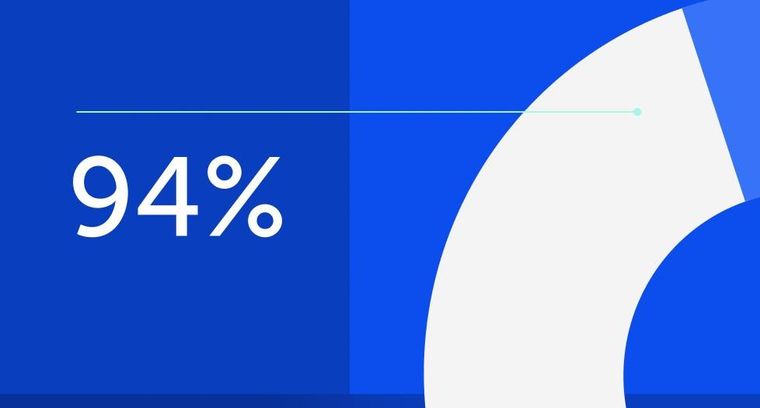
94% of researchers rate our articles as excellent or good
Learn more about the work of our research integrity team to safeguard the quality of each article we publish.
Find out more
REVIEW article
Front. Environ. Sci., 13 July 2022
Sec. Toxicology, Pollution and the Environment
Volume 10 - 2022 | https://doi.org/10.3389/fenvs.2022.944010
This article is part of the Research TopicWaste Management of Petroleum Hydrocarbons in Marine EnvironmentView all 9 articles
Mechanical oil recovery, a most used tool for oil spill response, can generate a considerable proportion of oil-contaminated water (10–70%). Large storage space is commonly required to transport the recovered oil and water mixture to shore. Transit and transportation consume loads of time, money, and resources while reducing oil recovery’s overall efficiency and capacity. Techniques of on-site treatment and disposal of oily wastewater provide benefits for oil recovery by freeing storage space. However, the high petroleum content of decant water can lead to uncertain risks, if discharged into the marine ecosystem. Insufficient ecological toxicity data and research limit the standardization and establishment of regulative tools. To fill the knowledge gaps, this review comprehensively summarized recent studies on the potential impacts of the organic composition in decant water, including oil–water accommodated fractions, dispersed oil droplets, and other related chemicals, on various marine species (i.e., bacterium, invertebrates, fishes, plants, reptiles, and mammals). The toxicity effects and the ecological endpoints of oils, TPH, and PAHs on different species were discussed. Recommendations for future ecological impacts and decant water composition were provided to support the on-site disposal of the water fraction.
Mechanical oil recovery is a widely accepted response tool in a marine oil spill by stakeholders due to the capacity of collecting spilled oil and reducing environmental impacts on spilled sites (Li et al., 2016; Walker et al., 2018). In a general process, spilled oil is thickened by booms, recovered by skimmers, stored and separated on offshore vessels, and shipped onshore for treatment or disposal (Etkin & Nedwed, 2021). One of the disadvantages of mechanical oil recovery is the generation of a large volume of oil and water emulsion during oil collections (Gaaseidnes & Turbeville, 1999). The water content in the recovered fluid can reach more than 60% and even 90% (Gaaseidnes & Turbeville, 1999; Etkin & Nedwed, 2021). The high water content not only reduces the storage capacity for recovered oil and intensifies the demands on offshore operational and logistical support, but also greatly increases the workload and pressure of onshore disposal/treatments with a large amount of oily waste (National Academies of Sciences & Medicine, 2020). An alternative that has been frequently mentioned to mitigate the problem is oil decanting, which is a process of oil–water separation and water disposal at sea (Figure 1) (Nuka Research and Planning Group, LLC., 2012, 2017; IPIECA, 2013; Buchholz et al., 2016; The U.S. National Response Team, 2018). The process allows for more efficient use of onboard storage space by storing more concentrated oil, thus facilitating mechanical recovery operations (Buchholz et al., 2016).
Unlike mechanical recovery, the decanting operation needs permission from authorities (Buchholz et al., 2016). The International Spill Control Organization suggests the decanting process should follow the International Convention for the Prevention of Pollution from Ships (MARPOL) Annex 1, which states that all the discharges of offshore oily wastewater should have an oil content less than 15 ppm (IMO, 2012; ISCO, 2015). However, many authorities in the U.S. lack decanting protocols (Buchholz et al., 2016) and the approval of decanting varies in regions (Etkin & Nedwed, 2021). In Canada, no regulations have been established for decant water (National Energy Board, 2010).
The major concern of decant water discharge is the release of dispersed oil and other additives into the ocean. The contact of oil on the surface and in the water column would result in the acute (<96 h) and chronic (>96 h) toxicity to plants, invertebrates, fishes, reptiles and amphibians, birds, and mammals (Nordtug et al., 2011; Dupuis & Ucan-Marin, 2015; Lee et al., 2015). The toxicity studies on the dispersed oil could be used for the ecological assessment of decant water to the marine environment. SL Ross Environmental Research Ltd (2005) suggested using water accommodated fraction of oil (WAF) to investigate the ecological impacts of the decant water discharge. WAF includes hydrocarbons that dissolve and suspend in water, namely water-soluble fractions of oil (WSF) and oil droplets, respectively (National Research Council, 2005; Lee et al., 2015). The different composition of WSF, such as low molecular weight saturates, monocyclic and polycyclic aromatic hydrocarbons (PAHs), and their homologs, phenols, naphthenic acids, and heterocyclic aromatic compounds, lead to the different toxicologic effects to the marine species (Holth et al., 2009; Kang et al., 2014; Lee et al., 2015; Chand et al., 2022). Oil droplets also showed toxicity to Atlantic cod and haddock (Olsvik et al., 2011; Sørhus et al., 2015; Sørensen et al., 2017). The potential additives and treatment by-products should be considered as well.
To better understand the ecological impacts of decant water, the acute and chronic impacts of WAF compositions, suspended oil, demulsifiers, and potential by-products after treatment to plants, invertebrates, fishes, reptiles, and mammals are reviewed. The most commonly referenced indicators are considered, including the species used for toxicity (e.g., Vibrio fischeri, mysid shrimp), as well as commercially significant invertebrates and fish species in Canada (e.g., crab snow, shrimp, cod, flatfish, herring, salmon) (DFO, 2017). The ecological endpoints of petroleum hydrocarbons and PAHs to marine species are summarized. The findings of this study provide the information required for future science-based decision-making on policies and regulations for the management of on-site discharges of decant water.
In a decanting process, recovered oil and water being vigorously mixed in the skimming and pumping systems and settled in temporary storage tanks for a certain time (1–24 h) (SL Ross Environmental Research Ltd., 2005). The process is similar to the Chemical Response to Oil Spill Ecological Research Forum (CROSERF) methods in terms of WAF preparation (Aurand & Coelho, 2005; DFO, 2017). In general, a certain amount of oil (0.01–25 g/L) is added to filtered seawater and stirred for 18–24 h (Singer et al., 2000; Özhan et al., 2014; Philibert et al., 2021). For the preparation of high-energy WAF, oil and water are vigorously vortexed for 18 h. The water phase after a 6 h settling is used for toxicity analysis (Özhan et al., 2014). Whereas the preparation of oil and water emulsion for decanting uses a high oil dose (<25%). The mixing method for emulsification is more intensive than that for WAF preparation (SL Ross Environmental Research Ltd., 2005; Özhan et al., 2014). It, therefore, requires a longer settling time to reduce the oil content in decant waters. For example, the total petroleum hydrocarbons (TPHs) in WAF were 7.2–43 mg/L (Faksness et al., 2008; Özhan et al., 2014), which was similar to the oil concentration of the decant water after settling for 24 h (SL Ross Environmental Research Ltd., 2005).
The composition of WAFs is dependent on the solubility and abundance of oil compounds in different oils. Faksness et al. (2008) compared the concentration of TPHs, BTEX (benzene, toluene, ethylbenzene, and xylenes), phenols, and PAHs in WAF using different crude and refined oils. For instance, light condensate (0.761 g/ml) had the highest concentration of mono-aromatic hydrocarbons (BTEX + C3-benzenes) but the lowest concentrations of two to three and four to six ring PAHs, while a heavy fluid oil contained low concentrations of aromatics. BTEX (0.24–68.6 ppm), and naphthalene and alkylated naphthalene (0.04–0.27 ppm) in WAF were correlated to those in oil samples. Because of the high-water solubilities, naphthalene and alkylated naphthalene, and phenols (0.01–1.82 ppm) were the major semi-volatile compounds in WAF. Specific to each oil, Snøhvit condensate had the lowest density, and its WAF had the highest BTEX and phenol concentrations. Heavy oils such as Heidrun Åre, Grane, and Bunker oil had the lowest oil concentration in WAF, probably owing to the low content of soluble compounds. Bitumen natural-gas condensate blend (dilbit), as unconventional crude oil, had lower levels of TPHs, BTEX, naphthalenes, and fluorenes in WAF than those in conventional crude oils (Philibert et al., 2016). It was be noticed that, biodegradation of oil increased the concentration of TPH in WAF (Faksness et al., 2008).
The weathering of spilled oil, including dilution, evaporation, and biodegradation, affects the oil composition of WAF as well. The components with boiling points lower than C12 in Macondo oils can be quickly evaporated in 1–2 days after the spill (Faksness et al., 2015). Further weathering (>3 days) would reduce the oil and PAHs concentrations in WAF to less than one 10th of those prepared by the source oil, leaving only unresolved complex materials. Similar results were reported by Forth et al. (2017). Oil slick recovered in skimmer vessels had no BTEX, and most lighter PAHs were lost, while the total PAHs concentration was reduced by 68–85%. Nevertheless, weathered oil has substantial water-in-oil emulsification (Forth et al., 2017).
Volatile hydrocarbons in WAF, including low molecular weight (LMW) alkanes (C6-C9), monoaromatics, and 2-ring PAHs (naphthalene and alkylated naphthalene), can be intaken by invertebrates, fishes, mammals, birds, and reptiles (Dupuis & Ucan-Marin, 2015). These hydrophobic compounds penetrate the respiratory membrane and partition into cell membrane and nervous tissue, disrupting the function of central nervous systems (Lee et al., 2015). The narcotic effect can result in the mortality of species in the short-term exposure to WSF (Dupuis & Ucan-Marin, 2015). n-alkanes are considered less toxic in the marine environment owing to their high biodegradability. Cyclo-alkanes and iso-alkanes are more resistant to biodegradation than n-alkanes at the same molecular weight (Head et al., 2006). In addition, cyclo-alkanes deform cell membrane by partition.
Aromatic hydrocarbons are recognized as the main toxic substances in the WSF. Monoaromatics (BTEX) are volatile and most soluble, thus having the highest contribution to the acute toxicity of WSF (Kang et al., 2014; Philibert et al., 2016). PAHs in WAF are mostly two to five rings (Faksness et al., 2008). PAHs are more persistent in the marine environment, leading to acute and chronic diseases and eventually mortality of marine species. Naphthalene and its homologs have similar acute toxicity to BTEX (Dupuis & Ucan-Marin, 2015). PAHs can activate the transcription of downstream genes such as the CYP1A gene, increasing the synthesis of CYP1A proteins, releasing reactive oxygen species, and oxidatively damaging organisms (Schlezinger et al., 1999; Wu et al., 2012). PAHs show cardiotoxic responses and developmental abnormalities in embryos and larvae fish (Colavecchia et al., 2006; Incardona et al., 2013). 3-5-ringed alkyl PAH is considered the main cause of embryotoxicity (Hodson, 2017). Benzo(a)pyrene is considered the most carcinogenic compound (Dupuis & Ucan-Marin, 2015). Some PAHs have carcinogenic endocrine-disrupting effects (Neff et al., 2011). The immunotoxicity responses of PAHs in different species are observed (Reynaud & Deschaux, 2006). PAHs under irradiation promote the generation of oxidative species and damage the tissue (Barron, 2017). Phototoxicity has shown a significant enhancement in crude oil toxicity to fish. For certain PAHs that can absorb solar radiation spectrum (fluoranthene and anthracene), the increase in toxicity can approach 100-fold.
The toxicities of different WAFs are summarized in Table 1. Generally, light oil WAF is more toxic than heavy oil. Heavy fuel oil WAF was nontoxic to fish owing to its low to no bioavailability. Once the fish exposures to oil, the toxicity of heavy fuel oil WAF was higher than other crude oils because of the high concentrations of alkyl PAHs (Martin et al., 2014). Philibert et al. (2016) observed similar toxicity of conventional crude oils and dilbit to the survival and pericardial edema of zebrafish embryos. Because the WAFs have similar levels of monoaromatic hydrocarbon content. Di Toro et al. (2007) suggested that oil weathering could significantly reduce the more bioavailable, lower-molecular-weight, and higher toxic fractions, thus decreasing the toxicity of WAF.
Toxicity endpoints and subsequent ecological impacts of oil fractions in water on various marine species (i.e. plants, invertebrates, fishes, reptiles and amphibians, birds, and mammals) have been comprehensively reviewed by many researchers (National Research Council, 2005; Perhar & Arhonditsis, 2014; Lee et al., 2015; Hodson, 2017; Liu et al., 2022). Generally, toxicity is considered either acute toxicity (<96 h) or chronic toxicity (>96 h) based on the exposure duration and bioaccumulation potential. Commonly applied indicators include LC50 (concentration of a chemical that kills 50% of the total population), EC50 (concentration of a chemical that affects 50% of the total population), and IC50 (concentration of a chemical inhibits 50% of biological activity) (Lee et al., 2015; Walton et al., 2021). Some studies use a more stringent term, like EC10, to evaluate the toxicity of oil fractions to marine species (Brakstad et al., 2018; Sørensen et al., 2019). Terms of lowest observed effect concentration (LOEC) and no observed effect concentration (NOEC) are used to describe chronic toxicity (Brand et al., 2001; Barron et al., 2013). The ecotoxic effects and endpoints of different oils and components in WAF are summarized in Table 1 and Supplementary Table S1, respectively.
The Vibrio fischeri test is a well-established toxicity test that has been widely applied in the evaluation of the acute and chronic ecotoxicity of WSF (Wells et al., 1997; Perkins et al., 2003; Brakstad et al., 2018). A concentration of a chemical in water resulting in a 50% decrease in the bioluminescence of the target bacterium at a certain time (5–15 min) is used as an indicator for the toxicity assessment (King et al., 2014). EC50 values of oil WSF ranged from 1.08 to 17.18 mg/L, depending on the composition of oils (Hokstad et al., 2000; Rhoton et al., 2001; Fuller et al., 2004; Reá tegui-Zirena et al., 2014). Normally, aromatics in WSF have a much higher toxicity than saturates. The acute toxicity tests (15 min) of individual compounds showed that the monocyclic aromatics (e.g., benzene, toluene and ethylbenzene), had a EC50 value of 13.8 mg/L; Xylene was ranging from 4.23 to 2.63 mg/L depending on the structure; PAHs with increasing aromatic rings had a declined trend of EC50, in which most of the PAHs were less than 0.5 mg/L (Lee et al., 2013). The toxicity of individual naphthenic acids was tested by Jones et al. (2011). The results showed a toxicity range of 0.004–0.7 mM, in which the bicyclic acids (e.g., 3-decalin-1-yl propanoic acid) were considered as the most toxic among the naphthenic acids. Their toxic effect to V. fisheri was found to be nonpolar narcosis, with an EC50 value of 46 mg/L (Swigert et al., 2015). Mirjani et al. (2021) used Aliivibrio fischeri to evaluate the acute toxicity of a crude oil WSF in 6 h. The authors suggested that the longer time evaluation may be more accurate for the toxicity analysis, as the low assimilation rate of petroleum compounds may delay the toxicologic response. The EC10 and EC50 values of TPH were 0.03 mg/L and 1.77 mg/L, respectively (Mirjani et al., 2021).
Decant water plumes can transport material vertically to the water column and may interact with sediment in nearshore waters, where WSF can form oil particulate aggregates or be absorbed on the sediment (SL Ross Environmental Research Ltd., 2005; Yang et al., 2018). In this instance, benthic invertebrates, such as crustaceans, can be directly affected.
Mysid shrimp, an estuarine crustacean found in the Atlantic, is one of the most common species for both acute and chronic toxicity tests relevant to marine estuarine organisms (USEPA, 2009). Mysid shrimp is considered to be more toxicologically sensitive than other species (Roast et al., 1998; Verslycke et al., 2004). Perkins et al. (2003) assessed the toxicity of volatile organics (C6-C9, including BTEX) and petroleum hydrocarbon fractions (C10-C36) of Alaska North Slope crude oil using mysid shrimp. Their analysis showed that mysid shrimp had a high tolerance to volatile organics (LC50 = 7.87 mg/L) but relatively low tolerance to C10-C36 (LC50 = 1.79 mg/L). They further examined the toxicity of different compounds to tanner crab larvae, showing that tanner crab larvae were less sensitive than mysid shrimp to volatiles (13.37 mg/L) but was more sensitive to C10-C36 (LC50 = 0.408 mg/L). Phenol has a chronic lethal effect on opossum shrimp (Duan et al., 2018). The LC50 (96 h) was 0.26 mg/L (Kim & Chin, 1995).
Zooplankton, a key marine food source, showed a population decrease in the presence of WSF after 16 h (Jiang et al., 2010; Almeda et al., 2013). Jiang et al. (2010) summarized the toxicity of oil WSF, BTEX, PAHs and alkyl PAHs on different phytoplankton species after exposure for 72 h. Their data discovered that oil WSF had a lower toxicity on phytoplankton (EC50 > 10 mg/L), and heavy oil WSF was less toxic than fuel oil and marine oil. PAHs had a toxicity range from 7.30 mg/L to 18 μg/L, depending on the species and specific chemicals under consideration. For the marine copepod, fluoranthene and pyrene had higher toxicity than anthracene and naphthalene, and alkyl PAHs were more toxic than the parent PAHs. The phototoxicity of PAHs increases the lethality rate for mesozooplankton (Almeda et al., 2013).
Sublethal effects of PAHs on zooplankton have also been studied. Bioaccumulation of PAHs can cause changes in cell size and growth rate, and result in hormone-disruption (Saiz et al., 2009; Almeda et al., 2013). The development of copepod (Amphiascus tenuiremis) was affected by the exposure to WAF of South Louisiana crude oil. The maturity of copepod was delayed for 1.9–2.2 days in the 50% WAFs (Total PAHs = 439 μg/L) (Bejarano et al., 2006).
SL Ross Environmental Research Ltd. (2005) summarized the acute toxicity of oil on crustacean species, including shrimps, lobster, and crabs. The EC50 values at 96 h were found to range from 0.54 to 49 mg/L depending on the subject species, stages of growth, and selected oil types. Saco-Álvarez et al. (2008) investigated the acute toxicity of marine fuel oil on marine invertebrates, including mussels, sea urchins, and copepods. Undiluted marine fuel WAF has low toxicity to mussel embryos, while similar impacts were observed on sea urchins and copepods. However, the toxicity of the WSF was significantly increased under irradiation. The EC50 value of WSF dilution to mussel was 20%, demonstrating the enhancing effects of phototoxicity. It could attribute to the presence of PAHs in WSF (Total PAHs = 42 nM) (Saco-Álvarez et al., 2008). Overall, this study found that test invertebrates were more sensitive to oil than fish samples, especially under irradiation (Saco-Álvarez et al., 2008).
Field studies on the Deepwater Horizon (DWH) spill suggested that invertebrates in real-world conditions had a higher tolerance to WSF compared with those in laboratory studies. This was because the WSF concentration was more unstable and sensitive to the marine environment (Hodson et al., 2019). For example, the growth rate of shrimps, and the bioaccumulation effects in mussels and oysters were either not observed or directly linked to the oil spill. Further investigations based on species sensitivity distributions suggested that the sensitivity of invertebrates was not consistent as with that of fish species (Barron et al., 2013).
The major components of oil that present an acute lethal hazard to fish populations are the low molecular weight (LMW) petroleum hydrocarbons (i.e. monoaromatics, diaromatics, and short-chain alkanes), which rapidly penetrate lipid membranes by narcosis (Gardiner et al., 2013). However, the duration in which exposure to these compounds can occur is brief and localized due to weathering processes (Lee et al., 2015). High molecular weight compounds, such as PAHs with three or more rings, may be slowly taken up by the fish’s lipids causing chronic and sublethal effects. Fishes in the early development stages are particularly sensitive to PAHs, which could alter the organization of tissue along the dorsoventral axis and the development of the heart (Honda & Suzuki, 2020). For example, newly hatched haddock larvae showed morphological defects after 7 days of WSF exposure (Sørensen et al., 2017). Fish embryo and yolk sac larvae can be affected by the total PAH concentrations higher than 0.1 μg/L, at which point cardiac defect, bone deformity, slow development, and mortality can be observed (Hodson, 2017). Adult Achirus lineatus accumulates PAHs metabolites in bile and gut when exposing a sublethal level of WAF (Améndola-Pimenta et al., 2020). Fatty degeneration, inflammation, and structural disintegration were found in livers, spleen, and kidneys, respectively.
The toxicity of oil WAF to fish at different development stages was extensively reviewed by Lee et al. (2015). They summarized the acute and chronic toxicity thresholds (LC50 or EC50) of TPAHs and TPH for fish based on existing literature (Figure 2). It was shown that fish was able to survive in higher concentrations of oil WAF and TPAHs, however, they would widely develop sublethal diseases, particularly impacting recruitment and productivity in lower WAF concentration. TPAHs are roughly 100 to 1,000 times more toxic than TPHs. In addition, Pacific halibut juvenile has a LOEC of 1.6 mg oil (Alaska North Slope weathered)/g sediment (Moles & Norcross, 1998). The 10-d LOEC of PAHs in the Alaska North Slope to the embryo of pink salmon is 25–540 μg/L (Birtwell et al., 1999). The NOEC of PAHs in Alaska North Slope to the embryo of Pacific herding is 17 μg/L for 96 h exposure (Barron et al., 2013).
FIGURE 2. Lethal and sublethal concentrations of TPAH and TPH for fish subjected to acute or chronic exposure to oil WAF (Lee et al., 2015).
Hodson (2017) indicated that the chronic toxicity of PAHs to fish embryos was related to the molecular size and octanol-water partition coefficients (Kow) of each compound. The chronic EC50s for individual PAHs to various fish species were found to range from 0.3–100 μg/L, among which 3-5-ringed alkyl PAH had the lowest values. Jasperse et al. (2019) pointed out that a 14-day exposure to HEWAF increased liver somatic indices of Sheepshead minnow, and decreased fertilization. The decrease in egg production and fertilization rate was correlated with the PAHs bioaccumulation. The second and third generations of affected Sheepshead minnow decreased in weight, length, and prey capture, suggesting a transgenerational effect. Through the exposure to different oils, the gene expressions of the second generation were altered, which further affected the biotransformation, cardiac development, and neurodevelopment (Philibert et al., 2021).
Although oil can reduce the thermal insulation of mammals by fur oiling, and then lead to possible mortality, the short-term exposure to oil sheen did not have a lethal effect (Geraci & Smith, 1976). However, oil spills may affect the long-term population of mammals. For example, a loss of 33–41% of killer whales was observed in 18-months after the Exxon-Valdez oil spill, while only a loss of 2.5% was found in the unaffected area (Matkin et al., 2008). The inhalation, aspiration, and ingestion of oil would elevate the concentration of oil in the respiratory tract, gastrointestinal tract, and bloodstream, causing irritation and narcosis (National Academies of Sciences & Medicine, 2020). It may explain the occurrence and elevation of lung diseases and impaired stress on Barataria Bay dolphins after the Deepwater Horizon Spill (Schwacke et al., 2014; Smith et al., 2017). The inhalation of volatile organics was suggested to have caused the death of 302 harbor seals after the Exxon-Valdez oil spill (Peterson, 2001).
Wise et al. (2018) examined the cytotoxic and genotoxic effect of PAHs on sperm whale skin cells using WAF of Alaskan oil. After 24 h exposure, WAF showed a low level of genotoxic effect. A slight decrease in cell survival (8.5%) was found only in 20% of Alaskan WAF (Total PAH = 197.4 μg/L). Reynaud and Deschaux (2006) reviewed the immunotoxicity responses of PAHs by mammalian models and suggested the possible mechanisms, which were the metabolic activation of PAHs by cytochrome P450, binding of aryl-hydrocarbon receptors, and intracellular calcium mobilization.
Similar to marine mammals, oil can expose to sea turtles through inhalation and ingestion (Frasier et al., 2020). PAH absorption was detected in oiled sea turtle’s intestines and tissues (Ylitalo et al., 2017). The concentrations of PAH in turtle esophagus were ranged from 0.01–0.36 mg/g. Oiled sea turtles had higher total PAHs than unoiled sea turtles. In terms of phenanthrene equivalent, the PAH metabolites in the bile of visibly oiled sea turtles were 10-fold more than those in the non-visible oiled sea turtles (Ylitalo et al., 2017). Some research suggested that sea turtles did not seem to avoid oil spills (Frasier et al., 2020). Adult loggerheads did not change the foraging sites after spills, suggesting the long-term exposure to oil (Vander Zanden et al., 2016). Ingestion of contaminated crustaceans and mollusks could increase the risk of oil exposure in the oil-affected region (Milton et al., 2003). Nesting in the oil-contaminated site impacts the turtles at the early life stages, which may alter the sex distribution of hatchlings (Ruberg et al., 2021). However, the long-term effects of oil exposure on sea turtles have not been well quantified (Vander Zanden et al., 2016).
The toxic effect of oil on algae, mangroves, and seagrass has been comprehensively reviewed by Lewis and Pryor (2013). In the review, the toxic effect concentrations to alga were 0.002-10,000 ppm for crude oil, while a narrower range was found to be 0.09–50 ppm for refined oil. Mangroves are sensitive to oil contamination which need approximately 50 years to recover (Lewis & Pryor, 2013). Crude oils appeared moderate acute toxicity (>1.0 ppm) to signal algal species. Bunker C oil WAF had low toxicity to a microalga (Tetraselmis tetrathele) (Santander-Avanceña et al., 2016). Low concentrations of WSF (100–300 mg/L) in seawater resulted in minor damage to phytoplankton, and on some occasions, even promoted the algae growth (Ohwada et al., 2003). All of the PAH concentrations in WAF were lower than EC50 or NOEC, suggesting no inhibition of Tetraselmis tetrathele growth. Arctic marine phytoplankton and copepods showed a significant reduction of biomass-specific primary production (>52%) and fecal pellet production (>51%) when exposure to WAF (oil content 0.28–0.55 mg/L) of heavy fuel oil for 40 h (Lemcke et al., 2019). In a high WAF, a further reduction of 71–91% in primary production was occurring in light irradiation, indicating the phototoxic effect. A low concentration of phenol (<15 mg/L) promoted the growth of a marine diatom (Duan et al., 2018). On the contrary, the sub-lethal concentration (96 h EC50) of phenol using the same species was 13.66 mg/L (Duan et al., 2017).
The Arctic ecosystem has relatively simple food webs, in which species are characterized by slower metabolic rates, longer lifespan, and higher lipid content (Chapman and Riddle, 2005; Gardiner et al., 2013; Camus et al., 2015). Therefore, Arctic species may have different responses to oil toxicity compared to temperate species.
Gardiner et al. (2013) selected three Arctic species, Calanoid copepods, Arctic cod, and Sculpin, to investigate the toxicity of dispersed crude oil under Arctic conditions. The results indicated that cupboards were not affected by WAF (TPH below 1.0 mg/L). But wave and chemical-enhanced WAF has elevated TPH and PAHs levels in seawater, leading to acute toxicity. The 96 h LC50 values of WAF to cod and sculpin were 1.6 and 2.3 mg TPH/L, respectively. It was also found that the parent PAHs, especially naphthalene, were statistically correlated to acute toxicity. Hansen et al. (2011) compared an Arctic copepod with its temperate relative. The results showed lower sensitivity in the Arctic copepod which had a delayed response (toxic effect and mortality) to oil, especially for those that had high lipid content. Camus et al. (2015) investigated differing responses between the Arctic and temperate species from low to high trophic levels to the toxicity of artificial oil-contaminated water. Algae and Arctic fishes were more sensitive than temperate ones, while copepod and shrimp were less sensitive.
Oil and water separation can remove most large oil droplets from decant water. However, small oil droplets that present as oil/water emulsions are not easily removed during decanting. Although many studies have indicated that the WSF in decant water is more toxic, the emulsified oil droplets may also be a source of toxicity to exposed marine organisms (Gonzá lez-Doncel et al., 2008; Lee et al., 2015). Dispersion of oil can further increase the diffusion and bioavailability of oil droplets (Redman, 2015). The less dissolvable fractions of oil, such as PAHs, showed a much higher concentration in dispersed oil than in WSF (Echols et al., 2015; Hansen et al., 2015). Thus, dispersion increases the potential for uptake of these compounds by marine species (e.g., zooplankton) and subsequently the food web (Almeda et al., 2013). Other research indicated that fish were more sensitive to dispersed oil as oil adhered to the chorion of embryos (Sørhus et al., 2015).
Echols et al. (2015) collected water samples from exposure sites in the Gulf of Mexico after the DWH spill and evaluated the acute toxicities of WSF and dispersed oil in water. They indicated that the maximum TPAHs in oil in water dispersion (OWD) was ten-fold higher than that of WSF. The toxicity of SWFs was less than that from OWD to Atlantic Silverside fish, but not with mysid shrimp. Mysid shrimp was more sensitive than Silverside fish in mortality tests, but overall acute toxicity was minimal. They also found that the level of PAHs did not show a clear trend of acute toxicity to the Microtox® assays.
Chronic effects of dispersed oil should also be considered. Hansen et al. (2015) investigated egg production of copepods exposed to mechanically dispersed oil (0.2–5.5 mg/L) for 96 h. The results indicated that PAH levels were elevated in exposed copepods, in which proportions of naphthalene and two to three ring PAHs were dominant. This resulted in suppressing egg production 15 days after the exposure. The production rate recovered after the initial exposure to the mechanically dispersed oil at a high level (16.5 mg/L), indicating a temporary effect. In contrast, oil droplets were found to adhere to the chorion of haddock embryos, directly enhancing the uptake of PAHs and other petroleum hydrocarbons, consequently increasing the probability and severity of chronic toxicity (Sørhus et al., 2015). This phenomenon was not seen in Atlantic cod, however, whose chorion was not fouled by oil droplets (Sørensen et al., 2017). It is not clear on the physical-chemical mechanism of oil droplet fouling.
Oil droplet size affects oil toxicity to aquatic species via feeding apparatus coating and oil consumption (Bejarano et al., 2014; Uttieri et al., 2019). Olsvik et al. (2011) indicated oil droplets (<20–50 µm) could be ingested by copepods, and subsequently consumed by cod larvae, leading to bioaccumulation of PAHs, as well as CYP1A response. However, the effect was moderate on the gene transcription when compared with the effect of WSF.
Demulsifier is a chemical additive that is commonly applied to accelerate the separation of water in oil and oil in water emulsions. SL Ross Environmental Research Ltd. (2005) and Henderson et al. (1999) summarized the acute toxicity of several demulsifiers used in oil spill response and offshore oil and gas production. The acute toxicities (96 h EC50) of different demulsifiers on rainbow trout and brine shrimp ranged from 28 to 10,000 ppm. Henderson et al. (1999) evaluated the acute toxicity of three demulsifiers that were used for oil recovery enhancement. The 96 h-EC50 values to Vibrio fischeri ranged from 1–112 ppm, in which the blend of ionic and non-ionic demulsifier had the lowest toxicity. Meanwhile, demulsifiers are more water-soluble than WAF. Their concentrations in decant water were in the 100–1,000 ppm range, which sometimes exceeded the toxicity endpoints of demulsifiers (SL Ross Environmental Research Ltd., 2005). To reduce ecological impacts, more studies are focused on the development and application of demulsifiers with low toxicity. For example, novel demulsifiers produced from biogenic materials have EC50 values (Vibrio fischeri) more than 500 ppm (Cai et al., 2019; Cho et al., 2021; Zhu et al., 2022b). The key surface-active ingredients, such as dioctyl sodium sulfosuccinate, can be rapidly degraded (Cao et al., 2022b).
The interfacial active components in demulsifiers can not only enlarge the oil droplets that promote separation but also increase the solubility of oil in water. A double of the oil content in decant water was observed in the presence of demulsifiers (SL Ross Environmental Research Ltd., 2005). The increase of bioavailable oil could further increase oil toxicity. For example, the oil-treated by demulsifiers had increased toxicity to crustaceans when compared with untreated oil (Tamis et al., 2012). On the contrary, the addition of surfactant enriched cell abundance and increased the oil biodegradation in high salinities (Cao et al., 2022a).
Oxidation intermediates are expected during oil weathering and oily wastewater treatment. The primary oxidation products of crude oil in water are aliphatic and aromatic acids, as well as alcohols and phenols when under lower oxidation (Hansen, 1975; Zhu et al., 2022a). For example, during an advanced oxidation treatment, organic pollutants, such as PAHs and BTEX, undergo a series of oxidation and spontaneous transformation reactions. Parent PAHs and BTEX are more likely oxidized to form phenols and quinones, which are sometimes more toxic than their parent compounds (Shu & Huang, 1995; Lundstedt et al., 2007). Further oxidation results in ring cleavage and the release of ketones, aldehydes, and carboxylic acids, which are more biodegradable than aromatics (Liu et al., 2021). Developments in more efficient oxidation processes can promote the mineralization of oil and control the discharge of toxic intermediates into the environment (Dong et al., 2022).
The numerical modeling of oil toxicity of marine species can be used to predict ecological impacts of decant water. The composition of WAF prepared by different oils has been examined by different researchers (Faksness et al., 2008; Barron et al., 2020). The major composition of WAF can be determined by the water solubility of individual compounds (Faksness et al., 2008). The concentration of oil components, especially PAHs in the water phase can be calculated by Raoult’s law and the Setschenow equation (Kang et al., 2014). In addition, the release of oil components into water depends on the contact time and temperature. Oil with high viscosity/pour point requires longer than to reach a partition equilibrium. Barron et al. (2020) tested the concentration of alkanes and PAHs in the WAF prepared with three unweathered oils (0.87–0.91 g/cm3). A similar pattern of PAHs and alkanes among the three oils resulted in similar acute toxicity. All these results can be used to predict the composition of oil in decant water.
The toxicity of WAF in decant water can be predicted by the individual compounds. The PETROTOX model can be used to predict the acute and chronic toxicity of petroleum substances based on their Kow. The acute toxicity of hydrocarbons to aquatic species can be estimated by multiplying the concentration of compounds in water and their Kow and the LC50 is approximately 5 mM of tissue lipid (Lee et al., 2015). For multiple compound exposure, the acute and chronic toxicity of a substance, namely toxic unit, is normalized by the ratio of individual chemical concentration to its LC50 and EC10, respectively. The acute toxicity of 50% response and the limited chronic effects can be predicted when the sum of toxic unit equals to 1. For example, Kang et al. (2014) predicted the overall toxicity by summing up the individual contributions of BTEX, PAHs, and alkylated PAHs to the narcosis of Vibrio fischeri. The contribution of individual compounds was the ratio of concentration to EC50. The results revealed that BTEX and alkylated PAHs had the highest contribution to the acute toxicity, while 16 priority PAHs have only a 2% contribution. Liu et al. (2021) applied a multivariable regression model to evaluate the toxicity contribution of aromatics and degradation intermediates to produced water, indicating the major toxicity contribution was from phenols and followed by bromoform and PAHs. The phototoxicity of individual PAHs can be predicted using a phototoxic target lipid model, which quantifies the phototoxicity by different PAHs, species sensitivities, light sources, and irradiation time (Marzooghi et al., 2017). The removal of aromatics can significantly reduce acute toxicity (Liu et al., 2021; Ferreira et al., 2022).
The ecological impacts of decant water can be assessed using a framework for the ecological assessment of potentially toxic substances (Environment and Climate Change Canada, 2007). The framework helps assess the ecological impacts in a defined region, using the ratio of the predicted environmental concentrations of hazards to their predicted no-effect concentration in the region. The predicted environmental concentration of an observation point is the division of the hazard concentration in decant water to a weathering factor. It decreases with the increase of the distance from the discharge point. The predicted no-effect concentration is the lowest LC50/EC50 or NOEC values of marine species of the region divided by an assessment factor (10–1,000). The assessment factor was varied in the types of endpoints and available data.
Discharge of decant water is restricted by many authorities. One of the major concerns prohibiting the process was the consequent ecological impacts. To date, the ecological impacts of decant water have not yet been comprehensively studied. On the contrary, the ecotoxicity of WAF, which has a similar generation process as decanting, has been extensively studied. A thorough understanding of the ecotoxicity of WAF is needed to assess the acute and chronic toxicities of decant water and the potential environmental impacts on the marine ecosystem.
Monoaromatics, low molecular weight saturates and PAHs, and phenols were dominated in WAFs. Oil fractions with low molecular weights would cause narcosis and the deformation of cell membranes in a short-time of exposure. PAHs can lead to long-term effects, including cardiotoxicity, developmental abnormality, immunotoxicity, hormone disruption, phototoxicity decreased fertilization and transgenerational effects. The toxicity of WAF varied in different oil compositions and the degrees of weathering. Reduced toxicity can be found in heavy oil and highly weathered oil.
The ecological endpoints of WAF were different in species. The acute EC50s of WSF to bacterium are 1.08–17.18 mg/L, varying from oil to oil. The LC50s to invertebrates, fishes, and plants are 0.54–49 mg/L, 0.03–5.5 mg/L, and 0.002–1,000 mg/L, respectively. Meanwhile, most of the EC50s of PAHs were less than 0.5 mg/L. The acute LC50s of PAHs to invertebrates are 18 µg/L-7.30 mg/L. The chronic LC50s of PAHs to fishes are 0.015–100 μg/L, in which alkyl PAHs have the highest toxicity. In addition to dissolved oil, oil droplets in dispersed oil increase the exposure of PAHs to aquatic species. Demulsifiers have a high partition in water, sometimes more than their EC50.
Although the ecotoxicity of oil has been extensively studied, the chronic effects of oil and PAHs on population, trans-generation, and interspecies are less addressed. The chronic effects of oil droplets need to be further investigated. There is also an urgent need for the assessment and quantification of WAF toxicity to marine mammals and turtles, as most of the studies were based on the statistical changes in population before and after oil spills.
Currently, there are no standard methods of decant water preparation for ecotoxicological studies. Oil types, oil weathering, and decanting methods would affect the composition of decant water. Due to these variations, it is necessary to expand the chemicals of interest and study the changes of environmental impacts caused by the different decant water compositions. The toxicity studies on alkylated PAHs are much less than those on the priority PAHs, although some studies already showed a higher concentration of alkyl PAHs in WAF and possible higher toxicity. The metabolites of PAHs, which sometimes show increased toxicity, and the compounds generated from oil weathering (e.g., phenols, naphthenic acids) should be included as well. Quantitative structure activity relationship modeling can help simulate the aquatic toxicity of compounds by their physical/chemical properties. Furthermore, the interactive toxic effects, such as oil and demulsifiers, oil and treatment intermediates, are needed to predict and avoid the negative ecological impacts of decant water.
The original contributions presented in the study are included in the article, further inquiries can be directed to the corresponding author.
BL: Conceptualization, Methodology, Writing—original draft preparation, Visualization. BC: Conceptualization, Methodology, Writing—review, and editing. JL: Writing—original draft preparation. XY: Writing—original draft preparation. GD: Writing—original draft preparation, Visualization. EM: Writing—original draft preparation. BZ: Supervision, Writing—review, and editing.
This work is supported by Fisheries and Oceans Canada (DFO) under the Multi-Partner Research Initiative (MPRI) program, the Natural Science and Engineering Research Council of Canada (NSERC), and the Canada Foundation for Innovation (CFI).
The authors declare that the research was conducted in the absence of any commercial or financial relationships that could be construed as a potential conflict of interest.
All claims expressed in this article are solely those of the authors and do not necessarily represent those of their affiliated organizations, or those of the publisher, the editors, and the reviewers. Any product that may be evaluated in this article, or claim that may be made by its manufacturer, is not guaranteed or endorsed by the publisher.
We thank the Memorial University of Newfoundland for the support.
The Supplementary Material for this article can be found online at: https://www.frontiersin.org/articles/10.3389/fenvs.2022.944010/full#supplementary-material
Almeda, R., Wambaugh, Z., Wang, Z., Hyatt, C., Liu, Z., and Buskey, E. J. (2013). Interactions between Zooplankton and Crude Oil: Toxic Effects and Bioaccumulation of Polycyclic Aromatic Hydrocarbons. PLoS One 8 (6), e67212. doi:10.1371/journal.pone.0067212
Améndola-Pimenta, M., Cerqueda-García, D., Zamora-Briseño, J. A., Couoh-Puga, D., Montero-Muñoz, J., Árcega-Cabrera, F., et al. (2020). Toxicity Evaluation and Microbiota Response of the Lined Sole Achirus Lineatus (Chordata: Achiridae) Exposed to the Light Petroleum Water-Accommodated Fraction (WAF). J. Toxicol. Environ. Health, Part A 83 (8), 313–329. doi:10.1080/15287394.2020.1758861
Aurand, D., and Coelho, G. (2005). “Cooperative Aquatic Toxicity Testing of Dispersed Oil and the Chemical Response to Oil Spills: Ecological Effects Research Forum (CROSERF),”. Tech. Report, 07–03
Barron, M. G., Bejarano, A. C., Conmy, R. N., Sundaravadivelu, D., and Meyer, P. (2020). Toxicity of Oil Spill Response Agents and Crude Oils to Five Aquatic Test Species. Mar. Pollut. Bull. 153, 110954. doi:10.1016/j.marpolbul.2020.110954
Barron, M. G., Hemmer, M. J., and Jackson, C. R. (2013). Development of Aquatic Toxicity Benchmarks for Oil Products Using Species Sensitivity Distributions. Integr. Environ. Assess. Manag. 9 (4), 610–615. doi:10.1002/ieam.1420
Barron, M. G. (2017). Photoenhanced Toxicity of Petroleum to Aquatic Invertebrates and Fish. Arch. Environ. Contam. Toxicol. 73 (1), 40–46. doi:10.1007/s00244-016-0360-y
Bejarano, A. C., Chandler, G. T., He, L., and Coull, B. C. (2006). Individual to Population Level Effects of South Louisiana Crude Oil Water Accommodated Hydrocarbon Fraction (WAF) on a Marine Meiobenthic Copepod. J. Exp. Mar. Biol. Ecol. 332 (1), 49–59. doi:10.1016/j.jembe.2005.11.006
Bejarano, A. C., Clark, J. R., and Coelho, G. M. (2014). Issues and Challenges with Oil Toxicity Data and Implications for Their Use in Decision Making: A Quantitative Review. Environ. Toxicol. Chem. 33 (4), 732–742. doi:10.1002/etc.2501
Birtwell, I. K., Fink, R., Brand, D., Alexander, R., and McAllister, C. D. (1999). Survival of Pink Salmon (Oncorhynchus gorbuscha) Fry to Adulthood Following a 10-day Exposure to the Aromatic Hydrocarbon Water-Soluble Fraction of Crude Oil and Release to the Pacific Ocean. Can. J. Fish. Aquat. Sci. 56 (11), 2087–2098. doi:10.1139/f99-134
Brakstad, O. G., Størseth, T. R., Rønsberg, M. U., and Hansen, B. H. (2018). Biodegradation-mediated Alterations in Acute Toxicity of Water-Accommodated Fraction and Single Crude Oil Components in Cold Seawater. Chemosphere 204, 87–91. doi:10.1016/j.chemosphere.2018.04.032
Brand, D. G., Fink, R., Bengeyfield, W., Birtwell, I. K., and McAllister, C. D. (2001). Salt Water-Acclimated Pink Salmon Fry (Oncorhynchus gorbuscha) Develop Stress-Related Visceral Lesions after 10-Day Exposure to Sublethal Concentrations of the Water-Soluble Fraction of North Slope Crude Oil. Toxicol. Pathol. 29 (5), 574–584. doi:10.1080/019262301317226384
Buchholz, K., Krieger, A., Joeckel, J., Reiter, G., Walker, A. H., Etkin, D. S., et al. (2016). Oil Spill Response Equipment Capabilities Analysis (Volume II). Washington, D.C.: U.S. Department of the Interior Bureau of Safety and Environmental Enforcement.
Cai, Q., Zhu, Z., Chen, B., and Zhang, B. (2019). Oil-in-water Emulsion Breaking Marine Bacteria for Demulsifying Oily Wastewater. Water Res. 149, 292–301. doi:10.1016/j.watres.2018.11.023
Camus, L., Brooks, S., Geraudie, P., Hjorth, M., Nahrgang, J., Olsen, G. H., et al. (2015). Comparison of Produced Water Toxicity to Arctic and Temperate Species. Ecotoxicol. Environ. Safety 113, 248–258. doi:10.1016/j.ecoenv.2014.12.007
Cao, Y., Kang, Q., Zhang, B., Zhu, Z., Dong, G., Cai, Q., et al. (2022a). Machine Learning-Aided Causal Inference for Unraveling Chemical Dispersant and Salinity Effects on Crude Oil Biodegradation. Bioresour. Technol. 345, 126468. doi:10.1016/j.biortech.2021.126468
Cao, Y., Zhang, B., Greer, C. W., Lee, K., Cai, Q., Song, X., et al. (2022b). Metagenomic and Metatranscriptomic Responses of Chemical Dispersant Application during a Marine Dilbit Spill. Appl. Environ. Microbiol. 88 (5), e02151-21. doi:10.1128/aem.02151-21
Chand, P., Dutta, S., and Mukherji, S. (2022). Characterization and Biodegradability Assessment of Water-Soluble Fraction of Oily Sludge Using Stir Bar Sorptive Extraction and GCxGC-TOF MS. Environ. Pollut. 304, 119177. doi:10.1016/j.envpol.2022.119177
Chapman, P. M., and Riddle, M. J. (2005). Toxic Effects of Contaminants in Polar Marine Environments. Environ. Sci. Technol. 39 (9), 200A–206A. doi:10.1021/es0532537
Cho, C.-W., Pham, T. P. T., Zhao, Y., Stolte, S., and Yun, Y.-S. (2021). Review of the Toxic Effects of Ionic Liquids. Sci. Total Environ. 786, 147309. doi:10.1016/j.scitotenv.2021.147309
Colavecchia, M. V., Hodson, P. V., and Parrott, J. L. (2006). CYP1A Induction and Blue Sac Disease in Early Life Stages of White Suckers (Catostomus commersoni) Exposed to Oil Sands. J. Toxicol. Environ. Health, Part A 69 (10), 967–994. doi:10.1080/15287390500362154
DFO (2017). A Framework for the Development of Standard Methods to Evaluate the Toxicity of Petroleum Hydrocarbons on Aquatic Organisms. Fisheries and Oceans Canada. Available at https://publications.gc.ca/collections/collection_2018/mpo-dfo/fs70-6/Fs70-6-2017-053-eng.pdf.
Di Toro, D. M., McGrath, J. A., and Stubblefield, W. A. (2007). Predicting the Toxicity of Neat and Weathered Crude Oil: Toxic Potential and the Toxicity of Saturated Mixtures. Environ. Toxicol. Chem. 26 (1), 24–36. doi:10.1897/06174R.1
Dong, G., Chen, B., Liu, B., Hounjet, L. J., Cao, Y., Stoyanov, S. R., et al. (2022). Advanced Oxidation Processes in Microreactors for Water and Wastewater Treatment: Development, Challenges, and Opportunities. Water Res. 211, 118047. doi:10.1016/j.watres.2022.118047
Duan, W., Meng, F., Cui, H., Lin, Y., Wang, G., and Wu, J. (2018). Ecotoxicity of Phenol and Cresols to Aquatic Organisms: A Review. Ecotoxicol. Environ. Saf. 157, 441–456. doi:10.1016/j.ecoenv.2018.03.089
Duan, W., Meng, F., Lin, Y., and Wang, G. (2017). Toxicological Effects of Phenol on Four Marine Microalgae. Environ. Toxicol. Pharmacol. 52, 170–176. doi:10.1016/j.etap.2017.04.006
Dupuis, A., and Ucan-Marin, F. (2015). A Literature Review on the Aquatic Toxicology of Petroleum Oil: An Overview of Oil Properties and Effects to Aquatic Biota. USA: Canadian Science Advisory Secretariat Vancouver, BC.
Echols, B. S., Smith, A. J., Gardinali, P. R., and Rand, G. M. (2015). Acute Aquatic Toxicity Studies of Gulf of Mexico Water Samples Collected Following the Deepwater Horizon Incident (May 12, 2010 to December 11, 2010). Chemosphere 120, 131–137. doi:10.1016/j.chemosphere.2014.06.048
Environment and Climate Change Canada (2007). Overview of the Ecological Assessment of Substances under the Canadian Environmental Protection Act, 1999. Ottawa, ON, Canada: Environment Canada.
Etkin, D. S., and Nedwed, T. J. (2021). Effectiveness of Mechanical Recovery for Large Offshore Oil Spills. Mar. Pollut. Bull. 163, 111848. doi:10.1016/j.marpolbul.2020.111848
Faksness, L.-G., Altin, D., Nordtug, T., Daling, P. S., and Hansen, B. H. (2015). Chemical Comparison and Acute Toxicity of Water Accommodated Fraction (WAF) of Source and Field Collected Macondo Oils from the Deepwater Horizon Spill. Mar. Pollut. Bull. 91 (1), 222–229. doi:10.1016/j.marpolbul.2014.12.002
Faksness, L.-G., Brandvik, P. J., and Sydnes, L. K. (2008). Composition of the Water Accommodated Fractions as a Function of Exposure Times and Temperatures. Mar. Pollut. Bull. 56 (10), 1746–1754. doi:10.1016/j.marpolbul.2008.07.001
Ferreira, A. R., Breinholt, L., Kaarsholm, K. M. S., Sanchez, D. F., Chhetri, R. K., Muff, J., et al. (2022). Feasibility Study on Produced Water Oxidation as a Pretreatment at Offshore Platform. Process Saf. Environ. Prot. 160, 255–264. doi:10.1016/j.psep.2022.02.008
Forth, H. P., Mitchelmore, C. L., Morris, J. M., and Lipton, J. (2017). Characterization of Oil and Water Accommodated Fractions Used to Conduct Aquatic Toxicity Testing in Support of theDeepwater Horizonoil Spill Natural Resource Damage Assessment. Environ. Toxicol. Chem. 36 (6), 1450–1459. doi:10.1002/etc.3672
Frasier, K. E., Solsona-Berga, A., Stokes, L., and Hildebrand, J. A. (2020). “Impacts of the Deepwater Horizon Oil Spill on Marine Mammals and Sea Turtles,” in Deep Oil Spills, 431–462. doi:10.1007/978-3-030-11605-7_26
Fuller, C., Bonner, J., Page, C., Ernest, A., McDonald, T., and McDonald, S. (2004). Comparative Toxicity of Oil, Dispersant, and Oil Plus Dispersant to Several Marine Species. Environ. Toxicol. Chem. 23 (12), 2941. doi:10.1897/03-548.1
Gaaseidnes, K., and Turbeville, J. (1999). Separation of Oil and Water in Oil Spill Recovery Operations. Pure Appl. Chem. 71 (1), 95–101. doi:10.1351/pac199971010095
Gardiner, W. W., Word, J. Q., Word, J. D., Perkins, R. A., McFarlin, K. M., Hester, B. W., et al. (2013). The Acute Toxicity of Chemically and Physically Dispersed Crude Oil to Key Arctic Species under Arctic Conditions during the Open Water Season. Environ. Toxicol. Chem. 32 (10), 2284–2300. doi:10.1002/etc.2307
Geraci, J. R., and Smith, T. G. (1976). Direct and Indirect Effects of Oil on Ringed Seals (Phoca Hispida) of the Beaufort Sea. J. Fish. Res. Bd. Can. 33 (9), 1976–1984. doi:10.1139/f76-252
González-Doncel, M., González, L., Fernández-Torija, C., Navas, J. M., and Tarazona, J. V. (2008). Toxic Effects of an Oil Spill on Fish Early Life Stages May Not Be Exclusively Associated to PAHs: Studies with Prestige Oil and Medaka (Oryzias latipes). Aquat. Toxicol. 87 (4), 280. doi:10.1016/j.aquatox.2008.02.013
Hansen, B. H., Altin, D., Rørvik, S. F., Øverjordet, I. B., Olsen, A. J., and Nordtug, T. (2011). Comparative Study on Acute Effects of Water Accommodated Fractions of an Artificially Weathered Crude Oil on Calanus finmarchicus and Calanus glacialis (Crustacea: Copepoda). Sci. Total Environ. 409 (4), 704–709. doi:10.1016/j.scitotenv.2010.10.035
Hansen, B. H., Salaberria, I., Olsen, A. J., Read, K. E., Øverjordet, I. B., Hammer, K. M., et al. (2015). Reproduction Dynamics in Copepods Following Exposure to Chemically and Mechanically Dispersed Crude Oil. Environ. Sci. Technol. 49 (6), 3822–3829. doi:10.1021/es504903k
Hansen, H. P. (1975). Photochemical Degradation of Petroleum Hydrocarbon Surface Films on Seawater. Mar. Chem. 3 (3), 183–195. doi:10.1016/0304-4203(75)90001-8
Head, I. M., Jones, D. M., and Röling, W. F. M. (2006). Marine Microorganisms Make a Meal of Oil. Nat. Rev. Microbiol. 4 (3), 173–182. doi:10.1038/nrmicro1348
Henderson, S. B., Grigson, S. J. W., Johnson, P., and Roddie, B. D. (1999). Potential Impact of Production Chemicals on the Toxicity of Produced Water Discharges from North Sea Oil Platforms. Mar. Pollut. Bull. 38 (12), 1141–1151. doi:10.1016/s0025-326x(99)00144-7
Hodson, P. V., Adams, J., and Brown, R. S. (2019). Oil Toxicity Test Methods Must Be Improved. Environ. Toxicol. Chem. 38 (2), 302–311. doi:10.1002/etc.4303
Hodson, P. V. (2017). The Toxicity to Fish Embryos of PAH in Crude and Refined Oils. Arch. Environ. Contam. Toxicol. 73 (1), 12–18. doi:10.1007/s00244-016-0357-6
Hokstad, J. N., Faksness, L. G., Daling, P. S., and Buffagni, M. (2000). “Chemical and Toxicological Characterisation of Water Accommodated Fractions Relevant for Oil Spill Situations,” in SPE International Conference on Health, Safety and Environment in Oil and Gas Exploration and Production. doi:10.2118/61468-ms
Holth, T. F., Beylich, B. A., Skarphédinsdóttir, H., Liewenborg, B., Grung, M., and Hylland, K. (2009). Genotoxicity of Environmentally Relevant Concentrations of Water-Soluble Oil Components in Cod (Gadus morhua). Environ. Sci. Technol. 43 (9), 3329–3334. doi:10.1021/es803479p
Honda, M., and Suzuki, N. (2020). Toxicities of Polycyclic Aromatic Hydrocarbons for Aquatic Animals. Ijerph 17 (4), 1363. doi:10.3390/ijerph17041363
IMO (2012). Interpretations of, and Amendments to, MARPOL and Related Instruments. https://www.transportstyrelsen.se/contentassets/bba8fb5ccaac4c378fcedf299b6bddb2/64-7.pdf.
Incardona, J. P., Swarts, T. L., Edmunds, R. C., Linbo, T. L., Aquilina-Beck, A., Sloan, C. A., et al. (2013). Exxon Valdez to Deepwater Horizon: Comparable Toxicity of Both Crude Oils to Fish Early Life Stages. Aquat. Toxicol. 142-143, 303–316. doi:10.1016/j.aquatox.2013.08.011
IPIECA (2013). The Use of Decanting during Offshore Oil Spill Recovery Operations. London, United Kingdom: IPEICA.
ISCO (2015). Guidelines for Decanting Settled Out Water. http://www.spillcontrol.org/isco-what-s-new/338-guidelines-for-decanting-settled-out-water.
Jasperse, L., Levin, M., Rogers, K., Perkins, C., Bosker, T., Griffitt, R. J., et al. (2019). Transgenerational Effects of Polycyclic Aromatic Hydrocarbon Exposure on Sheepshead Minnows ( Cyprinodon variegatus ). Environ. Toxicol. Chem. 38 (3), 638–649. doi:10.1002/etc.4340
Jiang, Z., Huang, Y., Xu, X., Liao, Y., Shou, L., Liu, J., et al. (2010). Advance in the Toxic Effects of Petroleum Water Accommodated Fraction on Marine Plankton. Acta Ecol. Sin. 30 (1), 8–15. doi:10.1016/j.chnaes.2009.12.002
Jones, D., Scarlett, A. G., West, C. E., and Rowland, S. J. (2011). Toxicity of Individual Naphthenic Acids toVibrio Fischeri. Environ. Sci. Technol. 45 (22), 9776–9782. doi:10.1021/es201948j
Kang, H.-J., Lee, S.-Y., Roh, J.-Y., Yim, U. H., Shim, W. J., and Kwon, J.-H. (2014). Prediction of Ecotoxicity of Heavy Crude Oil: Contribution of Measured Components. Environ. Sci. Technol. 48 (5), 2962–2970. doi:10.1021/es404342k
Kim, J.-S., and Chin, P. (1995). Acute and Chronic Toxicity of Phenol to Mysid, Archaeomysis Kokuboi. Korean J. Fish. Aquatic Sci. 28 (1), 87–97.
King, S. M., Leaf, P. A., Olson, A. C., Ray, P. Z., and Tarr, M. A. (2014). Photolytic and Photocatalytic Degradation of Surface Oil from the Deepwater Horizon Spill. Chemosphere 95, 415–422. doi:10.1016/j.chemosphere.2013.09.060
Lee, K.-W., Shim, W. J., Yim, U. H., and Kang, J.-H. (2013). Acute and Chronic Toxicity Study of the Water Accommodated Fraction (WAF), Chemically Enhanced WAF (CEWAF) of Crude Oil and Dispersant in the Rock Pool Copepod Tigriopus Japonicus. Chemosphere 92 (9), 1161–1168. doi:10.1016/j.chemosphere.2013.01.080
Lee, K., Boufadel, M., Chen, B., Foght, J., Hodson, P., Swanson, S., et al. (2015). Expert Panel Report on the Behaviour and Environmental Impacts of Crude Oil Released into Aqueous Environments. Ottawa, ON, Canada: The Royal Society of Canada.
Lemcke, S., Holding, J., Møller, E. F., Thyrring, J., Gustavson, K., Juul-Pedersen, T., et al. (2019). Acute Oil Exposure Reduces Physiological Process Rates in Arctic Phyto- and Zooplankton. Ecotoxicology 28 (1), 26–36. doi:10.1007/s10646-018-1995-4
Lewis, M., and Pryor, R. (2013). Toxicities of Oils, Dispersants and Dispersed Oils to Algae and Aquatic Plants: Review and Database Value to Resource Sustainability. Environ. Pollut. 180, 345–367. doi:10.1016/j.envpol.2013.05.001
Li, P., Cai, Q., Lin, W., Chen, B., and Zhang, B. (2016). Offshore Oil Spill Response Practices and Emerging Challenges. Mar. Pollut. Bull. 110 (1), 6–27. doi:10.1016/j.marpolbul.2016.06.020
Liu, B., Chen, B., Ling, J., Matchinski, E. J., Dong, G., Ye, X., et al. (2022). Development of Advanced Oil/water Separation Technologies to Enhance the Effectiveness of Mechanical Oil Recovery Operations at Sea: Potential and Challenges. J. Hazard. Mater. doi:10.1016/j.jhazmat.2022.129340
Liu, B., Chen, B., Zhang, B., Song, X., Zeng, G., and Lee, K. (2021). Photocatalytic Ozonation of Offshore Produced Water by TiO2 Nanotube Arrays Coupled with UV-LED Irradiation. J. Hazard. Mater. 402, 123456. doi:10.1016/j.jhazmat.2020.123456
Lundstedt, S., White, P. A., Lemieux, C. L., Lynes, K. D., Lambert, I. B., Öberg, L., et al. (2007). Sources, Fate, and Toxic Hazards of Oxygenated Polycyclic Aromatic Hydrocarbons (PAHs) at PAH- Contaminated Sites. AMBIO A J. Hum. Environ. 36 (6), 475–485. doi:10.1579/0044-7447(2007)36[475:sfatho]2.0.co;2
Martin, J. D., Adams, J., Hollebone, B., King, T., Brown, R. S., and Hodson, P. V. (2014). Chronic Toxicity of Heavy Fuel Oils to Fish Embryos Using Multiple Exposure Scenarios. Environ. Toxicol. Chem. 33 (3), 677–687. doi:10.1002/etc.2486
Marzooghi, S., Finch, B. E., Stubblefield, W. A., Dmitrenko, O., Neal, S. L., and Di Toro, D. M. (2017). Phototoxic Target Lipid Model of Single Polycyclic Aromatic Hydrocarbons. Environ. Toxicol. Chem. 36 (4), 926–937. doi:10.1002/etc.3601
Matkin, C., Saulitis, E., Ellis, G., Olesiuk, P., and Rice, S. (2008). Ongoing Population-Level Impacts on Killer Whales Orcinus orca Following the 'Exxon Valdez' Oil Spill in Prince William Sound, Alaska. Mar. Ecol. Prog. Ser. 356, 269–281. doi:10.3354/meps07273
Milton, S., Lutz, P., and Shigenaka, G. (2003). “Oil Toxicity and Impacts on Sea Turtles,” in Oil and Sea Turtles: Biology, Planning, and Response (Silver Spring, MD: NOAA National Ocean Service), 35–47.
Mirjani, M., Soleimani, M., and Salari, V. (2021). Toxicity Assessment of Total Petroleum Hydrocarbons in Aquatic Environments Using the Bioluminescent Bacterium Aliivibrio Fischeri. Ecotoxicol. Environ. Saf. 207, 111554. doi:10.1016/j.ecoenv.2020.111554
Moles, A., and Norcross, B. L. (1998). Effects of Oil-Laden Sediments on Growth and Health of Juvenile Flatfishes. Can. J. Fish. Aquat. Sci. 55 (3), 605–610. doi:10.1139/f97-278
National Academies of Sciences and Medicine (2020). The Use of Dispersants in Marine Oil Spill Response. Washington, DC: The National Academies Press. doi:10.17226/25161
National Energy Board (2010). “Offshore Waste Treatment Guideline,” in Offshore Waste Treatment Guidelines; National Energy Board Canada-Newfoundland and Labrador Offshore Petroleum Board and Canada-Nova Scotia Offshore Petroleum Board. http://www.cnlopb.ca/legislation/guidelines.php.
National Research Council (2005). “Toxicological Effects of Dispersants and Dispersed Oil,” in Oil Spill Dispersants: Efficacy and Effects (Washington, DC: National Academies Press), 193–275.
Neff, J., Lee, K., and DeBlois, E. M. (2011). “Produced Water: Overview of Composition, Fates, and Effects,” in Produced Water: Environmental Risks and Advances in Mitigation Technologies. Editors K. Lee, and J. Neff (New York: Springer), 3–54. doi:10.1007/978-1-4614-0046-2_1
Nordtug, T., Olsen, A. J., Altin, D., Meier, S., Overrein, I., Hansen, B. H., et al. (2011). Method for Generating Parameterized Ecotoxicity Data of Dispersed Oil for Use in Environmental Modelling. Mar. Pollut. Bull. 62 (10), 2106–2113. doi:10.1016/j.marpolbul.2011.07.015
Nuka Research and Planning Group, LLC (2012). Oil Spill Response Gap and Response Capacity Analysis for Proposed Northern Gateway Tanker Oil Spills in Open Water and Protected Water Operating Environments. Seldovia, AK: Haisla Nation Council.
Nuka Research and Planning Group, LLC (2017). Prince William Sound Oil Spill Recovery Optimization Analysis. Seldovia, AK: Prince William Sound Regional Citizens’ Advisory Council.
Ohwada, K., Nishimura, M., Wada, M., Nomura, H., Shibata, A., Okamoto, K., et al. (2003). Study of the Effect of Water-Soluble Fractions of Heavy-Oil on Coastal Marine Organisms Using Enclosed Ecosystems, Mesocosms. Mar. Pollut. Bull. 47 (1), 78–84. doi:10.1016/S0025-326X(03)00112-7
Olsvik, P. A., Hansen, B. H., Nordtug, T., Moren, M., Holen, E., and Lie, K. K. (2011). Transcriptional Evidence for Low Contribution of Oil Droplets to Acute Toxicity from Dispersed Oil in First Feeding Atlantic Cod (Gadus morhua) Larvae. Comp. Biochem. Physiology Part C Toxicol. Pharmacol. 154 (4), 333–345. doi:10.1016/j.cbpc.2011.07.002
Özhan, K., Miles, S. M., Gao, H., and Bargu, S. (2014). Relative Phytoplankton Growth Responses to Physically and Chemically Dispersed South Louisiana Sweet Crude Oil. Environ. Monit. Assess. 186 (6), 3941–3956. doi:10.1007/s10661-014-3670-4
Perhar, G., and Arhonditsis, G. B. (2014). Aquatic Ecosystem Dynamics Following Petroleum Hydrocarbon Perturbations: A Review of the Current State of Knowledge. J. Gt. Lakes. Res. 40, 56–72. doi:10.1016/j.jglr.2014.05.013
Perkins, R. A., Rhoton, S., and Behr-Andres, C. (2003). Toxicity of Dispersed and Undispersed, Fresh and Weathered Oil to Larvae of a Cold- Water Species, Tanner Crab ( C. Bairdi), and Standard Warm- Water Test Species. Cold Regions Sci. Technol. 36 (1), 129. doi:10.1016/s0165-232x(03)00004-1
Peterson, C. H. (2001). The “Exxon Valdez” Oil Spill in Alaska: Acute, Indirect and Chronic Effects on the Ecosystem. Adv. Marine Biol. 39, 1–103. doi:10.1016/S0065-2881(01)39008-9
Philibert, D. A., Lyons, D. D., and Tierney, K. B. (2021). Comparing the Effects of Unconventional and Conventional Crude Oil Exposures on Zebrafish and Their Progeny Using Behavioral and Genetic Markers. Sci. Total Environ. 770, 144745. doi:10.1016/j.scitotenv.2020.144745
Philibert, D. A., Philibert, C. P., Lewis, C., and Tierney, K. B. (2016). Comparison of Diluted Bitumen (Dilbit) and Conventional Crude Oil Toxicity to Developing Zebrafish. Environ. Sci. Technol. 50 (11), 6091–6098. doi:10.1021/acs.est.6b00949
Reátegui-Zirena, E. G., Stewart, P. M., Whatley, A., Chu-Koo, F., Sotero-Solis, V. E., Merino-Zegarra, C., et al. (2014). Polycyclic Aromatic Hydrocarbon Concentrations, Mutagenicity, and Microtox® Acute Toxicity Testing of Peruvian Crude Oil and Oil-Contaminated Water and Sediment. Environ. Monit. Assess. 186 (4), 2171–2184. doi:10.1007/s10661-013-3527-2
Redman, A. D. (2015). Role of Entrained Droplet Oil on the Bioavailability of Petroleum Substances in Aqueous Exposures. Mar. Pollut. Bull. 97 (1–2), 342–348. doi:10.1016/j.marpolbul.2015.05.068
Reynaud, S., and Deschaux, P. (2006). The Effects of Polycyclic Aromatic Hydrocarbons on the Immune System of Fish: A Review. Aquat. Toxicol. 77 (2), 229–238. doi:10.1016/j.aquatox.2005.10.018
Rhoton, S. L., Perkins, R. A., Behr-Andres, C., and Braddock, J. F. (2001). A Cold-Weather Species' Response to Chemically Dispersed Fresh and Weathered Alaska North Slope Crude Oil. Int. Oil Spill Conf. Proc. 2001, 1231–1236. doi:10.7901/2169-3358-2001-2-1231
Roast, S. D., Thompson, R. S., Widdows, B. J., and Jones, M. B. (1998). Mysids and Environmental Monitoring: A Case for Their Use in Estuaries. Mar. Freshw. Res. 49 (8), 827. doi:10.1071/mf97099
Robidoux, P. Y., Virginie, B., Judith, L., and Marc, D. (2018). Assessment of Acute and Chronic Toxicity of Unweathered and Weathered Diluted Bitumen to Freshwater Fish and Invertebrates. Ecotoxicol. Environ. Saf. 164, 331–343. doi:10.1016/j.ecoenv.2018.08.010
Ruberg, E. J., Elliott, J. E., and Williams, T. D. (2021). Review of Petroleum Toxicity and Identifying Common Endpoints for Future Research on Diluted Bitumen Toxicity in Marine Mammals. Ecotoxicology 30 (4), 537–551. doi:10.1007/s10646-021-02373-x
Saco-Álvarez, L., Bellas, J., Nieto, Ó., Bayona, J. M., Albaigés, J., and Beiras, R. (2008). Toxicity and Phototoxicity of Water-Accommodated Fraction Obtained from Prestige Fuel Oil and Marine Fuel Oil Evaluated by Marine Bioassays. Sci. Total Environ. 394 (2), 275–282. doi:10.1016/j.scitotenv.2008.01.045
Saiz, E., Movilla, J., Yebra, L., Barata, C., and Calbet, A. (2009). Lethal and Sublethal Effects of Naphthalene and 1,2-dimethylnaphthalene on Naupliar and Adult Stages of the Marine Cyclopoid Copepod Oithona Davisae. Environ. Pollut. 157 (4), 1219–1226. doi:10.1016/j.envpol.2008.12.011
Santander-Avanceña, S. S., Sadaba, R. B., Taberna, H. S., Tayo, G. T., and Koyama, J. (2016). Acute Toxicity of Water-Accommodated Fraction and Chemically Enhanced WAF of Bunker C Oil and Dispersant to a Microalga Tetraselmis Tetrathele. Bull. Environ. Contam. Toxicol. 96 (1), 31–35. doi:10.1007/s00128-015-1696-0
Schlezinger, J. J., White, R. D., and Stegeman, J. J. (1999). Oxidative Inactivation of Cytochrome P-450 1A (CYP1A) Stimulated by 3,3′,4,4′-Tetrachlorobiphenyl: Production of Reactive Oxygen by Vertebrate CYP1As. Mol. Pharmacol. 56 (3), 588–597. doi:10.1124/mol.56.3.588
Schwacke, L. H., Smith, C. R., Townsend, F. I., Wells, R. S., Hart, L. B., Balmer, B. C., et al. (2014). Health of Common Bottlenose Dolphins (Tursiops truncatus) in Barataria Bay, Louisiana, Following theDeepwater HorizonOil Spill. Environ. Sci. Technol. 48 (1), 93–103. doi:10.1021/es403610f
Shu, H.-Y., and Huang, C.-R. (1995). Degradation of Commercial Azo Dyes in Water Using Ozonation and UV Enhanced Ozonation Process. Chemosphere 31 (8), 3813–3825. doi:10.1016/0045-6535(95)00255-7
Singer, M. M., Aurand, D., Bragin, G. E., Clark, J. R., Coelho, G. M., Sowby, M. L., et al. (2000). Standardization of the Preparation and Quantitation of Water-Accommodated Fractions of Petroleum for Toxicity Testing. Mar. Pollut. Bull. 40 (11), 1007–1016. doi:10.1016/S0025-326X(00)00045-X
SL Ross Environmental Research Ltd. (2005). Transfer of Decanting Technology Research to Oil Spill Response Organizations and Regulators. Ottawa, ON, Canada: Bureau of Safety and Environmental Enforcement, U.S.
Smith, C., Rowles, T., Hart, L., Townsend, F., Wells, R., Zolman, E., et al. (2017). Slow Recovery of Barataria Bay Dolphin Health Following the Deepwater Horizon Oil Spill (2013-2014), with Evidence of Persistent Lung Disease and Impaired Stress Response. Endang. Species. Res. 33, 127–142. doi:10.3354/esr00778
Sørensen, L., McCormack, P., Altin, D., Robson, W. J., Booth, A. M., Faksness, L.-G., et al. (2019). Establishing a Link between Composition and Toxicity of Offshore Produced Waters Using Comprehensive Analysis Techniques - A Way Forward for Discharge Monitoring? Sci. Total Environ. 694, 133682. doi:10.1016/j.scitotenv.2019.133682
Sørensen, L., Sørhus, E., Nordtug, T., Incardona, J. P., Linbo, T. L., Giovanetti, L., et al. (2017). Oil Droplet Fouling and Differential Toxicokinetics of Polycyclic Aromatic Hydrocarbons in Embryos of Atlantic Haddock and Cod. PloS One 12 (7), e0180048. doi:10.1371/journal.pone.0180048
Sørhus, E., Edvardsen, R. B., Karlsen, Ø., Nordtug, T., Van Der Meeren, T., Thorsen, A., et al. (2015). Unexpected Interaction with Dispersed Crude Oil Droplets Drives Severe Toxicity in Atlantic Haddock Embryos. PLoS ONE 10, e0124376. doi:10.1371/journal.pone.0124376
Swigert, J. P., Lee, C., Wong, D. C. L., White, R., Scarlett, A. G., West, C. E., et al. (2015). Aquatic Hazard Assessment of a Commercial Sample of Naphthenic Acids. Chemosphere 124 (1), 1–9. doi:10.1016/j.chemosphere.2014.10.052
Tamis, J. E., Jongbloed, R. H., Karman, C. C., Koops, W., and Murk, A. J. (2012). Rational Application of Chemicals in Response to Oil Spills May Reduce Environmental Damage. Integr. Environ. Assess. Manag. 8 (2), 231–241. doi:10.1002/ieam.273
The U.S. National Response Team (2018). Guidance for the Decanting of Contact Water in Inland, Ocean, and Coastal Waters. Philadelphia, PA: The U.S. National Response Team (NRT).
USEPA (2009). Mysid (Americamysis Bahia) Survival, Growth, and Fecundity Toxicity Tests. Supplement to Training Video Whole Effluent Toxicity Training Video Series, Saltwater Series. Available at https://www.epa.gov/sites/production/files/2015-09/documents/mysidstesting.pdf.
Uttieri, M., Nihongi, A., Hinow, P., Motschman, J., Jiang, H., Alcaraz, M., et al. (2019). Copepod Manipulation of Oil Droplet Size Distribution. Sci. Rep. 9 (1), 547. doi:10.1038/s41598-018-37020-9
Vander Zanden, H. B., Bolten, A. B., Tucker, A. D., Hart, K. M., Lamont, M. M., Fujisaki, I., et al. (2016). Biomarkers Reveal Sea Turtles Remained in Oiled Areas Following the Deepwater Horizon Oil Spill. Ecol. Appl. 26 (7), 2145–2155. doi:10.1002/eap.1366
Verslycke, T. A., Fockedey, N., McKenney, C. L., Roast, S. D., Jones, M. B., Mees, J., et al. (2004). Mysid Crustaceans as Potential Test Organisms for the Evaluation of Environmental Endocrine Disruption: A Review. Environ. Toxicol. Chem. 23 (5), 1219. doi:10.1897/03-332
Walker, A. H., Scholz, D., McPeek, M., French-McCay, D., Rowe, J., Bock, M., et al. (2018). Comparative Risk Assessment of Spill Response Options for a Deepwater Oil Well Blowout: Part III. Stakeholder Engagement. Mar. Pollut. Bull. 133, 970–983. doi:10.1016/j.marpolbul.2018.05.009
Walton, H. E., Davison, J. J., Uzyczak, J., Martin, C., Milliken, P., and Kirby, M. F. (2021). The Development of New Toxicity Testing and Approval Processes for Oil Spill Treatment Products in the UK. Int. Oil Spill Conf. Proc. 2021 (1), 687653. doi:10.7901/2169-3358-2021.1.687653
Wells, P. G., Lee, K., and Blaise, C. (1997). Microscale Testing in Aquatic Toxicology: Advances, Techniques, and Practice. Boca Raton, Florida: CRC Press.
Wise, C. F., Wise, J. T. F., Wise, S. S., and Wise, J. P. (2018). “Chemically Dispersed Oil Is Cytotoxic and Genotoxic to Sperm Whale Skin Cells,” in 8th Aquatic Models of Human Disease Conference Held at the University of Alabama at Birmingham, January 2017, 64–70. doi:10.1016/j.cbpc.2017.10.009
Wu, D., Wang, Z., Hollebone, B., McIntosh, S., King, T., and Hodson, P. V. (2012). Comparative Toxicity of Four Chemically Dispersed and Undispersed Crude Oils to Rainbow Trout Embryos. Environ. Toxicol. Chem. 31 (4), 754–765. doi:10.1002/etc.1739
Yang, Z., Hua, Y., Mirnaghi, F., Hollebone, B. P., Jackman, P., Brown, C. E., et al. (2018). Effect of evaporative weathering and oil-sediment interaction on the fate and behavior of diluted bitumen in marine environments. Part 2. The water accommodated and particle-laden hydrocarbon species and toxicity of the aqueous phase. Chemosphere 191, 145–155. doi:10.1016/j.chemosphere.2017.10.033
Ylitalo, G., Collier, T., Anulacion, B., Juaire, K., Boyer, R., da Silva, D., et al. (2017). Determining oil and dispersant exposure in sea turtles from the northern Gulf of Mexico resulting from the Deepwater Horizon oil spill. Endang. Species. Res. 33, 9–24. doi:10.3354/esr00762
Zhu, Z., Merlin, F., Yang, M., Lee, K., Chen, B., Liu, B., et al. (2022a). Recent Advances in Chemical and Biological Degradation of Spilled Oil: A Review of Dispersants Application in the Marine Environment. J. Hazard. Mater. 436, 129260. doi:10.1016/j.jhazmat.2022.129260
Keywords: decanting, ecotoxicity, water accommodated fraction, oil spill, mechanical oil recovery, demulsification, toxicity prediction
Citation: Liu B, Chen B, Ling J, Ye X, Dong G, Matchinski EJ and Zhang B (2022) Ecotoxicity Studies for On-Site Disposal of Decant Water During Oil Spills: A Review. Front. Environ. Sci. 10:944010. doi: 10.3389/fenvs.2022.944010
Received: 14 May 2022; Accepted: 13 June 2022;
Published: 13 July 2022.
Edited by:
Tania Martellini, University of Florence, ItalyReviewed by:
Bao Mutai, Ocean University of China, ChinaCopyright © 2022 Liu, Chen, Ling, Ye, Dong, Matchinski and Zhang. This is an open-access article distributed under the terms of the Creative Commons Attribution License (CC BY). The use, distribution or reproduction in other forums is permitted, provided the original author(s) and the copyright owner(s) are credited and that the original publication in this journal is cited, in accordance with accepted academic practice. No use, distribution or reproduction is permitted which does not comply with these terms.
*Correspondence: Bing Chen, YmNoZW5AbXVuLmNh
Disclaimer: All claims expressed in this article are solely those of the authors and do not necessarily represent those of their affiliated organizations, or those of the publisher, the editors and the reviewers. Any product that may be evaluated in this article or claim that may be made by its manufacturer is not guaranteed or endorsed by the publisher.
Research integrity at Frontiers
Learn more about the work of our research integrity team to safeguard the quality of each article we publish.