughts, and prolonging the dry season (Figure 4). Driving mechanisms behind the fires have varied in different historical periods. Pechony et al. (2010) argued that in the preindustrial period, fires were strongly driven by lightning, rather than temperature. With the Industrial Revolution, this changed to an anthropogenic-driven regime (Sofiev et al., 2013), and there are now indications that we are moving towards a temperature-driven fire regime during this century. However, currently the main cause of wildfires is anthropogenic activities related to agriculture and forest management practices, as well as from recreational habits (Sofiev, 2013; Dijkstra et al., 2022). In Southern Europe, more than 95% of the fires with a known cause have an anthropogenic origin (Ganteaume et al., 2013; Parente et al., 2018), while in central Europe, more than 99% of fires are caused by humans (Ganteaume et al., 2013).
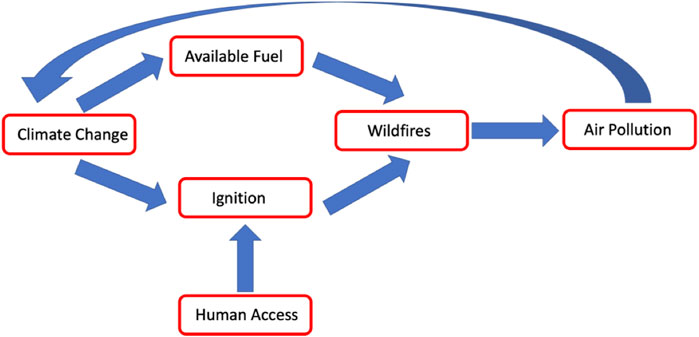
Figure 4. Feedback between climate change and wildfires adopted from Fischer et al. (2016).
Modelling the impacts of climate change on wildland fires is very challenging due to the complex dynamics of fuel accumulation, vegetation dynamics and their interactions with changing climate, as well as the difficulty in representing the fire behavior patterns due to impacts of land management and human ignitions (Hantson et al., 2020; Sanderson and Fisher, 2020). Thus, projection of wildland fires over decadal to century timescales requires more mechanistic approaches, capable of capturing the numerous interacting system components that affect the evolution of fire risk. Process-based global fire models based on these principles have progressed rapidly over the past decade, although their use in fully coupled earth system models is still not standard practice (Sanderson et al., 2020), leading to omitting a potentially important component of the global carbon–climate feedback.
Interaction between the anthropogenic activity, land cover, meteorological conditions and intensity of wildfires (expressed as the amount of biomass burned or pollutants released) basically resides to the human activities playing the fire ignition and suppression roles whereas vegetation and weather modulate the natural fire lifetime and potential for spreading (Prestemon et al., 2013; Fusco et al., 2016; Balch et al., 2017). Most studies that have estimated future fires (risk or area burned) have relied on statistical regressions. However, it is highly regionalized. For instance, in Indonesia (one of the largest sources of fire-induced pollution worldwide) the use of fire for clearing and preparing land on degraded peat is a routine repeated every year—with fires routinely getting out of control. However, it is the El-Nino-induced drought that can make them devastating (Field et al., 2015). In the Western US, worsening climatic conditions will lead the fires to become the main source of PM2.5 if human behavior and practices remain the same (Yue et al., 2013; Liu et al., 2016). Similarly, Ford et al. (2018) found that, despite the reduction of anthropogenic PM2.5, raising fire contribution will lead to a net increase of PM2.5 in several US regions.
Wildfires are known to be the largest contributor to global biomass burning. They are a large source of atmospheric trace gasses and aerosols, in addition to major vegetation disturbance (Knorr et al., 2016). They emit into the atmosphere primarily CO2 among other greenhouse gasses and carbonaceous particulate matter among other aerosols (Saarikoski et al., 2007). However, our knowledge of wildfires as an integrated part of the Earth system is still rather weak (Bowman et al., 2009; Langmann et al., 2009; Keywood et al., 2013).
In Europe, climate change is expected to increase the probability of forest fires in areas where increase in summertime temperature is accompanied by decrease in precipitation, as in the Mediterranean area (Mouillot et al., 2002; Bedia et al., 2014; Kalabokidis et al., 2014). In more northern regions the estimations of precipitation amounts are less conclusive (Jylhä et al., 2009; Ylhäisi et al., 2010; Venäläinen et al., 2020; Ruosteenoja and Jylhä 2021), which makes predicting the fire sensitivity much more difficult. A global modelling study of Knorr et al. (2017), unlike the research quoted above, gives a calmer picture. The authors conclude that at the global scale anthropogenic emission reduction will exceed increases in wildfire emissions and the WHO target of 10 μg m−3 will be in reach in many regions. However, the authors also admitted high uncertainty connected with the fire abatement measures that were accounted for.
Air pollution due to PM2.5 released from vegetation fires is a notable risk factor for public health everywhere in the world, also in Europe (Johnson et al., 2020). This should be taken into consideration when evaluating the overall health and socio-economic impacts of the fires. Kollanus et al. (2017) evaluated that in 27 European countries, over a thousand premature deaths were attributable to the vegetation-fire originated PM2.5 concentrations in 2005 and 2008. Estimated impacts were highest in southern and Eastern Europe, but all countries were affected by fire-originated PM2.5. Saarnio et al. (2010) analyzed smoke plumes originating from wild-land fires, which were detected in Helsinki, Finland, during a 1-month period in August 2006. The measurements showed that the major growth in PM concentration was caused by submicron particles consisting mainly of particulate organic matter. They reported an increase of a factor of 1.5–3.5 in PM2.5 concentrations. Saarikoski et al. (2007) calculated that the wildland fires increased the PM2.5 concentrations by a factor of 3.6–4.3. Sofiev et al. (2008) considered the influence of wild-land fires on air quality in Europe, using spring and summer seasons of 2006–2007 as prominent examples. High concentrations of nearly all pollutants were detected in Central, Eastern and Northern Europe in late spring of 2006, causing widespread allergic symptoms and other illnesses associated with poor air quality.
The aerosols produced by fires seem to be different from those emitted from e.g., anthropogenic industrial or transport sources: intriguing observations of Dusek et al. (2005) showed that peat-fire smoke has much weaker interaction with clouds than one would expect: less than 50% of smoke particles of suitable size were activated to CCN, whereas this number often approaches 100%. Therefore, the fire aerosols may not be very efficient shielding agents compared to other types of particles. The other factor is darkening of the surface after the fires: albeit small (at 1 km scale the albedo change is ∼0.002 in sub-Saharan Africa (Gatebe et al., 2014)) these changes occur over wide areas and constitute noticeable additional radiative forcing.
Generally, there is some agreement that carbonaceous aerosol emissions from anthropogenic sources may decrease in the future (Lamarque et al., 2011; Bond et al., 2013; Shindell et al., 2013). Global total carbonaceous emissions are expected to be reduced due to new, less polluting technologies along with a shift away from the burning of coal and wood in the residential sector, while this reduction will vary regionally and not be reduced everywhere (Streets et al., 2004). However, the future trends of wildfires and their aerosol emissions is more uncertain due to complicated interactions between fire, land, atmosphere, and, most importantly, anthropogenic activities. Even now the state-of-the-art Earth system models have very limited capacity to simulate wildfire—climate interactions (Carslaw et al., 2010).
Estimating the fire emission heights is of particular importance when evaluating the coupling between terrestrial and atmospheric processes. Emission heights affect the aerosol long-range transport, aerosol-cloud interaction, and radiation (Luderer et al., 2006; Samset et al., 2013). One large problem is that emission heights are strongly affected by fire intensity and atmospheric stability. As illustrated by Joshi et al. (2007) and Luo et al. (2013), both of these are expected to change in the future. The emission height and its effect on atmospheric black carbon and its transport has been evaluated using present day climate conditions by Veira et al. (2015a,b), Sofiev et al. (2012, 2013), Kukkonen et al. (2014), and Daskalakis et al. (2015). Attempts to apply that same approach to future climate have more recently been done by Veira et al. (2016).
Radiative forcing due to aerosols originating from wildfires has mostly been investigated using present-day climatology and prescribed wildfire emission inventories (satellite based). Based on the literature review by Veira et al. (2016), the most recent climate-aerosol models find that the total wildfire top of the atmosphere radiative forcing range between +0.18 W m−2 (Tosca et al., 2013) and −0.29 W m−2 (Jones et al., 2007).
6 Co-benefits and trade-offs related to GHG and air pollution mitigation and adaptation policies
There is a general consensus among studies that there are additional (co) benefits from mitigation of green house gases (GHG) emissions in terms of improved air quality (West et al., 2013; Bollen 2015; de Oliveira and Doll 2016; Klausbruckner et al., 2016; Rao et al., 2017), but these are rarely taken into account in policy developments and assessments at the European and national scale (Sokhi et al., 2022). The estimated improvements in air quality under different climate mitigation scenarios depend on socioeconomic and technological development and the assumptions on the development of air pollution in the next few decades. Rao et al. (2017) examined the impacts of different air pollution narratives under the global Shared Socio-economic Pathway (SSP) framework, concluding that scenarios with limited climate change mitigation present higher air pollution impacts than scenarios with more stringent mitigation trajectories. An overview paper (von Schneidemesser et al., 2015) concludes that any policy actions intended to mitigate one of these two issues must necessarily take into account the feedback with the other, to avoid that benefits to one sector will worsen the situation in another.
The effect of air pollution policies on climate have been investigated in some studies. Shindell et al. (2012) identified 14 emission control measures targeting short-lived climate pollutants (SLCPs) and relative to their reference scenario, found that all CH4 and BC control measures avoid a global mean surface temperature increase of ∼0.5°C by 2050, although significant uncertainties exist regarding the extent to which reductions of SLCFs impact climate change (Myhre et al., 2013). In light of the estimated large adverse health impacts of air pollution around the globe, emission controls on SO2, BC, and O3 precursors may continue to be imposed irrespective of climate policy (Anenberg et al., 2012; Silva et al., 2013).
Reduction of non-SLCF pollutants, such as NO2 and SO2, generally leads to exacerbation of climate change because these species are the key aerosol precursors and responsible for almost 1 W m−2 or shielding from the solar radiation roughly-equally split between direct and indirect effects (IPCC AR5, Physical science basis). The secondary inorganic aerosols mainly consisting of nitrates and sulfates are highly reflective and highly soluble, thus facilitating both direct (reflection of sunlight back to space) and indirect (increasing the cloud albedo and modifying the cloud cover and rain patterns) aerosol effects. Recent studies showed that, e.g., The International Convention for the Prevention of Pollution from Ships—Annex VI (MARPOL-VI) required reduction of sulfur content in ship fuels brings substantial health benefits (global avoided mortality 74–200 thousand cases annually, 95% confidence interval) but adds about 0.07 W m−2 due to lost cooling effects of sulfates (Sofiev et al., 2018). Estimates of other studies of the shipping cooling effect showed similar results suggesting the range from ∼0.04 W m−2 up to 0.11 W m−2 (Eyring et al., 2007; Fuglestvedt et al., 2008; Skeie et al., 2009; Lund et al., 2012).
Several studies show potential air quality co-benefits in Europe, including in the United Kingdom and Ireland (Karlsson et al., 2020; Alam et al., 2017, 2018; Wilkinson et al., 2009) and the Nordic countries (Åström et al., 2013; Williams, 2007). Rafaj et al. (2014) associate large potential co-benefits in Europe with renewable energy, due to reduced Mercury emissions to air. Collette et al. (2013) looked at combined projections of air quality and climate impact at the regional scale over Europe under the CMIP5 climate scenarios and conclude that exposure to air pollution will decrease substantially by 2050 according to a mitigation pathway that aims at keeping global warming below 2°C by the end of the century where exposure weighted SOMO35 and PM2.5 are reduced by 80% and 78%, respectively in Europe. Large potentials for reaping air quality co-benefits are also shown for several European cities, e.g., Rotterdam (Tobollik et al., 2016) and Barcelona, Malmö, Sofia and Freiburg (Creutzig et al., 2012). Markandya et al. (2018) estimated that the co-benefits would make a valuable contribution towards covering the mitigation costs, from 7% to 84% in the EU-27 countries.
The impacts of climate adaptation policies on greenhouse gas emissions and air pollution have also been discussed. Classic adaptation strategies to increased temperatures like air conditioning have been shown to result in increased GHG emissions as well as increased air pollution (Abel et al., 2018; Watts et al., 2019). In a study in Eastern US, Abel et al. (2018) found that in a warmer climate 3.8% of the total increase in PM2.5 and 6.7% of the total increase in O3 are attributable to extra air conditioning use. Other climate adaptation actions like improving urban design to reduce heat stress can have significant co-benefits for air pollution (Cheng and Berry, 2013).
7 Summary and recommendations
The atmospheric concentrations of secondary pollutants like ozone or organic aerosols are expected to increase with the projected global warming, mainly due to an increase in the oxidation capacity of the atmosphere and the increase in natural emissions. On the other hand, the projected increases in precipitation are expected to enhance removal of pollutants from the atmosphere, resulting in a weak positive or negative change in the pollutants concentrations, in particular in the first half of the century. This strongly suggests that feedback between pollutants and climate change play a crucial role in the direction of the policy response on future climate change and air pollution. The net changes caused by the feedback vary geographically and seasonally, and, importantly, all studies reviewed here showed that changes in anthropogenic emissions had much larger impacts on air pollutant concentrations in the future, compared to the corresponding impacts caused by the projected changes in climate.
Studies showed that in most cases, there may be immediate air quality co-benefits from mitigation of GHGs due to reduced emissions of co-emitted air pollutants, such as aerosols, from the same sources such as energy production and industrial combustion. However, for instance increasing the residential combustion of wood due to climate policies may result in a substantial deterioration of air quality (e.g., Kukkonen et al., 2020). Noteworthy, the reverse relation does not necessarily hold: improving air quality often raises the challenges to climate change mitigation, since it may diminish the amount of solar radiation scattered back into space.
Socioeconomic and technological developments may influence the potential for air quality co-benefits. Recent findings suggest that limiting climate change mitigation today would entail higher air pollution levels in the future. Due to the adverse human health impacts of these pollutants, policies will continue to be implemented to reduce the emissions, in order to follow for example, the recent air quality recommendations from WHO. This implies that stricter climate policies are needed to counteract the increased global warming resulting from the pollutant removals.
As this review shows, the projections of how climate and health relevant air pollution components (e.g., PM2.5 and O3) will develop in the future across Europe, are still associated with major uncertainties. The differences in the modeled projections can to a large degree be attributed to the differences in the design in these studies e.g., in terms of the applied emission and climate scenarios. However, the chemistry-transport models also show differences in meteorology-air pollution relationships and so far, these differences have not been analyzed in detail (e.g., Kukkonen et al., 2012).
More advanced ecosystem models in recent studies have shown that the complex feedbacks between ecosystems and changes in climate, CO2 levels, land-use etc. will modify the natural emissions of O3 precursors to a larger degree than has been previously known. A robust quantification of the direction of this change is not possible with the current understanding, which is one of the main knowledge-gaps related to projections of future surface concentrations of O3.
More research is needed for further development of climate and air pollution models, in terms of their capabilities in representing different chemical/physical processes and the response to climate change (Sokhi et al., 2022). There is a growing need to have integrated air pollution and related observations from both ground-based and remote sensing instruments, including in particular those on satellites. Use of upcoming new satellite observations such as the Plankton, Aerosol, Cloud, ocean Ecosystem (PACE) observations in general and SPEXone observations in particular, which can provide novel aerosol products related to size, composition, and absorption with high accuracy, while other instruments on PACE can provide accurate and novel cloud retrievals (droplet number concentration, droplet size, cloud phase), which can help improve the climate models in better quantifying aerosol-radiation and aerosol-cloud-precipitation interactions. Another emerging and growing field in observation science is the use of low-cost sensors, which can provide widespread information of air pollutant concentrations, but yet associated with large uncertainties when compared to existing monitoring networks. In order to get more robust projections with current generation models, multi-model studies will be needed, where scenarios and projection timelines are harmonized, as well as large ensemble studies using harmonized scenarios. For health impact studies, it is also important to increase the spatial resolution of the driving climate data in order to perform spatially high-resolution simulations of the evolution of the main air pollutants in the future. Higher accuracy in the air pollution simulations will also lead to more robust forecasting on the impacts of extreme weather and natural hazards such as wildland fires.
Author contributions
UI conducted the review, wrote the manuscript, and prepared the plots. CG, JK, and CS helped with the review and edited the manuscript, RH and MS helped with the wildland fires section and edited the manuscript, SR helped the mitigation/adaptation section and edited the manuscript, NS, ØH, JS, JC, RB, and KA edited the manuscript.
Funding
The author(s) declare that financial support was received for the research, authorship, and/or publication of this article. This project has received funding from the European Union's Horizon 2020 EMERGE project (No. 874990) and the Horizon 2020 EXHAUSTION project (Grant Agreement No. 820655).
Acknowledgments
The authors acknowledge the European Union's Horizon 2020 EXHAUSTION project (Grant Agreement No. 820655). This work reflects only the authors' view and the Innovation and European Climate, Infrastructure and Environment Executive Agency (CINEA) is not responsible for any use that may be made of the information it contains.
Conflict of interest
The authors declare that the research was conducted in the absence of any commercial or financial relationships that could be construed as a potential conflict of interest.
Publisher’s note
All claims expressed in this article are solely those of the authors and do not necessarily represent those of their affiliated organizations, or those of the publisher, the editors and the reviewers. Any product that may be evaluated in this article, or claim that may be made by its manufacturer, is not guaranteed or endorsed by the publisher.
References
Abel, D. W., Holloway, T., Harkey, M., Meier, P., Ahl, D., Limaye, V. S., et al. (2018). Air-quality-related health impacts from climate change and from adaptation of cooling demand for buildings in the eastern United States: An interdisciplinary modeling study. PLoS Med. 15 (7), e1002599. doi:10.1371/journal.pmed.1002599
Alam, M. S., Hyde, B., Duffy, P., and McNabola, A. (2017). An assessment of PM2.5 reductions as a result of transport fleet and fuel policies addressing CO2 emissions and climate change. WIT Trans. Ecol. Environ. 211, 15–27. doi:10.2495/AIR170021
Anenberg, S. C., Schwartz, J., Shindell, D., Amann, M., Faluvegi, G., Klimont, Z., et al. (2012). Global air quality and health co-benefits of mitigating near-term climate change through methane and black carbon emission controls. Environ. Health Perspect. 120 (6), 831–839. doi:10.1289/ehp.1104301
Arneth, A., Miller, P. A., Scholze, M., Hickler, T., Schurgers, G., Smith, B., et al. (2007). CO2 inhibition of global terrestrial isoprene emissions: Potential implications for atmospheric chemistry. Geophys. Res. Lett. 34, 18L18813. doi:10.1029/2007GL030615
Åström, S., Tohka, A., Bak, J., Lindblad, M., and Arnell, J. (2013). Potential impact on air pollution from ambitious national CO2 emission abatement strategies in the Nordic countries - environmental links between the UNFCCC and the UNECE - CLRTAP. Energy Policy 53, 114–124. doi:10.1016/j.enpol.2012.10.075
Balch, J. K., Bradley, B. A., Abatzoglou, J. T., Nagy, R. C., Fusco, E. J., and Mahood, A. L. (2017). Human-started wildfires expand the fire niche across the United Statesfires expand the fire niche across the United States. Proc. Natl. Acad. Sci. U. S. A. 114 (11), 2946–2951. doi:10.1073/pnas.1617394114
Bauwens, M., Stavrakou, T., Müller, J.-F., Van Schaeybroeck, B., De Cruz, L., De Troch, R., et al. (2018). Recent past (1979–2014) and future (2070–2099) isoprene fluxes over Europe simulated with the MEGAN–MOHYCAN model. Biogeosciences 15, 3673–3690. doi:10.5194/bg-15-3673-2018
Bedia, J., Herrera, S., Camia, A., Moreno, J. M., and Gutiérrez, J. M. (2014). Erratum to: Forest fire danger projections in the Mediterranean using ENSEMBLES regional climate change scenarios. Clim. Change 122, 343–344. doi:10.1007/s10584-014-1073-8
Bellouin, N., Quaas, J., Gryspeerdt, E., Kinne, S., Stier, P., Watson-Parris, D., et al. (2020). Bounding global aerosol radiative forcing of climate change. Rev. Geophys. 58, e2019RG000660. doi:10.1029/2019RG000660
Bollen, J. (2015). The value of air pollution co-benefits of climate policies: Analysis with a global sector-trade CGE model called WorldScan. Technol. Forecast. Soc. Change 90, 178–191. doi:10.1016/j.techfore.2014.10.008
Bond, T. C., Doherty, S. J., Fahey, D. W., Forster, P. M., Berntsen, T., DeAngelo, B. J., et al. (2013). Bounding the role of black carbon in the climate system: A scientific assessment. J. Geophys. Res. Atmos. 118, 5380–5552. doi:10.1002/jgrd.50171
Boucher, O., Randall, D., Artaxo, P., Bretherton, C., Feingold, G., Forster, P., et al. (2013). “Clouds and aerosols,” in Climate change 2013: The phys- ical science basis, contribution of working group I to the fifth assessment report of the intergovernmental panel on climate change. Editors T. F. Stocker, D. Qin, G.-K. Plattner, M. Tig- nor, S. K. Allen, J. Boschunget al. (Cambridge, United Kingdom and New York, NY, USA: Cambridge University Press), 571–657.
Bowman, D. M. J. S., Balch, J. K., Artaxo, P., Bond, W. J., Carlson, J. M., Cochrane, M. A., et al. (2009). Fire in the earth system. Science 324 (5926), 481–484. doi:10.1126/science.1163886
Carslaw, K. S., Boucher, O., Spracklen, D. V., Mann, G. W., Rae, J. G. L., Woodward, S., et al. (2010). A review of natural aerosol interactions and feedbacks within the Earth system. Atmos. Chem. Phys. 10 (4), 1701–1737. doi:10.5194/acp-10-1701-201010.5194/acp-10-1701-2010
Cheng, C. S., Campbell, M., Li, Q., Li, G., Auld, H., Day, N., et al. (2007). A synoptic climatological approach to assess climatic impact on air quality in south-central Canada. Part II: Future estimates. Water Air Soil Pollut. 182, 117–130. doi:10.1007/s11270-006-9326-4
Cheng, J. J., and Berry, P. (2013). Health co-benefits and risks of public health adaptation strategies to climate change: A review of current literature. Int. J. Public Health 58 (2), 305–311. doi:10.1007/s00038-012-0422-5
Cholakian, A., Colette, A., Coll, I., Ciarelli, G., and Beekmann, M. (2019). Future climatic drivers and their effect on PM<sub>10</sub> components in Europe and the Mediterranean Sea. Atmos. Chem. Phys. 19, 4459–4484. doi:10.5194/acp-19-4459-2019
Clifford, H. M., Spaulding, N. E., Kurbatov, A. V., More, A., Korotkikh, E. V., and Sneed, S. B. (2019). A 2000 year Saharan dust event proxy record from an ice core in the European Alps. J. Geophys. Res. Atmos. 124, 12,882–12,900. doi:10.1029/2019JD030725
Colette, A., Andersson, C., Baklanov, A., Bessagnet, B., Brandt, J., Christensen, J. H., et al. (2015). Is the ozone climate penalty robust in Europe? Environ. Res. Lett. 10, 084015. doi:10.1088/1748-9326/10/8/084015
Colette, A., Bessagnet, B., Vautard, R., Szopa, S., Rao, S., Schucht, S., et al. (2013). European atmosphere in 2050, a regional air quality and climate perspective under CMIP5 scenarios. Atmos. Chem. Phys. 13, 7451–7471. doi:10.5194/acp-13-7451-2013
Creutzig, F., Mühlhoff, R., and Römer, J. (2012). Decarbonizing urban transport in European cities: Four cases show possibly high co-benefits. Environ. Res. Lett. 7 (4), 044042. doi:10.1088/1748-9326/7/4/044042
D'Andrea, S. D., Acosta Navarro, J. C., Farina, S. C., Scott, C. E., Rap, A., Farmer, D. K., et al. (2015). Aerosol size distribution and radiative forcing response to anthropogenically driven historical changes in biogenic secondary organic aerosol formation. Atmos. Chem. Phys. 15, 2247–2268. doi:10.5194/acp-15-2247-2015
Daskalakis, N., Myriokefalitakis, S., and Kanakidou, M. (2015). Sensitivity of tropospheric loads and lifetimes of short lived pollutants to fire emissions. Atmos. Chem. Phys. 15, 3543–3563. doi:10.5194/acp-15-3543-2015
Dawson, J. P., Adams, P. J., and Pandis, S. N. (2007). Sensitivity of PM<sub>2.5</sub> to climate in the eastern US: A modeling case study. Atmos. Chem. Phys. 7, 4295–4309. doi:10.5194/acp-7-4295-2007
de Oliveira, J. A. P., and Doll, C. N. H. (2016). Governance and networks for health co-benefits of climate change mitigation: Lessons from two Indian cities. Environ. Int. 97, 146–154. doi:10.1016/j.envint.2016.08.020
Dijkstra, J., Durrant, T., San-Miguel-Ayanz, J., and Veraverbeke, S. (2022). Anthropogenic and lightning fire incidence and burned area in Europe. Land 11, 651. doi:10.3390/land11050651
Doherty, R. M., Heal, M. R., and O’Connor, F. M. (2017). Climate change impacts on human health over Europe through its effect on air quality. Environ. Health 16, 118. doi:10.1186/s12940-017-0325-2
Dupuy, J. L., Fargeon, H., Martin-StPaul, N., Pimont, F., Ruffault, J., Guijarro, M., et al. (2020). Climate change impact on future wildfire danger and activity in southern Europe: a review. J. Trop. For. Sci. 77, 35. doi:10.1007/s13595-020-00933-5
Dusek, U., Frank, G. P., Helas, G., Iinuma, Y., Zeromskiene, K., Gwaze, P., et al. (2005). ‘‘Missing’’ cloud condensation nuclei in peat smoke. Geophys. Res. Lett. 32, L11802. doi:10.1029/2005GL022473
EEA (2019a). European energy agency, energy and climate change. Available at: https://www.eea.europa.eu/signals/signals-2017/articles/energy-and-climate-change.
EEA (2019b). European Energy Agency, Total greenhouse gas emission trends and projections in Europe. EEA Report No 15/2019. Available at: https://www.eea.europa.eu//publications/trends-and-projections-in-europe-1.
Eyring, V., Stevenson, D. S., Lauer, A., Dentener, F. J., Butler, T., Collins, W. J., et al. (2007). Multi-model simulations of the impact of international shipping on Atmospheric Chemistry and Climate in 2000 and 2030. Atmos. Chem. Phys. 7, 757–780. doi:10.5194/acp-7-757-2007
Field, D. R., van der Werf, Guido R., Fanin, Thierry, Fetzer, Eric J., Fuller, Ryan, Jethva, Hiren, et al. (2015). Indonesian fire activity and smoke pollution in 2015 show persistent nonlinear sensitivity to El Niño-induced drought. Proc. Natl. Acad. Sci. U. S. A. 113, 9204–9209. doi:10.1073/pnas.1524888113
Fiore, A. M., Naik, V., Spracklen, D. V., Steiner, A., Unger, N., Prather, M., et al. (2012). Global air quality and climate. Chem. Soc. Rev. 41, 6663–6683. doi:10.1039/c2cs35095e
Fiore, M., Oliveri Conti, G., Caltabiano, R., Buffone, A., Zuccarello, P., Cormaci, L., et al. (2019). Role of emerging environmental risk factors in thyroid cancer: a brief review. Int. J. Environ. Res. Publ. Health 16, 1185. doi:10.3390/ijerph16071185
Fischer, A. P., Spies, T. A., Steelman, T. A., Moseley, C., Johnson, B. R., Bailey, J. D., et al. (2016). Wildfire risk as a socioecological pathology. Front. Ecol. Environ. 14, 276–284. doi:10.1002/fee.1283
Flanner, M. G. (2013). Arctic climate sensitivity to local black carbon. J. Geophys. Res. Atmos. 118, 1840–1851. doi:10.1002/jgrd.50176
Ford, B., Val Martin, M., Zelasky, S. E., Fischer, E. V., Anenberg, S. C., Heald, C. L., et al. (2018). Future fire impacts on smoke concentrations, visibility, and health in the contiguous united statesfire impacts on smoke concentrations, visibility, and health in the contiguous United States. GeoHealth 2, 229–247. doi:10.1029/2018GH000144
Fortems-Cheiney, A., Foret, G., Siour, G., Vautard, R., Szopa, S., Dufour, G., et al. (2019). A 3 °C global RCP8.5 emission trajectory cancels benefits of European emission reductions on air quality. Nat. Commun. 8, 89. doi:10.1038/s41467-017-00075-9
Fowler, D., Steadman, C. E., Stevenson, D., Coyle, M., Rees, R. M., Skiba, U. M., et al. (2015). Effects of global change during the 21st century on the nitrogen cycle. Atmos. Chem. Phys. 15 (24), 13849–13893. doi:10.5194/acp-15-13849-2015
Fu, T. M., and Tian, H. (2019). Climate change penalty to ozone air quality: Review of current understandings and knowledge gaps. Curr. Pollut. Rep. 5, 159–171. doi:10.1007/s40726-019-00115-6
Fuglestvedt, J., Berntsen, T., Myhre, G., Rypdal, K., and Skeie, R. B. (2008). Climate forcing from the transport sectors. Proc. Natl. Acad. Sci. U. S. A. 105, 454–458. doi:10.1073/pnas.0702958104
Fusco, E. J., Abatzoglou Balch, J. K., Finn, J. T., and Bradley, B. A. (2016). Quantifying the human influence on fire ignition across the Western USA fluence on fire ignition across the Western USA. Ecol. Appl. 26 (8), 2390–2401. doi:10.1002/eap.1395
Ganteaume, A., Camia, A., Jappiot, M., San-Miguel-Ayanz, J., Long-Fournel, M., and Lampin, C. (2013). A review of the main driving factors of forest fire ignition over Europe. Environ. Manage. 51, 651–662. doi:10.1007/s00267-012-9961-z
Gatebe, C. K., Ichoku, C. M., Poudyal, R., Román, M. O., and Wilcox, E. (2014). Surface albedo darkening from wildfires in northern sub-Saharan Africafires in northern sub-Saharan Africa. Environ. Res. Lett. 9, 065003. doi:10.1088/1748-9326/9/6/065003
Geels, C., Andersson, C., Hänninen, O., Lansø, A. S., Schwarze, P., Ambelas Skjøth, C., et al. (2015). Future premature mortality due to O3, secondary inorganic aerosols and primary PM in Europe — sensitivity to changes in climate, anthropogenic emissions, population and building stock. Int. J. Environ. Res. Public Health 12, 2837–2869. doi:10.3390/ijerph120302837
Glotfelty, T., Zhang, Y., Karamchandani, P., and Streets, D. (2016). Changes in future air quality, deposition, and aerosol-cloud interactions under future climate and emission scenarios. Atmos. Environ. 139, 176–191. doi:10.1016/j.atmosenv.2016.05.008
Grote, R. (2019). Environmental impacts on biogenic emissions of volatile organic compounds (VOCs). Karlsruher Institut für Technologie, Garmisch-Partenkirchen, Report No. (UBA-FB) 002772/ENG.
Guenther, A., Karl, T., Harley, P., Wiedinmyer, C., Palmer, P. I., and Geron, C. (2006). Estimates of global terrestrial isoprene emissions using MEGAN (model of emissions of gases and aerosols from nature), atmos. Chem. Phys. 6, 3181–3210. doi:10.5194/acp-6-3181-2006
Hantson, S., Kelley, D. I., Arneth, A., Harrison, S. P., Archibald, S., Bachelet, D., et al. (2020). Quantitative assessment of fire and vegetation properties in historical simulations with fire-enabled vegetation models from the Fire Model Intercomparison Project. Geosci. Model. Dev. Discuss. in review. doi:10.5194/gmd-2019-261
Hantson, S., Knorr, W., Schurgers, G., Pugh, T. A. M., and Arneth, A. (2017). Global isoprene and monoterpene emissions under changing climate, vegetation, CO2 and land use. Atmos. Environ. 155, 35–45. doi:10.1016/j.atmosenv.2017.02.010
Hedegaard, G. B., Brandt, J., Christensen, J. H., Frohn, L. M., Geels, C., Hansen, K. M., et al. (2008). Impacts of climate change on air pollution levels in the Northern Hemisphere with special focus on Europe and the Arctic. Atmos. Chem. Phys. 8, 3337–3367. doi:10.5194/acp-8-3337-2008
Hodnebrog, Ø., Solberg, S., Stordal, F., Svendby, T. M., Simpson, D., Gauss, M., et al. (2012). Impact of forest fires, biogenic emissions and high temperatures on the elevated Eastern Mediterranean ozone levels during the hot summer of 2007. Atmos. Chem. Phys. 12 (18), 8727–8750. doi:10.5194/acp-12-8727-2012
Hoegh-Guldberg, O., Jacob, D., Taylor, M., Bindi, M., Brown, S., Camilloni, I., et al. (2018). “Impacts of 1.5°C global warming on natural and human systems,” in Global Warming of 1.5°C. An IPCC Special Report on the impacts of global warming of 1.5°C above pre-industrial levels and related global greenhouse gas emission pathways, in the context of strengthening the global response to the threat of climate change, sustainable development, and efforts to eradicate poverty [Masson-Delmotte, V., P. Zhai, H.-O. Pörtner, D. Roberts, J. Skea, P.R. Shukla, A. Pirani, W. Moufouma-Okia, C. Péan, R. Pidcock, S. Connors, J.B.R. Matthews, Y. Chen, X. Zhou, M.I. Gomis, E. Lonnoy, T. Maycock, M. Tignor, and T. Waterfield (eds.)] (Cambridge, UK and New York, NY, USA: Cambridge University Press), 175–312. doi:10.1017/9781009157940.005
Im, U., Markakis, K., Koçak, M., Gerasopoulos, E., Daskalakis, N., Mihalopoulos, N., et al. (2012). Summertime aerosol chemical composition in the eastern mediterranean and its sensitivity to temperature: A modeling case study. Atmos. Environ. 50, 164–173.
Im, U., Markakis, K., Poupkou, A., Melas, D., Unal, A., Gerasopoulos, E., et al. (2011). The impact of temperature changes on summer time ozone and its precursors in the Eastern Mediterranean. Atmos. Chem. Phys. 11, 3847–3864. doi:10.5194/acp-11-3847-2011
Im, U., Tayanç, M., and Yenigun, O. (2006). Analysis of major photochemical pollutants with meteorological factors for high ozone days in Istanbul, Turkey. Water Air Soil Pollut. 175, 335–359. doi:10.1007/s11270-006-9142-x
Im, U., Tayanç, M., and Yenigun, O. (2008). Interaction patterns of major photochemical pollutants in Istanbul, Turkey. Atmos. Res. 89, 382–390. doi:10.1016/j.atmosres.2008.03.015
IPCC (2013). “Climate change 2013: The physical science basis,” in Contribution of working group I to the fifth assessment report of the intergovernmental panel on climate change [stocker, T.F., D. Qin, G.-K. Plattner, M. Tignor, S.K. Allen, J. Boschung, A. Nauels, Y. Xia, V. Bex and P.M. Midgley (eds.)] (Cambridge, United Kingdom and New York, NY, USA: Cambridge University Press), 1535.
IPCC (2022). “Climate change 2022: Impacts, adaptation, and vulnerability,” in Contribution of working group II to the Sixth assessment report of the intergovernmental panel on climate change [H.-O. Pörtner, D.C. Roberts, M. Tignor, E.S. Poloczanska, K. Mintenbeck, A. Alegría, M. Craig, S. Langsdorf, S. Löschke, V. Möller, A. Okem, B. Rama (eds.)] (Cambridge: Cambridge University Press). In Press.
Isaksen, I. S. A., Granier, C., Myhre, G., Berntsen, T. K., Dalsøren, S. B., Gauss, M., et al. (2009). Atmospheric composition change: Climate–Chemistry interactions. Atmos. Environ. 43, 5138–5192. doi:10.1016/j.atmosenv.2009.08.003
Jacob, D. J., and Winner, D. A. (2009). Effect of climate change on air quality. Atmos. Environ. 43 (1), 51–63. doi:10.1016/j.atmosenv.2008.09.051
Jacobson, M. Z., and Streets, D. G. (2009). Influence of future anthropogenic emissions on climate, natural emissions, and air quality. J. Geophys. Res. 114, D08118. doi:10.1029/2008JD011476
Jiang, X., Guenthera, A., Potosnak, M., Geron, C., Seco, R., Karl, T., et al. (2018). Isoprene emission response to drought and the impact on global atmospheric chemistry. Atmos. Environ. X. 183, 69–83. doi:10.1016/j.atmosenv.2018.01.026
Johnson, A. L., Abramson, M. J., Dennekamp, M., Williamson, G. J., and Guo, Y. (2020). Particulate matter modelling techniques for epidemiological studies of open biomass fire smoke exposure: A review. Air Qual. Atmos. Health 13, 35–75. doi:10.1007/s11869-019-00771-z
Jones, A., Haywood, J. M., and Boucher, O. (2007). Aerosol forcing, climate response and climate sensitivity in the Hadley Centre climate model. J. Geophys. Res. 112, D20211. doi:10.1029/2007JD008688
Joshi, M. M., Gregory, J. M., Webb, M. J., David, M. H. S., and Johns, T. C. (2007). Mechanisms for the land/sea warming contrast exhibited by simulations of climate change. Clim. Dyn. 30 (5), 455–465. doi:10.1007/s00382-007-0306-1
Juda-Rezler, K., Reizer, M., Huszar, P., Krüger, B. C., Zanis, P., Syrakov, D., et al. (2012). Modelling the effects of climate change on air quality over central and eastern Europe: Concept, evaluation and projections. Clim. Res. 53, 179–203. doi:10.3354/cr01072
Jylhä, K., Ruosteenoja, K., Räisänen, J., Venäläinen, A., Tuomenvirta, H., Ruokolainen, L., et al. (2009). The changing climate in Finland: Estimates for adaptation studies (in Finnish with English abstract, extended abstract and captions for figures and tables). ACCLIM project report, Report 4. Helsinki: Finnish Meteorological Institute.
Kalabokidis, K., Palaiologou, P., Kostopoulou, E., Zerefos, C. S., Gerasopoulos, E., and Giannakopoulos, C. (2014). “Effect of climate projections on the behavior and impacts of wildfires in Messenia, Greece,” in COMECAP 2014 e-book of proceedings ISBN: 978-960-524-430-9 vol 1, 395–400.
Kanakidou, M., Seinfeld, J. H., Pandis, S. N., Barnes, I., Dentener, F. J., Facchini, M. C., et al. (2005). Organic aerosol and global climate modelling: A review. Atmos. Chem. Phys. 5, 1053–1123. doi:10.5194/acp-5-1053-2005
Karlsson, M., Alfredsson, E., and Westling, N. (2020). Climate policy co-benefits: A review. Clim. Policy 20, 292–316. doi:10.1080/14693062.2020.1724070
Keywood, M., Kanakidou, M., Stohl, A., Dentener, F., Grassi, G., Meyer, J. P., et al. (2013). Fire in the Air: Biomass burning impacts in a changing climate. Crit. Rev. Environ. Sci. Technol. 43, 40–83. doi:10.1080/10643389.2011.604248
Klausbruckner, C., Annegar, H., Henneman, L. R. F., and Rafaj, P. (2016). A policy review of synergies and trade-offs in South African climate change mitigation and air pollution control strategies. Environ. Sci. Policy 57, 70–78. doi:10.1016/j.envsci.2015.12.001
Kleeman, M. J. (2007). A preliminary assessment of the sensitivity of air quality in California to global change. Clim. Change 87, S273–S292. doi:10.1007/s10584-007-9351-3
Klingmüller, K., Lelieveld, J., Karydis, V. A., and Stenchikov, G. L. (2019). Direct radiative effect of dust–pollution interactions. Atmos. Chem. Phys. 19, 7397–7408. doi:10.5194/acp-19-7397-2019
Knorr, W., Dentener, F., Lamarque, J.-F., Jiang, L., and Arneth, A. (2017). Wildfire air pollution hazard during the 21st centuryfire air pollution hazard during the 21st century. Atmos. Chem. Phys. 17, 9223–9236. doi:10.5194/acp-17-9223-2017
Knorr, W., Jiang, W., and Arneth, A. (2016). Climate, CO<sub>2</sub> and human population impacts on global wildfire emissions. Biogeosciences 13, 267–282. doi:10.5194/bg-13-267-2016
Koch, D., Park, J., and Del Genio, A. (2003). Clouds and sulfate are anticorrelated: A new diagnostic for global sulfur models. J. Geophys. Res. 108, D244781. doi:10.1029/2003JD003621
Kollanus, V., Prank, M., Gens, A., Soares, J., Vira, J., Kukkonen, J., et al. (2017). Mortality due to vegetation-fire originated PM2.5 exposure in Europe – assessment for the years 2005 and 2008. Environ. Health Perspect. 125 (1), 30–37. doi:10.1289/EHP194
Kukkonen, J., López-Aparicio, S., Segersson, D., Geels, C., Kangas, L., Kauhaniemi, M., et al. (2020). The influence of residential wood combustion on the concentrations of PM<sub>2.5</sub> in four Nordic cities. Atmos. Chem. Phys. 20, 4333–4365. doi:10.5194/acp-20-4333-2020
Kukkonen, J., Nikmo, J., Sofiev, M., Riikonen, K., Petäjä, T., Virkkula, A., et al. (2014). Applicability of an integrated plume rise model for the dispersion from wild-land fires. Geosci. Model. Dev. 7, 2663–2681. doi:10.5194/gmd-7-2663-2014
Kukkonen, J., Olsson, T., Schultz, D. M., Baklanov, A., Klein, T., Miranda, A. I., et al. (2012). A review of operational, regional-scale, chemical weather forecasting models in Europe. Atmos. Chem. Phys. 12, 1–87. doi:10.5194/acp-12-1-2012
Lacressonniere, G., Foret, G., Beekmann, M., Siour, G., Engardt, M., Gauss, M., et al. (2016). Impacts of regional climate change on air quality projections and associated uncertainties. Clim. Change 136, 309–324. doi:10.1007/s10584-016-1619-z
Lacressonnière, G., Peuch, V.-H., Vautard, R., Arteta, J., Déqué, M., Joly, M., et al. (2014). European air quality in the 2030s and 2050s: Impacts of global and regional emission trends and of climate change. Atmos. Environ. 92, 348–358. doi:10.1016/j.atmosenv.2014.04.033
Lacressonniere, G., Watson, L., Gauss, M., Engardt, M., Andersson, C., Beekmann, M., et al. (2017). Particulate matter air pollution in Europe in a +2ºC warming world. Atmos. Environ. 154, 129–140. doi:10.1016/j.atmosenv.2017.01.037
Lamarque, J. F., Kyle, G. P., Meinshausen, M., Riahi, K., Smith, S. J., van Vuuren, D. P., et al. (2011). Global and regional evolution of short-lived radiatively-active gases and aerosols in the Representative Concentration Pathways. Clim. Change 109 (1-2), 191–212. doi:10.1007/s10584-011-0155-0
Langmann, B., Duncan, B., Textor, C., Trentmann, J., and van der Werf, G. R. (2009). Vegetation fire emissions and their impact on air pollution and climate. Atmos. Environ. 43 (1), 107–116. doi:10.1016/j.atmosenv.2008.09.047
Langner, J., Engardt, M., Baklanov, A., Christensen, J. H., Gauss, M., Geels, C., et al. (2012). A multi-model study of impacts of climate change on surface ozone in Europe. Atmos. Chem. Phys. 12, 10423–10440. doi:10.5194/acp-12-10423-2012
Lee, L. A., Reddington, C. L., and Carslaw, K. S. (2016). On the relationship between aerosol model uncertainty and radiative forcing uncertainty. Proc. Natl. Acad. Sci. U. S. A. 113 (21), 5820–5827. doi:10.1073/pnas.1507050113
Lemaire, V. E. P., Colette, A., and Menut, L. (2016). Using statistical models to explore ensemble uncertainty in climate impact studies: The example of air pollution in Europe. Atmos. Chem. Phys. 16, 2559–2574. doi:10.5194/acp-16-2559-2016
Liao, H., Zhang, Y., Chen, W. T., Raes, F., and Seinfeld, J. H. (2009). Effect of chemistry-aerosol-climate coupling on predictions of future climate and future levels of tropospheric ozone and aerosols. J. Geophys. Res. 114, D10306. doi:10.1029/2008JD010984
Lin, C. Y. C., Mickley, L. J., Hayhoe, K., Maurer, E. P., and Hogrefe, C. (2007). Rapid calculation of future trends in ozone exceedances over the northeast United States : Results from three models and two scenarios, presented at the consequences of global change for air quality festival. Research Triangle Park, NC: EPA. February 20–21.
Liu, J. C., Mickley, L. J., Sulprizio, M. P., Dominici, F., Yue, X., Ebisu, K., et al. (2016). Particulate air pollution from wildfires in the Western US under climate changefires in the Western US under climate change. Clim. Change 138 (3-4), 655–666. doi:10.1007/s10584-016-1762-6
Liu, S. C., Trainer, M., Fehsenfeld, F. C., Parrish, D. D., Williams, E. J., Fahey, D. W., et al. (1987). Ozone production in the rural troposphere and the implications for regional and global ozone distributions. J. Geophys. Res. 92, 4191–4207. doi:10.1029/jd092id04p04191
Lozano, O. M., Salis, M., Ager, A. A., Arca, B., Alcasena, F. J., Monteiro, A. T., et al. (2017). Assessing climate change impacts on wildfire exposure in mediterranean areas. Risk Anal. 37, 1898–1916. doi:10.1111/risa.12739
Luderer, G., Trentmann, J., Winterrath, T., Textor, C., Herzog, M., Graf, H. F., et al. (2006). Modeling of biomass smoke injection into the lower stratosphere by a large forest fire (Part II): Sensitivity studies. Atmos. Chem. Phys. 6 (4), 5261–5277. doi:10.5194/acpd-6-6081-2006
Lund, M. T., Eyring, V., Fuglestvedt, J., Hendricks, J., Lauer, A., Lee, D., et al. (2012). Global-mean temperature change from shipping toward 2050: Improved representation of the indirect aerosol effect in simple climate models. Environ. Sci. Technol. 46, 8868–8877. doi:10.1021/es301166e
Luo, L., Tang, Y., Zhong, S., Bian, X., and Heilman, W. E. (2013). Will future climate favor more erratic wildfires in the Western United States? J. Appl. Meteorol. Climatol. 52 (11), 2410–2417. doi:10.1175/JAMC-D-12-0317.1
Mahowald, N. M., Muhs, D. R., Levis, S., Rasch, P. J., Yoshioka, M., Zender, C. S., et al. (2006). Change in atmospheric mineral aerosols in response to climate: Last glacial period, preindustrial, modern, and doubled carbon dioxide climates. J. Geophys. Res. 111, D10202. doi:10.1029/2005JD006653
Malavelle, F., Haywood, J., Jones, A., Gettelman, A., Clarisse, L., Bauduin, S., et al. (2017). Strong constraints on aerosol–cloud interactions from volcanic eruptions. Nature 546, 485–491. doi:10.1038/nature22974
Manders, A. M. M., van Meijgaard, E., Mues, A. C., Kranenburg, R., van Ulft, L. H., and Schaap, M. (2012). The impact of differences in large-scale circulation output from climate models on the regional modeling of ozone and PM. Atmos. Chem. Phys. 12, 9441–9458. doi:10.5194/acp-12-9441-2012
Markandya, A., Sampedro, J., Smith, S. J., Van Dingenen, R., Pizarro-Irizar, C., Arto, I., et al. (2018). Health co-benefits from air pollution and mitigation costs of the paris agreement: A modelling study. Lancet Planet. Health 2, 126–133. doi:10.1016/S2542-5196(18)30029-9
Mårtensson, E. M., Nilsson, E. D., de Leeuw, G., Cohen, L. H., and Hansson, H.-C. (2003). Laboratory simulations and parameterization of the primary marine aerosol production. J. Geophys. Res. 108, 4297. doi:10.1029/2002JD002263
McCarty, J. L., Aalto, J., Paunu, V.-V., Arnold, S. R., Eckhardt, S., Klimont, Z., et al. (2021). Reviews and syntheses: Arctic fire regimes and emissions in the 21st century. Biogeosciences 18, 5053–5083. doi:10.5194/bg-18-5053-2021
Megaritis, A. G., Fountoukis, C., Charalampidis, P. E., Denier van der Gon, H. A. C., Pilinis, C., and Pandis, S. N. (2014). Linking climate and air quality over Europe: Effects of meteorology on PM<sub>2.5</sub> concentrations. Atmos. Chem. Phys. 14, 10283–10298. doi:10.5194/acp-14-10283-2014
Monahan, E. C., Spiel, D. E., and Davidson, K. L. (1986). “A model of marine aerosol generation via whitecaps and wave disruption,” in Oceanic whitecaps. Editors E. C. Monahan, and G. Mac-Niochaill (Norwell, USA: D. Reidel), 167–193.
Mouillot, F., Rambal, S., and Joffre, R. (2002). Simulating climate change impacts on fire frequency and vegetation dynamics in a Mediterranean-type ecosystem. Glob. Chang. Biol. 8, 423–437. doi:10.1046/j.1365-2486.2002.00494.x
Mülmenstädt, J., and Feingold, G. (2018). The radiative forcing of aerosol–cloud interactions in liquid clouds: Wrestling and embracing uncertainty. Curr. Clim. Change Rep. 4, 23–40. doi:10.1007/s40641-018-0089-y
Myhre, G., Shindell, D., Breon, F.-M., Collins, W., Fuglestvedt, J., Huang, J., et al. (2013). “Anthropogenic and natural radiative forcing,” in Climate change 2013: The physical science basis. Contribution of working group I to the fifth assessment report of the inter- governmental panel on climate change. Editors T. F. Stocker, D. Qin, G.-K. Plattner, M. Tignor, S. K. Allen, J. Boschunget al. (Cambridge, United Kingdom and New York, NY, USA: Cambridge University Press), 659–740.
Nilsson, E. D., Mårtensson, E. M., Van Ekeren, J. S., de Leeuw, G., Moerman, M., and O’Dowd, C. (2007). Primary marine aerosol emissions: Size resolved eddy covariance measurements with estimates of the sea salt and organic carbon fractions. Atmos. Chem. Phys. 7, 13345–13400. doi:10.5194/acpd-7-13345-2007
Otero, N. F., Sillmann, J., Schnell, J. L., Rust, H. W., and Butler, T. (2016). Synoptic and meteorological drivers of extreme ozone concentrations over Europe. Environ. Res. Lett. 11, 024005. doi:10.1088/1748-9326/11/2/024005
Otero, N., Sillmann, J., and Butler, T. (2018). Assessment of an extended version of the Jenkinson–Collison classification on CMIP5 models over Europe. Clim. Dyn. 50, 1559–1579. doi:10.1007/s00382-017-3705-y
Parente, J., Pereira, M. G., Amraoui, M., and Tedim, F. (2018). Negligent and intentional fires in Portugal: Spatial distribution characterization. Sci. Total Environ. 624, 424–437. doi:10.1016/j.scitotenv.2017.12.013
Park, S., Allen, R. J., and Lim, C. H. (2020). A likely increase in fine particulate matter and premature mortality under future climate change. Air Qual. Atmos. Health 13, 143–151. doi:10.1007/s11869-019-00785-7
Pechony, O., and Shindell, D. T. (2010). Driving forces of global wildfires over the past millennium and the forthcoming century. Proc. Natl. Acad. Sci. U. S. A. 107 (45), 19167–19170. doi:10.1073/pnas.1003669107
Pegoraro, E., Rey, A., Greenberg, J., Harley, P., Grace, J., Malhi, Y., et al. (2004). Effect of drought on isoprene emission rates from leaves of Quercus virginiana Mill. Atmos. Environ. X. 38, 6149–6156. doi:10.1016/j.atmosenv.2004.07.028
Pfahl, S., and Wernli, H. (2012). Quantifying the relevance of atmospheric blocking for co-located temperature extremes in the Northern Hemisphere on (sub-) daily time scales. Geophys. Res. Lett. 39, L12807. doi:10.1029/2012GL052261
Prestemon, J. P., Hawbaker, T. J., Bowden, M., Carpenter, J., Scranton, S., Brooks, M. T., et al. (2013). Wild fire ignitions: A review of the science and recommendations for empirical modeling (general technical report No. SRS-171). Asheville, NC: USDA Forest Service, Southern Research Station.
Rafaj, P., Cofala, J., Kuenen, J., Wyrwa, A., and Zyśk, J. (2014). Benefits of European climate policies for mercury air pollution. Atmosphere 5, 45–59. doi:10.3390/atmos5010045
Rao, S., Klimont, Z., Smith, S. J., Van Dingenen, R., Dentener, F., Bouwman, L., et al. (2017). Future air pollution in the shared socio-economic pathways. Glob. Environ. Change 42, 346–358. doi:10.1016/j.gloenvcha.2016.05.012
Ruosteenoja, K., and Jylha, K. (2021). Projected climate change in Finland during the 21st century calculated from CMIP6 model simulations. Geophysica 56 (1), 39–69.
Russo, S., Dosio, A., Graverson, R. G., Sillmann, J., Carrao, H., Bunbar, M. B., et al. (2014). Magnitude of extreme heat waves in present climate and their projection in a warming world. J. Geophys. Res. Atmos. 119, 12500–12512. doi:10.1002/2014JD022098
Saarikoski, S., Sillanpää, M., Sofiev, M., Timonen, H., Saarnio, K., Teinilä, K., et al. (2007). Chemical composition of aerosols during a major biomass burning episode over northern Europe in spring 2006: Experimental and modelling assessments. Atmos. Environ. 41, 3577–3589. doi:10.1016/j.atmosenv.2006.12.053
Saarnio, K., Aurela, M., Timonen, H., Saarikoski, S., Teinilä, K., Mäkelä, T., et al. (2010). Chemical composition of fine particles in fresh smoke plumes from boreal wild-land fires in Europe. Sci. Total Environ. 408, 2527–2542. doi:10.1016/j.scitotenv.2010.03.010
Samset, B. H., Myhre, G., Schulz, M., Balkanski, Y., Bauer, S., Berntsen, T. K., et al. (2013). Black carbon vertical profiles strongly affect its radiative forcing uncertainty. Atmos. Chem. Phys. 13 (5), 2423–2434. doi:10.5194/acp-13-2423-2013
Sanderson, B. M., and Fisher, R. A. (2020). A fiery wake-up call for climate science. Nat. Clim. Chang. 10, 175–177. doi:10.1038/s41558-020-0707-2
Schaller, N., Sillmann, J., Anstey, J., Fischer, E. M., Grams, C. M., and Russo, S. (2018). Influence of blocking on Northern European and Western Russian heatwaves in large climate model ensembles. Environ. Res. Lett. 13, 054015. doi:10.1088/1748-9326/aaba55
Schnell, J. L., Prather, M. J., Josse, B., Naik, V., Horowitz, L. W., Zeng, G., et al. (2016). Effect of climate change on surface ozone over North America, Europe, and east Asia. Geophys. Res. Lett. 43 (7), 3509–3518. doi:10.1002/2016GL068060
Seinfeld, J. H., and Pandis, S. N. (2016). Atmospheric chemistry and physics: From air pollution to climate change. 3rd Edition, 1152. ISBN: 978-1-118-94740-1.
Sheehan, P. E., and Bowman, F. M. (2001). Estimated effects of temperature on secondary organic aerosol concentrations. Environ. Sci. Technol. 35 (11), 2129–2135. doi:10.1021/es001547g
Shindell, D., and Faluvegi, G. (2009). Climate response to regional radiative forcing during the twentieth century. Nat. Geosci. 2, 294–300. doi:10.1038/ngeo473
Shindell, D., Kuylenstierna, J. C. I., Vignati, E., van Dingenen, R., Amann, M., Klimont, Z., et al. (2012). Simultaneously mitigating near-term climate change and improving human health and food security. Science 335 (6065), 183–189. doi:10.1126/science.1210026
Shindell, D. T., Lamarque, J.-F., Schulz, M., Flanner, M., Jiao, C., Chin, M., et al. (2013). Radiative forcing in the ACCMIP historical and future climate simulations. Atmos. Chem. Phys. 13 (6), 2939–2974. doi:10.5194/acp-13-2939-2013
Silva, R. A., West, J. J., Lamarque, J.-F., Shindell, D. T., Collins, W. J., Faluvegi, G., et al. (2017). Future global mortality from changes in air pollution attributable to climate change. Nat. Clim. Chang. 7 (9), 647–651. doi:10.1038/nclimate3354
Silva, R. A., West, J. J., Zhang, Y., Anenberg, S. C., Lamarque, J.-F., Shindell, D. T., et al. (2013). Global premature mortality due to anthropogenic outdoor air pollution and the contribution of past climate change. Environ. Res. Lett. 8 (3), 034005. doi:10.1088/1748-9326/8/3/034005
Skeie, R. B., Fuglestvedt, J., Berntsen, T., Lund, M. T., Myhre, G., and Rypdal, K. (2009). Global temperature change from the transport sectors: Historical development and future scenarios. Atmos. Environ. 43, 6260–6270. doi:10.1016/j.atmosenv.2009.05.025
Skjøth, C. A., and Geels, C. (2013). The effect of climate and climate change on ammonia emissions in Europe. Atmos. Chem. Phys. 13, 117–128. doi:10.5194/acp-13-117-2013
Soares, J., Sofiev, M., Geels, C., Christensen, J. H., Andersson, C., Tsyro, S., et al. (2016). Impact of climate change on the production and transport of sea salt aerosol on European seas. Atmos. Chem. Phys. 16, 13081–13104. doi:10.5194/acp-16-13081-2016
Sofiev, M., Ermakova, T., and Vankevich, R. (2012). Evaluation of the smoke injection height from wild-land fires using remote sensing data. Atmos. Chem. Phys. 12, 1995–2006. doi:10.5194/acp-12-1995-2012
Sofiev, M., Lanne, M., Vankevich, R., Prank, M., Karppinen, A., and Kukkonen, J. (2008). “Impact of wild-land fires on European air quality in 2006-2008,” in 01/2008; edition: WIT transactions on ecology and the environment. Editors J. D. L. Heras, C. A. Brebbia, D. Viegas, and V. Leone (Southampton, UK: WIT Press), Vol. 119, 353–361. ISBN: 978-1-84564-141-2. doi:10.2495/FIVA080351
Sofiev, M., Vankevich, R., Ermakova, T., and Hakkarainen, J. (2013). Global mapping of maximum emission heights and resulting vertical profiles of wildfire emissions. Atmos. Chem. Phys. 13, 7039–7052. doi:10.5194/acp-13-7039-2013
Sofiev, M. (2013). “Wildland fires: Monitoring, plume modelling, impact on atmospheric composition and climate. Chapter 21 in matyssek, R., clarke, N., cudlin, P., mikkelsen, T.N., tuovinen, J.-P. Wieser, G., paoletti, E. Climate change, air pollution and global challenges,” in Developments in environmental science (London: Elsevier & Book Aid Intern.), Vol. 13, 451–474. ISBN: 978-0-08-098349-3 ISSN: 1474-8177.
Sofiev, M., Winebrake, J. J., Johansson, L., Carr, E. W., Prank, M., Soares, J., et al. (2018). Cleaner fuels for ships provide public health benefits with climate tradeoffsfits with climate tradeoffs. Nat. Commun. 9, 406. doi:10.1038/s41467-017-02774-9
Sokhi, R. S., Moussiopoulos, N., Baklanov, A., Bartzis, J., Coll, I., Finardi, S., et al. (2022). Advances in air quality research – current and emerging challenges. Atmos. Chem. Phys. 22, 4615–4703. doi:10.5194/acp-22-4615-2022
Stohl, A., Aamaas, B., Amann, M., Baker, L. H., Bellouin, N., Berntsen, T. K., et al. (2015). Evaluating the climate and air quality impacts of short-lived pollutants. Atmos. Chem. Phys. 15, 10529–10566. doi:10.5194/acp-15-10529-2015
Streets, D. G., Bond, T. C., Lee, T., and Jang, C. (2004). On the future of carbonaceous aerosol emissions. J. Geophys. Res. 109, D24212. doi:10.1029/2004JD004902
Sutton, M. A., Reis, S., Riddick, S. N., Dragosits, U., Nemitz, E., Theobald, M. R., et al. (2013). Towards a climate-dependent paradigm of ammonia emission and deposition. Phil. Trans. R. Soc. B 368, 20130166. doi:10.1098/rstb.2013.0166
Tegen, I., Werner, M., Harrison, S. P., and Kohfeld, K. E. (2004). Relative importance of climate and land use in determining present and future global soil dust emission. Geophys. Res. Lett. 31, L05105. doi:10.1029/2003GL019216
Tobollik, M., Keuken, M., Sabel, C., Cowie, H., Tuomisto, J., Sarigiannis, D., et al. (2016). Health impact assessment of transport policies in Rotterdam: Decrease of total traffic and increase of electric car use. Environ. Res. 146, 350–358. doi:10.1016/j.envres.2016.01.014
Toll, V., Christensen, M., Quaas, J., and Bellouin, N. (2019). Weak average liquid-cloud-water response to anthropogenic aerosols. Nature 572, 51–55. doi:10.1038/s41586-019-1423-9
Tosca, M. G., Randerson, J. T., and Zender, C. S. (2013). Global impact of smoke aerosols from landscape fires on climate and the Hadley circulation. Atmos. Chem. Phys. 13 (10), 5227–5241. doi:10.5194/acp-13-5227-2013
Tsigaridis, K., and Kanakidou, M. (2007). Secondary organic aerosol importance in the future atmosphere. Atmos. Environ. 41, 4682–4692. doi:10.1016/j.atmosenv.2007.03.045
Turnock, S. T., Allen, R. J., Andrews, M., Bauer, S. E., Deushi, M., Emmons, L., et al. (2020). Historical and future changes in air pollutants from CMIP6 models. Atmos. Chem. Phys. 20, 14547–14579. doi:10.5194/acp-20-14547-2020
Veira, A., Kloster, S., Schutgens, N. A. J., and Kaiser, J. W. (2015b). Fire emission heights in the climate system—Part 2: Impact on transport, black carbon concentrations and radiation. Atmos. Chem. Phys. 15 (13), 7173–7193. doi:10.5194/acp-15-7173-2015
Veira, A., Kloster, S., Wilkenskjeld, S., and Remy, S. (2015a). Fire emission heights in the climate system—Part 1: Global plume height patterns simulated by ECHAM6-HAM2. Atmos. Chem. Phys. 15 (13), 7155–7171. doi:10.5194/acp-15-7155-2015
Veira, A., Lasslop, G., and Kloster, S. (2016). Wildfires in a warmer climate: Emission fluxes, emission heights, and black carbon concentrations in 2090–2099. J. Geophys. Res. Atmos. 121 (7), 3195–3223. doi:10.1002/2015JD024142
Venäläinen, A., Lehtonen, I., Laapas, M., Ruosteenoja, K., Tikkanen, O.-P., Viiri, H., et al. (2020). Climate change induces multiple risks to boreal forests and forestry in Finland: A literature review. Glob. Change Biol. 26 (8), 4178–4196. doi:10.1111/gcb.15183
von Schneidemesser, E., Monks, P., Allan, J., Bruhwiler, L., Forster, P., Fowler, D., et al. (2015). Chemistry and the linkages between air quality and climate change. Chem. Rev. 115, 3856–3897. doi:10.1021/acs.chemrev.5b00089
Watson, L., Lacressonniere, G., Gauss, M., Engardt, M., Andersson, C., Josse, B., et al. (2016). Impact of emissions and +2°C climate change upon future ozone and nitrogen dioxide over Europe. Atmos. Environ. 142, 271–285. doi:10.1016/j.atmosenv.2016.07.051
Watts, N., Amann, M., Arnell, N., Ayeb-Karlsson, S., Belesova, K., Boykoff, M., et al. (2019). The 2019 report of the lancet countdown on health and climate change: Ensuring that the health of a child born today is not defined by a changing climate. Lancet 394 (10211), 1836–1878. doi:10.1016/S0140-6736(19)32596-6
West, J. J., Smith, S. J., Silva, R. A., Naik, N., Zhang, Y., Adelman, Z., et al. (2013). Co-benefits of mitigating global greenhouse gas emissions for future air quality and human health. Nat. Clim. Chang. 3 (10), 885–889. doi:10.1038/NCLIMATE2009
Westervelt, D. M., Horowitz, L. W., Naik, V., Tai, A. P. K., Fiore, A. M., and Mauzerall, D. L. (2016). Quantifying PM2.5-meteorology sensitivities in a global climate model. Atmos. Environ. 142, 43–56. doi:10.1016/j.atmosenv.2016.07.040
Wilkinson, P., Smith, K. R., Davies, M., Adair, H., Armstrong, B. G., Barrett, M., et al. (2009). Public health benefits of strategies to reduce greenhouse-gas emissions: household energy. Lancet 374 (9705), 1917–1929. doi:10.1016/S0140-6736(09)61713-X
Williams, M. L. (2007). UK air quality in 2050-synergies with climate change policies. Environ. Sci. Policy 10, 169–175.
Woodward, S., Roberts, D. L., and Betts, R. A. (2005). A simulation of the effect of climate change-induced desertification on mineral dust aerosol. Geophys. Res. Lett. 32, L18810. doi:10.1029/2005GL023482
Ylhäisi, J. S., Tietäväinen, H., Peltonen-Sainio, P., Venäläinen, A., Eklund, J., Räisänen, J., et al. (2010). Growing season precipitation in Finland under recent and projected climate. Nat. Hazards Earth Syst. Sci. 10, 1563–1574. doi:10.5194/nhess-10-1563-2010
Yue, X., Mickley, L. J., Logan, J. A., and Kaplan, J. O. (2013). Ensemble projections of wildfire activity and carbonaceous aerosol concentrations over the Western United States in the mid-21st centuryfire activity and carbonaceous aerosol concentrations over the Western United States in the mid-21st century. Atmos. Environ. 77, 767–780. doi:10.1016/j.atmosenv.2013.06.003
Keywords: climate change, air pollution, Europe, wildland fires, ozone, particulate matter
Citation: Im U, Geels C, Hanninen R, Kukkonen J, Rao S, Ruuhela R, Sofiev M, Schaller N, Hodnebrog Ø, Sillmann J, Schwingshackl C, Christensen JH, Bojariu R and Aunan K (2022) Reviewing the links and feedbacks between climate change and air pollution in Europe. Front. Environ. Sci. 10:954045. doi: 10.3389/fenvs.2022.954045
Received: 26 May 2022; Accepted: 20 July 2022;
Published: 12 September 2022.
Edited by:
Yuqiang Zhang, University of North Carolina at Chapel Hill, United StatesReviewed by:
Chi Li, Washington University in St. Louis, United StatesDien Wu, California Institute of Technology, United States
Copyright © 2022 Im, Geels, Hanninen, Kukkonen, Rao, Ruuhela, Sofiev, Schaller, Hodnebrog, Sillmann, Schwingshackl, Christensen, Bojariu and Aunan. This is an open-access article distributed under the terms of the Creative Commons Attribution License (CC BY). The use, distribution or reproduction in other forums is permitted, provided the original author(s) and the copyright owner(s) are credited and that the original publication in this journal is cited, in accordance with accepted academic practice. No use, distribution or reproduction is permitted which does not comply with these terms.
*Correspondence: Ulas Im, dWxhc0BlbnZzLmF1LmRr