- 1Department of Biology, University of Naples Federico II, Naples, Italy
- 2Department of Chemical Sciences, Analytical Chemistry for the Environment, University of Naples Federico II, Naples, Italy
- 3CeSMA Advanced Metrological and Technological Service Center, University of Naples Federico II, Naples, Italy
The application of rare earth elements (REEs) in several areas, including high-tech technology, agriculture, medicine, and fuels, has made them an essential component of our everyday life. This extensive use of REEs in several technologies is expected to potentially impact human health. Even if several studies investigated the levels of REEs in human matrices, until now no standard method has been established for analyzing these elements in human matrices. The sample analysis should be of high quality, and the methods should be validated properly to ensure the quality of the procedure and traceability of the analytical data. In this research, we compared the validation and effectiveness of two different methods of sample preparation for human urine samples: a simple dilution of the sample (DIL) was compared with microwave assisted-acid decomposition (MIN) for tracing REE levels in human urine samples. The analysis was carried out by inductively coupled plasma mass spectrometry (ICP-MS). The working conditions have been set in high-sensitivity mode. Accuracy of the proposed method was evaluated by spiking the sample matrix with known concentrations of analyte standards. Both methods showed adequate precision of repeatability and intra-laboratory reproducibility, with the DIL method showing better precision of both repeatability and reproducibility than the MIN method. The CVr% values of repeatability range from 1.5 to 12% for the DIL and from 8.4 to 16% for the MIN method. The CVr% values of reproducibility range from 6.2–23% for the DIL and from 8.6 to 24% for the MIN method. REE recoveries for both methods were very close to 100%. Both methods proved to be effective for the determination of REE levels in human urine matrices.
1 Introduction
REEs have become an essential component of present-day life due to their pervasive applications in several industrial, agricultural, and medical applications. This extensive use of REEs in several technologies is expected to impact human health, including occupational and environmental REE exposures (Rim, 2016; Pagano, 2017; Pagano et al., 2019). Unlike experimental studies, the consequences of REE exposures to human health have been subjected to relatively fewer investigations. The likely environmental threats arising from REE exposures deserve a new line of research efforts (Pagano et al., 2015b). Environmental exposures have been investigated in populations residing in REE mining areas, showing REE bioaccumulation in scalp hair, excess REE urine levels, and defective gene expression (Pagano et al., 2015a; Pagano et al., 2015b).
Several studies investigated the bioaccumulation of REEs in human urine matrices. Hao et al. (2015) found elevated levels of REEs in the hair of people living near Baiyun Obo deposit (China), the world’s largest REE deposit, compared to the selected control area. Li et al. (2016) found higher levels of La, Ce, Nd, and Gd in the urine of workers manufacturing cerium and lanthanum oxide ultrafine and nano-sized particles. Li et al. (2017) found higher levels of La and Ce in the urine of the same kind of workers. The levels of Y, La, Ce, Pr, Nd, and Sm were higher in the urine of children living near the Bayan Obo mining area (Liang et al., 2018). Liu et al. (2019) concluded that increased maternal urinary Ce and Yb level could be associated to decreased neonatal TSH levels. People living near smelting areas were more exposed to REEs than people living near mining areas since their urine contains higher levels of these metals (Meryem et al., 2016). The urines of e-waste recyclers at Agbogbloshie (Ghana) contain elevated levels of La, Eu, and Tb (Takyi et al., 2021). Cirtiu et al. (2022) investigated the REE levels in urines of residents in Nunavik (Canada) before the beginning of REE exploitation in this area. Allain et al. (1990) investigated REE levels in healthy men and found that their levels were below the related detection limits.
Few innovative methods based on Inductively Coupled Plasma Mass Spectrometry (ICP-MS) have been developed for determination of REEs in urine matrices. The Italian Institute of Health (ISS) described a method for the determination of chemical elements in human biological samples (serum, urine, blood, and similar fluids) by ICP-MS, but no REEs were investigated (Alimonti et al., 2015). A method based on polymer monolithic capillary microextraction combined on-line with microconcentric nebulization ICP-MS for the determination of REEs in biological samples was developed by Zhang et al. (2013). Li et al. (2016) explored a sensitive and reliable indicator of the exposure level to REEs in urines. Li et al. (2017) identified the occupational exposure to REEs by analyzing urines using ICP-MS based on closed-vessel, microwave-assisted wet digestion. Bettinelli et al. (2002) developed a method with electrothermal vaporization ICP-MS (ETV-ICPMS) for determining REEs in urine, in which the sample was injected into the graphite tube and trifluoromethane (Freon-23) and was used as a chemical modifier to reduce vaporization temperature and the memory effect of most of the lanthanides.
Even if several studies investigated REE levels in human matrices, until today, no standard method has been established for analyzing these elements in human matrices. The sample analysis should be of high quality and the methods should be validated properly to ensure quality of the procedure and traceability of the analytical data. It is essential, therefore, to establish methods for effective tracing of REEs in human matrices, including urines.
In this research, we described a simple sample preparation method by dilution with nitric acid solution (2%, v/v) in a ratio 1:5 (namely, DIL) and compared it to the microwave-assisted-acid digestion (namely, MIN) for the preparation of human urine samples for subsequent determination of REE levels by ICP-MS.
2 Materials and methods
2.1 Reagents and materials
All analyses were conducted at the laboratory of Analytical Chemistry for the Environment of the University of Naples Federico II. High-purity water (resistivity of 18.2 MΩ cm) was obtained from a Milli-Q unit (Millipore, United States) and was used for all solution preparation and sample dilution. Nitric acid (HNO3, 69% v/v Ultratrace@ ppb-trace analysis grade) was provided by Scharlau (Barcelona, Spain). The multicomponent solution of 16 rare earth elements (50 mg/L each) was of ultrapure grade for ICP, TraceCERT® and was purchased from Merck (Darmstadt, Germany). All glassware used for preparation of samples and standards was washed overnight by immersion in ultrapure HNO3 solution (10% v/v), while plastic containers used for sampling were washed by HNO3 diluted solution (2% v/v), following a rinse with deionized water and dryness before use. All the materials have been tested and found free of rare earth elements.
2.2 Ethical statement
All methods were performed in accordance with the guidelines and regulations contained in the Declaration of Helsinki (World Medical Association, 2013). All experimental protocols were approved by the Ethics Committee of the University of Naples Federico II (Italy) (authorization n. 181/21). All the recruited participants provided their written informed consent to participate to the study before the sample collection within the H2020 PANORAMA project (see the acknowledgment for more details).
2.3 Sampling and sample preparation
Urine samples were collected in 100-ml containers and deposited at −18°C and then thawed for preparation prior to ICP-MS analysis. Two different methods of digestion were considered and compared to identify the most adequate method of urine sample preparation for subsequent ICP-MS analysis.
2.3.1 Method 1—simple dilution with nitric acid
In a 10-ml PP test tube, 1 ml of the urine sample was placed and 4 ml of HNO3 solution (2%, v/v) were added. The mixture was gently stirred with the use of a vortex, and the samples thus prepared were analyzed directly by ICP-MS in the shortest possible time to avoid the possible precipitation of undigested organic compounds.
2.3.2 Method 2—Microwave-assisted-acid decomposition method
In a 10-ml glass vessel provided by CEM Corporation (Charlotte, North Carolina), 2 ml of the urine sample was mixed with 0.5 ml of concentrated HNO3 (69%, v/v). A microwave-assisted-acid decomposition was followed using the automated digestion system Discover SP-D Clinical (CEM Corporation, Charlotte, North Carolina). After the introduction of a small stirring bar, the vessel was capped and placed in the microwave oven for digestion with the following steps: heating for 2 min up to 120°C, remaining at 120°C for 2 min, and then cooling down for 30 s to room temperature. After the decomposition, the mixture was transferred to a 10-ml PP test tube and diluted with HNO3 solution (2%, v/v) to a final volume of 10 ml for subsequent analysis by ICP-MS.
2.4 Method validation
A validation program was built up according to Eurachem Guide–The Fitness for Purpose of Analytical Methods (Magnusson and Ornemark, 2014) and UNI CEI EN ISO/IEC 17025 (2018) to ensure the quality of both DIL and MIN methods. The characteristic parameters of the method were evaluated for each element, that is, the limit of detection (LOD) and quantification (LOQ), working and linear ranges, repeatability and reproducibility precision, accuracy, and recovery.
LOD and LOQ were determined according to Eurachem Guide (Magnusson and Ornemark, 2014) with the determination of 10 replicate measurements of blank samples containing only HNO3 solution (2%, v/v). LOD was calculated as 3s and LOD as 10s, s being the standard deviation of the measured concentrations.
The working range was evaluated by analyzing one blank and six standards at different concentrations, and the linearity was tested verifying the linear regression coefficient (R2) of the calibration curve. The linearity was acceptable, with R2 value greater than 0.996.
Precision was evaluated under repeatability conditions with the analysis of 10 replicates of spiked urine matrix carried out by the same analyst, equipment, and laboratory in a short timescale.
Precision was evaluated under reproducibility conditions with the analysis of 10 replicates of the same spiked urine sample carried out by different analysts, equipment, and same laboratory in an extended timescale, over a 2-week period.
Both repeatability and reproducibility precision are generally dependent on analyte concentrations, and so in this study, they were evaluated at three different concentrations of the calibration curve.
By determining the standard deviation of the replicates, the repeatability standard deviation (sr) and the intra-laboratory reproducibility deviation (sR) were obtained at each concentration level.
Because at our best knowledge there are no certified reference materials of all the investigated REEs for urine, the accuracy was evaluated by recovery studies. Urine unfortified samples were spiked with a certified REE solution at three different concentrations in the range from 0.05 to 75 μg/L. At least six replicates were executed with both DIL and MIN methods. The recovery is the percentage of fortified concentration, obtained for each element in each sample at the four concentration levels, according to formula presented in the Eurachem Guide (Magnusson and Ornemark, 2014).
2.5 Coupled plasma—mass spectrometry analysis
Multi-elemental analysis was performed by the Inductively Coupled Plasma—Mass Spectrometry (ICP-MS) by using an Aurora M90ICP-MS instrument (Bruker, Germany). The experimental conditions are given in Table 1. The analysis was performed in the high-sensitivity mode. All standards used for analysis in ICP-MS were prepared in HNO3 solution (2%, v/v). Calibration curves for determining REEs ranged from 0.005 to 100 μg/L and were constructed daily by analysis of standard solutions prepared immediately before analysis. Calibration ranges for each REE are presented in Table 2. The internal standard was 115In for both the calibration curve and sample analysis. A blank for reagents and a blank for the digestion method were performed to verify REE contamination of all materials used for each batch and verified in the instrumental sequence every 10 samples. At least two control standards were verified for every 20 samples.
3 Results and discussion
3.1 Limit of detection and limit of quantification values
LOD and LOQ values for the ICP-MS determination and for the dilution (DIL) and digestion (MIN) urine sample preparation procedures for each REE are shown in Table 3. For the ICP-MS determination, LOD values ranged from 0.001 to 0.004 μg/L with Tb, Er, and Tm showing the lowest value and Nd the highest value, while LOQ values ranged from 0.005 to 0.013 μg/L, with Tb, Dy, Er, Tm, and Lu showing the lowest and Nd the highest value. For both urine sample preparation procedures, LOD values ranged from 0.007 to 0.019 μg/L, with Tb, Er, and Tm showing the lowest and Nd the highest value, while LOQ values ranged from 0.023 to 0.065 μg/L, with Tb showing the lowest and Nd the highest value. Our findings are in accordance with LOD and LOQ values for determination of REEs from other studies (Hao et al., 2015; Li et al., 2016; Li et al., 2017; Liu et al., 2019). For all elements, the values were normally distributed (Shapiro–Wilk 5% test; p < 0.05), and the presence of irregular data was not statistically significant (Dixon 5% single test; p < 0.05).
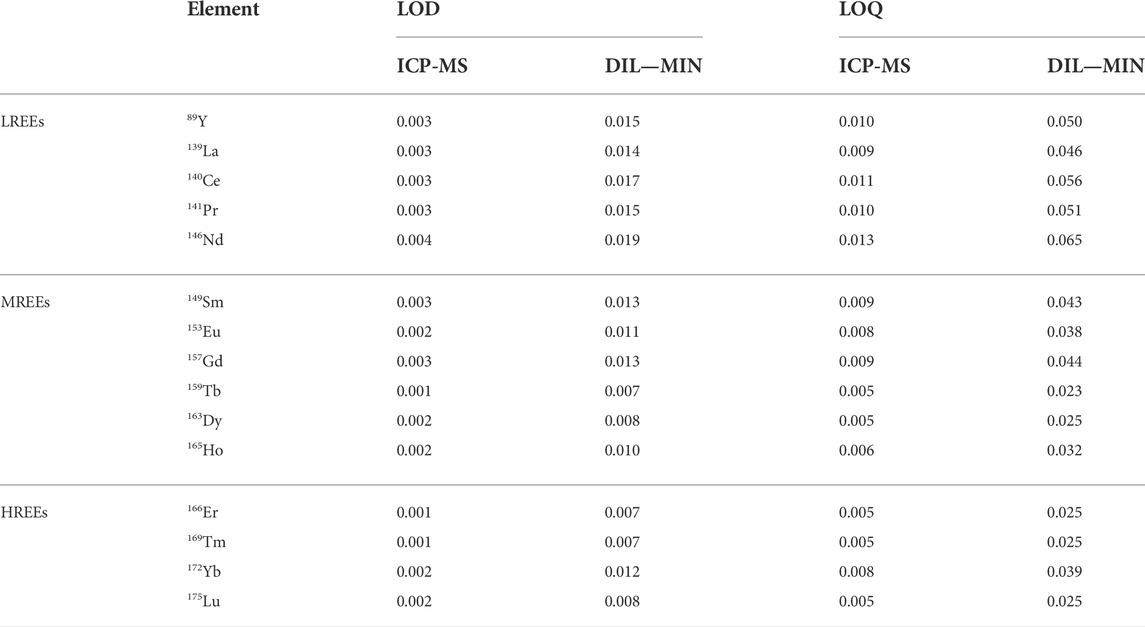
TABLE 3. Limits of detection (LODs) and limits of quantifications (LOQs) evaluated for the instrument (ICP-MS) and for the preparation methods (dilution, DIL; digestion, MIN). All values are expressed in µg/L.
3.2 Precision
The results of the evaluation of repeatability precision and intra-laboratory reproducibility precision were obtained for both DIL and MIN methods at three different concentration levels are presented in Table 4 and Table 5, respectively.
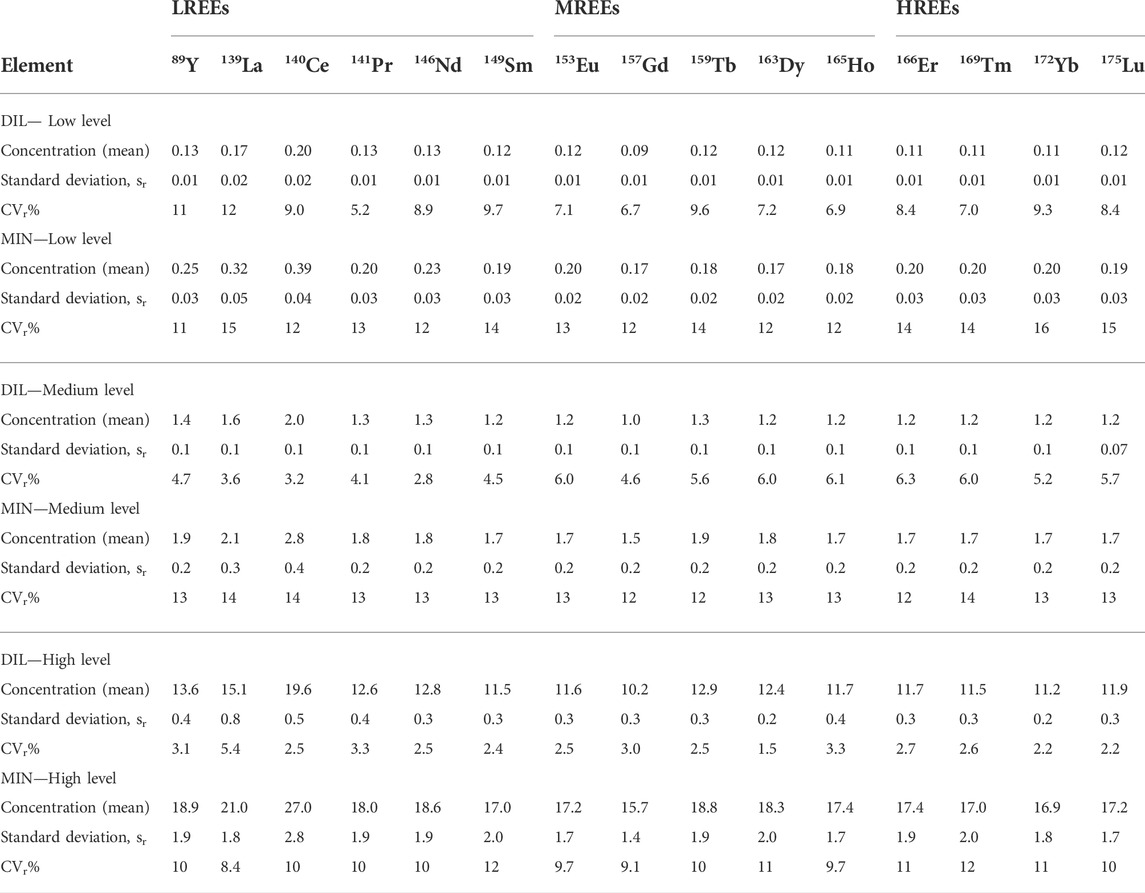
TABLE 4. Results of repeatability precision expressed as standard deviation, sr (µg/L) and relative standard deviation (CVr%) at three different levels of concentrations for the dilution (DIL) and digestion (MIN) sample preparation procedures.
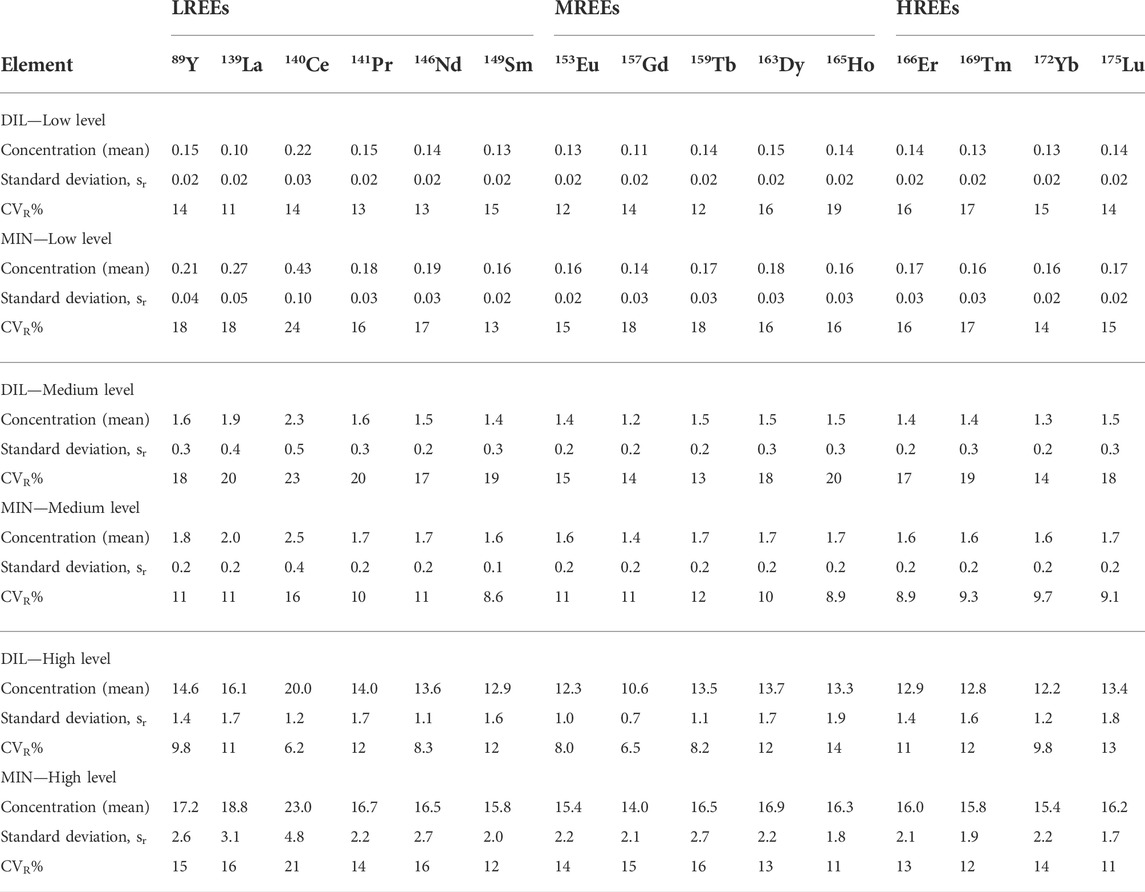
TABLE 5. Results of reproducibility precision expressed as standard deviation, sR (µg/L) and relative standard deviation (CVR%) at three different levels of concentrations (µg/L) for the dilution (DIL) and digestion (MIN) sample preparation procedures.
The DIL sample preparation method shows a better level of repeatability than the MIN method. The mean values of relative standard deviation (CVr%) for the DIL method range from 1.5 to 12% and for the MIN method, they range from 8.4 to 15%. For both methods, as expected, the values are lower with increasing concentrations.
Intra-laboratory reproducibility precision also shows better values of relative standard deviation (CVR%) for the DIL method for low and high levels of concentrations, with slightly higher values at the medium level than for the MIN method.
However, the CVR% values were generally similar for both methods ranging from 6.2 to 23% for the DIL method and from 8.6 to 24% for the MIN method.
3.3 Accuracy and recovery
The accuracy of analytical methods was evaluated by analyzing urine samples spiked with known concentrations of REEs at four different levels. The starting unfortified sample was analyzed and shows REE values below the LOQs. The urine sample for the DIL method was fortified with the REE multi-component solution at concentrations of 0.05, 0.25, 3.0, and 75 μg/L identified as level 1, level 2, level 3, and level 4, respectively. The urine sample for the MIN method was fortified with the same solution at concentrations of 0.05, 0.50, 5.0, and 50 μg/L identified as level 1, level 2, level 3, and level 4, respectively.
In both tested sample preparation methods and at all levels, the recovery values ranged within ±15% in all samples. The results are presented in Table 6.
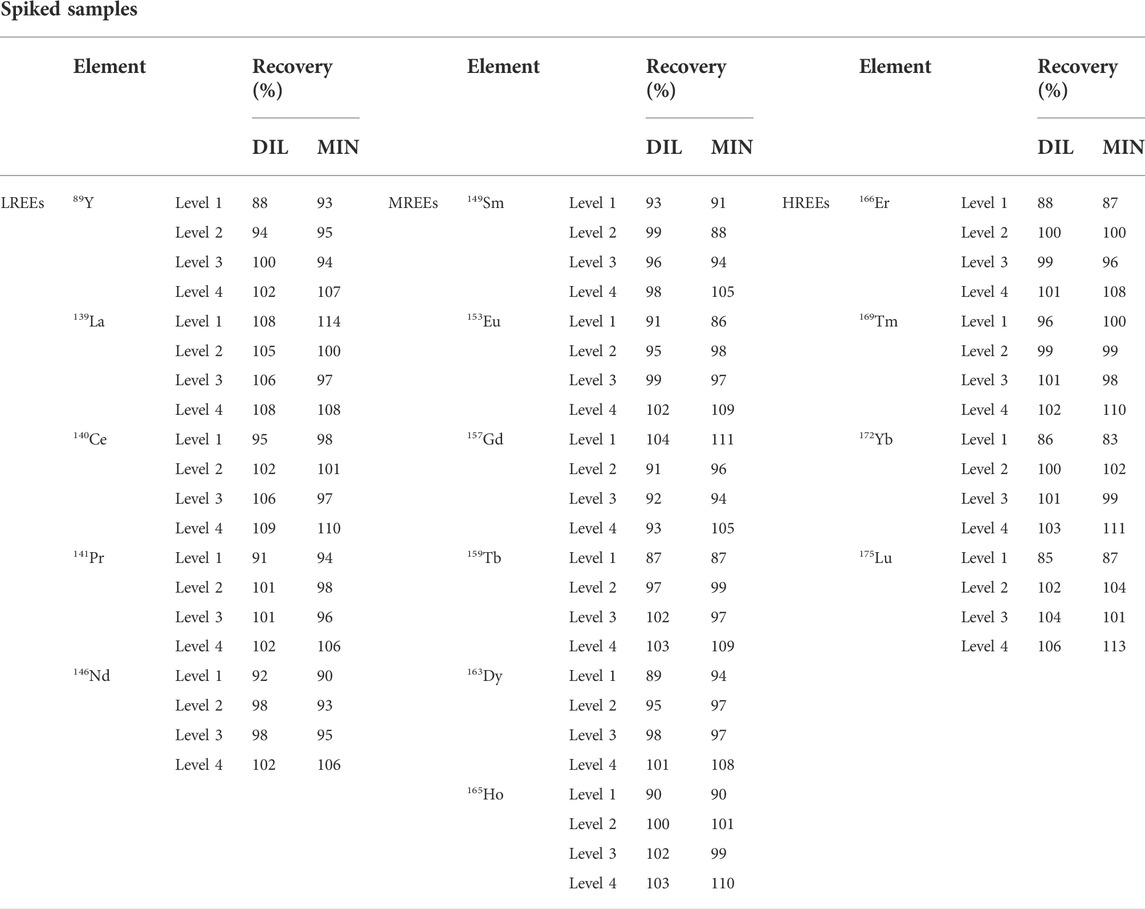
TABLE 6. Results of accuracy expressed as recovery (%) based on analysis of spiked samples at four different levels for the dilution (DIL) and digestion (MIN) sample preparation procedures.
Methods using sample dilution for analyzing REE levels in urine samples have been used in several studies. Allain et al. (1990) also prepared the samples by a simple 6-fold dilution with HNO3 solution, 10 ml/L. Cirtiu et al. (2022) made a 20-fold dilution with HNO3 solution, 0.5% (v/v). Hao et al. (2015) made a 10-fold dilution with deionized water. Li et al. (2016) made a 20-fold dilution with HNO3 solution, 2% (v/v).
In our study, we demonstrated precision, accuracy, and reliability of the simple dilution for urine sample preparation. Use of HNO3 solution, 2% (v/v) allowed direct analysis to the ICP-MS, and in this way, we did not go beyond a 5-fold dilution, and this allows obtaining lower detection limits.
Similar preparations to our MIN method have been used before. Zhang et al. (2013) also used a microwave digestion method for preparing the urine samples, adding 2.5 ml of concentrated HNO3 to 1 ml of urine. Li et al. (2017) prepared 1 ml of the urine sample with 5 ml of 65% HNO3, 2 ml of H2O2, 30% (v/v), and 2 ml Milli-Q water and used a closed-vessel microwave-assisted wet digestion.
In our study, we decided to leave the dilution ratio between the two methods unchanged for a better comparison of results. We demonstrated precision, accuracy, and reliability of the microwave-assisted urine preparation method with a reduced amount of nitric acid, adding only 0.5 ml of concentrated HNO3 to 2 ml of urine. Furthermore, the digested aliquot was brought to a final volume of 10 ml using of HNO3 solution, 2% (v/v), allowing direct analysis to the ICP-MS. In this way, the dilution ratio 1 to 5 is maintained and, therefore, the possibility of obtaining lower detection limits in urine matrix.
The choice of the methods used in this study was also guided by the possibility of carrying out in the future the analysis of trace metals with the same methods, optimizing the analytical times and the quantity of the sample required.
Several studies in the literature had used acid dilution for urine sample preparation also for the determination of trace elements. Batista et al. (2009) made a 20-fold dilution with a solution containing HNO3, 0.5% (v/v) and Triton X-100 (0.005%, v/v). Bocca et al. (2019) made a 5-fold dilution with high-purity deionized water. Cui et al. (2005) made a 10-fold dilution with high purity deionized water. De Matos et al. (2022) made a 20-fold dilution with a solution containing HNO3, 0.4% (v/v) and Triton X-100 (0.005%, v/v). Asante et al. (2012) made a 5-fold dilution with Milli-Q water to determine arsenic. Schmied et al. (2021) made a 10-fold dilution with HNO3 solution, 2% (v/v) and internal standard solution. Tong et al. (2021) made a 10-fold dilution with HNO3 solution, 1% (v/v).
In other studies, researchers carried out microwave digestion for preparing urine samples to determine trace elements (Asante et al., 2012; Choe and Gajek, 2016; Pham et al., 2017; Moore et al., 2018).
A future goal will be to lead a validation study of the two different methods of digestion used in the present work for the determination of trace element levels in human urine samples.
4 Conclusion
The digesting capacity of different sample preparation methods is a crucial step which can affect the accuracy of analytical results.
In this study, we showed the comparison between the validation data obtained from two different digestion procedures for determination of REEs in human urine samples. The analysis was carried out by ICP-MS in high-sensitivity mode, that is, a widely used technique in clinical, forensic, occupational, and environmental fields for determination of metals and metalloids.
We proposed a simple dilution of the sample with diluted nitric acid solution (2%, v/v) and a microwave-assisted-acid digestion.
Both validated methods presented in this work meet the performance criteria and the requirements set out in European regulations for method validation.
Both methods showed adequate precision of repeatability and reproducibility. The mean values of relative repeatability standard deviation (CVr%) for the DIL method range from 1.5 to 12% and for the MIN method range from 8.4 to 15%. The reproducibility CVR% values range from 6.2 to 23% for the DIL method and from 8.6 to 24% for the MIN method.
Accuracy of the proposed method was evaluated by spiking the sample matrix, and REE recoveries for both methods were very close to 100% ranging from 91 to 109% for the DIL method and from 88 to 113% for the MIN method.
Therefore, both tested methods of preparation are validated for analyzing REEs in urine samples, according to the method validation and the criteria of the guidelines.
Furthermore, both methods offer satisfactory LOD values ranging from 0.007 to 0.019 μg/L in the urine matrix sample.
Therefore, this allows defining them suitable for the analysis of REEs in environmental health investigations.
Data availability statement
The original contributions presented in the study are included in the article/Supplementary Material; further inquiries can be directed to the corresponding authors.
Ethics statement
The studies involving human participants were reviewed and approved by Ethics Committee of University of Naples Federico II (Italy) (authorization n. 181/21). The patients/participants provided their written informed consent to participate in this study.
Author contributions
Conceptualization, AB, AG, and MT; data curation, AB, AN, AG, AM, and FL; methodology, AB, AN, AG, AM, FL, and MT; software, AB, AN, AG, AM, and FL; validation, AB, AN, AG, AM, FL, and MT; formal analysis, AB, AN, AG, AM, and FL; investigation, AB, AN, AG, AM, FL, and MT; resources, MT, MG, and GL; writing—original draft preparation, AB, AG, and MT; writing—review and editing, AB, AG, AM, MT, and GL; visualization, AB, AG, and AM; supervision, MT and GL; Project administration, MT and GL; funding acquisition, MT, MG, and GL. All authors have read and agreed to the published version of the manuscript.
Funding
This research has received funding from European Union’s Horizon 2020 research and innovation program under the Marie Sklodowska-Curie Grant Agreement N°857989.
Acknowledgments
We acknowledge Giovanni Pagano for his contribution to the project and for his advice and exchanges of views.
Conflict of interest
The authors declare that the research was conducted in the absence of any commercial or financial relationships that could be construed as a potential conflict of interest.
Publisher’s note
All claims expressed in this article are solely those of the authors and do not necessarily represent those of their affiliated organizations, or those of the publisher, the editors, and the reviewers. Any product that may be evaluated in this article, or claim that may be made by its manufacturer, is not guaranteed or endorsed by the publisher.
References
Alimonti, A., Bocca, B., and Ruggieri, F. (2015). Reference method for the determination of chemical elements in human biological matrices: analytical performances and uncertainty of data. Rapporti ISTISAN 15/30. Roma: Istituto Superiore di Sanità. Available at: https://www.iss.it/documents/20126/45616/15_30_web.pdf/4f95e0d8-38eb-82cd-0ccc-3336d29cfac1?t=1581099105455.
Allain, P., Berre, S., Premel-Cabic, A., Mauras, Y., and Delaporte, T. (1990). Concentrations of rare Earth elements in plasma and urine of healthy subjects determined by inductively coupled plasma mass spectrometry. Clin. Chem. 36, 2011–2012. doi:10.1093/clinchem/36.11.2011b
Asante, K. A., Agusa, T., Biney, C. A., Agyekum, W. A., Bello, M., Otsuka, M., et al. (2012). Multi-trace element levels and arsenic speciation in urine of e-waste recycling workers from Agbogbloshie, Accra in Ghana. Sci. Total Environ. 424, 63–73. doi:10.1016/j.scitotenv.2012.02.072
Batista, B. L., Rodrigues, J. L., Tormen, L., Curtius, A. J., and Barbosa, F. (2009). Reference concentrations for trace elements in urine for the Brazilian population based on q-ICP-MS with a simple dilute-and-shoot procedure. J. Braz. Chem. Soc. 20, 1406–1413. doi:10.1590/S0103-50532009000800004
Bettinelli, M., Spezia, S., Terni, C., Ronchi, A., Balducci, C., and Minoia, C. (2002). Determination of rare Earth elements in urine by electrothermal vaporization inductively coupled plasma mass spectrometry. Rapid Commun. Mass Spectrom. 16, 579–584. doi:10.1002/rcm.609
Bocca, B., Ruggieri, F., Pino, A., Rovira, J., Calamandrei, G., Martínez, M. A., et al. (2019). Human biomonitoring to evaluate exposure to toxic and essential trace elements during pregnancy. Part A. concentrations in maternal blood, urine and cord blood. Environ. Res. 177, 108599. doi:10.1016/j.envres.2019.108599
Choe, K. Y., and Gajek, R. (2016). Determination of trace elements in human urine by ICP-MS using sodium chloride as a matrix-matching component in calibration. Anal. Methods 8, 6754–6763. doi:10.1039/c6ay01877g
Cirtiu, C. M., Valcke, M., Gagne, M., Bourgault, M. H., Narame, C., Gadio, S., et al. (2022). Biological monitoring of exposure to rare Earth elements and selected metals in the Inuit population of Nunavik, Canada. Chemosphere 289, 133142. doi:10.1016/j.chemosphere.2021.133142
Cui, Y., Zhu, Y. G., Zhai, R., Huang, Y., Qiu, Y., and Liang, J. (2005). Exposure to metal mixtures and human health impacts in a contaminated area in Nanning, China. Environ. Int. 31, 784–790. doi:10.1016/j.envint.2005.05.025
De Matos, A. R., Silva Faria, M. C., Freire, B. M., Pereira, R. M., Batista, B. L., and Rodrigues, J. L. (2022). Determination of 14 trace elements in blood, serum and urine after environmental disaster in the doce river basin: relationship between mining waste and metal concentration in the population. J. Trace Elem. Med. Biol. 70, 126920. doi:10.1016/j.jtemb.2021.126920
Hao, Z., Li, Y., Li, H., Wei, B., Liao, X., Liang, T., et al. (2015). Levels of rare Earth elements, heavy metals and uranium in a population living in Baiyun Obo, Inner Mongolia, China: a pilot study. Chemosphere 128, 161–170. doi:10.1016/j.chemosphere.2015.01.057
Li, Y., Yu, H., Li, P., and Bian, Y. (2017). Assessment the exposure level of rare earth elements in workers producing cerium, lanthanum oxide ultrafine and nanoparticles. Biol. Trace Elem. Res. 175, 298–305. doi:10.1007/s12011-016-0795-z
Li, Y., Yu, H., Zheng, S., Miao, Y., Yin, S., Li, P., et al. (2016). Direct quantification of rare Earth elements concentrations in urine of workers manufacturing Cerium, Lanthanum oxide ultrafine and nanoparticles by a developed and validated ICP-MS. Int. J. Environ. Res. Public Health 13, 350. doi:10.3390/ijerph13030350
Liang, Q., Yin, H., Li, J., Zhang, L., Hou, R., and Wang, S. (2018). Investigation of rare Earth elements in urine and drinking water of children in mining area. Medicine 97, e12717. doi:10.1097/MD.0000000000012717
Liu, Y., Wu, M., Zhang, L., Bi, J., Song, L., Wang, L., et al. (2019). Prenatal exposure of rare Earth elements cerium and ytterbium and neonatal thyroid stimulating hormone levels: findings from a birth cohort study. Environ. Int. 133, 105222. doi:10.1016/j.envint.2019.105222
Magnusson, B., and Ornemark, U. (2014). Eurachem Guide: The Fitness for Purpose of Analytical Methods - a laboratory guide to method validation and related topics. 2nd edition. Available at: https://www.eurachem.org/images/stories/Guides/pdf/MV_guide_2nd_ed_EN.pdf (Accessed August 05, 2022).
Meryem, B., Hongbing, J. I., Yang, G., Huajian, D., and Cai, L. (2016). Distribution of rare Earth elements in agricultural soil and human body (scalp hair and urine) near smelting and mining areas of Hezhang, China. J. Rare Earths 34, 1156–1167. doi:10.1016/S1002-0721(16)60148-5
Moore, R. E. T., Rehkamper, M., Kreissig, K., Strekopytov, S., and Larner, F. (2018). Determination of major and trace element variability in healthy human urine by ICP-QMS and specific gravity normalisation. RSC Adv. 8, 38022–38035. doi:10.1039/c8ra06794e
Pagano, G., Aliberti, F., Guida, M., Oral, R., Siciliano, A., Trifuoggi, M., et al. (2015a). Rare Earth elements in human and animal health: State of art and research priorities. Environ. Res. 142, 215–220. doi:10.1016/j.envres.2015.06.039
Pagano, G., Guida, M., Tommasi, F., and Oral, R. (2015b). Health effects and toxicity mechanisms of rare Earth elements—knowledge gaps and research prospects. Ecotoxicol. Environ. Saf. 115, 40–48. doi:10.1016/j.ecoenv.2015.01.030
Pagano, G. (2017). Rare earth elements in human and environmental health: At the crossroads between toxicity and safety. New York, NY: CRC Press.
Pagano, G., Thomas, P. J., Di Nunzio, A., and Trifuoggi, M. (2019). Human exposures to rare Earth elements: present knowledge and research prospects. Environ. Res. 171, 493–500. doi:10.1016/j.envres.2019.02.004
Pham, L. H., Nguyen, H. T., Tran, C. V., Nguyen, H. M., Nguyen, T. H., and Tu, M. B. (2017). Arsenic and other trace elements in groundwater and human urine in ha nam province, the northern vietnam: contamination characteristics and risk assessment. Environ. Geochem. Health 39, 517–529. doi:10.1007/s10653-016-9831-3
Rim, K. T. (2016). Effects of rare Earth elements on the environment and human health: a literature review. Toxicol. Environ. Health Sci. 8, 189–200. doi:10.1007/s13530-016-0276-y
Schmied, A., Murawski, A., Kolossa-Gehring, M., and Kujath, P. (2021). Determination of trace elements in urine by inductively coupled plasma-tandem mass spectrometry – biomonitoring of adults in the German capital region. Chemosphere 285, 131425. doi:10.1016/j.chemosphere.2021.131425
Takyi, S. A., Basu, N., Arko-Mensah, J., Dwomoh, D., Houessionon, K. G., and Fobil, J. N. (2021). Biomonitoring of metals in blood and urine of electronic waste (E-waste) recyclers at Agbogbloshie, Ghana. Chemosphere 280, 130677. doi:10.1016/j.chemosphere.2021.130677
Tong, S., Yang, L., Gong, H., Wang, L., Li, H., Yu, J., et al. (2021). Association of selenium, arsenic, and other trace elements in drinking water and urine in residents of the plateau region in China. Environ. Sci. Pollut. Res. 29, 26498–26512. doi:10.1007/s11356-021-17418-1
UNI CEI EN ISO/IEC 17025 (2018). General requirements for the competence of testing and calibration laboratories. Available at: http://store.uni.com/catalogo/uni-cei-en-iso-iec-17025-2018 (Accessed August 05, 2022).
World Medical Association (2013). Declaration of Helsinki – ethical principles for medical research involving human subjects. Available at: https://www.wma.net/policies-post/wma-declaration-of-helsinki-ethical-principles-for-medical-research-involving-human-subjects/ (Accessed August 05, 2022).
Keywords: rare earth elements, human urine, ICP—mass spectrometry, analytical method, method validation
Citation: Brouziotis AA, Giarra A, Marano A, Di Nunzio A, Lombardo F, Guida M, Libralato G and Trifuoggi M (2022) Comparative study of two methods for rare earth elements analysis in human urine samples using inductively coupled plasma-mass spectrometry. Front. Environ. Sci. 10:942441. doi: 10.3389/fenvs.2022.942441
Received: 12 May 2022; Accepted: 20 July 2022;
Published: 26 August 2022.
Edited by:
Andrew Hursthouse, University of the West of Scotland, United KingdomReviewed by:
Zikri Arslan, United States Geological Survey (USGS), United StatesTatiana Saint Pierre, Pontifical Catholic University of Rio de Janeiro, Brazil
Copyright © 2022 Brouziotis, Giarra, Marano, Di Nunzio, Lombardo, Guida, Libralato and Trifuoggi. This is an open-access article distributed under the terms of the Creative Commons Attribution License (CC BY). The use, distribution or reproduction in other forums is permitted, provided the original author(s) and the copyright owner(s) are credited and that the original publication in this journal is cited, in accordance with accepted academic practice. No use, distribution or reproduction is permitted which does not comply with these terms.
*Correspondence: Antonios Apostolos Brouziotis, YW50b25pcy5icm91emlvdGlzQG91dGxvb2suY29t; Marco Trifuoggi, bWFyY28udHJpZnVvZ2dpQHVuaW5hLml0
†These authors have contributed equally to this work and share first authorship