- 1Escuela Profesional de Ingeniería Ambiental de la Universidad Nacional de Moquegua, Moquegua, Perú
- 2Laboratorio de Contaminantes Orgánicos y Ambiente del IINDEP de la Universidad Nacional deMoquegua, Moquegua, Perú
- 3Programa de Doctorado en Ciencia, Tecnología y Medio Ambiente, Escuela de Posgrado, Universidad Nacional del Altiplano de Puno, Puno, Perú
Consumption of different pharmaceuticals has increased since the COVID-19 pandemic. Some health institutions worldwide approved the use of drugs such as ivermectin, hydroxychloroquine, azithromycin, dexamethasone, favipiravir, remdesivir, lopinavir-ritonavir, chloroquine, dexamethasone for the treatment of the virus. Once consumed by humans, these compounds are released in urine and faeces, ending up in wastewater and conducted to treatment plants or directly discharged without prior treatment into surface water and soil, with minimum values recorded between 7 ng/L and < 0.08 μg/L for azithromycin and ivermectin respectively, as well as dexamethasone with 0.73 ng/L in surface water and an average of 50–60 ng/L for favipiravir. Their presence has numerous toxicological effects on aquatic and terrestrial species, influencing population decline and altering the growth of organisms. However, the environmental consequences of pharmaceuticals in the environment are poorly known, especially for antivirals studied in this article. This work aims to analyze the presence, treatment and ecotoxicity of drugs used in the pandemic COVID 19, mainly focusing on aquatic and terrestrial ecosystems since that is where they arrive through wastewater. Ecotoxicological effects on flora, fauna and humans are also analyzed. Once there, they persist in the environment causing severe ecological damage, developmental and growth disorders in animals and plants and, in many cases, even the death of species.
1 Introduction
The new coronavirus pandemic (COVID-19) outbreak in Wuhan, China, has spread rapidly around the world (Zhao et al., 2020). The origin of its zoonosis dates back to ecosystem changes that have reduced biodiversity, greatly facilitating contact between humans and animal reservoirs, in this case, bats that carry pathogens, such as SARS-CoV-2 (Platto et al., 2021). Since then, efforts have mainly been directed to rapidly acquiring epidemiological, microbiological, pathogenetic, clinical, diagnostic, therapeutic, and preventive healthcare information to fight against pandemic features (Forestieri et al.,2021). There was no specific vaccine or antiviral medication for the treatment of COVID-19. Illness management strategies included supportive care, quarantine and prevention of complications of this disease (Mhadhbi et al., 2020), which mainly affects the lungs, but evidence of systemic disease affecting various organs such as the liver is emerging (Cheng et al., 2021).
In this new health situation, several drugs have been used for its treatment; among the most commonly used were hydroxychloroquine (Gautret et al., 2020), azithromycin (Rosendaal, 2020), remdesivir ceftaroline fosamil, ceftolozane/tazobactam, cefditoren, ceftriaxone, colistin, doxycycline and linezolid (Gonzalez-Zorn, 2021), and Ivermectin (Zaheer et al., 2021). Azithromycin is an antibiotic derived from erythromycin, belonging to the macrolide group and with a broad antibacterial spectrum (Mhadhbi et al., 2020). Hydroxychloroquine is an antimalarial drug with properties to treat autoimmune diseases such as lupus and rheumatism (El-Din Abuo-Rahma et al., 2020), and ivermectin is an antiparasitic for veterinary use (Jans and Wagstaff, 2021), Were also prescribed redeliver and dexamethasone in high amounts (Desgens-Martin and Keller, 2021). These drugs have also been used to treat COVID 19, although evidence of their effectiveness is limited in the case of Azithromycin and Hydroxychloroquine (Cavalcanti et al., 2020; Lagier et al., 2020). As a result, its consumption has increased (Gonzalez-Zorn, 2021), representing a significant environmental presence.
Today, several pollutants are present in the environment around the world (Rasheed et al., 2021), also in places like the European community (Rodriguez-Mozaz et al., 2020), prompting regulatory organisms to impose increasingly stringent limits on water (Díaz-Cruz and Barceló, 2007; Olu-Owolabi et al., 2021). Antibiotics are widely used in human medicine, animal husbandry, agriculture and aquaculture (Vilca et al., 2021); their residues have become a global environmental problem. However, antibiotics in the aquatic environment are not yet well known (Díaz-Cruz and Barceló, 2007). These drugs are also found in terrestrial ecosystems, as they reach them through irrigation and fertilization with biosolids from wastewater treatment plants (Gottschall et al., 2012) and animal manure used to fertilize agricultural fields (Liu et al., 2016). Moreover, that contaminates the crop fields with the residual medicines they contain, thus affecting mainly the life that develops in the soil (Tarazona et al., 2021); or using manure from the excrement of animals treated with these drugs. So it is common for soils to become contaminated with drug residues after being fertilized with animal manure (Sun et al., 2021).
The glucocorticoid, dexamethasone has generated significant concern due to its widespread contamination in the environment and its application in treating patients with COVID-19 (Gao et al., 2022). Dexamethasone is a pharmaceutical compound prescribed in human medicine for allergies, asthma and autoimmune disorders (Isobe et al., 2015), also in veterinary medicine to restore muscle strength. As a growth promoter in marine aquaculture farms (Chang et al., 2007), this compound’s residue can contaminate natural watersheds through inputs from wastewater treatment facilities and confined animal feeding operations (Lalone et al., 2012). As well as antivirals such as lopinavir/ritonavir for its antiviral property, or its immunomodulatory property, such as dexamethasone, for its wide use to treat diarrhoea caused by lopinavir/ritonavir (Domingo-Echaburu et al., 2022), in addition to other antivirals as shown by clinical studies, Favipiravir and Remdesivir are new antiviral drugs that are effective against COVID-19 (Emam et al., 2022).
Unfortunately, the population’s use of these drugs to treat COVID-19 has been uncontrolled, with self-medication being one of the primary forms of consumption. This has led to an increase in the consumption of drugs, consequently increasing their residues in the environment. Furthermore, although the toxicological effects are not yet described in detail, it is known that these pharmaceuticals are toxic for flora and fauna species, which is necessary to evaluate to treat this type of residue better. This review compiles information on the presence of residues of these drugs in aquatic and terrestrial ecosystems, as they reach them through wastewater and the use of biosolids as fertilizer, and also analyzes their ecotoxicological effects on flora, fauna and humans in order to have a better understanding of these effects in our environment.
2 Methodology (sources of information and research)
The literature review process was carried out directly in databases such as Science Direct, Scopus, Pubmed, Springer, ACS and Taylor & Francis, through scientific and review articles reporting the detection and treatment technologies of drugs used in the treatment of COVID-19. The selection of drugs was developed from information disseminated by the World Health Organization and studies warning pharmacological treatment of COVID-19. In the present review, articles published in the last ten years were selected; where the search included keywords such as Azithromycin, Chloroquine, Hydroxychloroquine, Ivermectin, Dexamethasone, Remdesivir, Favipiravir, Water, Wastewater, Effluents, River, Soils, Detection And Environmental Matrices, COVID-19, SARS-CoV-2, Drugs, Antivirals, Antibiotics, Antiparasitics, Antiprotozoals, Glucocorticoids, Presence, Occurrence, Degradation, Ecotoxicity, Plants, Biota, Disposal, Removal and Treatment, where the search was done individually for each compound, maintaining the order in work (Figure 1).
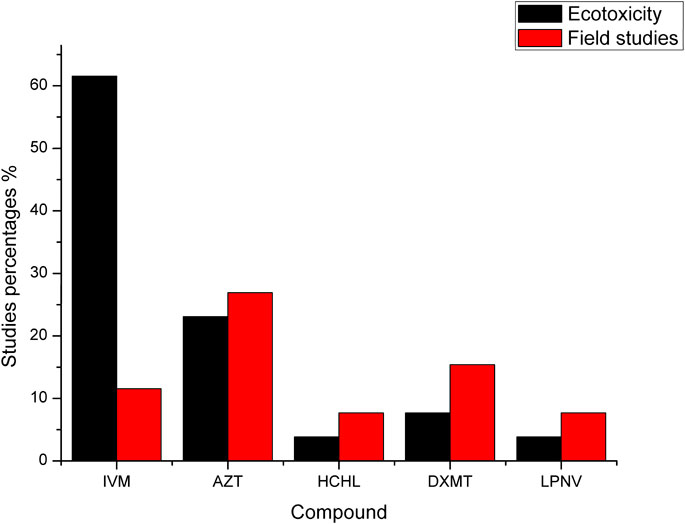
FIGURE 1. In laboratories, the percentage of studies on the presence of ivermectin, azithromycin, hydroxyquinone, Dexamethasone and Antivirals toxicity studies.
3 Pharmaceuticals used in pandemic
The macrolide antibiotic azithromycin has been a recommended treatment against COVID-19, along with hydroxychloroquine or redeliver (Cavalcanti et al., 2020). Other drugs, such as the mentioned hydroxychloroquine or chloroquine with or without azithromycin, have been widely used to treat COVID-19 after the emergence of the first in vitro antiviral effects against Severe Acute Respiratory Syndrome Coronavirus 2 (SARS-CoV-2) (Fiolet et al., 2021), increasing its consumption by up to 2,000% in the United States between March 2019, and March 2020 (Cavalcanti et al., 2020). Another study mentions that March 2020 was the month with the highest rate recorded for azithromycin consumption since January 2017 in Spain; it was 400% of the use of this molecule in February 2020 and more than 320% of the consumption in January 2019 (Gonzalez-Zorn, 2021). Additionally, due to the efficiency of antiviral treatments, the U.S. FDA approved chloroquine and hydroxychloroquine in the emergency clinical management protocols used in COVID-19, but with some restrictions (El-Din Abuo-Rahma et al., 2020). Another drug that has been used in large quantities to reduce the spread of COVID-19 is ivermectin (Zaheer et al., 2021), since its approval by the U.S. FDA due to its potential as an antiviral, in this pandemic where a clinical benefit has been proven in some trials (Jans and Wagstaff, 2021). Ivermectin has also been effective against specific flaviviruses (dengue, Japanese encephalitis, and tick-borne encephalitis virus) and the chikungunya virus (Gupta et al., 2020).
However, using these drugs does not show conclusive results concerning the improvement of Covid patients (Zaheer et al., 2021). Such is the case of using ivermectin, in which its efficacy was compared with a control group. The results indicated that there was no significant difference between the control group and the group treated with ivermectin, although there was a slight trend of reduction in the length of hospital stay in the group treated with this drug, indicating that this drug can be used as additional therapy in the treatments used to treat COVID 19 (Abd-Elsalam et al., 2021). Similar results were found for hydroxychloroquine and azithromycin applied together and alone; even high doses of chloroquine (600 mg twice a day) are associated with high mortality (Abd-Elsalam et al., 2021). Therefore, despite the lack of concrete evidence of their effectiveness, these drugs have been and continue to be widely used to treat COVID 19, generating residues in the environment.
The recent COVID-19 pandemic has shown that dexamethasone effectively reduced postinfection hyper inflammation, resulting in a significant decrease in mortality (Johnson and Vinetz 2020), as were other selected antiviral drugs such as remdesivir (a nucleoside analogue prodrug), oseltamivir (a potent and selective influenza A and B neuraminidase inhibitor, also known as Tamiflu), lopinavir (a second-generation protease inhibitor), darunavir (a second-generation protease inhibitor), and umifenovir (a dual-acting direct antiviral and multi-host targeted agent) (Reis et al., 2019). Among these, several studies suggest that remdesivir is an antiviral drug against Ebola but may also be effective in shortening recovery time in hospitalized adults with COVID-19 (Beigel et al., 2020), as well as lopinavir and Ritonavir alone or in combination with other drugs, causing an increase in the concentration of antiviral drugs in hospital wastewater and effluent (Czech et al., 2022).
3.1 Physical and chemical properties
3.1.1 Ivermectin
Since 1987, this compound has been widely used in veterinary medicine, and its use in humans has become widespread (Vokřál et al., 2019). Thus, nearly 3.7 billion doses of ivermectin have been distributed worldwide in mass drug administration (MDA) campaigns over the past 30 years (Nicolas et al., 2020). Ivermectin is a specific inhibitor of importin α/β-mediated nuclear transport and shows antiviral potential against various RNA viruses by blocking the nuclear localization of viral proteins (Lv et al., 2018). Its primary use is antiparasitic, anti-helminthic (Howard 2007; Vokřál et al., 2019), and to treat diseases caused by several single-strain RNA viruses in humans (Barrows et al., 2016).
Ivermectin is a lipophilic substance and dissolves better in organic solvents (Fisher and Mrozik 1989), with particular physicochemical characteristics. Generally, the physicochemical properties of compounds, such as water octanol coefficient (Kow), distribution coefficient (Kd), dissociation constant, as well as vapour pressure or Henry’s Law constant (KH), contribute to determining whether a substance has the affinity to concentrate further in water, soil or air (Díaz-Cruz and Barceló, 2007). The vapour pressure of ivermectin is less than or equal to 1.5 × 10−9 mmHg, which indicates that this compound is unlikely to be volatile and distributed in the atmosphere (Bloom and Matheson, 1993). With a pKa value of around 6.5, it is more soluble in very alkaline media (Rolim et al., 2014). Its solubility in water is low, having a solubility value less than or equal to 4 mg/L (Fisher and Mrozik, 1989). The sorption coefficient (Koc), ranging from 1.26 × 104 y 1.57 × 104, indicates a greater affinity for soil (Krogh et al., 2008). Also, high values of the octanol/water partition coefficient (Kow) and the distribution coefficient (KD) indicate that the substance has a higher affinity for soil (Díaz-Cruz and Barceló, 2007), where it can even form complexes with the inorganic matter in the soil (Krogh et al., 2008). Therefore, these values show that this compound has a greater affinity for the soil than in aquatic environments (Brinke et al., 2010).
3.1.2 Azithromycin
Azithromycin is an antibiotic with a broad spectrum of applications and is used to combat various infections caused by Gram-negative and Gram-positive bacteria (Cano et al., 2020). So it has also been considered therapy for treating COVID-19 (Vouri et al., 2021) and even mixed with hydroxychloroquine (Lagier et al., 2020).
Azithromycin is an antibiotic belonging to the macrolide group (Jafari Ozumchelouei et al., 2020). It is synthesized by incorporating a nitrogen atom into the lactone ring of erythromycin A, its chemical name is 9-deoxy-9a-aza-9a-methyl-9a-homo-erythromycin A, and its molecular weight is 748.98 (Jafari Ozumchelouei et al., 2020). The pKa value is 8.1 and 8.8, which shows that at low pH, azithromycin is in a molecular form and tends to attract positive charges, influencing its higher removal by adsorption of positive charge (Cano et al., 2020). Likewise, protonated azithromycin can hardly cross cell membranes, so a neutral to primary medium is necessary for the molecules to cross cell membranes (Derendorf, 2020). Additionally, it is worth mentioning that high Kd values indicate a higher affinity for sediment or soil materials than water, as in this case (Jafari Ozumchelouei et al., 2020). Therefore, we will likely find higher concentrations in sediments and soils than in water. These physicochemical characteristics of the three target antibiotics are found in Table 1.
3.1.3 Hydroxychloroquine
Hydroxychloroquine, approved by the Food and Drug Administration (FDA) as an antimalarial drug, was reused to treat COVID-19 patients (da Luz et al., 2021; Mendonça-Gomes et al., 2021). Cell culture experiments have shown solid antiviral action of hydroxychloroquine against a variety of viruses, including HIV-1, dengue, Zika and West Nile viruses, Venezuelan equine encephalitis virus, Chikungunya, pseudorabies virus, adenovirus, and SARS-CoV-2 (COVID-19) (Gautret et al., 2021; Jans and Wagstaff 2021), in dermatology and rheumatology and emerging roles in oncology (Yusuf et al., 2017). It can control and reduce inflammatory pathways (Danza et al., 2016). Hydroxychloroquine is likely to slow the severe progression of COVID-19, inhibiting the cytokine storm by suppressing T-cell activation. Also, it has a safer clinical profile and is suitable for pregnant women (Zhou et al., 2020). On the other hand, the combination of hydroxychloroquine with azithromycin can give some pharmacological interactions; that is why a study should be done on patients with heart disease, abnormal electrocardiogram, dyslexia or the routine use of other interacting drugs, all this before its application (Gautret et al., 2021).
Hydroxychloroquine has three nitrogens in its chemical structure, with pKa values of 4.0, 8.3 and 9.7; however, only high values can be protonated, affecting its permeability in cell membranes’ intracellular distribution (Derendorf, 2020). The Kow value (3.84), shows that it has a lipophilic tendency. This property can effectively bind to tissues, resulting in high distribution volumes as in blood and plasma (Alves da Silva et al., 2021). Concerning water solubility, it can reach up to 26.1 mg/L. Therefore, the evaluated compound has an affinity for water and even shows a lipophilic characteristic (Alves da Silva et al., 2021); this represents a greater risk for the species that inhabit the ecosystems.
3.1.4 Dexamethasone
The glucocorticoid dexamethasone has pharmacological properties similar to cortisol, a natural steroid (Dusi et al., 2011), so they form an essential group of these drugs and have a wide range of therapeutic applications, such as in the treatment of asthma or other inflammatory diseases. However, they also have an essential effect as growth promoters (Herrero et al., 2012). Dexamethasone was obtained by modifying the initial structure of cortisol by introducing the 9-α-fluoro group, a 16-α-methyl substituent, and an extra double bond between carbon 1 and 2 on the A-ring (Table 1). Dexamethasone binds glucocorticoids more efficiently than cortisol; the presence of the fluorine atom makes it more lipophilic, while the methyl group attached to the C-16 carbon increases its affinity for the mineralocorticoid receptor (Rang et al., 2012).
3.1.5 Antivirals (favipiravir, lopinavir, ribavirin, remdimisir)
Lopinavir is an antiretroviral protease inhibitor (C37 H48N4O5, molecular weight of 628.80 g/mol, logKOW of 5.94), which is often used in HIV treatment in combination with Ritonavir which is an HIV protease inhibitor (C37H48N6O5S2, molecular weight of 720.94 g/mol, logKow of 6.29) to increase its half-life by inhibiting its degradation by the cytochrome (Krumm et al., 2021). On the other hand, the antiviral lopinavir is reported with a concentration of 0.033 μg/L in the environment; thus, the Swedish environmental classification of pharmaceuticals states that lopinavir has a high bioaccumulation potential (log Dow = 4.7, which is > 4) (Domingo-Echaburu et al., 2022). At the same time, favipiravir is an antiviral drug used to combat influenza due to its influenza virus RNA polymerase inhibitory properties (Madelain et al., 2020).
4 Presence of pharmaceutical residues used to treat COVID 19
4.1 Aquatic ecosystem
Today, several emerging pollutants are present in the environment around the world, prompting regulatory organisms to impose increasingly stringent limits on water (Rodriguez-Mozaz et al., 2020; Olu-Owolabi et al., 2021). Antibiotics are widely used in human medicine, animal husbandry, agriculture and aquaculture (Vilca et al., 2021), and their residues have become a global environmental problem. However, antibiotics in the aquatic environment are not yet well known (Díaz-Cruz and Barceló, 2007). One of the most significant problems of the widespread use of antibiotics that causes environmental contamination is the alarming increase in antibiotic-resistant infections (Martinez, 2009). For example, they were Having azithromycin reports 7 ng/L in surface water and 130 ± 46 ng/L in treatment plant effluents due to the consumption and sales of pharmaceuticals in Italy, together with data related to their excretion and disposal during wastewater treatment (Verlicchi et al., 2014), 2.4 μg/L in water samples, 2,956.5 μg/L in industrial effluents, since they are released from the human body through urine and faeces as a mixture of the unchanged parent compound and various metabolites (Senta et al., 2017), 0.14 μg/L in wastewater input to WWTP attributed to the increase in human consumption of personal care pharmaceutical products (Golovko et al., 2014), and 4.29 μg/L in Msunduzi River (Al Aukidy et al., 2012), and listed by the USEPA as an emerging contaminant since 2009 (Guo et al., 2018). This antibiotic is also known to be one of the antibiotics found in high concentrations in water samples (Pereira et al., 2017; Mao et al., 2021). For this reason, it is even included in the European Union’s water policy watch list (González-Pleiter et al., 2021); this indicates that its presence in the environment is dangerous.
A similar situation is presented concerning ivermectin, given that since the end of March 2020, its use for the treatment of COVID 19 has been confirmed, which has had an impact on its high consumption by the population (Essid et al., 2020), which in turn has influenced the increase of waste in the aquatic environment (Olu-Owolabi et al., 2021). Moreover, hydroxychloroquine is a well-known antimalarial and antirheumatic drug that has recently garnered unprecedented attention as a potential therapeutic agent against viral infections (Perrone et al., 2020) COVID 19 disease. Increased consumption of hydroxychloroquine results in increased residues in the aquatic environment through wastewater (Bensalah et al., 2020). Therefore, the residues of these drugs used to treat COVID 19 end up in aquatic ecosystems due to ineffective treatment at the wastewater treatment plant. The reported concentrations can be seen in Table 2.
Glucocorticoids, including dexamethasone, enter the aquatic environment mainly through excretion of the compound and its metabolites from patients and, to a lesser extent, from use in livestock and aquaculture (Liu et al., 2015), where the highest reported concentrations of the glucocorticoid family were measured in untreated hospital wastewater in Switzerland, where a total concentration of 3,423 ng/L, comprising 1,720 ng/L of dexamethasone, was found (Macikova et al., 2014). Considering the data presented by (Carpagnano et al., 2021), the increased use of antivirals such as lopinavir and Ritonavir during the COVID-19 pandemic increased their concentrations in hospital wastewater and effluents (Figure 2).
4.2 Terrestrial ecosystem
These drugs are also found in terrestrial ecosystems, as they reach them through irrigation and fertilization with biosolids from wastewater treatment plants (Gottschall et al., 2012) and animal manure used to fertilize agricultural fields (Liu et al., 2016). Moreover, they contaminate the crop fields with the residual medicines they contain, thus affecting mainly the life that develops in the soil (Tarazona et al., 2021); or using manure from the excrement of animals treated with these drugs. So it is common for soils to become contaminated with drug residues after being fertilized with animal manure (Sun et al., 2021). Such is the case of a study in which dry biosolids from a wastewater treatment plant were applied to determine the concentrations of drug residues in the water, soil and wheat grown in the area, finding a total of 80 residues of pharmaceutical and personal care products, including azithromycin in soil samples (Sun et al., 2021). However, trace residues of drugs, including azithromycin, have also been found in soils that are not influenced by wastewater treatment plant discharges, which explains that there are other non-point factors related to anthropogenic pressure and its different activities that contribute to the presence of these compounds (Yi et al., 2019). Because this compound arrives on agricultural land through irrigation water contaminated with ivermectin; for example, a study shows different water ecosystems polluted with ivermectin < 0.08 μg/L (Imanda reservoir outlet), 6.57 μg/L (Río Umgeni) and < 0.08 μg/L (Blue lake), this for medication in humans and accumulation of animal tissues (Omotola and Olatunji, 2020) which represent risks for environment (Table 2).
5 Ecotoxicological aspects of the drugs used for Covid
5.1 Ivermectin
5.1.1 Toxicity to aquatic organisms
During the COVID-19 pandemic in 2020, ivermectin was used in more significant quantities than in previous years; if it continues to be widely used during this pandemic, high amounts of ivermectin could be deposited in the water ecosystems (Essid et al., 2020). This compound exerts acute and chronic toxic effects on aquatic organisms and can cause changes in biological diversity and ecosystem functioning (Goessens et al., 2020). Due to its widespread use and poor metabolism in animals, the toxicity of ivermectin in aquatic environments has received increasing attention. The primary source of potential toxicity is the accumulation of animal tissues and the excretion of urine and faeces in the environment (Zhang et al., 2020). In the aquatic environment system, ivermectin represents an ecological risk for aquatic organisms (biota) (Mesa et al., 2017) because it can cause toxicity in living beings. One study found that ivermectin can cause oxidative damage, including cytotoxicity and genotoxicity, through direct DNA damage (Zhang et al., 2020). In addition to acute and chronic toxicity in freshwater (Bundschuh et al., 2016).
Their rapid sorption to sediment particles and high persistence in aquatic sediments have raised concerns about benthic and meiobenthic organisms (Brinke et al., 2010). Ivermectin represents an ecological risk to aquatic ecosystems, underscoring the need for management strategies for its use to limit its entry into water bodies (Mesa et al., 2017).
5.1.2 Toxicity to terrestrial organisms
Ivermectin binds to the soil by forming complexes with immobile inorganic matter (Krogh et al., 2008) and persists in the environment with toxic effects on terrestrial invertebrate species (Guimarães et al., 2019). (Verdú et al., 2018) report that at a concentration of 100 μg/kg, they cause profound short- and long-term ecological effects, decreasing dung beetle populations, manure degradation processes and soil properties and functions. Therefore, it is necessary to continue developing practices management that reduces the potential risks associated with these highly toxic compounds (Bai and Ogbourne, 2016). The terrestrial ecosystems’ contamination occurs because this compound’s residues continue to be discharged into the soil through sewage irrigation and contaminated manure (Zhao et al., 2019). This compound is an antiparasitic widely used in livestock; animals excrete it through manure and negatively affect the survival and reproduction of organisms that degrade manure, such as dung beetles and entomopathogenic nematodes (González-Tokman et al., 2017; Villada-Bedoya et al., 2021). Ivermectin affects the survival of Pristionchus maupasi (Kar et al., 2020), Eisenia fétida (Zhao et al., 2019), Folsomia candida (Guimarães et al., 2019), Hypoaspis aculeifer (Römbke et al., 2010), Thorectes lusitanicus (Verdú et al., 2020), Sepsis punctum (Conforti et al., 2018), Enchytraeus crypticus, Folsomia fimetaria, Hypogastrura assimilis (Jensen and Scott-Fordsmand, 2012), among others.
This situation has led to greater attention being paid to the toxic effects that their presence in the environment could trigger, so several ecotoxicity studies have been conducted (Römbke et al., 2010; Syslová et al., 2019; Vokřál et al., 2019; Villada-Bedoya et al., 2021) or in species communities (Brinke et al., 2010). This allows us to understand the magnitude of this compound’s damage to the terrestrial ecosystem.
5.1.3 Toxicity of ivermectin in humans
This compound has toxic effects on humans since the ingestion of ivermectin is associated with the capacity to generate teratogenesis, congenital anomalies and abortions (Nicolas et al., 2020), which is corroborated by studies where it was found that miscarriages and infant malformation are associated with accidental ingestion of ivermectin by mothers (Gyapong et al., 2003). Therefore, there is a severe risk for pregnant women who accidentally consume this drug directly or indirectly through food and water.
5.2 Azithromycin
5.2.1 Toxicity to aquatic organisms
Lakes and rivers are sources of livelihoods, food and water in many parts of the world, and these aquatic ecosystems are vulnerable to new and known environmental pollutants as emerging water pollutants, including antibiotics (Vilca et al., 2021). Since azithromycin is a primary contaminating antibiotic in water, its toxicity to aquatic organisms has been investigated (Li et al., 2020). The presence of this antibiotic in environmental matrices and the effluents of conventional wastewater treatment plants has been evidenced in recent years, which leads to the need to develop new treatment alternatives that allow its total removal and minimize the eventual adverse effects, as well as a selection of resistant bacterial strains, associated with their presence in bodies of water (Cano et al., 2020).
The presence of azithromycin and hydroxychloroquine at 12.5 μg/L affects wild aquatic life, such as neotropical tadpoles of Physalaemus cuvieri species. In fishes like Zebrafish (Danio rerio), azithromycin and hydroxychloroquine at 2.5 μg/L induce protein reduction, redox imbalance, and possible oxidative stress (Mendonça-Gomes et al., 2021). In the European Sea, Bass (Dicentrarchus labrax) azithromycin causes oxidative stress, peroxidative damage and neurotoxicity at 20 mg/L, inducing 18% and 7.5% of larvae mortality and morphological abnormalities, respectively (Mhadhbi et al., 2020). They affect the microfauna inhibit the growth of algae, and microalgae reduce energy reserves (proteins, carbohydrates and lipids) and deteriorate the cellular structure of Chlorella pyrenoidosa (Mao et al., 2021). Another study suggests a common concern for chloroquines and dexamethasone while a very high environmental impact for ivermectin and azithromycin, even at use levels below the default value of 1% of the population (Tarazona et al., 2021). On the other hand, contamination with microplastics is observed, so these are exposed to a variety of other pollutants, which can transport and react with antibiotics such as azithromycin in the aquatic environment (González-Pleiter et al., 2021).
In other research carried out with 53 analyzed antibiotics in the sampling stages, 17 were detected in the treated wastewater effluents, and one of them was azithromycin (Rodriguez-Mozaz et al., 2020), which is considered a marker of contamination; this is why proper wastewater treatment systems are essential (Pazoki et al., 2016). Similarly, macrolides, including azithromycin, which is considered genetically toxic to fish, have been reported to bioaccumulate in fish tissues, as well as in aquatic environments such as rivers and surface waters, also causing damage to developmental, cardiovascular systems and metabolic, as well as in the alteration of the antioxidant and immune responses of fish (Yang et al., 2020). These studies show that azithromycin has toxic effects on living organisms (Bai and Ogbourne, 2016), and its presence in the environment represents a severe environmental and human health problem.
5.2.2 Toxicity to terrestrial organisms
Azithromycin has toxic properties frequently detected in municipal biosolids and identified by the USEPA as an emerging contaminant (Gravesen and Judy, 2020). Soil toxicity is mainly due to manure fertilizers and the reuse of domestic wastewater in crop irrigation (Ezzariai et al., 2018). Pharmaceutical effluents alter the physicochemical characteristics and the bacterial community of the river sediments, which contribute to the enrichment of genes for antibiotic resistance (Sun et al., 2021). The bioavailability of azithromycin for terrestrial organisms is often limited in the context of biosolids, but they can alter and enter the ecological food web. For example, a study with earthworms exposed at 0.03–0.16 mg/kg (dw) shows minimal toxicity in earthworms’ microbial functioning (Sidhu et al., 2019).
The widespread presence of azithromycin in soils is a growing concern for public health, and the amount that exists is directly related to the density of the population (Yi et al., 2019). Soil vulnerability to antibiotic contamination is essential in determining the extent of contamination and the likelihood of antibiotic resistance and ecotoxicological effects (De La Torre et al., 2012). In general, soil exposure to macrolide antibiotics such as azithromycin increases the relative abundance of numerous genetic targets associated with resistance to macrolides and other antibiotics and mobile genetic elements (Sun et al., 2021). Likewise, biosolids or composting expose living organisms to the toxic effect of pharmaceutical products such as azithromycin (Buta et al., 2021). Meanwhile, fertilizer colloids, porous areas and soil pH are the main factors influencing the transport behaviours of antibiotics; also, the biodegradation of antibiotics in the soil is highly dependent on the soil microbiome, soil pH, and soil temperature, and interactions between antibiotics (Zhi et al., 2019).
5.2.3 Toxicity of azithromycin in humans
Azithromycin is an antibiotic that inhibits protein synthesis in bacteria. It reduces biofilm formation (Parnham et al., 2014), whose primary threat of presence in the environment is the generation of antibiotic-resistant genes (Martinez, 2009), which can occur in the environment and humans when they have a prolonged treatment, i.e., bacterial resistance can occur in the patient’s own body (Li et al., 2014) or also generate sensitivity to the drug, and in some cases heart problems (Parnham et al., 2014). Therefore, its administration should be prudence, thus protecting environmental and human health.
5.3 Hydroxychloroquine
5.3.1 Toxicity to aquatic organisms
Pharmaceutical compounds such as hydroxychloroquine have become emerging environmental pollutants due to humans’ massive administration and more remarkable persistence in the environment. Following the massive and widespread use of hydroxychloroquine to treat COVID-19 worldwide (Lagier et al., 2020; Mendonça-Gomes et al., 2021), an appreciable high amount is expected to end up in sewage systems in coastal marine areas (Table 3). It may significantly affect the meiobenthic nematode community, both quantitatively and qualitatively (Ben Ali et al., 2021), thus affecting the diversity of these species and the community structure itself, which affects the functioning of the ecosystem. This compound and its metabolites in the aquatic environment harm all organisms. In addition, the ease of arrival of these substances into the environment can also severely affect public health (Bensalah et al., 2020).
5.3.2 Toxicity to terrestrial organisms
Due to intensive use and continuous release, high and persistent concentrations of antibiotics are found in soils worldwide; this contamination exacerbates the risks associated with exposure and antibiotic resistance to soil ecosystems and human health (Zhi et al., 2019). Ecological impacts can range from subtle to overt due to acute exposure to higher levels of hydroxychloroquine, such as in the case of ingestion by wildlife of improperly disposed waste (Daughton, 2014). Sludge and manure are the primary sources of contamination by antibiotics of this type in all environment compartments (soil, sediment, surface and groundwater) (Ezzariai et al., 2018).
5.3.3 Toxicity of hydroxychloroquine in humans
Prolonged exposure during treatment can cause damage to health, such as the case of a study in which they show that there are risks related to prolonged use of hydroxychloroquine, such as choriocapillaris degeneration eyes with retinopathy disease (Ahn et al., 2019). Similarly, in another study of prolonged exposure to hydroxychloroquine (10.4 years on average), patients were found to experience toxic effects on the retina, which diminished somewhat when the medication was discontinued (Mititelu et al., 2013); toxic effects are not only seen in the eyes. In another study conducted on patients with rheumatoid arthritis, it was found that those treated with hydroxychloroquine had more significant bone erosion than the other group treated with another drug (Van Der Heijde et al., 1989). Therefore, voluntary exposure, in long-term treatments, or involuntary exposure, through the environment, to hydroxychloroquine leads to toxic effects in humans.
5.4 Dexamethasone
5.4.1 Toxicity to aquatic organisms
Dexamethasone (DEX) is a class of steroid hormones that can be potentially harmful due to their endocrine-disrupting properties (Quaresma et al., 2021). So (Lalone et al., 2012) evaluated the toxicity of dexamethasone by mimicking environmental conditions and showed that concentrations of 500 μg/L can alter fish’s reproduction, growth, and development. Chronic exposure to glucocorticoids within them dexamethasone has also been found to cause reproductive abnormalities in rainbow trout, tilapia, carp, and goldfish and can also make the fish more aggressive (Dunlap et al., 2002).
5.4.2 Toxicity to terrestrial organisms
Different environments such as soils are reservoirs for probably thousands of emerging contaminants, including dexamethasone released along with treated/untreated wastewater, and despite extensive studies on dexamethasone in surface water and wastewater, other environmental compartments remain to be thoroughly investigated (Biswas et al., 2022). Soil characteristics, including pH, organic matter content, clay content, and redox potential, may influence the transport and fate of this compound (Nag et al., 2022). In the search on this topic, it was found that there is little information on dexamethasone in environments such as sediments, so comprehensive reviews of the evidence on environmental health risks in terrestrial environments are lacking.
5.4.3 Human toxicity of dexamethasone
The use of antiviral drugs and related therapeutic agents increases exponentially during pandemics; considering the global spread, the assessment of the environmental impact of treatment drugs for COVID-19 is very relevant (Tarazona et al., 2021). In humans, dexamethasone has an elimination half-life ranging from 1 to 5 h, although the biological half-life can last up to days, where its biological effects on anti-inflammatory activity are known to continue for 36–72 h after dosing (Becker, 2013). Excessive levels of dexamethasone negatively feedback the hypothalamus, resulting in reduced production of corticotropin-releasing hormone and adrenocorticotropic hormone (Cain and Cidlowski 2015). Non-specific administration of dexamethasone can cause several side effects, such as obesity, diabetes, immunosuppression, hypertension, and osteoporosis, making it difficult to use in long-term therapy (Numpilai et al., 2017).
Therefore, due to the constant detection of this compound in surface waters and the potential toxicity to humans and animals, the efficient removal of dexamethasone from drinking water is a relevant environmental concern.
5.5 Antivirals (favipiravir, lopinavir, ribavirin, remdesivir)
5.5.1 Toxicity to aquatic organisms
Considering their negative impact on: algae, Daphnia and fish, antiviral drugs were reported to be the most dangerous and toxic pharmaceuticals (Zhou et al., 2015). Studies reported that the administered drugs are not completely metabolized in the human body, generating residues and metabolites. The drug residue and metabolite are discharged to the environment through wastewater, which generates peaks of antiviral drugs in wastewater and environmental waters (Race et al., 2020), where studies report that Ritonavir shows the highest chronic toxicity to aquatic organisms (2.9 ng/L), followed by lopinavir (5.9 ng/L), and Ritonavir (20 ng/L) (Kuroda et al., 2021). Also, due to the ionic structure of antiviral drugs, a high bioaccumulation factor of Ritonavir and lopinavir in fish was estimated, implying that hydrophobic or lipophilic chemicals bioaccumulate favourably in fish tissues (Kumari and Kumar, 2021). (Kuroda et al., 2021) conclude that water treated through urban water treatment plants could contain high concentrations of these drugs and their metabolites (4,231 ng/L Favipiravir, 730 ng/L Lopinavir, 7,402 ng/L Ribavirin and 319 ng/L Remdesivir).
5.5.2 Toxicity of antivirals to humans
If several pharmaceuticals that have a similar mode of action are present in environmental waters, whether surface or subsurface, then the toxicity of this mixture is greater than that of any one pharmaceutical present; this could result in an underestimation of risk, as typical exposure to multicomponent chemicals would be an unacceptable risk to habitats and human health (Oliver et al., 2015). One of these, Ritonavir, is the primary drug responsible for risk to human health through consuming food (in the form of fish) grown in pharmaceutically contaminated waters (Kumari and Kumar, 2021). Much research has been conducted on SARS-COV-2, the causative agent of COVID-19, but none of the reported studies has addressed the ecological-human risk aspects with actual concentrations (Kumari and Kumar, 2021).
Furthermore, concerning toxicological studies, more were performed with ivermectin. This could be because there are more studies on antibiotics to detect their presence and the generation of bacterial resistance, which is the most significant risk for the environment and human beings (Martinez, 2009). At the same time, ivermectin stands out for its toxicological effect on the environment and human beings, such as Folsomia candida (Guimarães et al., 2019) and its effects during the pregnancy period (Nicolas et al., 2020), which is why it has so many toxicological studies. Furthermore, concerning hydroxychloroquine, it is a drug used specifically for some diseases, i.e., used in smaller proportions, so it is understood that it has less interest in studies of residues in the environment; also, concerning its toxicological effects, the studies that have been found, highlight toxic effects for humans mainly during prolonged use (Mititelu et al., 2013).
However, in this context of the pandemic caused by COVID 19, the use of drugs has increased, so further studies are needed on their presence in the environment, and the toxic effects on living beings, mainly hydroxychloroquine, since its physicochemical characteristics indicate that it can be found in soil and water, depending on the pH. Therefore, it is necessary to carry out further studies on its presence in the environment and its toxicity to prevent future environmental and human health damage.
6 Potential entry of ivermectin, azithromycin and hydroxychloroquine into a trophic chain
Many antibiotics have been detected in the environment due to their wide use (Díaz-Cruz and Barceló, 2007). In addition to being used for human therapy (azithromycin, hydroxychloroquine), pharmaceuticals are widely used in livestock (ivermectin) and agriculture (Martinez, 2009). These veterinary and pharmacological drugs enter the environment and can affect living organisms, including plants (Syslová et al., 2019). Antibiotic build-up in soil and plants is a growing problem in agriculture and is receiving increasing attention (Sun et al., 2021); antibiotics and other pharmaceuticals are the most abundant in sludge and compost (Ezzariai et al., 2018). In this context, plants, mainly those of agricultural interest, will be exposed to these compounds when they are present in the soil or irrigated with contaminated water (Sun et al., 2021).
Pharmaceuticals often contaminate agricultural products and enter the food chain through absorption by plants (Khan et al., 2021). The research detected 16 of the 28 antibiotics, erythromycin, in plant tissue samples. These 16 antibiotics were detected in root samples, eleven in stem samples, and nine in leaf samples (Chen et al., 2020). Environmental contamination by antibiotics may worsen in the future in the context of global change, with population growth, agricultural and industrial activity intensification, and water shortages in vulnerable areas (Rodriguez-Mozaz et al., 2020). If ivermectin is widely used to suppress the spread of COVID-19, high amounts of ivermectin will likely be deposited in the sea (Essid et al., 2020), directly affecting the food chain (Bai and Ogbourne, 2016). These wastes directly affect primary producers, such as algae, which form part of the base of the food chain (Li et al., 2020). Moreover, they reach humans through foodstuffs such as fish (Yang et al., 2020), causing severe health problems. Therefore, exhaustive studies are needed to evaluate the effects of these compounds on the trophic chain of the various terrestrial and aquatic ecosystems to know the environmental risks and take mitigation measures.
7 Treatment technologies for the pharmaceuticals studied
The increased presence of drugs in the environment is a severe potential hazard due to their toxicity and persistence, as studied in the review. Unfortunately, conventional treatment techniques, such as those used in wastewater treatment plants are ineffective for treating wastewater containing antibiotics. Recently, some technologies for removing these compounds have been discovered, and one of them is based on algae, a sustainable and promising technique for its removal (Li et al., 2022). Adsorption and photodegradation are two ideal methods for antibiotic treatment in water because they are: inexpensive, easy to operate and reusable, while metal-organic structures are excellent adsorbents and photocatalysts due to their high porosity, adaptability and good crystalline form (Du et al., 2021), likewise ozonation, Fenton/photo-Fenton and semiconductor photocatalysis are the most proven and efficient methodologies (Homem and Santos, 2011) as well as a variety of graphene-based nanomaterials and advanced oxidation processes efficiently remove antibiotics (Wang et al., 2019; Rasheed et al., 2021). A new family of 2D-MXenes materials has immense potential as adsorbents for the removal of compounds, and adsorption is one of the most promising purification approaches due to its excellent removal efficiency, simplicity and low cost under ambient conditions (Rasheed et al., 2022).
On the other hand, the confinement and non-use of the vehicle fleet during the pandemic had positive impacts on the environment, particularly in the context of air quality due to the reduction in concentrations of particulate matter (PM), NO2 and CO in significant cities around the world; thus the COVID-19 pandemic disaster lockdown strategies offer an important message to all countries of the world to restore environmental quality and the stability of the natural ecosystem (Bhat et al., 2021a). In addition to the primary sources of contamination, such as the antibiotics studied in the present review, the use of different products in the pandemic, such as the use of disinfectants, requires an immediate evaluation of the environmental effect in order to reduce the adverse effects on people and the environment, whether in water, soil or air (Bhat et al., 2021b). Table 4 below shows the leading antibiotic treatment technologies studied in this review article.
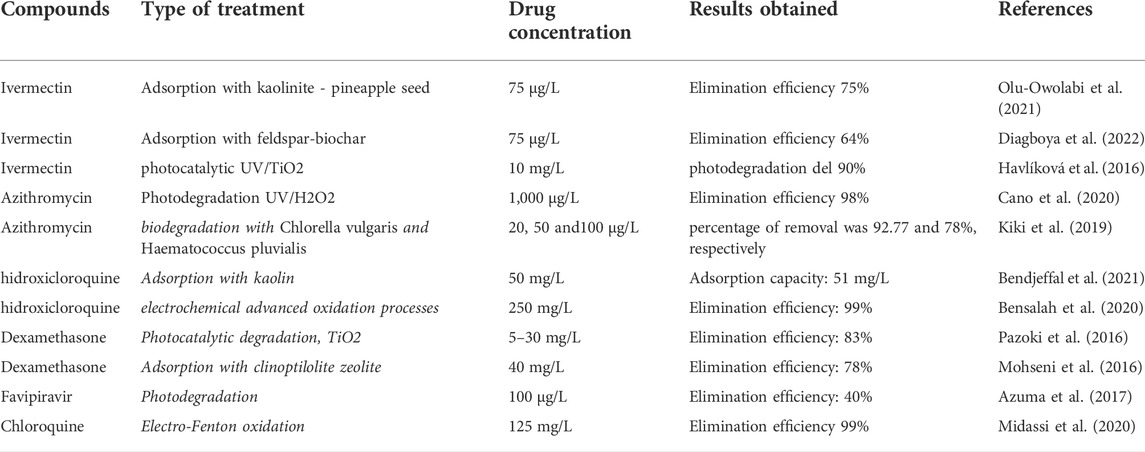
TABLE 4. Reported treatment technologies for the elimination of pharmaceutical compounds used in COVID-19 therapies.
8 Conclusion
According to the review of articles on the detection, presence and environmental implications of the primary drugs tested for the treatment of COVID-19, data have been analyzed showing that these pharmaceutical products are present in different environmental matrices, especially in surface water, sediments, wastewater treatment plants and domestic water, which has generated an incipient concern about the environmental impact. However, it has been seen that there is little information on the subject, mainly regarding antiviral residues in the soil and ecotoxicity of fauna, which makes it a challenge to know the magnitude of the risk faced by living beings in general. Given the increase in the consumption of these pharmaceutical products by COVID-19, it is innovative to propose technological alternatives that allow the elimination of these contaminants in an efficient and economically viable manner. Combined processes seem to be the best solution for treating effluent-containing antibiotics, especially those using renewable energies and derived materials, such as adsorption, advanced oxidation processes, photodegradation, photo-Fenton, UV photodegradation, algae treatment and photocatalysis.
Author contributions
DM, writing–original draft. IM, revised, corrected, edited, and validate the manuscript. FV, conceptualization, supervision, project administration, review, editing, and validate the manuscript.
Funding
To the National University of Moquegua for funding this project (Resolución de Comisión Organizadora N° 0310–2020 -UNAM).
Acknowledgments
To Huijing Zhong from the Universidad Científica del Sur, for the English editing.
Conflict of interest
The authors declare that the research was conducted in the absence of any commercial or financial relationships that could be construed as a potential conflict of interest.
Publisher’s note
All claims expressed in this article are solely those of the authors and do not necessarily represent those of their affiliated organizations, or those of the publisher, the editors and the reviewers. Any product that may be evaluated in this article, or claim that may be made by its manufacturer, is not guaranteed or endorsed by the publisher.
References
Abafe, O. A., Späth, J., Fick, J., Jansson, S., Buckley, C., Stark, A., et al. (2018). LC-MS/MS determination of antiretroviral drugs in influents and effluents from wastewater treatment plants in KwaZulu-Natal, South Africa. Chemosphere 200, 660–670. doi:10.1016/j.chemosphere.2018.02.105
Abd-Elsalam, S., Noor, R. A., Badawi, R., Khalaf, M., Esmail, E. S., Soliman, S., et al. (2021). Clinical study evaluating the efficacy of ivermectin in COVID-19 treatment: a randomized controlled study. J. Med. Virol. 93, 5833. doi:10.1002/jmv.27122
Ahn, S. J., Ryu, J., Lim, H. W., and Lee, B. R. (2019). Toxic effects of hydroxychloroquine on the choroid. Retina 39 (5), 1016–1026. doi:10.1097/iae.0000000000002047
Al Aukidy, M., Verlicchi, P., Jelic, A., Petrovic, M., and Barcelò, D. (2012). Monitoring release of pharmaceutical compounds: Occurrence and environmental risk assessment of two WWTP effluents and their receiving bodies in the Po Valley, Italy. Sci. Total Environ. 438, 15–25. doi:10.1016/j.scitotenv.2012.08.061
Alves da Silva, A. E., de Abreu, P. M. B., Geraldes, D. C., and de Oliveira Nascimento, L. (2021). Hydroxychloroquine: pharmacological, physicochemical aspects and activity enhancement through experimental formulations. J. Drug Deliv. Sci. Technol. 63, 102512. doi:10.1016/j.jddst.2021.102512
Azuma, T., Ishida, M., Hisamatsu, K., Yunoki, A., Otomo, K., Kunitou, M., et al. (2017). Fate of new three anti-influenza drugs and one prodrug in the water environment. Chemosphere 169, 550–557. doi:10.1016/j.chemosphere.2016.11.102
Bai, S. H., and Ogbourne, S. M. (2016). Eco-toxicological effects of the avermectin family with a focus on abamectin and ivermectin. Chemosphere 154, 204–214. doi:10.1016/j.chemosphere.2016.03.113
Barrows, N. J., Campos, R. K., Powell, S. T., Prasanth, K. R., Schott-Lerner, G., Soto-Acosta, R., et al. (2016). A screen of FDA-approved drugs for inhibitors of Zika virus infection. Cell Host Microbe 20 (2), 259–270. doi:10.1016/j.chom.2016.07.004
Becker, D. E. (2013). Basic and clinical pharmacology of Glucocorticosteroids. Anesth. Prog. 60 (1), 25–32. doi:10.2344/0003-3006-60.1.25
Beigel, J. H., Tomashek, K. M., Dodd, L. E., Mehta, A. K., Zingman, B. S., Kalil, A. C., et al. (2020). Remdesivir for the treatment of covid-19 — final report. N. Engl. J. Med. Overseas. Ed. 383 (19), 1813–1826. doi:10.1056/nejmoa2007764
Ben Ali, M., Hedfi, A., Almalki, M., Karachle, P. K., and Boufahja, F. (2021). Toxicity of hydroxychloroquine, a potential treatment for COVID-19, on free-living marine nematodes. Mar. Pollut. Bull. 167, 112361. doi:10.1016/j.marpolbul.2021.112361
Bendjeffal, H., Ziati, M., Aloui, A., Mamine, H., Metidji, T., Djebli, A., et al. (2021). Adsorption and removal of hydroxychloroquine from aqueous media using Algerian kaolin: full factorial optimisation, kinetic, thermodynamic, and equilibrium studies. Int. J. Environ. Anal. Chem., 1–22. doi:10.1080/03067319.2021.1887162
Bensalah, N., Midassi, S., Ahmad, M. I., and Bedoui, A. (2020). Degradation of hydroxychloroquine by electrochemical advanced oxidation processes. Chem. Eng. J. 402, 126279. doi:10.1016/j.cej.2020.126279
Bhat, S. A., Bashir, O., Bilal, M., Ishaq, A., Din Dar, M. U., Kumar, R., et al. (2021a). Impact of COVID-related lockdowns on environmental and climate change scenarios. Environ. Res. 195, 110839. doi:10.1016/j.envres.2021.110839
Bhat, S. A., Sher, F., Kumar, R., Karahmet, E., Haq, S. A. U., Zafar, A., et al. (2021b). Environmental and health impacts of spraying COVID-19 disinfectants with associated challenges. Environ. Sci. Pollut. Res. Int, 1–10. doi:10.1007/s11356-021-16575-7
Biswas, P., Vellanki, B. P., and Kazmi, A. A. (2022). Investigating a broad range of emerging contaminants in a set of anthropogenically impacted environmental compartments. Sci. Total Environ. 824, 153757. doi:10.1016/j.scitotenv.2022.153757
Bloom, R. A., and Matheson, J. C. (1993). Environmental assessment of avermectins by the US food and drug administration. Veterinary Parasitol. 48 (1–4), 281–294. doi:10.1016/0304-4017(93)90163-h
Brinke, M., Höss, S., Fink, G., Ternes, T. A., Heininger, P., Traunspurger, W., et al. (2010). Assessing effects of the pharmaceutical ivermectin on meiobenthic communities using freshwater microcosms. Aquat. Toxicol. 99 (2), 126–137. doi:10.1016/j.aquatox.2010.04.008
Brinke, M., Heininger, P., and Traunspurger, W. (2011). A semi-fluid gellan gum medium improves nematode toxicity testing. Ecotoxicol. Environ. Saf. 74 (7), 1824–1831. doi:10.1016/j.ecoenv.2011.07.007
Bundschuh, M., Hahn, T., Ehrlich, B., Höltge, S., Kreuzig, R., Schulz, R., et al. (2016). Acute toxicity and environmental risks of five veterinary pharmaceuticals for aquatic macroinvertebrates. Bull. Environ. Contam. Toxicol. 96 (2), 139–143. doi:10.1007/s00128-015-1656-8
Buta, M., Hubeny, J., Zieliński, W., Harnisz, M., and Korzeniewska, E. (2021). Sewage sludge in agriculture – the effects of selected chemical pollutants and emerging genetic resistance determinants on the quality of soil and crops – a review. Ecotoxicol. Environ. Saf. 214, 112070. doi:10.1016/j.ecoenv.2021.112070
Cain, D. W., and Cidlowski, J. A. (2015). Specificity and sensitivity of glucocorticoid signaling in health and disease. Best Pract. Res. Clin. Endocrinol. Metabolism 29 (4), 545–556. doi:10.1016/j.beem.2015.04.007
Cano, P. A., Jaramillo-Baquero, M., Zúñiga-Benítez, H., Londoño, Y. A., and Peñuela, G. A. (2020). Use of simulated sunlight radiation and hydrogen peroxide in azithromycin removal from aqueous solutions: Optimization & mineralization analysis. Emerg. Contam. 6, 53–61. doi:10.1016/j.emcon.2019.12.004
Carpagnano, G. E., Migliore, G., Grasso, S., Procacci, V., Resta, E., Panza, F., et al. (2021). More skilled clinical management of COVID-19 patients modified mortality in an intermediate respiratory intensive care unit in Italy. Respir. Res. 22 (1), 16. doi:10.1186/s12931-021-01613-2
Cavalcanti, A. B., Zampieri, F. G., Rosa, R. G., Azevedo, L. C. P., Veiga, V. C., Avezum, A., et al. (2020). Hydroxychloroquine with or without azithromycin in mild-to-moderate covid-19. N. Engl. J. Med. Overseas. Ed. 383 (21), 2041–2052. doi:10.1056/nejmoa2019014
Chang, H., Hu, J., and Shao, B. (2007). Occurrence of natural and synthetic glucocorticoids in sewage treatment plants and receiving river waters. Environ. Sci. Technol. 41 (10), 3462–3468. doi:10.1021/es062746o
Charuaud, L., Jardé, E., Jaffrézic, A., Liotaud, M., Goyat, Q., Mercier, F., et al. (2019). Veterinary pharmaceutical residues in water resources and tap water in an intensive husbandry area in France. Sci. Total Environ. 664, 605–615. doi:10.1016/j.scitotenv.2019.01.303
Chen, Q., Jia, A., Snyder, S. A., Gong, Z., and Lam, S. H. (2016). Glucocorticoid activity detected by in vivo zebrafish assay and in vitro glucocorticoid receptor bioassay at environmental relevant concentrations. Chemosphere 144, 1162–1169. doi:10.1016/j.chemosphere.2015.09.089
Chen, J., He, L. X., Cheng, Y. X., Ye, P., Wu, D. L., Fang, Z. Q., et al. (2020). Trace analysis of 28 antibiotics in plant tissues (root, stem, leaf and seed) by optimized QuEChERS pretreatment with UHPLC-MS/MS detection. J. Chromatogr. B Anal. Technol. Biomed. Life Sci. 1161, 21–23. doi:10.1016/j.jchromb.2020.122450
Chen, X., Lei, L., Liu, S., Han, J., Li, R., Men, J., et al. (2021). Occurrence and risk assessment of pharmaceuticals and personal care products (PPCPs) against COVID-19 in lakes and WWTP-river-estuary system in Wuhan, China. Sci. Total Environ. 792, 148352. doi:10.1016/j.scitotenv.2021.148352
Cheng, A. P., Cheng, M. P., Gu, W., Sesing Lenz, J., Hsu, E., Schurr, E., et al. (2021). Cell-free DNA tissues of origin by methylation profiling reveals significant cell, tissue, and organ-specific injury related to COVID-19 severity. Med 2 (4), 411–422.e5. doi:10.1016/j.medj.2021.01.001
Conforti, S., Dietrich, J., Kuhn, T., Koppenhagen, N. V., Baur, J., Rohner, P. T., et al. (2018). Comparative effects of the parasiticide ivermectin on survival and reproduction of adult sepsid flies. Ecotoxicol. Environ. Saf. 163, 215–222. doi:10.1016/j.ecoenv.2018.07.029
Czech, B., Krzyszczak, A., Boguszewska-Czubara, A., Opielak, G., Jośko, I., Hojamberdiev, M., et al. (2022). Revealing the toxicity of lopinavir- and ritonavir-containing water and wastewater treated by photo-induced processes to Danio rerio and Allivibrio fischeri. Sci. Total Environ. 824, 153967. doi:10.1016/j.scitotenv.2022.153967
da Luz, T. M., Araújo, A. P. da C., Estrela, F. N., Braz, H. L. B., Jorge, R. J. B., Charlie-Silva, I., et al. (2021). Can use of hydroxychloroquine and azithromycin as a treatment of COVID-19 affect aquatic wildlife? A study conducted with neotropical tadpole. Sci. Total Environ. 780, 146553. doi:10.1016/j.scitotenv.2021.146553
Danza, Á., Graña, D., Goñi, M., Vargas, A., and Ruiz-Irastorza, G. (2016). Hidroxicloroquina en el tratamiento de las enfermedades autoinmunes sistémicas. Rev. Med. Chile 144 (2), 232–240. doi:10.4067/s0034-98872016000200012
Daughton, C. G. (2014). Eco-directed sustainable prescribing: feasibility for reducing water contamination by drugs. Sci. Total Environ. 493, 392–404. doi:10.1016/j.scitotenv.2014.06.013
De La Torre, A., Iglesias, I., Carballo, M., Ramírez, P., and Muñoz, M. J. (2012). An approach for mapping the vulnerability of European Union soils to antibiotic contamination. Sci. Total Environ. 414, 672–679. doi:10.1016/j.scitotenv.2011.10.032
Derendorf, H. (2020). Excessive lysosomal ion-trapping of hydroxychloroquine and azithromycin. Int. J. Antimicrob. Agents 55 (6), 106007. doi:10.1016/j.ijantimicag.2020.106007
Desgens-Martin, V., and Keller, A. A. (2021). COVID-19 treatment agents: do they pose an environmental risk? ACS Est. Water 1 (7), 1555–1565. doi:10.1021/acsestwater.1c00059
Diagboya, P. N., Mtunzi, F. M., Adebowale, K. O., Düring, R. A., and Olu-Owolabi, B. I. (2022). Comparative empirical evaluation of the aqueous adsorptive sequestration potential of low-cost feldspar-biochar composites for ivermectin. Colloids Surfaces A Physicochem. Eng. Aspects 634, 127930. doi:10.1016/j.colsurfa.2021.127930
Díaz-Cruz, M. S., and Barceló, D. (2007). Chapter 2.1 Analysis of antibiotics in aqueous samples. Compr. Anal. Chem. 50 (07), 61–93. doi:10.1016/S0166-526X(07)50002-4
Domingo-Echaburu, S., Irazola, M., Prieto, A., Rocano, B., Lopez de Torre-Querejazu, A., Quintana, A., et al. (2022). Drugs used during the COVID-19 first wave in Vitoria-Gasteiz (Spain) and their presence in the environment. Sci. Total Environ. 820, 153122. doi:10.1016/j.scitotenv.2022.153122
Du, C., Zhang, Z., Yu, G., Wu, H., Chen, H., Zhou, L., et al. (2021). A review of metal organic framework (MOFs)-based materials for antibiotics removal via adsorption and photocatalysis. Chemosphere 272, 129501. doi:10.1016/j.chemosphere.2020.129501
Dunlap, K. D., Pelczar, P. L., and Knapp, R. (2002). Social interactions and cortisol treatment increase the production of aggressive electrocommunication signals in male electric fish, Apteronotus leptorhynchus. Hormones Behav. 42 (2), 97–108. doi:10.1006/hbeh.2002.1807
Dusi, G., Gasparini, M., Curatolo, M., Assini, W., Bozzoni, E., Tognoli, N., et al. (2011). Development and validation of a liquid chromatography–tandem mass spectrometry method for the simultaneous determination of nine corticosteroid residues in bovine liver samples. Anal. Chim. Acta 700 (1–2), 49–57. doi:10.1016/j.aca.2011.01.011
El-Din Abuo-Rahma, G. A., Mohamed, M. F. A., Ibrahim, T. S., Shoman, M. E., Samir, E., Abd El-Baky, R. M., et al. (2020). Potential repurposed SARS-CoV-2 (COVID-19) infection drugs. RSC Adv. 10 (45), 26895–26916. doi:10.1039/d0ra05821a
Emam, A. A., Abdelaleem, E. A., Abdelmomen, E. H., Abdelmoety, R. H., and AbdelFattah, R. M. (2022). Rapid and ecofriendly UPLC quantification of Remdesivir, Favipiravir and Dexamethasone for accurate therapeutic drug monitoring in Covid-19 Patient's plasma. Microchem. J. 179, 107580. doi:10.1016/j.microc.2022.107580
Essid, N., Allouche, M., Lazzem, M., Harrath, A. H., Mansour, L., Alwasel, S., et al. (2020). Ecotoxic response of nematodes to ivermectin, a potential anti-COVID-19 drug treatment. Mar. Pollut. Bull. 157, 111375. doi:10.1016/j.marpolbul.2020.111375
Ezzariai, A., Hafidi, M., Khadra, A., Aemig, Q., El Fels, L., Barret, M., et al. (2018). Human and veterinary antibiotics during composting of sludge or manure: global perspectives on persistence, degradation, and resistance genes. J. Hazard. Mater. 359, 465–481. doi:10.1016/j.jhazmat.2018.07.092
Fiolet, T., Guihur, A., Rebeaud, M. E., Mulot, M., Peiffer-Smadja, N., Mahamat-Saleh, Y., et al. (2021). Effect of hydroxychloroquine with or without azithromycin on the mortality of coronavirus disease 2019 (COVID-19) patients: a systematic review and meta-analysis. Clin. Microbiol. Infect. 27 (1), 19–27. doi:10.1016/j.cmi.2020.08.022
Fisher, M. H., and Mrozik, H. (1989). “Chemistry,” in Ivermectin and abamectin (New York, NY: Springer), 1–23.
Forestieri, S., Pintus, R., Marcialis, M. A., Pintus, M. C., and Fanos, V. (2021). COVID-19 and developmental origins of health and disease. Early Hum. Dev. 155, 105322. doi:10.1016/j.earlhumdev.2021.105322
Gao, X., Huang, K., Zhang, A., Wang, C., Sun, Z., Liu, Y., et al. (2022). Simultaneous degradation of glucocorticoids and sterilization using bubbling corona discharge plasma based systems: a promising terminal water treatment facility for hospital wastewater. Chem. Eng. J. 430, 132845. doi:10.1016/j.cej.2021.132845
Gautret, P., Lagier, J. C., Parola, P., Hoang, V. T., Meddeb, L., Mailhe, M., et al. (2020). Hydroxychloroquine and azithromycin as a treatment of COVID-19: results of an open-label non-randomized clinical trial. Int. J. Antimicrob. Agents 56 (1), 105949. doi:10.1016/j.ijantimicag.2020.105949
Gautret, P., Lagier, J. C., Honoré, S., Hoang, V. T., Colson, P., Raoult, D., et al. (2021). Hydroxychloroquine and azithromycin as a treatment of COVID-19: results of an open label non-randomized clinical trial revisited. Int. J. Antimicrob. Agents 57 (1), 106243. doi:10.1016/j.ijantimicag.2020.106243
Goessens, T., Baere, S. D., Troyer, N. D., Deknock, A., Goethals, P., Lens, L., et al. (2020). Highly sensitive multi-residue analysis of veterinary drugs including coccidiostats and anthelmintics in pond water using UHPLC-MS/MS: application to freshwater ponds in Flanders, Belgium. Environ. Sci. Process. Impacts 22 (10), 2117–2131. doi:10.1039/d0em00215a
Golovko, O., Kumar, V., Fedorova, G., Randak, T., and Grabic, R. (2014). Seasonal changes in antibiotics, antidepressants/psychiatric drugs, antihistamines and lipid regulators in a wastewater treatment plant. Chemosphere 111, 418–426. doi:10.1016/j.chemosphere.2014.03.132
Gong, J., Lin, C., Xiong, X., Chen, D., Chen, Y., Zhou, Y., et al. (2019). Occurrence, distribution, and potential risks of environmental corticosteroids in surface waters from the Pearl River Delta, South China. Environ. Pollut. 251, 102–109. doi:10.1016/j.envpol.2019.04.110
González-Pleiter, M., Pedrouzo-Rodríguez, A., Verdú, I., Leganés, F., Marco, E., Rosal, R., et al. (2021). Microplastics as vectors of the antibiotics azithromycin and clarithromycin: Effects towards freshwater microalgae. Chemosphere 268, 128824. doi:10.1016/j.chemosphere.2020.128824
González-Tokman, D., Martínez, M., I., Villalobos-Ávalos, Y., Munguía-Steyer, R., Ortiz-Zayas, M., del, R., et al. (2017). Ivermectin alters reproductive success, body condition and sexual trait expression in dung beetles. Chemosphere 178, 129–135. doi:10.1016/j.chemosphere.2017.03.013
Gonzalez-Zorn, B. (2021). Antibiotic use in the COVID-19 crisis in Spain. Clin. Microbiol. Infect. 27 (4), 646–647. doi:10.1016/j.cmi.2020.09.055
Gottschall, N., Topp, E., Metcalfe, C., Edwards, M., Payne, M., Kleywegt, S., et al. (2012). Pharmaceutical and personal care products in groundwater , subsurface drainage , soil , and wheat grain , following a high single application of municipal biosolids to a field. Chemosphere 87, 194–203. doi:10.1016/j.chemosphere.2011.12.018
Gravesen, C., and Judy, J. D. (2020). Effect of biosolids characteristics on retention and release behavior of azithromycin and ciprofloxacin. Environ. Res. 184, 109333. doi:10.1016/j.envres.2020.109333
Guimarães, B., Maria, V. L., Römbke, J., and Amorim, M. J. B. (2019). Multigenerational exposure of Folsomia candida to ivermectin – using avoidance, survival, reproduction, size and cellular markers as endpoints. Geoderma 337, 273–279. doi:10.1016/j.geoderma.2018.09.030
Guo, Q., Du, Z., and Shao, B. (2018). Simulation and experimental study on the mechanism of the chlorination of azithromycin. J. Hazard. Mater. 359, 31–39. doi:10.1016/j.jhazmat.2018.07.024
Gupta, D., Sahoo, A. K., and Singh, A. (2020). Ivermectin: potential candidate for the treatment of covid 19. Braz. J. Infect. Dis. 24 (4), 369–371. doi:10.1016/j.bjid.2020.06.002
Gyapong, J. O., Chinbuah, M. A., and Gyapong, M. (2003). Inadvertent exposure of pregnant women to ivermectin and albendazole during mass drug administration for lymphatic filariasis. Trop. Med. Int. Health 8 (12), 1093–1101. doi:10.1046/j.1360-2276.2003.01142.x
Harada, A., Komori, K., Nakada, N., Kitamura, K., and Suzuki, Y. (2008). Biological effects of PPCPs on aquatic lives and evaluation of river waters affected by different wastewater treatment levels. Water Sci. Technol. 58 (8), 1541–1546. doi:10.2166/wst.2008.742
Havlíková, L., Šatínský, D., and Solich, P. (2016). Aspects of decontamination of ivermectin and praziquantel from environmental waters using advanced oxidation technology. Chemosphere 144, 21–28. doi:10.1016/j.chemosphere.2015.08.039
Herrero, P., Borrull, F., Pocurull, E., and Marcé, R. M. (2012). Determination of glucocorticoids in sewage and river waters by ultra-high performance liquid chromatography–tandem mass spectrometry. J. Chromatogr. A 1224, 19–26. doi:10.1016/j.chroma.2011.12.054
Homem, V., and Santos, L. (2011). Degradation and removal methods of antibiotics from aqueous matrices – a review. J. Environ. Manag. 92 (10), 2304–2347. doi:10.1016/j.jenvman.2011.05.023
Howard, B. (2007). “Ivermectin,” in xPharm: The comprehensive pharmacology reference (Huntington, USA: Elsevier), 1–5.
Isobe, T., Sato, K., Joon-Woo, K., Tanabe, S., Suzuki, G., Nakayama, K., et al. (2015). Determination of natural and synthetic glucocorticoids in effluent of sewage treatment plants using ultrahigh performance liquid chromatography-tandem mass spectrometry. Environ. Sci. Pollut. Res. 22 (18), 14127–14135. doi:10.1007/s11356-015-4626-0
Jafari Ozumchelouei, E., Hamidian, A. H., Zhang, Y., and Yang, M. (2020). Physicochemical properties of antibiotics: a review with an emphasis on detection in the aquatic environment. Water Environ. Res. 92 (2), 177–188. doi:10.1002/wer.1237
Jans, D. A., and Wagstaff, K. M. (2021). The broad spectrum host-directed agent ivermectin as an antiviral for SARS-CoV-2. Biochem. Biophysical Res. Commun. 538, 163–172. doi:10.1016/j.bbrc.2020.10.042
Jensen, J., and Scott-Fordsmand, J. J. (2012). Ecotoxicity of the veterinary pharmaceutical ivermectin tested in a soil multi-species (SMS) system. Environ. Pollut. 171, 133–139. doi:10.1016/j.envpol.2012.07.014
Johnson, R. M., and Vinetz, J. M. (2020). Dexamethasone in the management of Covid -19. BMJ 370, m2648. doi:10.1136/bmj.m2648
Kar, S., Sanderson, H., Roy, K., Benfenati, E., and Leszczynski, J. (2020). Ecotoxicological assessment of pharmaceuticals and personal care products using predictive toxicology approaches. Green Chem. 22, 1458–1516. doi:10.1039/c9gc03265g
Khan, K. Y., Ali, B., Zhang, S., Stoffella, P. J., Yuan, S., Xia, Q., et al. (2021). Effects of antibiotics stress on growth variables, ultrastructure, and metabolite pattern of Brassica rapa ssp. chinensis. Sci. Total Environ. 778, 146333. doi:10.1016/j.scitotenv.2021.146333
Kiki, C., Rashid, A., Wang, Y., Li, Y., Zeng, Q., Yu, C., et al. (2019). Dissipation of antibiotics by microalgae: kinetics, identification of transformation products and pathways. J. Hazard. Mater. 387, 121985. doi:10.1016/j.jhazmat.2019.121985
Koch, D. E., Bhandari, A., Close, L., and Hunter, R. P. (2005). Azithromycin extraction from municipal wastewater and quantitation using liquid chromatography/mass spectrometry. J. Chromatogr. A 1074 (1–2), 17–22. doi:10.1016/j.chroma.2005.03.052
Krogh, K. A., Søeborg, T., Brodin, B., and Halling-Sørensen, B. (2008). Sorption and mobility of ivermectin in different soils. J. Environ. Qual. 37 (6), 2202–2211. doi:10.2134/jeq2007.0592
Krumm, Z. A., Lloyd, G. M., Francis, C. P., Nasif, L. H., Mitchell, D. A., Golde, T. E., et al. (2021). Precision therapeutic targets for COVID-19. Virol. J. 18 (1), 66. doi:10.1186/s12985-021-01526-y
Kumari, M., and Kumar, A. (2021). Can pharmaceutical drugs used to treat covid-19 infection leads to human health risk? a hypothetical study to identify potential risk. Sci. Total Environ. 778, 146303. doi:10.1016/j.scitotenv.2021.146303
Kuroda, K., Li, C., Dhangar, K., and Kumar, M. (2021). Predicted occurrence, ecotoxicological risk and environmentally acquired resistance of antiviral drugs associated with COVID-19 in environmental waters. Sci. Total Environ. 776, 145740. doi:10.1016/j.scitotenv.2021.145740
Lagier, J. C., Million, M., Gautret, P., Colson, P., Cortaredona, S., Giraud-Gatineau, A., et al. (2020). Outcomes of 3, 737 COVID-19 patients treated with hydroxychloroquine/azithromycin and other regimens in marseille, France: a retrospective analysis. Travel Med. Infect. Dis. 36, 101791. doi:10.1016/j.tmaid.2020.101791
Lalone, C. A., Villeneuve, D. L., Olmstead, A. W., Medlock, E. K., Kahl, M. D., Jensen, K. M., et al. (2012). Effects of a glucocorticoid receptor agonist, dexamethasone, on fathead minnow reproduction, growth, and development. Environ. Toxicol. Chem. 31 (3), 611–622. doi:10.1002/etc.1729
Li, H., Liu, D. H., Chen, L. L., Zhao, Q., Yu, Y. Z., Ding, J. J., et al. (2014). Meta-Analysis of the adverse effects of long-term azithromycin use in patients with chronic lung diseases. Antimicrob. Agents Chemother. 58 (1), 511–517. doi:10.1128/aac.02067-13
Li, Y., Ma, Y., Yang, L., Duan, S., Zhou, F., Chen, J., et al. (2020). Effects of azithromycin on feeding behavior and nutrition accumulation of Daphnia magna under the different exposure pathways. Ecotoxicol. Environ. Saf. 197, 110573. doi:10.1016/j.ecoenv.2020.110573
Li, S., Loke, P., Hao, H., and Ho, S. (2022). Algae-mediated antibiotic wastewater treatment: A critical review. Environ. Sci. Ecotechnology 9, 100145. doi:10.1016/j.ese.2022.100145
Liu, S., Chen, H., Xu, X. R., Liu, S. S., Sun, K. F., Zhao, J. L., et al. (2015). Steroids in marine aquaculture farms surrounding Hailing Island, South China: Occurrence, bioconcentration, and human dietary exposure. Sci. Total Environ. 502, 400–407. doi:10.1016/j.scitotenv.2014.09.039
Liu, L., Liu, Y., Liu, C., and Huang, X. (2016). Accumulation of antibiotics and tet resistance genes from swine wastewater in wetland soils. Environ. Eng. Manag. J. 15, 2137–2145. doi:10.30638/eemj.2016.231
Lv, C., Liu, W., Wang, B., Dang, R., Qiu, L., Ren, J., et al. (2018). Ivermectin inhibits DNA polymerase UL42 of pseudorabies virus entrance into the nucleus and proliferation of the virus in vitro and vivo. Antivir. Res. 159, 55–62. doi:10.1016/j.antiviral.2018.09.010
Macikova, P., Groh, K. J., Ammann, A. A., Schirmer, K., and Suter, M. J. F. (2014). Endocrine disrupting compounds affecting corticosteroid signaling pathways in Czech and Swiss Waters: Potential impact on fish. Environ. Sci. Technol. 48 (21), 12902–12911. doi:10.1021/es502711c
Madelain, V., Mentré, F., Baize, S., Anglaret, X., Laouénan, C., Oestereich, L., et al. (2020). Modeling favipiravir antiviral efficacy against emerging viruses: From animal studies to clinical trials. CPT. Pharmacometrics Syst. Pharmacol. 9 (5), 258–271. doi:10.1002/psp4.12510
Mao, Y., Yu, Y., Ma, Z., Li, H., Yu, W., Cao, L., et al. (2021). Azithromycin induces dual effects on microalgae: Roles of photosynthetic damage and oxidative stress. Ecotoxicol. Environ. Saf. 222, 112496. doi:10.1016/j.ecoenv.2021.112496
Martinez, J. L. (2009). Environmental pollution by antibiotics and by antibiotic resistance determinants. Environ. Pollut. 157 (11), 2893–2902. doi:10.1016/j.envpol.2009.05.051
Mendonça-Gomes, J. M., da Costa Araújo, A. P., da Luz, T. M., Charlie-Silva, I., Braz, H. L. B., Jorge, R. J. B., et al. (2021). Environmental impacts of COVID-19 treatment: Toxicological evaluation of azithromycin and hydroxychloroquine in adult zebrafish. Sci. Total Environ. 790, 148129. doi:10.1016/j.scitotenv.2021.148129
Mesa, L. M., Lindt, I., Negro, L., Gutierrez, M. F., Mayora, G., Montalto, L., et al. (2017). Aquatic toxicity of ivermectin in cattle dung assessed using microcosms. Ecotoxicol. Environ. Saf. 144, 422–429. doi:10.1016/j.ecoenv.2017.06.016
Mhadhbi, L., El Ayari, T., Tir, M., and Kadri, D. (2020). Azithromycin effects on the European sea bass (Dicentrarchus labrax) early life stages following acute and chronic exposure: Laboratory bioassays. Drug Chem. Toxicol. 45, 1295–1301. doi:10.1080/01480545.2020.1822388
Midassi, S., Bedoui, A., and Bensalah, N. (2020). Efficient degradation of chloroquine drug by electro-Fenton oxidation: Effects of operating conditions and degradation mechanism. Chemosphere 260, 127558. doi:10.1016/j.chemosphere.2020.127558
Minguez, L., Pedelucq, J., Farcy, E., Ballandonne, C., Budzinski, H., Halm-Lemeille, M. P., et al. (2016). Toxicities of 48 pharmaceuticals and their freshwater and marine environmental assessment in northwestern France. Environ. Sci. Pollut. Res. 23 (6), 4992–5001. doi:10.1007/s11356-014-3662-5
Mititelu, M., Wong, B. J., Brenner, M., Bryar, P. J., Jampol, L. M., Fawzi, A. A., et al. (2013). Progression of hydroxychloroquine toxic effects after drug therapy cessation: New evidence from multimodal imaging. JAMA Ophthalmol. 131 (9), 1187. doi:10.1001/jamaophthalmol.2013.4244
Mohseni, S. N., Amooey, A. A., Tashakkorian, H., and Amouei, A. I. (2016). Removal of dexamethasone from aqueous solutions using modified clinoptilolite zeolite (equilibrium and kinetic). Int. J. Environ. Sci. Technol. (Tehran). 13 (9), 2261–2268. doi:10.1007/s13762-016-1045-9
Nag, R., O'Rourke, S. M., and Cummins, E. (2022). Risk factors and assessment strategies for the evaluation of human or environmental risk from metal(loid)s – a focus on Ireland. Sci. Total Environ. 802, 149839. doi:10.1016/j.scitotenv.2021.149839
Nicolas, P., Maia, M. F., Bassat, Q., Kobylinski, K. C., Monteiro, W., Rabinovich, N. R., et al. (2020). Safety of oral ivermectin during pregnancy: a systematic review and meta-analysis. Lancet Glob. Health 8 (1), e92–e100. doi:10.1016/s2214-109x(19)30453-x
Numpilai, T., Witoon, T., Chareonpanich, M., and Limtrakul, J. (2017). Impact of physicochemical properties of porous silica materials conjugated with dexamethasone via pH-responsive hydrazone bond on drug loading and release behavior. Appl. Surf. Sci. 396, 504–514. doi:10.1016/j.apsusc.2016.10.183
Olatunde, J. O., Chimezie, A., Tolulope, B., and Aminat, T. T. (2014). Determination of pharmaceutical compounds in surface and underground water by solid phase extraction-liquid chromatography. J. Environ. Chem. Ecotoxicol. 6 (3), 20–26. doi:10.5897/jece2013.0312
Oliver, A. L. S., Muñoz-Olivas, R., Sanz Landaluze, J., Rainieri, S., and Cámara, C. (2015). Bioaccumulation of ionic titanium and titanium dioxide nanoparticles in zebrafish eleutheroembryos. Nanotoxicology 9 (7), 835–842. doi:10.3109/17435390.2014.980758
Olu-Owolabi, B. I., Diagboya, P. N., Mtunzi, F. M., and Düring, R. A. (2021). Utilizing eco-friendly kaolinite-biochar composite adsorbent for removal of ivermectin in aqueous media. J. Environ. Manag. 279, 111619. doi:10.1016/j.jenvman.2020.111619
Omotola, E. O., and Olatunji, O. S. (2020). Quantification of selected pharmaceutical compounds in water using liquid chromatography-electrospray ionisation mass spectrometry (LC-ESI-MS). Heliyon 6 (12), e05787. doi:10.1016/j.heliyon.2020.e05787
Parnham, M. J., Haber, V. E., Giamarellos-Bourboulis, E. J., Perletti, G., Verleden, G. M., Vos, R., et al. (2014). Azithromycin: Mechanisms of action and their relevance for clinical applications. Pharmacol. Ther. 143 (2), 225–245. doi:10.1016/j.pharmthera.2014.03.003
Pazoki, M., Parsa, M., and Farhadpour, R. (2016). Removal of the hormones dexamethasone (DXM) by Ag doped on TiO2 photocatalysis. J. Environ. Chem. Eng. 4 (4), 4426–4434. doi:10.1016/j.jece.2016.09.034
Pereira, A. M. P. T., Silva, L. J. G., Laranjeiro, C. S. M., Meisel, L. M., Lino, C. M., Pena, A., et al. (2017). Human pharmaceuticals in Portuguese rivers: the impact of water scarcity in the environmental risk. Sci. Total Environ. 609, 1182–1191. doi:10.1016/j.scitotenv.2017.07.200
Perrone, M., Laquintana, V., Lopedota, A. A., Cutrignelli, A., Lopalco, A., Franco, M., et al. (2020). Stability data of extemporaneous suspensions of hydroxychloroquine sulphate in oral liquid bases after tablet manipulation. Data Brief 33, 106575. doi:10.1016/j.dib.2020.106575
Platto, S., Wang, Y., Zhou, J., and Carafoli, E. (2021). History of the COVID-19 pandemic : Origin , explosion , worldwide spreading. Biochem. Biophys. Res. Commun. 538, 14–23. doi:10.1016/j.bbrc.2020.10.087
Prasse, C., Schlüsener, M. P., Schulz, R., and Ternes, T. A. (2010). Antiviral drugs in wastewater and surface waters: a new pharmaceutical class of environmental relevance? Environ. Sci. Technol. 44 (5), 1728–1735. doi:10.1021/es903216p
Praveena, S. M., Shaifuddin, S. N. M., Sukiman, S., Nasir, F. A. M., Hanafi, Z., Kamarudin, N., et al. (2018). Pharmaceuticals residues in selected tropical surface water bodies from Selangor (Malaysia): occurrence and potential risk assessments. Sci. Total Environ. 642, 230–240. doi:10.1016/j.scitotenv.2018.06.058
Quaresma, A. V., Rubio, K. T. S., Taylor, J. G., Sousa, B. A., Silva, S. Q., Werle, A. A., et al. (2021). Removal of dexamethasone by oxidative processes: structural characterization of degradation products and estimation of the toxicity. J. Environ. Chem. Eng. 9 (6), 106884. doi:10.1016/j.jece.2021.106884
Race, M., Ferraro, A., Galdiero, E., Guida, M., Núñez-Delgado, A., Pirozzi, F., et al. (2020). Current emerging SARS-CoV-2 pandemic: Potential direct/indirect negative impacts of virus persistence and related therapeutic drugs on the aquatic compartments. Environ. Res. 188, 109808. doi:10.1016/j.envres.2020.109808
Rang, H. P., Dale, M. M., Ritter, J. M., Flower, R. J., and Henderson, G. (2012). Rang & dale's pharmacology. Edimburgo: Elsevier Saunders.
Rasheed, T., Rizwan, K., Bilal, M., Sher, F., and Iqbal, H. M. N. (2021). Tailored functional materials as robust candidates to mitigate pesticides in aqueous matrices-a review. Chemosphere 282, 131056. doi:10.1016/j.chemosphere.2021.131056
Rasheed, T., Kausar, F., Rizwan, K., Adeel, M., Sher, F., Alwadai, N., et al. (2022). Two dimensional MXenes as emerging paradigm for adsorptive removal of toxic metallic pollutants from wastewater. Chemosphere 287, 132319. doi:10.1016/j.chemosphere.2021.132319
Reis, E. O., Foureaux, A. F. S., Rodrigues, J. S., Moreira, V. R., Lebron, Y. A. R., Santos, L. V. S., et al. (2019). Occurrence, removal and seasonal variation of pharmaceuticals in Brasilian drinking water treatment plants. Environ. Pollut. 250, 773–781. doi:10.1016/j.envpol.2019.04.102
Rodriguez-Mozaz, S., Vaz-Moreira, I., Varela Della Giustina, S., Llorca, M., Barceló, D., Schubert, S., et al. (2020). Antibiotic residues in final effluents of European wastewater treatment plants and their impact on the aquatic environment. Environ. Int. 140, 105733. doi:10.1016/j.envint.2020.105733
Rolim, L. A., dos Santos, F. C. M., Chaves, L., Goncalves, M., Freitas-Neto, J., do Nascimento, A., et al. (2014). Preformulation study of ivermectin raw material. J. Therm. Anal. Calorim. 120, 807–816. doi:10.1007/s10973-014-3691-9
Römbke, J., Krogh, K. A., Moser, T., Scheffczyk, A., and Liebig, M. (2010). Effects of the veterinary pharmaceutical ivermectin on soil invertebrates in laboratory tests. Arch. Environ. Contam. Toxicol. 58 (2), 332–340. doi:10.1007/s00244-009-9414-8
Roncalli, R. A. (1989). “Environmental aspects of use of ivermectin and abamectin in livestock: Effects on cattle dung fauna,” in Ivermectin and abamectin (New York, NY: Springer), 173–181.
Rosendaal, F. R., Lagier, J. C., Parola, P., Hoang, V. T., Meddeb, L., Mailhe, M., et al. (2020). Hydroxychloroquine and azithromycin as a treatment of COVID-19: results of an open-label non-randomized clinical trial. Int. J. Antimicrob. Agents 56 (1), 105949. doi:10.1016/j.ijantimicag.2020.105949
Senta, I., Krizman-Matasic, I., Terzic, S., and Ahel, M. (2017). Comprehensive determination of macrolide antibiotics, their synthesis intermediates and transformation products in wastewater effluents and ambient waters by liquid chromatography–tandem mass spectrometry. J. Chromatogr. A 1509, 60–68. doi:10.1016/j.chroma.2017.06.005
Shiogiri, S., Ven, C., Carraschi, S. P., da Cruz, C., and Fernandes, M. N. (2016). Effects of azithromycin on tilapia (Oreochromis niloticus): health status evaluation using biochemical, physiological and morphological biomarkers. Aquac. Res. 48, 3669–3683. doi:10.1111/are.13191
Sidhu, H., O'Connor, G., Ogram, A., and Kumar, K. (2019). Bioavailability of biosolids-borne ciprofloxacin and azithromycin to terrestrial organisms: Microbial toxicity and earthworm responses. Sci. Total Environ. 650, 18–26. doi:10.1016/j.scitotenv.2018.09.004
Sun, Y., Guo, Y., Shi, M., Qiu, T., Gao, M., Tian, S., et al. (2021). Effect of antibiotic type and vegetable species on antibiotic accumulation in soil-vegetable system, soil microbiota, and resistance genes. Chemosphere 263, 128099. doi:10.1016/j.chemosphere.2020.128099
Syslová, E., Landa, P., Navrátilová, M., Stuchlíková, L. R., Matoušková, P., Skálová, L., et al. (2019). Ivermectin biotransformation and impact on transcriptome in Arabidopsis thaliana. Chemosphere 234, 528–535. doi:10.1016/j.chemosphere.2019.06.102
Tarazona, J. V., Martínez, M., Martínez, M. A., and Anadón, A. (2021). Environmental impact assessment of COVID-19 therapeutic solutions. a prospective analysis. Sci. Total Environ. 778, 146257. doi:10.1016/j.scitotenv.2021.146257
Van Der Heijde, D. M., Van Riel, P. L., Gribnau, F. W., Nuver-Zwart, I. H., and Van De Putte, L. B. (1989). Effects of hydroxychloroquine and sulphasalazine on progression of joint damage in rheumatoid arthritis. Lancet 333, 1036–1038. doi:10.1016/s0140-6736(89)92442-2
Verdú, J. R., Lobo, J. M., Sánchez-Piñero, F., Gallego, B., Numa, C., Lumaret, J. P., et al. (2018). Ivermectin residues disrupt dung beetle diversity, soil properties and ecosystem functioning: An interdisciplinary field study. Sci. Total Environ. 618, 219–228. doi:10.1016/j.scitotenv.2017.10.331
Verdú, J. R., Cortez, V., Ortiz, A. J., Lumaret, J. P., Lobo, J. M., Sánchez-Piñero, F., et al. (2020). Biomagnification and body distribution of ivermectin in dung beetles. Sci. Rep. 10 (1), 9073. doi:10.1038/s41598-020-66063-0
Verlicchi, P., Al Aukidy, M., Jelic, A., Petrović, M., and Barceló, D. (2014). Comparison of measured and predicted concentrations of selected pharmaceuticals in wastewater and surface water: a case study of a catchment area in the Po valley (Italy). Sci. Total Environ. 470–471, 844–854. doi:10.1016/j.scitotenv.2013.10.026
Vilca, F. Z., Galarza, N. C., Tejedo, J. R., Cuba, W. A. Z., Quiróz, C. N. C., Tornisielo, V. L., et al. (2021). Occurrence of residues of veterinary antibiotics in water, sediment and trout tissue (Oncorhynchus mykiss) in the southern area of Lake Titicaca, Peru. J. Gt. Lakes. Res. 47 (4), 1219–1227. doi:10.1016/j.jglr.2021.04.012
Villada-Bedoya, S., Chávez-Ríos, J. R., Montoya, B., Castelán, F., Córdoba-Aguilar, A., Escobar, F., et al. (2021). Heat shock proteins and antioxidants as mechanisms of response to ivermectin in the dung beetle Euoniticellus intermedius. Chemosphere 269, 128707. doi:10.1016/j.chemosphere.2020.128707
Vokřál, I., Michaela, Š., Radka, P., Jiří, L., Lukáš, P., Dominika, S., et al. (2019). Ivermectin environmental impact: Excretion profile in sheep and phytotoxic effect in Sinapis alba. Ecotoxicol. Environ. Saf. 169, 944–949. doi:10.1016/j.ecoenv.2018.11.097
Vouri, S. M., Thai, T. N., and Winterstein, A. G. (2021). An evaluation of co-use of chloroquine or hydroxychloroquine plus azithromycin on cardiac outcomes: a pharmacoepidemiological study to inform use during the COVID19 pandemic. Res. Soc. Adm. Pharm. 17 (1), 2012–2017. doi:10.1016/j.sapharm.2020.04.031
Wang, X., Yin, R., Zeng, L., and Zhu, M. (2019). A review of graphene-based nanomaterials for removal of antibiotics from aqueous environments. Environ. Pollut. 253, 100–110. doi:10.1016/j.envpol.2019.06.067
Yang, C., Song, G., and Lim, W. (2020). A review of the toxicity in fish exposed to antibiotics. Comp. Biochem. Physiology Part C Toxicol. Pharmacol. 237, 108840. doi:10.1016/j.cbpc.2020.108840
Yi, X., Lin, C., Ong, E. J. L., Wang, M., and Zhou, Z. (2019). Occurrence and distribution of trace levels of antibiotics in surface waters and soils driven by non-point source pollution and anthropogenic pressure. Chemosphere 216, 213–223. doi:10.1016/j.chemosphere.2018.10.087
Yusuf, I. H., Sharma, S., Luqmani, R., and Downes, S. M. (2017). Hydroxychloroquine retinopathy. Eye (Basingstoke) 31 (6), 828–845. doi:10.1038/eye.2016.298
Zaheer, T., Pal, K., Abbas, R. Z., Torres, M., and del, P. R. (2021). COVID-19 and Ivermectin: Potential threats associated with human use. J. Mol. Struct. 1243, 130808. doi:10.1016/j.molstruc.2021.130808
Zhang, P., Ni, H., Zhang, Y., Xu, W., Gao, J., Cheng, J., et al. (2020). Ivermectin confers its cytotoxic effects by inducing AMPK/mTOR-mediated autophagy and DNA damage. Chemosphere 259, 127448. doi:10.1016/j.chemosphere.2020.127448
Zhao, H., Shi, S., Zhao, H., Guo, J., Yang, Z., Gao, H., et al. (2019). Proteomic analysis of the earthworm: Eisenia fetida exposed to oxytetracycline in soil. RSC Adv. 9 (71), 41628–41638. doi:10.1039/c9ra06004a
Zhao, N., Liu, Y., Smargiassi, A., and Bernatsky, S. (2020). Tracking the origin of early COVID-19 cases in Canada. Int. J. Infect. Dis. 96, 506–508. doi:10.1016/j.ijid.2020.05.046
Zhi, D., Yang, D., Zheng, Y., Yang, Y., He, Y., Luo, L., et al. (2019). Current progress in the adsorption, transport and biodegradation of antibiotics in soil. J. Environ. Manag. 251, 109598. doi:10.1016/j.jenvman.2019.109598
Zhou, C., Chen, J., Xie, Q., Wei, X., Zhang, Y. N., Fu, Z., et al. (2015). Photolysis of three antiviral drugs acyclovir, zidovudine and lamivudine in surface freshwater and seawater. Chemosphere 138, 792–797. doi:10.1016/j.chemosphere.2015.08.033
Keywords: azithromycin, environmental risk, hydroxychloroquine, ivermectin, pandemic, toxicology
Citation: Efrain Merma Chacca D, Maldonado I and Vilca FZ (2022) Environmental and ecotoxicological effects of drugs used for the treatment of COVID 19. Front. Environ. Sci. 10:940975. doi: 10.3389/fenvs.2022.940975
Received: 10 May 2022; Accepted: 14 July 2022;
Published: 10 August 2022.
Edited by:
Juliana Heloisa Pinê Américo-Pinheiro, Brazil University, BrazilReviewed by:
Sasan Zahmatkesh, University of Science and Technology of Mazandaran, IranFarooq Sher, Nottingham Trent University, United Kingdom
Copyright © 2022 Efrain Merma Chacca, Maldonado and Vilca. This is an open-access article distributed under the terms of the Creative Commons Attribution License (CC BY). The use, distribution or reproduction in other forums is permitted, provided the original author(s) and the copyright owner(s) are credited and that the original publication in this journal is cited, in accordance with accepted academic practice. No use, distribution or reproduction is permitted which does not comply with these terms.
*Correspondence: Franz Zirena Vilca, ZnppcmVuYXZAdW5hbS5lZHUucGU=