- 1Department of Biology, University of Waterloo, Waterloo, ON, Canada
- 2Department of Geography and Environmental Studies, Wilfrid Laurier University, Waterloo, ON, Canada
Exploitation of bitumen-rich deposits in the Alberta Oil Sands Region (AOSR) by large-scale mining and processing activities has generated widespread concern about the potential for dispersal of harmful contaminants to aquatic ecosystems via fluvial and atmospheric pathways. The release of mercury has received attention because it is a potent neurotoxin for wildlife and humans. However, knowledge of baseline mercury concentration prior to disturbance is required to evaluate the extent to which oil sands development has contributed mercury to aquatic ecosystems. Here, we use stratigraphic analysis of total mercury concentration ([THg]) in radiometrically dated sediment cores from nine floodplain lakes in the AOSR and downstream Peace-Athabasca Delta (PAD) and two upland lakes in the PAD region to establish pre-1900 baseline [THg] and evaluate if [THg] has become enriched via fluvial and atmospheric pathways since oil sands mining and processing began in 1967. Concentrations of THg in sediment cores from the study lakes range from 0.022–0.096 mg/kg (dry wt.) and are below the Canadian interim sediment quality guidelines for freshwater (0.17 mg/kg). Results demonstrate no enrichment of [THg] above pre-1900 baseline via fluvial pathways at floodplain lakes in the AOSR or PAD. Enrichment of [THg] was detected via atmospheric pathways at upland lakes in the PAD region, but this occurred prior to oil sands development and aligns with long-range transport of emissions from coal combustion and other anthropogenic sources across the northern hemisphere recognized in many other lake sediment records. The inventory of anthropogenic [THg] in the upland lakes in the AOSR is less than at the Experimental Lakes Area of northwestern Ontario (Canada), widely regarded as a “pristine” area. The absence of enrichment of [THg] in lake sediment via fluvial pathways is a critical finding for stakeholders, and we recommend that monitoring at the floodplain lakes be used to inform stewardship as oil sands operators prepare to discharge treated oil sands process waters directly into the Athabasca River upstream of the PAD.
Introduction
Oil production from bitumen mining within the Alberta Oil Sands Region (AOSR) has been an important source of economic development and energy security for Canada but it also has garnered societal concern regarding its environmental impacts. These include, but are not limited to, extensive changes to land cover, carbon releases, consumptive water use, and dispersal of contaminants (Gosselin et al., 2010; Rooney et al., 2012; Arcszewski et al., 2021; Roberts et al., 2021). Distress over consequences of contaminant emissions for ecosystems and humans has existed for decades at locations proximal to the industry within the Lower Athabasca River watershed and at the Peace-Athabasca Delta (PAD), a Ramsar Wetland of International Importance located ∼200 km downstream within Wood Buffalo National Park, a UNESCO World Heritage Site (Dowdeswell et al., 2010; Gosselin et al., 2010; Schindler 2010; Mikisew Cree First Nation, 2014; WHC/IUCN, 2017; Independent Environment Consultants, 2018; Wood Buffalo National Park, 2019; WHC/IUCN, 2021). Yet, evaluation of contaminant dispersal requires understanding of natural, pre-disturbance concentrations to decipher the roles of anthropogenic and natural processes.
During the past decade, paleolimnological studies spanning from the AOSR to the PAD have provided critical knowledge of baseline, reference concentrations of contaminants and changes in contaminant deposition in aquatic ecosystems since onset of industrial development (Wiklund et al., 2012; Hall et al., 2012; Kurek et al., 2013; Wiklund et al., 2014; Cooke et al., 2017; Kay et al., 2020, in review; Klemt et al., 2020). For example, analyses of sediment cores from lakes within the AOSR have demonstrated 2.5- to 23-fold increases in flux of total PACs (polycyclic aromatic compounds) above pre-industrial levels via atmospheric pathways, with marked increases in C1-C4 alkylated PAHs (polycyclic aromatic hydrocarbons) and dibenzothiophenes which are abundant in the mined bitumen ore (Kurek et al., 2013). At the most enriched site analyzed by Kurek et al. (2013), total PAC concentrations exceeded Canadian interim sediment quality guidelines after the mid-1980s. Concentrations and flux rates of several trace elements (metals, metalloids) have also been shown to increase in lake sediments since onset of oil sands development at near- and mid-field locations within the AOSR via atmospheric pathways (Cooke et al., 2017; Klemt et al., 2020).
To date, paleolimnological studies of trace element deposition within the AOSR have focused primarily on vanadium (V) and nickel (Ni) because they serve as key indicator metals of emissions from oil sands development due to their high concentrations in the McMurray Formation bitumen relative to other geological sources and in wastes released from the mining operations (Gosselin et al., 2010; Shotyk et al., 2021). Paleolimnological analyses at upland lakes and floodplain lakes have demonstrated enrichment of V and Ni within 50 km of oil sands operations via atmospheric pathways but no enrichment of V, Ni or other trace elements (e.g., Be, Cr, Pb, Zn) via fluvial pathways within the AOSR and farther downstream at the PAD (Cooke et al., 2017; Kay et al., 2020; Klemt et al., 2020; Owca et al., 2020; see synthesis by Kay et al. in review1). Pre-industrial lake sediments have also been used to evaluate sediment quality monitoring data from the Athabasca River in the AOSR and PAD, which has demonstrated no enrichment of several trace elements due to industrial activities or other causes (Wiklund et al., 2014; Owca et al., 2020; Klemt et al., 2021).
Mercury deposition has been a focus of high concern within and downstream of the AOSR due to emissions from bitumen upgrading facilities, vehicle operations, deforestation, coke piles and dust from open pit mines (Dowdeswell et al., 2010; Kirk et al., 2014). Elevated supply of Hg can pose negative consequences for aquatic and terrestrial ecosystems because Hg can be converted to methylmercury, a toxic organic form that may bioaccumulate and biomagnify through food webs and pose risks to higher trophic levels including human consumers (Gilmour et al., 1992; Ullrich et al., 2001; Driscoll et al., 2013). As reported by Hebert et al. (2013), data from Canada’s National Pollutant Release Inventory indicate that total annual Hg emissions from oil sands operations within the AOSR almost tripled between 2000 and 2010. Also, measurements of total Hg concentration ([THg]) in snowpack within the AOSR have demonstrated net loading is highest between the Steepbank and Muskeg rivers, closest to the bitumen upgraders, and declines rapidly in a bullseye pattern with increasing distance from AR6, a central location within the AOSR (Kirk et al., 2014). Consistent with these findings, river water [THg] in the AOSR are positively correlated with discharge and have been shown to be higher downstream of mining operations compared to upstream locations (Wasiuta et al., 2019). Such knowledge of the spatial distribution of [THg] has led to fish consumption advisories in the Athabasca River but monitoring of [THg] has not revealed clear temporal trends that would implicate oil sands development as a key source of THg to fish (Evans and Talbot 2012; Kirk et al., 2014). Studies have also identified higher [THg] in aquatic bird eggs at sites downstream of the AOSR located within the PAD and western end of Lake Athabasca than at nearby locations along the Peace River and other locations in western Canada, and that [THg] in eggs at the PAD and Lake Athabasca were higher following years of high discharge of the Athabasca River (Hebert et al., 2013; Dolgova et al., 2018; Hebert, 2019). These studies identify the Athabasca River is an important vector for Hg transport within the Lower Athabasca River watershed, which has raised concern for toxicant exposure to wildlife and accumulation in the environment (Mikisew Cree First Nation, 2014; Independent Environment Consultants, 2018). However, it remains uncertain if releases from oil sands development exert strong control on [THg] in waterbird eggs because measurements were not made until several decades after onset of oil sands development and the paucity of biological monitoring data continues to hamper ability to adequately understand complex interactions among physical and ecological pathways that regulate Hg bioavailability to aquatic biota (Dolgova et al., 2018; Hebert, 2019). Importantly, these studies highlight the need for baseline, reference data to accurately discern 1) the roles of natural processes vs. industrial activities on THg loading within the AOSR and at the PAD, and 2) loading of anthropogenic THg from localized point sources within the AOSR by oil sands industrial activities vs. from diffuse anthropogenic sources outside of the AOSR.
Despite the above concerns and important knowledge gaps about releases of Hg from oil sands development to the Lower Athabasca River watershed, few paleolimnological studies have yet been conducted to establish pre-industrial baselines and assess for temporal patterns of change in Hg deposition via atmospheric and fluvial pathways. To our knowledge, only three studies report temporal analyses of [THg] in lake sediment cores within the Lower Athabasca River watershed (Bourbonniere and Telford 1996; Wiklund et al., 2012; Cooke et al., 2017) and other environmental archives such as peat cores have not been examined for temporal trends in Hg deposition. Bourbonniere and Telford (1996) examined [THg] in a sediment core from the center of Lake Athabasca and demonstrated no enrichment of [THg]. However, this site is distant from the mouth of the Athabasca River and is influenced by numerous input sources beyond the Athabasca River, thus it is unlikely to provide an informative record of temporal variation for [THg] of sediment conveyed by the Athabasca River. Cooke et al. (2017) reports THg deposition profiles from 20 upland lakes within the AOSR, whereas Wiklund et al. (2012) reports stratigraphic variation in THg deposition from a single upland lake within the PAD. Lakes reported in both these studies receive input of contaminants exclusively via atmospheric pathways (i.e., not via fluvial pathways), yet none of their sediment records have demonstrated evidence of enrichment of [THg] that can be attributed to oil sands development. At the AOSR, the records identify that enrichment of [THg] began in the early to mid-1900s, long before oil sands development, and far-field sites show greater enrichment than one near-field site (Cooke et al., 2017). At the upland lake in the PAD (PAD 18), enrichment of [THg] began before oil sands development (at ∼1940) and declined after ∼1985 when oil sands production increased rapidly (Wiklund et al., 2012). Both studies concluded that the lake sediment records reflect mid-20th century increases in emissions of Hg from coal combustion and other industrial sources, which is consistent with lake sediment core profiles from other regions at similar and higher latitudes across Canada (Muir et al., 2009), western North America (Drevnick et al., 2016), and elsewhere (Biester et al., 2007; Engstrom et al., 2014). Thus, evidence of [THg] enrichment by oil sands industrial activities via atmospheric pathways has failed to materialize from analyses of upland lake sediment core records in the AOSR and PAD.
Given that the Athabasca River is an important conduit for Hg transport (Hebert, 2019), an emerging priority is to determine pre-industrial baseline [THg] for the Lower Athabasca River and apply the baselines to quantify [THg] enrichment in sediment from floodplain lakes where supply will be dominated by fluvial processes. Mercury enrichment at the PAD via fluvial pathways has been identified as a threat by the Mikisew Cree First Nation (Mikisew Cree First Nation, 2014) to the Outstanding Universal Value of the Wood Buffalo National Park World Heritage Site, and a recent Strategic Environmental Assessment concluded “more work needs to be done to determine if these higher levels are solely a result of greater mercury supply from river-associated sources of THg (e.g., oil sands) or other factors” (Independent Environment Consultants, 2018, pg. 4–13). Also, knowledge of temporal patterns of THg deposition via atmospheric pathways at the PAD remains based on a single sediment record from upland lake PAD 18. Thus, independent verification is required to confirm temporal patterns of atmospheric Hg deposition at the PAD.
Here, we use measurements of [THg] in sediment cores spanning the past ∼220 years at nine floodplain lakes in the AOSR and PAD and two upland lakes in and adjacent to the PAD to evaluate 1) the extent of [THg] enrichment via fluvial pathways at floodplain lakes proximal to the oil sands industry within the AOSR, 2) the extent of [THg] enrichment via fluvial pathways at floodplain lakes distal to the oil sands industry within the PAD, and 3) the extent and origin of [THg] enrichment via atmospheric pathways at upland lakes in the PAD region. Findings provide critical knowledge to inform stewardship of the Lower Athabasca River region, and the baseline reference data serve to identify important locations for ongoing aquatic ecosystem monitoring of [THg] and other contaminants.
Methods
Study lake locations
We took advantage of previously obtained floodplain lake sediment records in the AOSR and floodplain and upland lake sediment records from the PAD region to perform stratigraphic analysis of [THg] (Supplementary Table SA1; Figure 1). Cores from most of the lakes have been radiometrically dated and measured for concentrations of other trace elements (Wiklund et al., 2012; Kay et al., 2020; Klemt et al., 2020) and are similarly well positioned to evaluate for enrichment of [THg] by oil sands mining and processing activities. Within the AOSR, we analyzed [THg] in sediment cores from three floodplain lakes located adjacent to the Athabasca River (Down 1, Down 26 and Down 58). These were the best options available based on a prior study by Klemt et al. (2020), which demonstrated that the cores consist predominantly of sediment carried by the Athabasca River. Paleohydrological data at Down 58, the most river-proximal of the three floodplain lakes in the AOSR, indicates strong river influence throughout the entire sediment record. Due to rapid sedimentation at Down 58, the core did not capture sediment deposited before oil sands development, but the lake is located near the downstream perimeter of the AOSR and can serve as an important archive of temporal changes in river sediment [THg]. Down 1 and Down 26 became less river influenced after ∼1980 and ∼2012, respectively, due to declines in Athabasca River discharge, a feature which enables assessment of THg deposition via atmospheric pathways as river influence waned (Klemt et al., 2020). Of note, the sediment record from Down 26 remained dominated by fluvial processes when Hg emissions from oil sands operations within the AOSR nearly tripled between 2000 and 2010 (Hebert et al., 2013).
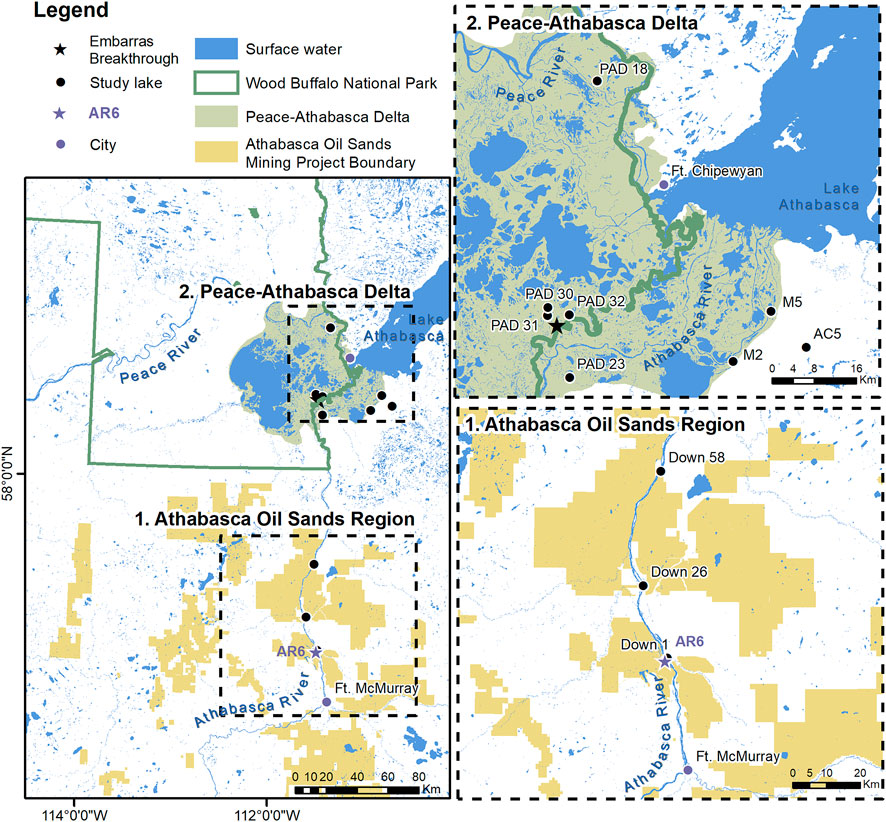
FIGURE 1. Map showing sampling sites for lakes (black circles) in the AOSR and PAD region, located in northeastern Alberta (Canada). The Wood Buffalo National Park boundary is denoted by the dark green line, the PAD is shaded in green and the Athabasca Oil Sands mining footprint including holdings for future developments in 2021 is shaded in brown. Fort McMurray and Fort Chipewyan are denoted by purple circles and AR6, a central location in the AOSR, is a purple star. Source: https://services3.arcgis.com/sLVWntsRoDa37BS8/arcgis/rest/services/OSIP_2015_Project_Boundaries/FeatureServer.
Within the PAD, six floodplain lakes (PAD 23, PAD 30, PAD 31, PAD 32, M2 and M5) were selected based on previous studies by Wiklund et al. (2014) and Kay et al. (2019, 2020), which demonstrated the Athabasca River has been a dominant source of their sediment. In July 1982, a natural geomorphic event known as the Embarras Breakthrough increased frequency and magnitude of river flooding at study lakes PAD 30 and PAD 31 and reduced influence of river flooding at PAD 32 and M5 (Kay et al., 2019). The Embarras Breakthrough provides unique opportunity to identify the role of Athabasca River on THg deposition since these sites have received marked increase (PAD 30, PAD 31) or decrease (PAD 32, M5) in sediment flux via Athabasca River floodwaters since 1982. The Embarras Breakthrough did not discernibly alter the flood regime at PAD 23 and M2 (Kay et al., 2019). Additionally, an engineered change to the Athabasca River occurred in 1972 (known as the Athabasca River Cutoff), which decreased flood susceptibility at PAD 23 (Wolfe et al., 2008). Upland lakes PAD 18 and AC5 are exclusive archives of atmospheric deposition because PAD 18 is elevated above the reach of ice-jam floods (Yi et al., 2008) and AC5 is located ∼10 km outside the delta and beyond the spatial extent of floodwaters. For the above reasons, sediment cores from the study lakes are well situated to develop long-term records of THg deposition for the region via fluvial and atmospheric pathways (See Supplementary Table SA1 for further information about study lakes).
Sediment core collection and analyses
Sediment cores were collected from each of the study lakes during 2010–2019 using a gravity corer (Glew, 1989) or hammer-driven gravity corer (Telford et al., 2021), as described in Wiklund et al. (2012), Kay et al. (2019) and Klemt et al. (2020). Sediment cores were sectioned into 0.5- or 1.0-cm intervals using a vertical extruder (Glew, 1988; Telford et al., 2021) within 24 h of collection at a local field base, and the samples were transported to the University of Waterloo, refrigerated (4°C) and stored in the dark until further analysis. Because the sediment records had been utilized for other purposes, stratigraphic sampling and analysis was dependent on available material, with the exception of the previously published THg record from PAD 18 (Wiklund et al., 2012). Continuous 1-cm sediment intervals from PAD 23, PAD 31, PAD 32 and M5 were analyzed for [THg]. For cores from PAD 30, M2, Down 1, Down 26 and Down 58, approximately every second 1-cm sediment interval was analyzed for [THg]. At AC5, every second 0.5-cm sediment interval was analyzed for [THg]. Total Hg concentrations were determined on freeze-dried sediment samples from Down 1, Down 26, Down 58, PAD 30, PAD 32, M2, M5, and AC5 at the Biotron Laboratory (Western University, Ontario, Canada) using thermal decomposition and atomic adsorption spectrophotometry and following EPA Method 7,473. The analytical uncertainty expressed as a relative percentage difference (RPD) is 3.0% based on sample duplicates. Freeze-dried continuous 1-cm sediment intervals from PAD 23 and PAD 31 were analyzed for [THg] at ALS Canada Ltd. (Edmonton) following EPA Method 200.2/245.7 and the analytical uncertainty is 4.0% based on sample duplicates.
Sediment cores were radiometrically dated using 210Pb and 137Cs methods at the University of Waterloo, and the Constant Rate of Supply (CRS) model was used to calculate 210Pb-based ages and sedimentation rates (Supplementary Figure SA1; Appleby, 2001; Sanchez-Cabeza and Ruiz-Fernandez, 2012). Age-depth relations for all cores used in this study, except Down 58 and AC5, have been previously published (Hall et al., 2012; Wiklund et al., 2012; Kay et al., 2019; Klemt et al., 2020). The complex sedimentary environment of floodplain lakes may challenge sediment core dating by 210Pb and 137Cs. The floodplain lakes in this study span a gradient of flood frequency. Lakes such as PAD 30 and PAD 31 can flood multiple times per year in spring and summer, whereas others flood episodically when ice-jams form on the rivers (e.g., Wiklund et al., 2012; Kay et al., 2019; Remmer et al., 2020). To our knowledge and based on other paleolimnological analyses, the study lakes have retained water throughout the time captured by the sediment cores. Thus, sediment deposition has been continuous, but flood events cause deposition rates to increase markedly due to influx of suspended sediment.
For floodplain lakes in the PAD, sediment core chronologies were based entirely on the 210Pb activity profiles (Kay et al., 2019). Confidence in the chronologies for the floodplain lakes is supported by consistent results obtained on cores collected from PAD 31 in 2001 and 2010 which determined an age of ca. 1982 for a shift from organic-rich to mineral-rich sediment following the Embarras Breakthrough (Wolfe et al., 2008; Kay et al., 2019). At Down 26 and Down 58 in the AOSR, however, 210Pb-based chronologies were not possible due to rapid sedimentation rates that diluted the unsupported 210Pb activity (Klemt et al., 2020; Supplementary Figure SA1). A chronology was developed for the core from Down 26 based on the 137Cs activity profile, and a cumulative dry-mass sedimentation rate was used to extrapolate the age-depth relation downcore below the 1963 137Cs activity peak (Klemt et al., 2020). At Down 58, there was no discernible 137Cs activity peak. However, measurable 137Cs activity occurred at the base of the core indicating lowermost sediments are younger than 1963 and that the entire core likely captures a period after onset of oil sands development (Klemt et al., 2020). For upland lakes PAD 18 (Wiklund et al., 2012) and AC5, CRS modelling of 210Pb activity profiles was used to develop age-depth relations and 137Cs and 241Am activity profiles were used for independent assessment at AC5. We provide further supporting details on sediment core dating in the Appendix and report the radiometric dating results for cores from all lakes in Supplementary Figure SA1.
Enrichment factor and anthropogenic flux calculations
Enrichment factors (EFs) are an effective metric for expressing trace element concentrations relative to a pre-industrial reference or baseline, which allows for quantification of temporal patterns of enrichment since onset of industrial activities (Boës et al., 2011). Baseline concentrations were defined as pre-1900 because analyses by Wiklund et al. (2012) demonstrated no evidence of [THg] enrichment until after ∼1930, which aligns with data from other lake sediment core studies in western North America (Drevnick et al., 2016). Thus, we define the pre-1900 period as pre-industrial while post-1900 is considered post-industrial even though oil sands development did not begin until 1967. Geochemical and organic matter normalization of [THg] were explored to determine an appropriate agent to account for potential confounding influence of variation in grain size and sediment composition, as was done for assessment of other trace elements in lake sediment cores in the region (Wiklund et al., 2014; Kay et al., 2020, in review; Klemt et al., 2020; Owca et al., 2020; Klemt et al., 2021). However, relations between [THg] and lithogenic elements did not meet the requirement for geochemical normalization (Loring, 1991) and organic matter content produced non-significant statistical relations for most lake sediment records. Normalization to organic matter content was used previously at PAD 18 (Wiklund et al., 2012) but was not applied here to remain consistent with data handling and presentation of the other lake sediment records. Thus, EFs were computed without normalization and used [THg] (Supplementary Tables SA2–SA12) following Eq. 1:
where [THg]x is the concentration of THg at a specific sediment interval in the core and [THg]pre-industrial is the pre-1900 average [THg] determined for the core from each individual lake. Two lakes, Down 58 and M5, did not have pre-1900 data for EF computations. At Down 58, an average of pre-1900 [THg] from Down 1 and Down 26 was used to estimate the pre-industrial baseline [THg]. At M5, the closest lake with a similar hydrological setting (M2; Kay et al., 2019; Remmer et al., 2020; Neary et al., 2021) was used to estimate the pre-industrial baseline [THg]. An EF of 1.0 indicates the [THg] in a specific stratigraphic interval is equivalent to the pre-industrial baseline, whereas an EF of 2.0 indicates a doubling above baseline. To quantify the magnitude of enrichment, we incorporated thresholds recommended by Birch (2017), which have been widely applied in this region and elsewhere (Kay et al., 2020, in review; Klemt et al., 2020; Owca et al., 2020; Jasiak et al., 2021; Klemt et al. 2021). An EF value of ≤1.5 was considered to represent “pristine conditions” and EFs from 1.5–3.0 were considered “minimal enrichment”.
For the sediment cores obtained from upland lakes PAD 18 and AC5 where [THg] enrichment was identified, anthropogenic THg fluxes were calculated using Eq. 2 from Wiklund et al. (2017), which incorporates focus-factor-corrections following recommendations from Muir et al. (2009):
where THgconci is the total Hg concentration at interval I, EFi is enrichment factor at a given interval i calculated from Eq. 1,
Anthropogenic inventories of THg were calculated for upland lakes PAD 18 and AC5 as a metric to evaluate the total mass of excess THg deposited per unit area at each lake, and to quantify atmospheric deposition of anthropogenic-sourced Hg. We followed the recommended Eq. 5 from Wiklund et al. (2017), where the sum of the excess THg flux was multiplied by the time span of all intervals (Eq. 3):
where massi is the dry mass (kg) of a specific core interval and core area is cross-sectional area of the core tube (m2).
Results
THg concentrations in sediment cores from the nine floodplain lakes in the AOSR and PAD and two upland lakes in the PAD region span from 0.022–0.096 mg/kg (dry wt.), and all values are below the Canadian interim sediment quality guidelines for freshwater (0.17 mg/kg, CCME 1999; Supplementary Tables SA2–SA12). Enrichment factors from the floodplain lakes in the AOSR (Down 1, Down 26 and Down 58) remain stable around 1.0 during both the pre-industrial and post-industrial periods and do not exceed the 1.5 threshold for “minimal enrichment” (Figures 2A–C). The only exceptions to this are three samples at the bottom portion of the sediment core from undated Down 58, but growth of oil sands mining and processing activities and associate releases of THg would be expected to result in rising EFs towards the upper strata of the core. Enrichment factors at Down 1 and Down 26 remain stable around 1.0 during intervals of weaker river influence, which began at ∼1985 and ∼2012, respectively, when rise of V and Ni enrichment was shown by Klemt et al. (2020). Similarly, floodplain lakes in the PAD (PAD 23, PAD 30, PAD 31, PAD 32, M2, M5) reveal minor variations in THg EFs and values mostly remain stable at ∼1.0 (Figures 2D–I). Only six sediment samples have THg EFs that exceed the “minimal enrichment” threshold of 1.5. These include five samples at PAD 30 (∼1961, ∼1974, ∼1983, ∼1999, ∼2001) and one sample at PAD 31 (∼1983; Figures 2E,F). Interestingly, EFs in samples deposited at ∼1983 at both PAD 30 and PAD 31 identify “minimal enrichment’ in the year following the Embarras Breakthrough. However, the rising trend for THg EFs at PAD 30 started at ∼1960 and exceeded the 1.5 “minimal enrichment” threshold before the Embarras Breakthrough, while the increase in THg EFs at PAD 31 occurred after the Embarras Breakthrough. Enrichment factors from floodplain lakes (PAD 32, M2 and M5) downstream of the Embarras Breakthrough do not noticeably change after 1982 and remain below the “minimal enrichment” threshold throughout their records.
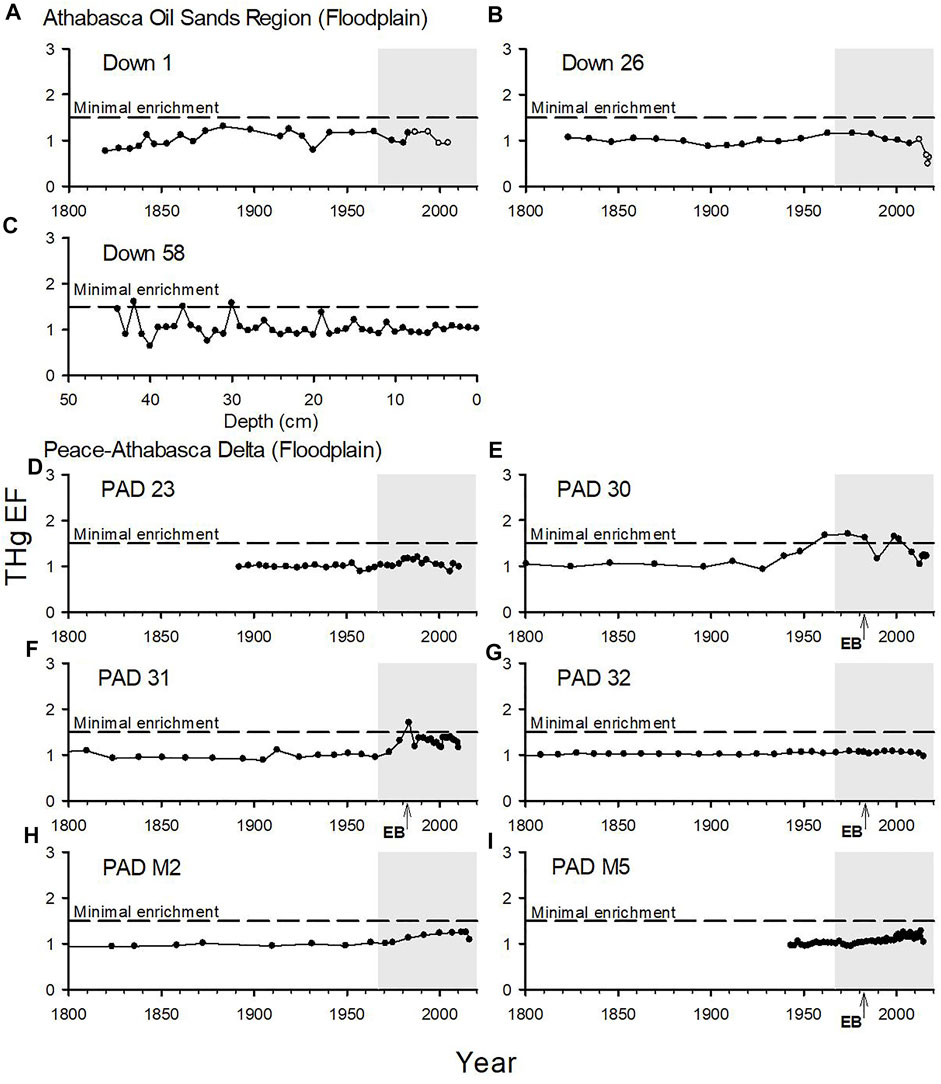
FIGURE 2. Time series of THg enrichment factors (EFs) relative to the average pre-1900 concentration at floodplain lakes in the AOSR (A–C) and PAD (D–I). The horizontal black dashed line at EF of 1.5 marks the “minimal enrichment” threshold (Birch 2017) and the grey shaded region denotes the interval of oil sands mining and processing activities on the Lower Athabasca River beginning in 1967. The white circles for Down 1 and Down 26 indicate periods of weak river influence identified by Klemt et al. (2020). The EB denotes the 1982 Embarras Breakthrough, which resulted in increased river influence at PAD 30 and PAD 31 and decreased river influence at PAD 32 and M5.
In contrast to the floodplain lakes in both the AOSR and PAD, sediment records at upland lakes PAD 18 and AC5 display systematic rising trends in THg EFs. This trend is more apparent at AC5 than at PAD 18 but the rise began several decades before onset of oil sands development at both lakes (Figures 3A,B). At PAD 18, the rising trend began at ∼1930 and reached a peak EF value (1.44) in a sample deposited ∼1968. At AC5, the rising trend also began at ∼1930 and reached a peak of 2.54 in a sample deposited ∼1966. A declining trend began shortly after the peak EF values at both lakes with EFs returning to 1.5 (AC5) or lower (PAD 18). Enrichment factors from AC5 exceed the “minimal enrichment” threshold of 1.5 while EFs from PAD 18 do not. The estimated anthropogenic THg fluxes are similar at both upland lakes, where values ranged 0–1.0 µg THg m−2 yr−1 before 1900 and rose distinctly after ∼1930 (Figures 3C,D). Anthropogenic THg fluxes at PAD 18 and AC5 remain relatively stable between ∼1960 and ∼1990 at ∼1.5 µg THg m−2 yr−1 with some variation about the average value. The peak anthropogenic THg flux for PAD 18 was 2.23 µg THg m−2 yr−1 in ∼1968 while the peak value at AC5 was 1.81 µg THg m−2 yr−1 in ∼2011. In the uppermost strata, both lakes show a general decline in anthropogenic THg flux.
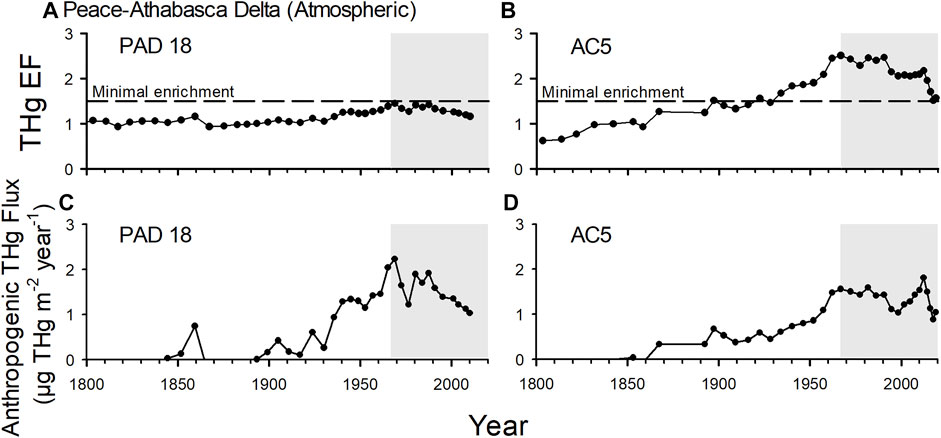
FIGURE 3. Time series of THg enrichment factors (EFs) relative to the average pre-1900 concentration at upland lakes in the PAD region: (A) PAD 18 and (B) AC5. The bottom two graphs are time series of focus factor corrected anthropogenic Hg flux for (C) PAD 18 and (D) AC5. The grey shaded region denotes the interval of oil sands mining and processing activities on the Lower Athabasca River beginning in 1967.
Discussion
Releases of Hg from oil sands mining and processing have raised questions about the extent of Hg pollution within aquatic ecosystems of the Lower Athabasca River watershed and at the PAD (Kelly et al., 2010; Kirk et al., 2014; Hebert, 2019). This is despite evidence that concentrations of Hg are low in geological materials within the AOSR, including oil sands mining source materials, and that spatial distributions of concentrations and stable isotope composition of Hg in a species of epiphytic tree lichen (Hypogymnia physodes) have shown no evidence of a significant point source of Hg from oil sands development (Blum et al., 2012). Nonetheless, it has remained difficult to further address these concerns because of insufficient knowledge of pre-industrial, reference [THg]. Our study builds upon similarly designed and executed determination of trace element enrichment in sediment profiles from lakes in the AOSR (Cooke et al., 2017; Klemt et al., 2020), PAD (Kay et al., 2020) and elsewhere (e.g., Slave River Delta: MacDonald et al., 2016 and Central Northwest Territories: Cheney et al., 2020; Jasiak et al., 2021), which have been crucial for determining pre-industrial baseline concentrations (Kay et al. in review1). Here, we capitalized on opportunity to measure [THg] from the same sediment cores used in the trace element studies from the AOSR (Klemt et al., 2020) and PAD (Kay et al., 2020) to determine [THg] before onset of oil sands development, quantify the extent of [THg] enrichment and decipher the roles for emissions from oil sands operations vs. other sources and factors operating at local to regional scales. Notably, [THg] measured in the stratigraphic profiles, including both pre- and post-industrial intervals, from floodplain and upland lakes do not exceed the Canadian interim sediment quality guidelines for freshwater (0.17 mg/kg; CCME 1999) and are lower than the mean background [THg] in Canadian lake (0.074 mg/kg) and stream (0.075 mg/kg) sediment (CCME 1999).
Stratigraphic records of THg enrichment factors at the nine floodplain lakes in the AOSR and PAD illustrate that sediments carried by the Lower Athabasca River are not enriched above pre-industrial baselines, including in sediments deposited since the onset of oil sands development. In the PAD, this includes lakes that have received increased river floodwaters and more minerogenic sediment since the 1982 Embarras Breakthrough (PAD 30 and PAD 31) and lakes that have received less river floodwaters and sediment is more organic-rich (PAD 32 and M5). These results identify neither shifting hydrological conditions or sediment composition for lakes examined within this study have measurably influenced [THg]. A minor and single exception is a discernable rise of [THg] enrichment at PAD 30, which began in the late 1930s and peaked in the early 1970s, an interval that mostly precedes oil sands development and corresponds with when the lake received deposition mainly by atmospheric pathways rather than via fluvial pathways (Kay et al., 2019). A similar pattern of Zn enrichment was observed at PAD 30 (Kay et al., 2020) and we hypothesize that localized catchment or within-lake processes are responsible for this trend (Supplementary Figure SA2). Despite this, PAD 30 remains a strong candidate to track [THg] enrichment via the Athabasca River due to its current, post-Embarras Breakthrough flood-prone status (Kay et al., 2019). Given the absence of evidence for enrichment of [THg] in floodplain lake sediment conveyed by the Lower Athabasca River after 1967, our study suggests oil sands development is not responsible for higher concentrations and altered stable isotope ratios of Hg in aquatic bird eggs at the PAD and Lake Athabasca relative to other locations (Hebert et al., 2013; Hebert, 2019). As acknowledged by Hebert (2019), periods of elevated flow on the Athabasca River likely increase delivery of THg to the PAD, but our results suggest this occurs via natural processes and at concentrations typical of pre-industrial times.
Delivery of Hg from bitumen mining in the AOSR via atmospheric pathways to aquatic ecosystems at the PAD is another chief concern (Kirk et al., 2014; Independent Environment Consultants, 2018). Temporal patterns of Hg deposition prior to oil sands development have been assessed from stratigraphic analysis of a sediment core at a single upland lake (PAD 18) within the PAD, and results provided no measurable evidence of far-field [THg] enrichment via atmospheric pathways attributable to oil sands development since 1967 (Wiklund et al., 2012). To further test and verify the findings of that study, [THg] analysis of a sediment core from AC5 was used as an independent replicate of PAD 18. Temporal patterns of variation in [THg] enrichment are similar but differ in magnitude. Since the early 1900s, THg EFs have exceeded the threshold of “minimal enrichment” at AC5 but not at PAD 18. Anthropogenic flux calculations account for differences in sedimentation rate and focusing factor between the lake sediment records, and their close correspondence provides strong confidence in the reproducibility of the results. Anthropogenic fluxes began to rise several decades before onset of oil sands development at both lakes, then plateaued and declined as oil sands mining and processing activities increased. This provides compelling evidence that oil sands development has not increased delivery of THg to the PAD via atmospheric pathways. Indeed, the two upland lakes in the PAD region have remarkably similar EF profiles to upland lakes in the AOSR (Cooke et al., 2017), where little to no evidence of increase in enrichment or flux of THg was observed after the onset of oil sands activities. Notably, the temporal patterns of variation in THg EFs and anthropogenic flux are different from those of vanadium, a key oil sands indicator, which increased with onset of oil sands development at the same upland lakes in the AOSR (Cooke et al., 2017). In contrast, stratigraphic patterns of [THg] enrichment from the upland lakes in the AOSR and PAD align with those from other lake sediment records across western North America (Drevnick et al., 2016), attributed to long-range transport of emissions from coal combustion and other anthropogenic sources, and they match closely with industrial development in North America and elsewhere (Callender and van Metre, 1997; Couture et al., 2008). Interestingly, a decline in EFs and anthropogenic fluxes is observed at PAD 18 and AC5 since the onset of oil sands development but values have not yet completely returned to baseline. This pattern has been noted as a common feature for sediment deposited in recent decades at remote lakes in northern Canada where contaminants are supplied predominantly via atmospheric pathways (Muir et al., 2009). There, storage and gradual release of anthropogenic THg in catchment soils and biomass (Harris et al., 2007) or maintenance of THg flux from sources in Eurasia have been proposed as mechanisms that account for reduced decline of anthropogenic THg flux in recent decades compared to other trace elements (Muir et al., 2009).
To provide regional context to the anthropogenic THg fluxes and inventories derived from the upland lakes in PAD region, we compared results to other Canadian locations (Figure 4). The Experiment Lakes Area (ELA) is a remote region near the Ontario-Manitoba border sufficiently unaffected by human activities where whole lake experiments are conducted with minimal confounding effects of other anthropogenic influences. In contrast, the Flin Flon smelter was one of the largest point sources of THg in Canada when operational (∼1930–2010, peak emissions ∼1980; Pirrone et al., 1998). Data from ELA and Phantom Lake (5 km from a metals smelter near Flin Flon, Manitoba) were obtained from Wiklund et al. (2017), where the same methods were employed to calculate anthropogenic fluxes and inventories. For lakes in the ELA, mean values from 5 lakes (ELA 240, ELA 373, ELA 377, ELA 378 and ELA 442) were computed for THg anthropogenic fluxes and inventories (Wiklund et al., 2017). Distinct differences are noted among the three regions. Anthropogenic THg flux (0–2,857.59 µg Hg m−2 yr−1) and THg inventory (86,857 µg THg m−2) at smelter-proximal Phantom Lake are ∼700-fold higher than corresponding values at ELA (0–6.13 µg THg m−2 yr−1; 492.82 µg THg m−2), PAD 18 (0–2.23 µg THg m−2 yr−1; 127.15 µg THg m−2) and AC5 (0–1.41 µg THg m−2 yr−1; 124.35 µg THg m−2). Since 1930, the cumulative inventory of anthropogenic THg at the two upland lakes in the PAD region parallels the average values for the five lakes in the ELA but the values at the ELA are four-fold higher than at the PAD region (Figure 4C). This difference is likely attributed to greater industrial activity in the Great Lakes region. Nonetheless, the ELA is still widely regarded as a “pristine” environment (Pirrone et al., 1998; Hall et al., 2005; Wiklund et al., 2017) and, thus, it is notable that the upland lakes in the PAD region exhibit lower inventories of anthropogenic THg.
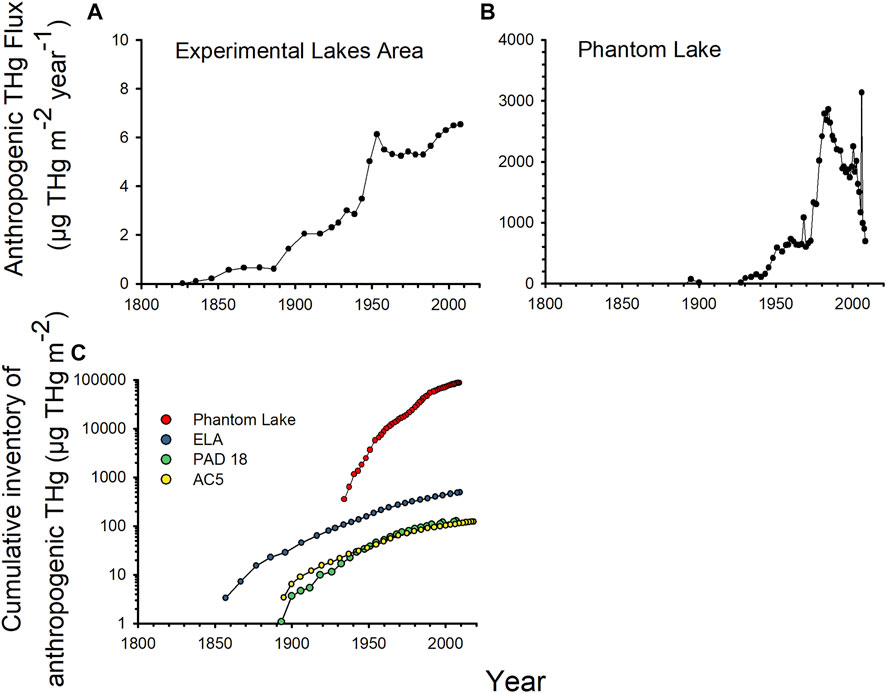
FIGURE 4. Panel (A) is the mean anthropogenic THg flux from five lakes (ELA 240, ELA 373, ELA 377, ELA 378 and ELA 442) in the Experimental Lakes Area of Ontario (Wiklund et al., 2017). Panel (B) is the anthropogenic THg flux from Phantom Lake, which is located ∼5 km from a smelter in Flin Flon, Manitoba (Wiklund et al., 2017). Panel (C) is the cumulative anthropogenic inventories of THg for Phantom Lake, ELA, PAD 18 and AC5 plotted on a log-scale. The ELA anthropogenic THg inventory is a mean value from the same five lakes as the anthropogenic flux calculation.
Our study reaffirms that paleolimnological analyses of temporal variation in deposition of substances of concern provide valuable, and often missing, pre-industrial baseline information, which is required to delineate the roles of natural and anthropogenic processes. Temporal patterns of THg EFs align with other paleolimnological studies of metals (Wiklund et al., 2014; Kay et al., 2020; Owca et al., 2020) and polycyclic aromatic compounds (Hall et al., 2012) in floodplain lakes of the PAD, which also demonstrate oil sands development has not enriched contaminant concentrations at the PAD above pre-industrial baselines via fluvial pathways. Additionally, anthropogenic flux and anthropogenic inventories of THg determined from sediment cores of upland lakes at the PAD region indicate that oil sands development has not increased delivery of THg to the PAD via atmospheric pathways. These findings are important given that the International Committee on Conservation of Nature/World Heritage Committee is currently evaluating Canada’s response to their assessment and recommendations regarding the World Heritage status of Wood Buffalo National Park. Degradation of aquatic ecosystems in the PAD by increased delivery of contaminants from oil sands development are prominent among the perceived threats (WHC/IUCN 2021), yet paleolimnological data constrained by knowledge of pre-industrial concentrations of substances of concern do not support such attribution. We suggest lakes presented in this study, equipped with knowledge of pre-industrial baselines, can continue to serve as a useful source of information for evaluating future surficial lake and river-bottom sediment in support of ongoing monitoring of [THg] as well as other trace elements (i.e., V, Ni). The informative nature of this approach has been illustrated previously by Wiklund et al. (2014), Owca et al. (2020) and Klemt et al (2021) for trace elements but could be expanded readily to be part of an integrated monitoring program, which evaluates [THg] in sediment, air, and biota throughout the Lower Athabasca River watershed. Application of this approach may be especially timely pending discharge of oil sands process waters directly into the Athabasca River (Hicks and Scrimgeour 2019).
Data availability statement
The original contributions presented in the study are included in the article/Supplementary Material. Further inquiries can be directed to the corresponding author.
Author contributions
CRediT: MK: Formal analysis, Investigation, Data Curation, Writing—Original Draft, Writing—Review and Editing, Visualization. JW: Formal Analysis, Validation, Writing—Review and Editing. XS: Investigation, Formal Analysis. CS: Visualization. JA: Investigation, Formal Analysis. LM: Investigation, Formal Analysis, Writing—Review and Editing. WK: Investigation, Formal Analysis. KB: Investigation, Formal Analysis. RH: Conceptualization, Methodology, Writing—Review and Editing, Supervision, Project Administration, Funding Acquisition. BW: Conceptualization, Methodology, Writing—Review and Editing, Supervision, Project Administration, Funding Acquisition.
Funding
This research was supported by multiple grants and contracts from federal (NSERC Discovery Grant Program: RGPIN-2015-05591, RGPIN-2016-03630, RGPIN-2017-05462; NSERC Northern Research Supplement Program: 305405-2016, Polar Continental Shelf Program of Natural Resources Canada; Northern Scientific Training Program), provincial (Alberta Environment and Parks) and industry (Suncor Energy, Canadian Natural Resources Limited) sources to RH and BW. MK was supported by a W. Garfield Weston Doctoral Award for Northern Research, Ontario Graduate Scholarship and Queen Elizabeth II Graduate Scholarship in Science and Technology. Logistical support was provided by Wood Buffalo National Park.
Acknowledgments
We thank all the students who assisted in the field and lab. We appreciate the insightful comments provided by the reviewers.
Conflict of interest
The authors declare that the research was conducted in the absence of any commercial or financial relationships that could be construed as a potential conflict of interest.
Publisher’s note
All claims expressed in this article are solely those of the authors and do not necessarily represent those of their affiliated organizations, or those of the publisher, the editors and the reviewers. Any product that may be evaluated in this article, or claim that may be made by its manufacturer, is not guaranteed or endorsed by the publisher.
Supplementary material
The Supplementary Material for this article can be found online at: https://www.frontiersin.org/articles/10.3389/fenvs.2022.949339/full#supplementary-material
Footnotes
1Kay, M. L., Jasiak, I., Klemt, W. H., Wiklund, J. A., Faber, J. A., MacDonald, L. A., et al. Spatio-temporal patterns of metal(loid) enrichment in lake sediment records from large-scale mining operations in northwestern Canada: A synthesis. in review.
References
Appleby, P. G. (2001). “Chronostratigraphic techniques in recent sediments,” in Tracking environmental change using lake sediments: Basin analysis, coring, and chronological techniques, developments in paleoenvironmental research. Editor J. P. Smol, 1 171–203.
Arciszewski, T. J., Hazewinkel, R. O., and Dubé, M. G. (2021). A critical review of the ecological status of lakes and rivers from Canada’s oil sands region. Integr. Environ. Assess. Manag. 18, 361–387. doi:10.1002/ieam.4524
Biester, H., Bindler, R., Martinez-Cortizas, A., and Engstrom, D. R. (2007). Modeling the past atmospheric deposition of mercury using natural archives. Environ. Sci. Technol. 41, 4851–4860. doi:10.1021/es0704232
Birch, G. F. (2017). Determination of sediment metal background concentrations and enrichment in marine environments – a critical review. Sci. Total Environ. 580, 813–831. doi:10.1016/j.scitotenv.2016.12.028
Blum, J. D., Johnson, M. W., Gleason, J. D., Demers, J. D., Landis, M. S., and Krupa, S. (2012). “Mercury concentration and isotopic composition of epiphytic tree lichens in the Athabasca Oil Sands Region,” in Developments in environmental science. Editor K. E. Percy, 11, 373–390.
Boës, X., Rydberg, J., Martinez-Cortizas, A., Bindler, R., and Renberg, I. (2011). Evaluation of conservative lithogenic elements (Ti, Zr, Al, and Rb) to study anthropogenic element enrichments in lake sediments. J. Paleolimnol. 46, 75–87. doi:10.1007/s10933-011-9515-z
Bourbonniere, R. A., and Telford, S. L. (1996). Depositional history of sediment in Lake Athabasca: geochronology, bulk parameters, contaminants and biogeochemical markers. Northern River Basins Study Project Report 72.
Callender, E., and van Metre, P. C. (1997). Environmental policy analysis, peer reviewed: Reservoir sediment cores show U.S. Lead declines. Environ. Sci. Technol. 31, 424A–428A. doi:10.1021/es972473k
Canadian Council of Ministers of the Environment (1999). “Canadian sediment quality guidelines for the protection of aquatic life: Mercury,” in Canadian environmental quality guidelines (Winnipeg: Canadian Council of Ministers of the Environment).
Cheney, C. L., Eccles, K. M., Kimpe, L. E., Thienpont, J. R., Korosi, J. B., Blais, J. M., et al. (2020). Determining the effects of past gold mining using a sediment palaeotoxicity model. Sci. Total Environ. 718, 137308. doi:10.1016/j/scitotenv.2020.137308
Cooke, C. A., Kirk, J. L., Muir, D. C., Wiklund, J. A., Wang, X., Gleason, A., et al. (2017). Spatial and temporal patterns in trace element deposition to lakes in the Athabasca Oil Sands Region (Alberta, Canada). Environ. Res. Lett. 12, 124001. doi:10.1088/1748-9326/aa9505
Couture, R. M., Gobeil, C., and Tessier, A. (2008). Chronology of atmospheric deposition of arsenic inferred from reconstructed sedimentary records. Environ. Sci. Technol. 42, 6508–6513. doi:10.1021/es800818j
Dolgova, S., Popp, B. N., Courtoreille, K., Espie, R. H. M., Maclean, B., McMaster, M., et al. (2018). Spatial trends in a biomagnifying contaminant: Application of amino acid compound–specific stable nitrogen isotope analysis to the interpretation of bird mercury levels. Environ. Toxicol. Chem. 37, 1466–1475. doi:10.1002/etc.4113
Dowdeswell, L., Dillon, P., Ghoshal, S., Miall, A., Rasmussen, J., and Smol, J. P. (2010). A foundation for the future: Building an environmental monitoring system for the oil sands. A report submitted to the Minister of Environment, 1–49.
Drevnick, P. E., Cooke, C. A., Barraza, D., Blais, J. M., Coale, K. H., Curtis, C. J., et al. (2016). Spatiotemporal patterns of mercury accumulation in lake sediments of Western North America. Sci. Total Environ. 568, 1157–1170. doi:10.1016/j.scitotenv.2016.03.167
Driscoll, C. T., Mason, R. P., Chan, H. M., Jacob, D. J., and Pirrone, N. (2013). Mercury as a global pollutant: Sources, pathways, and effects. Environ. Sci. Technol. 47, 4967–4983. doi:10.1021/es305071v
Engstrom, D. R., Fitzgerald, W. F., Colin, A., Cooke, C. A., Lamborg, C. H., Drevnick, P. E., et al. (2014). Atmospheric Hg emissions from preindustrial gold and silver extraction in the Americas: a reevaluation from lake-sediment archives. Environ. Sci. Technol. 48, 6533–6543. doi:10.1021/es405558e
Evans, M., and Talbot, A. (2012). Investigations of mercury concentrations in walleye and other fish in the Athabasca River ecosystem with increasing oil sands developments. J. Environ. Monit. 14, 1989. doi:10.1039/c2em30132f
Gilmour, C. C., Henry, E. A., and Mitchell, R. (1992). Sulfate stimulation of mercury methylation in fresh-water sediments. Environ. Sci. Technol. 26, 2281–2287. doi:10.1021/es00035a029
Glew, J. R. (1988). A portable extruding device for close interval sectioning of unconsolidated core samples. J. Paleolimnol. 1, 235–239. doi:10.1007/BF00177769
Glew, J. R. (1989). A new trigger mechanism for sediment samplers. J. Paleolimnol. 2, 241–243. doi:10.1007/BF00195474
Gosselin, P., Hrudey, S., Naeth, A., Plourde, A., Therrien, R., van der Kraar, G., et al. (2010). Environmental and health impacts of Canada's oil sands industry. Ottawa: The Royal Society of Canada.
Hall, B. D., Manolopoulos, H., Hurley, J. P., Schauer, J. J., Louis, St.V. L., Kenski, D., et al. (2005). Methyl and total mercury in precipitation in the Great Lakes region. Atmos. Environ. 39, 7557–7569. doi:10.1016/j.atmosenv.2005.04.042
Hall, R. I., Wolfe, B. B., Wiklund, J. A., Edwards, T. W. D., Farwell, A. J., Dixon, D. G., et al. (2012). Has Alberta Oil Sands development altered delivery of polycyclic aromatic compounds to the Peace-Athabasca Delta? PLoS ONE 7, e46089. doi:10.1371/journal.pone.0046089
Harris, R. C., Rudd, J. W. M., Amyot, M., Babiarz, C. L., Beaty, K. G., Blanchfield, P. J., et al. (2007). Whole-ecosystem study shows rapid fish-mercury response to changes in mercury deposition. Proc. Natl. Acad. Sci. U. S. A. 104, 16586–16591. doi:10.1073/pnas.0704186104
Hebert, C. E., Campbell, D., Kindopp, R., MacMillan, S., Martin, P., Neugebauer, E., et al. (2013). Mercury trends in colonial waterbird eggs downstream of the oil sands region of Alberta, Canada. Environ. Sci. Technol. 47, 11785–11792. doi:10.1021/es402542w
Hebert, C. E. (2019). The river runs through it: The Athabasca River delivers mercury to aquatic birds breeding far down stream. PLoS ONE 14, e0206192. doi:10.1371/journal.pone.0206192
Hicks, K., and Scrimgeour, G. (2019). A study design for enhanced environmental monitoring of the Lower Athabasca River. Edmonton: Government of Alberta, Ministry of Environment and Parks. Available at: https://open.alberta.ca/publications/9781460145364.
Independent Environment Consultants (IEC) (2018). Strategic environmental assessment of Wood Buffalo National Park World Heritage Site. Milestone 3 – Final SEA Report, Vol. 1. Markham, Ontario: Independent Environment Consultants IEC.
Jasiak, I., Wiklund, J. A., Leclerc, E., Telford, J. V., Couture, R. M. C., Venkiteswaran, J. J., et al. (2021). Evaluating spatiotemporal patterns of arsenic, antimony, and lead deposition from legacy gold mine emissions using lake sediment records. Appl. Geochem. 134, 105053. doi:10.1016/j.apgeochem.2021.105053
Kay, M. L., Wiklund, J. A., Remmer, C. R., Neary, L. K., Brown, K., Ghosh, A., et al. (2019). Bi-directional hydrological changes in perched basins of the Athabasca Delta (Canada) in recent decades caused by natural processes. Environ. Res. Commun. 1, 081001. doi:10.1088/2515-7620/ab37e7
Kay, M. L., Wiklund, J. A., Remmer, C. R., Owca, T. J., Klemt, W. H., Neary, L. K., et al. (2020). Evaluating temporal patterns of metals concentrations in floodplain lakes of the Athabasca Delta (Canada) relative to pre-industrial baselines. Sci. Total Environ. 20, 135309. doi:10.1016/j.scitotenv.2019.135309
Kelly, E. N., Schindler, D. W., Hodson, P. V., Short, J. W., Radmanovich, R., Nielsen, C. C., et al. (2010). Oil sands development contributes elements toxic at low concentrations to the Athabasca River and its tributaries. Proc. Natl. Acad. Sci. U. S. A. 107, 16178–16183. doi:10.1073/pnas.1008754107
Kirk, J. L., Muir, D. C., Gleason, A., Wang, X., Lawson, G., Frank, R. A., et al. (2014). Atmospheric deposition of mercury and methylmercury to landscapes and waterbodies of the Athabasca Oil Sands Region. Environ. Sci. Technol. 48, 7374–7383. doi:10.1021/es500986r
Klemt, W. H., Brua, R. B., Culp, J. M., Hicks, K., Wolfe, B. B., and Hall, R. I. (2021). Evaluating Lower Athabasca River sediment metals concentrations from Alberta Oil Sands monitoring programs using predevelopment baselines. Environ. Sci. Technol. 55, 8817–8828. doi:10.1021/acs.est.1c01761
Klemt, W. H., Kay, M. L., Wiklund, J. A., Wolfe, B. B., and Hall, R. I. (2020). Assessment of vanadium and nickel enrichment in lower Athabasca River floodplain lake sediment within the Athabasca oil sands region (Canada). Environ. Pollut. 265, 114920. doi:10.1016/j.envpol.2020.114920
Kurek, J., Kirk, J. L., Muir, D. C., Wang, X., Evans, M. S., Smol, J. P., et al. (2013). Legacy of a half century of Athabasca oil sands development recorded by lake ecosystems. Proc. Natl. Acad. Sci. U. S. A. 110, 1761–1766. doi:10.1073/pnas.1217675110
Loring, D. H. (1991). Normalization of heavy-metal data from estuarine and coastal sediments. ICES J. Mar. Sci. 48, 101–115. doi:10.1093/icesjms/48.1.101
MacDonald, L. A., Wiklund, J. A., Elmes, M. C., Wolfe, B. B., and Hall, R. I. (2016). Paleolimnological assessment of riverine and atmospheric pathways and sources of metal deposition at a floodplain lake (Slave River Delta, Northwest Territories, Canada). Sci. Total Environ. 544, 811–823. doi:10.1016/j.scitotenv.2015.11.173
Mikisew Cree First Nation (MCFN) (2014). Petition to the World Heritage Committee requesting inclusion of Wood Buffalo National Park on the list of World Heritage in Danger. Fort Chipewyan, 1–18.
Muir, D. C. G., Wang, X., Yang, F., Nguyen, N., Jackson, T. A., Evans, M. S., et al. (2009). Spatial trends and historical deposition of mercury in eastern and northern Canada inferred from lake sediment cores. Environ. Sci. Technol. 43, 4802–4809. doi:10.1021/es8035412
Neary, L. K., Remmer, C. R., Krist, J., Wolfe, B. B., and Hall, R. I. (2021). A new lake classification scheme for the Peace-Athabasca Delta (Canada) characterizes hydrological processes that cause lake-level variation. J. Hydrol. Reg. Stud. 38, 100948. doi:10.1016/j.ejrh.2021.100948
Owca, T. J., Kay, M. L., Faber, J. A., Remmer, C. R., Zabel, N., Wiklund, J. A., et al. (2020). Use of pre-industrial baselines to monitor anthropogenic enrichment of metals concentrations in recently deposited sediment of floodplain lakes in the Peace-Athabasca Delta (Alberta, Canada). Environ. Monit. Assess. 192, 196. doi:10.1007/s10661-020-8067-y
Pirrone, N., Allegrini, I., Keeler, G. J., Nriagu, J. O., Rossman, R., Robbins, J. A., et al. (1998). Historical atmospheric mercury emissions and depositions in North America compared to mercury accumulations in sedimentary records. Atmos. Environ. 32, 929–940. doi:10.1016/S1352-2310(97)00353-1
Remmer, C. R., Neary, L. K., Kay, M. L., Wolfe, B. B., and Hall, R. I. (2020). Multi-year isoscapes of lake water balances across a dynamic northern freshwater delta. Environ. Res. Lett. 15, 104066. doi:10.1088/1748-9326/abb267
Roberts, D., Hazewinkel, R. O., Arciszewski, T. J., Beausoleil, D., Davidson, C., Horb, E. C., et al. (2021). An integrated knowledge synthesis of regional ambient monitoring in Canada’s oil sands. Integr. Environ. Assess. Manag. 18, 428–441. doi:10.1002/ieam.4505
Rooney, R. C., Bayley, S. E., and Schindler, D. W. (2012). Oil sands mining and reclamation cause massive loss of peatland and stored carbon. Proc. Natl. Acad. Sci. U. S. A. 109, 4933–4937. doi:10.1073/pnas.1117693108
Sanchez-Cabeza, J. A., and Ruiz-Fernandez, A. C. (2012). 210Pb sediment radiochronology: An integrated formulation and classification of dating models. Geochim. Cosmochim. Acta 82, 183–200. doi:10.1016/j.gca.2010.12.024
Shotyk, W., Bicalho, B., Cuss, C., Donner, M., Grant-Weaver, I., Javed, M. B., et al. (2021). Trace elements in the Athabasca bituminous sands: A geochemical explanation for the paucity of environmental contamination by chalcophile elements. Chem. Geol. 581, 120392. doi:10.1016/j.chemgeo.2021.120392
Telford, J. V., Kay, M. L., Vander Heide, H., Wiklund, J. A., Owca, T. J., Faber, J. A., et al. (2021). Building upon open-barrel corer and sectioning systems to foster the continuing legacy of John Glew. J. Paleolimnol. 65, 271–277. doi:10.1007/s10933-020-00162-w
Ullrich, S. M., Tanton, T. W., and Abdrashitova, S. A. (2001). Mercury in the aquatic environment: a review of factors affecting methylation. Crit. Rev. Environ. Sci. Technol. 31, 241–293. doi:10.1080/20016491089226
Wasiuta, V., Kirk, J. L., Chambers, P. A., Alexander, A. C., Wyatt, F. R., Rooney, R. C., et al. (2019). Accumulating mercury and methylmercury burdens in watersheds impacted by Oil Sands pollution. Environ. Sci. Technol. 53, 12856–12864. doi:10.1021/acs.est.9b02373
WHC/IUCN (2017). Reactive monitoring mission to Wood Buffalo National Park. Canada Technical Report, 28–30.
WHC/IUCN (2021). United Nations Educational, Scientific and Cultural Organization Convention concerning the protection of the world cultural and natural heritage. online meeting. China, 188 (Retrieved from: https://whc.unesco.org/en/sessions/44com/(Accessed on February 11th, 2022).
Wiklund, J. A., Hall, R. I., Wolfe, B. B., Edwards, T. W. D., Farwell, A. J., Dixon, D. G., et al. (2012). Has Alberta oil sands development increased far-field delivery of airborne contaminants to the Peace–Athabasca Delta? Sci. Total Environ. 433, 379–382. doi:10.1016/j.scitotenv.2012.06074
Wiklund, J. A., Hall, R. I., Wolfe, B. B., Edwards, T. W. D., Farwell, A. J., Dixon, D. G., et al. (2014). Use of pre-industrial floodplain lake sediments to establish baseline river metal concentrations downstream of Alberta oil sands: a new approach for detecting pollution of rivers. Environ. Res. Lett. 9, 124019. doi:10.1088/1748-9326/9/12/124019
Wiklund, J. A., Kirk, J. L., Muir, D. C. G., Evans, M., Yang, F., Keating, J., et al. (2017). Anthropogenic mercury deposition in Flin Flon Manitoba and the Experimental Lakes Area Ontario (Canada): A multi-lake sediment core reconstruction. Sci. Total Environ. 586, 685–695. doi:10.1016/j.scitotenv.2017.02.046
Wolfe, B. B., Hall, R. I., Edwards, T. W. D., Vardy, S. R., Falcone, M. D., Sjunneskog, C., et al. (2008). Hydroecological responses of the Athabasca Delta, Canada, to changes in river flow and climate during the 20th century. Ecohydrol. 1, 142–148. doi:10.1002/eco.13
Keywords: Alberta Oil Sands Region, Wood Buffalo National Park, mercury, sediment quality, paleolimnology, aquatic ecosystem monitoring
Citation: Kay ML, Wiklund JA, Sun X, Savage CAM, Adams JK, MacDonald LA, Klemt WH, Brown KC, Hall RI and Wolfe BB (2022) Assessment of mercury enrichment in lake sediment records from Alberta Oil Sands development via fluvial and atmospheric pathways. Front. Environ. Sci. 10:949339. doi: 10.3389/fenvs.2022.949339
Received: 20 May 2022; Accepted: 13 July 2022;
Published: 10 August 2022.
Edited by:
Michael Essington, The University of Tennessee, United StatesReviewed by:
Joseph Graney, Binghamton University, United StatesR. Naresh Kumar, Birla Institute of Technology, Mesra, India
Copyright © 2022 Kay, Wiklund, Sun, Savage, Adams, MacDonald, Klemt, Brown, Hall and Wolfe. This is an open-access article distributed under the terms of the Creative Commons Attribution License (CC BY). The use, distribution or reproduction in other forums is permitted, provided the original author(s) and the copyright owner(s) are credited and that the original publication in this journal is cited, in accordance with accepted academic practice. No use, distribution or reproduction is permitted which does not comply with these terms.
*Correspondence: Mitchell L. Kay, bWl0Y2hlbGwua2F5QHV3YXRlcmxvby5jYQ==