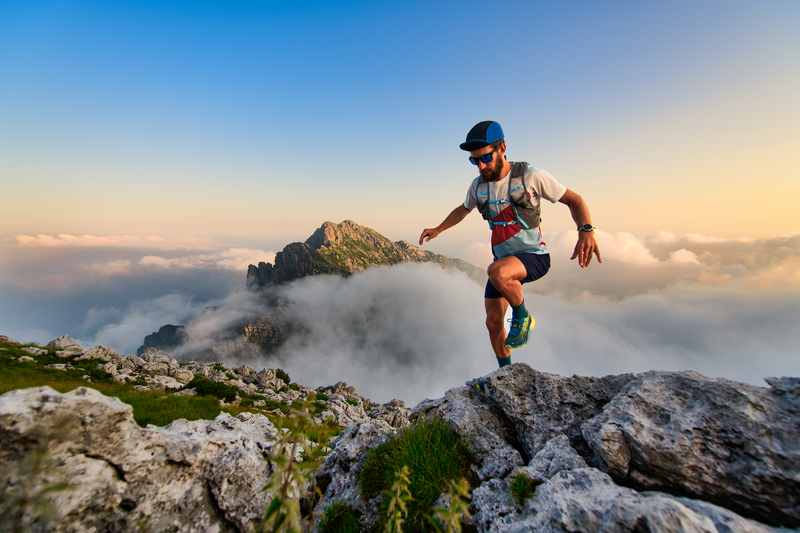
95% of researchers rate our articles as excellent or good
Learn more about the work of our research integrity team to safeguard the quality of each article we publish.
Find out more
ORIGINAL RESEARCH article
Front. Environ. Sci. , 08 August 2022
Sec. Toxicology, Pollution and the Environment
Volume 10 - 2022 | https://doi.org/10.3389/fenvs.2022.939860
This article is part of the Research Topic Chemometric Approach to Distribution, Source Apportionment, Ecological and Health Risk of Trace Pollutants View all 10 articles
The current study assessed the ecological–health risks of potentially toxic arsenic (As) and antimony (Sb) in the vegetable Centella asiatica, topsoils, and mangrove sediments sampled from Peninsular Malaysia. The As concentrations ranged from 0.21 to 4.33, 0.18 to 1.83, and 1.32 to 20.8 mg/kg dry weight, for the leaves, stems, and roots of the vegetable, respectively. The ranges of Sb concentrations were 0.31–0.62, 0.12–0.35, and 0.64–1.61 mg/kg dry weight, for leaves, stems, and roots of the vegetable, respectively. The children’s target hazard quotient (THQ) values indicated no non-carcinogenic risks of As and Sb in both leaves and stems, although children’s THQ values were higher than those in adults. The calculated values of estimated weekly intake were lower than established provisional tolerable weekly intake of As and Sb for both children and adult consumers. The carcinogenic risk (CR) values of As for children’s intake of leaves and stems of vegetables showed more public concern than those of adults. The levels of Sb and As in the topsoils were generally higher (although not significantly) than those in the mangrove sediments, resulting in a higher geoaccumulation index, contamination factor and ecological risk, hazard index, THQ, and CR values. This indicated that the anthropogenic sources of Sb and As originated from the land-based activities before reaching the mangrove near the coast. The CR of As signifies a dire need for comprehensive ecological–health risks exposure studies, as dietary intake involves more than just vegetable consumption. Therefore, risk management for As and Sb in Malaysia is highly recommended. The present findings of the ecological–health risks of As and Sb based on 2010–2012 samples can be used as an important baseline for future reference and comparison.
Reports on antimony (Sb) and arsenic (As) sources in Malaysia are scarce. In the central area of Sarawak, for example, Sb mineralization was found along the Lalang Fault Zone (Yeap et al., 1996), which also marks the boundary between the Upper Cretaceous Layar Member and the Eocene to Paleocene Kapit Member, both of which are part of the Belaga Formation. Pereira et al. (1994) also reported the use of soil geochemistry in the Buffalo Reef area, Kuala Medang, Pahang, to find Sb–Au mineralization. Recently, the concentrations of mineral-forming and trace elements including As and Sb were examined by Sia and Abdullah, (2012) in coal and coal ashes of the Balingian coalfield in Sarawak, Malaysia. However, the ecological and health effects of Sb and As in Malaysian soils were not addressed in the aforementioned studies.
In general, potentially toxic metals in the environmental media have received substantial concerns due to human health risk potentials (Egbueri et al., 2019; Ukah et al., 2019; Egbueri et al., 2020; Omeka et al., 2022). Murphy et al. (1989) developed risk assessment methodologies for two chronic As-contaminated soil exposure pathways: inhalation of fugitive dust emissions over a lifetime and unintentional soil/house dust intake. There is a wealth of knowledge about inorganic As oral toxicity, and several effects have been recorded, both non-carcinogenic and carcinogenic in nature. Fatigue, gastrointestinal complaints, irregular heart rhythm, bruises, and reduced nerve function can all occur due to ingestion (ATSDR, 2007). Other non-carcinogenic toxic effects due to As exposure include peripheral vascular diseases such as cyanosis, gangrene, and a condition known as “blackfoot disease” (Tseng et al., 2007). Oral ingestion of inorganic As has also been associated with an increased risk of cancer in the skin, liver, bladder, and lungs (Mandal, 2017). Bhattacharya et al. (2007) undertook a complete assessment of As in the environment, emphasizing the necessity for remediation of As-polluted groundwater, considering the carcinogenic toxicological effects on human health. According to Zhang et al. (2020), the combined effect of As in the atmosphere and groundwater may greatly worsen both the carcinogenic and non-carcinogenic effects associated with As pollution.
Sb is regarded as a valuable metal due to its vast range of applications, including catalysts, flameproof materials, ammunition, and bearings (Smichowski 2008; Reimann et al., 2010; Li et al., 2018; Wang et al., 2018). According to Zhou et al. (2015), based on projection scenarios employing the worldwide Sb emission inventory for 1995–2010, the global Sb emissions are expected to grow by a factor of two between 2010 and 2050. Sb is now recognized as a global pollutant, and it has recently sparked global concern (Muhammad et al., 2021; Chang et al., 2022). Sb has gradually become one of the prime hazardous elements of environmental interest due to anthropogenic activities’ elevated input (Kawamoto and Morisawa, 2003; Casado et al., 2007; Qi et al., 2011a). Environmental concerns of Sb in soils have been identified in Chinese coal mines (Qi et al., 2011a). In vitro and In vivo Sb, like As, is known to be a genotoxic element. However, little is known about the transfer of Sb from the environment to humans and its health risk (Wu et al., 2011; Muhammad et al., 2021). In the Yodo River basin, Kawamoto and Morisawa, (2003) investigated the distribution of Sb in the river water environment, which included water, sediment, aquatic vegetation, and fish. They found that the biota in the Yodo River basin had elevated Sb levels. Sb pollution in soils is a significant environmental issue, and it is critical to understand how Sb migrates and transforms in soils (Mu et al., 2022). To acquire a better picture of the environmental risk caused by Sb, Serafimovska et al. (2013) investigated the distribution of Sb and its species in soil fractions. According to Mu et al. (2022), Sb is geochemically stable in soil due to its high presence in the residue fractions.
Wilson et al. (2010) reviewed the environmental chemistry of inorganic Sb in soils and compared and contrasted their findings with those of As. Sb is a hazardous trace element primarily linked to As (Shtangeeva et al., 2011). Due to its greater mobility in the soil than Pb, this metal is thought to provide a greater long-term risk than Pb (Tschan et al., 2010). Nevertheless, knowledge concerning the biogeochemical properties of Sb is relatively limited compared to other frequent hazardous elements (Shtangeeva et al., 2011). Although Sb and As are generally comparable in biogeochemical behavior; discrepancies in regulating variables impacting their mobility and bioavailability in soils were evident and warrant further investigation (Chang et al., 2022). In some mineralized soils, Sb can be found in high amounts. Sb’s action in plants is poorly understood (Hajiani et al., 2015). Casado et al. (2007) investigated the extent of Sb and As pollution, their bioavailability in mining-affected grassland soils, and their concentrations in plant aboveground sections. Humans can be exposed to excessive levels of As and Sb via ingestion of polluted soil, dust, or food plants, which leads to serious health risks (Clemente, 2013; Muhammad et al., 2021). Furthermore, the food chain is a primary route for harmful substances to enter living creatures (Dubey et al., 2014). Tschan et al. (2009a) demonstrated that plants might absorb a considerable amount of Sb while appearing healthy.
Despite the growing concern about the ecological–health risks of As and Sb found in edible plants and mineralized topsoils, there is an acute lack of baseline knowledge regarding this aspect in Malaysia, or elsewhere in the Southeast Asia region. The vegetable plant Centella asiatica was selected given its reputation for being a good biomonitor of metals (Rainbow and Phillips, 1993; Ong, 2011; Ong, 2013). The aim of this study, therefore, was to assess the ecological–health risks of As and Sb in C. asiatica, topsoils, and mangrove sediments from Peninsular Malaysia.
In the present study, the mangrove sediments were collected from 10 sampling sites along the west coast of Peninsular Malaysia. These sites are flanked by a coastal fringe of mangrove forest, primarily in the vicinity of the residential area, hydroelectric power plant, fishing villages, shipping areas, tourism spots, fish and shrimp farms, and industrial area. The sampling seasons ranged from March to June 2012, between the seasons of the first inter-monsoonal period and the Southwest monsoon (Britannica, 2022). C asiatica plants, together with their habitat topsoils were collected from 12 sampling sites in Peninsular Malaysia. These sites are located near the residential area, agricultural areas such as oil palm plantation, industrial areas, highways, seaports, shop lots, and roadsides. The sampling seasons ranged from May to June 2010 during the Southwest monsoon season (Britannica, 2022).
Samples of the plant C. asiatica and their habitat topsoils were collected between March and June 2010, while the mangrove sediments were collected from the west coast between March and June 2012, from Peninsular Malaysia (Figure 1; Supplementary Table S1). Plant samples were collected from three parts: leaves, stems, and roots, while soils and sediments of the top 0–5 cm layer were collected.
FIGURE 1. Map showing the sampling sites in Peninsular Malaysia (See Supplementary Table S1). Note: numbers in black circles (1-10) are sampling sites (M1–M10 in Supplementary Table S1) for mangrove sediments without collection of Centella asiatica; numbers in white circles (1-12) are sampling sites (S1–S12 in Supplementary Table S1) for C. asiatica with habitat topsoils; and numbers in white circles (13–16) are sampling sites (S12–S16 in Supplementary Table S1) for topsoils only.
The leaves, stems, and roots from five plants were pooled for the analysis of As and Sb. Plants, topsoils, and mangrove sediment samples were dried at 65°C until the constant dry weight was achieved (approximately 5 days). Homogeneity of the dried samples was achieved by grinding them into a powder using an electronic agate homogenizer. The homogenous powder (0.15–0.20 g) of samples was manually shaken and sieved with a 63-µm siever placed into polyethylene vials. These polyethylene vials were heat-sealed until analysis.
Sb and As were analyzed using the TRIGA MARK II reactor at the Malaysian Nuclear Agency, Bangi, Selangor, Malaysia. Neutron flux of 4–5 × 1012 n/cm2 was used for long irradiation of Sb as its half-life is 60.9 days. Following the irradiation, the samples were allowed to cool for a while using closed-end coaxial high purity germanium detectors (Model GC3018 CANBERRA Inc and Model GMX 20180, EG4G ORTEC Nuclear Instrument) and its related electronics. Counting of cooling time ranged from 3 to 6 days, and the lifetime counting for Sb was 3,600 s (IAEA-TECDOC-1360, 2003).
For quality control and quality assurance, acid-washed apparatus was used to avoid external contamination. The Certified Reference Material (CRM) IAEA-SOIL-7 was prepared under identical conditions and used as quality control for each batch. The CRM used for this study was IAEA-SOIL-7 for quality control purposes where the recovery of Sb was 73.58% (certified: 1.70 ± 0.09 mg/kg dry weight; measured: 1.25 ± 0.27 mg/kg dry weight), while the As recovery was 89.25% (certified: 13.40 ± 0.67 mg/kg dry weight; measured: 11.96 ± 2.16 mg/kg dry weight). To achieve greater sensitivity, precision, and accuracy of the analysis, we used a detection limit of 0.001 mg/g for As and Sb by NAA.
The geoaccumulation index (Igeo) is determined by using the following formula (Eq. (1)) (Muller, 1969):
where
Cn = concentration of element;
Bn = concentrations of background reference for Sb (0.31 mg/kg dry weight) and As (2.00 mg/kg dry weight) by Wedepohl (1995); Factor 1.5 = background matrix correction factor due to lithogenic effects. The pollution intensities of Igeo were based on Muller (1969): “unpolluted” (<0); “unpolluted to moderately polluted” (0–1); “moderately polluted” (1–2); “moderately to strongly polluted” (2–3); “strongly polluted” (3–4); “strongly to very strongly polluted” (4–5); and “very strongly polluted” (>5).
Ecological risk (ER) of the individual element is an informative index reflecting the effects and toxicity of an element to the environment (Hakanson, 1980; Wang et al., 2018). First, the contamination factor (CF) is calculated as in Eq. 2 (Hakanson, 1980):
where CF = contamination factor of the element; Cs = the investigated metals in the soils or sediments; Cn = the background values for Sb and As by Wedepohl (1995).
Later, the ER is defined as the formula shown in Eq. 3 (Hakanson, 1980):
The toxic coefficient (Tr) for As was calculated as 10 based on Hakanson (1980). Since the Tr for Sb was not provided by Hakanson (1980), the Sb Tr was adopted from Wang et al. (2018) (7.0 mg/kg), which was calculated based on Hakanson’s principles.
Classifications based on Hakanson (1980) for CF are: “low contamination” (CF < 1); “mild contamination” (1 ≤ CF < 3); “considerable contamination” (3 ≤ CF < 6); and “high contamination” (CF ≥ 6). Classifications of the values of ER are (Hakanson 1980): “minimal potential ecological risk” (ER < 40); “mild potential ecological risk” (40 ≤ ER < 80); “considerable potential ecological risk” (80 ≤ ER < 160); “high potential ecological risk” (160 ≤ ER < 320); and “very high ecological risk” (ER ≥ 320).
For human health risk assessment (HHRA), the conversion factor (0.10) was applied to convert the dry weight (DW)-based data of As and Sb in leaves and stems of C. asiatica into wet weight (WW)-based data. To estimate the HHRA, three assessments were carried out, namely:
(a) Target hazard quotient
The estimated daily intake (EDI) and target hazard quotient (THQ) of elements were calculated to evaluate the once or long-term possible hazardous exposure to metals from eating edible leaves and stems of C. asiatica (USEPA, 1989) by the Peninsular Malaysian population. First, the EDI is calculated using the formula (Eq. 4):
where Mc is the concentrations of the element (mg/kg wet weight; after converted from dry weight-based data) in leaves and stems of C. asiatica. The body weights were 17 kg and 69.2 kg for children and adults, respectively (Nurul Izzah et al., 2012; Chong et al., 2017), while the consumption rates were 17.0 and 34.0 g/day for children and adults, respectively (Chong et al., 2017).
Second, the THQ is calculated based on Eq. 5:
The oral reference portion (RfD) was compared with the EDIs (µg/kg wet weight/day) of metals in vegetables. The RfD (µg/kg wet weight/day) values used in this study were based on USEPA regional screening level (USEPA, 2022) for Sb (0.40) and As (0.30).
(b) Comparisons between estimated weekly intake and provisional tolerable weekly intake
The Joint FAO/WHO Expert Committee on Food Additives (JECFA) considered As at its 33rd meeting (FAO/WHO, 1989) and confirmed its earlier evaluation by establishing a provisional tolerable weekly intake (PTWI) of 15 μg/kg body weight. Therefore, the present study used the PTWI of 15 μg/kg body weight for As (FAO/WHO, 1989). Thus, the As PTWI values for 17 and 69.2 kg body weights for children and adults in Malaysia are equivalent to 255 and 1,038 μg/week, respectively.
For Sb, based on the WHO (2017) and ATSDR (2019), which was initially based on drinking water quality guidelines (0.02 mg/L) for Sb and compounds (WHO, 2017), the tolerable daily intake (TDI) for Sb was recommended as 6 μg/kg/body weight. Therefore, 7 days of Sb TDI was calculated as 42 μg/kg/body weight. An adult with a body weight of 69.2 kg would have a PTWI of 2,906 μg/week, while a child with a body weight of 17 kg would have a PTWI of 714 μg/week.
In order to evaluate the risk exposure from C. asiatica consumption, the estimated weekly intake (EWI) values of Sb and As are calculated as follows using Eq. 6:
where EDI = [estimated daily intake calculated in Eq. 1] × 7 (number of days in a week). The comparison between calculated EWI and established PTWI limits for children and adults will reveal if the EWI values are lower than the established PTWI values.
(c) Carcinogenic risk of As in Centella asiatica
The incremental probability of an individual developing cancer during a lifetime is determined by the cancer risks (CRs) for As. For example, a CR of 1.0 × 10–4 indicates a probability of 1 in 10,000 individuals developing cancer (USEPA, 1989). The CR of As in the leaves and stems of C. asiatica was calculated by multiplying the average daily intake of As (in μg/kg/day over a lifetime) with a cancer slope factor (SF) according to Eq. (7).
Here, the SF actor for As after oral exposure has been set to 1.50 (mg/kg/day)− 1 by the USEPA (2022). According to USEPA methods, cancer risk lower than 1.0 × 10–6 is considered to be “negligible,” >1.0 × 10–4 is considered “unacceptable,” and in the range from 1.0 × 10–6 to 1.0 × 10–4 is considered “acceptable” (USEPA, 1989).
(a) Non-carcinogenic risks of As and Sb
The non-carcinogenic risk (NCR) of As and Sb to humans was measured by HHRA of topsoils and sediments via three exposure pathways: ingestion, inhalation, and skin contact based on the guidelines and Exposure Factors Handbook of the US Environmental Protection Agency (USEPA, 1986, 1989, 1997, 2001). The average daily doses (ADDs) (mg/kg day) of As and Sb through ingestion (ADDing), inhalation (ADDinh), and dermal contact (ADDder) for both children and adults are calculated by using Eqs. 8–10 as follows:
where ADDing, ADDinh, and ADDder are the daily amounts of exposure to As and Sb (mg/kg day) through ingestion, inhalation, and dermal contact, respectively. In this study, NCR values of As and Sb were assessed by using the hazard quotient (HQ) and hazard index (HI) (Chabukdhara and Nema, 2013; Qing et al., 2015). The definition, exposure factors, and reference values used to estimate the intake values and health risks of As and Sb in topsoils and sediments are presented in Supplementary Table S2.
The HQ is the proportion of the ADD of a metal to its reference dose (RfD) for the three exposure pathway(s) (USEPA, 1989), as shown in Eq. 11:
The RfD (mg/kg day) is the maximum daily dose of As and Sb from three exposure pathways for both children and adults, which will not pose significant risks of adverse effects on sensitive individuals throughout the course of their lives. ADD values lower than the RfD value (HQ ≤ 1) indicate no hazardous health effects, while higher ADD values than RfD values (HQ > 1) indicate potential hazardous health effects (USEPA, 1986, 2001). Despite substantial uncertainties, HQ values above 1 are regarded as an indicator of the potential risk of hazardous health effects to certain exposed individuals (USEPA, 1989).
(b) Carcinogenic risk of As in topsoils and sediments
The HHRA of topsoils and sediments was utilized to measure CR of As to humans by means of three exposure pathways, namely, ingestion, inhalation, and skin contact since the SF values were established for the three pathways for As but not for Sb (Cao et al., 2014; USEPA, 2022).
The CR was calculated by multiplying the average daily intake of As (in μg/kg/day over a lifetime) with an SF according to Eq. 12. The CR was estimated as the incremental probability of an individual developing cancer over a lifetime. For example, a CR of 1.0 × 10–4 indicates a probability of 1 in 10,000 individuals developing cancer (USEPA, 1989).
For both children and adults, the SF for As after oral exposure has been set to 1.5 (mg/kg/day)−1 by the USEPA (USEPA, 1989; Cao et al., 2014; USEPA, 2022). For inhalation and dermal contact pathways, they were 15.1 (mg/kg/day)−1 and 3.66 (mg/kg/day)−1, respectively (Cao et al. (2014). According to USEPA’s categories, a cancer risk lower than 1.0 × 10–6 is considered to be “negligible,” >1.0 × 10–4 is considered “unacceptable,” and in the range from 1.0 × 10–6 to 1.0 × 10–4 is considered “acceptable” (USEPA, 1989).
KaleidaGraph (Version 3.08, Synergy Software, Eden Prairie, MN, United States) was utilized to obtain the overall statistics of the data and to create the graphical bar charts in this study.
Figure 2 and Supplementary Table S3 show the levels of As and Sb. The As concentrations (mg/kg dry weight) ranged from 0.21 to 4.33, 0.18 to 1.83, and 1.32 to 20.8 for the leaves, stems, and roots of C. asiatica, respectively. The ranges of Sb concentrations (mg/kg dry weight) were 0.31–0.62, 0.12–0.35, and 0.64–1.61, for leaves, stems, and roots of C. asiatica, respectively. The As and Sb levels followed the order of roots > leaves > stems.
FIGURE 2. Mean concentrations (mg/kg dry weight) of Sb and As in leaves, stems, and roots of Centella asiatica and their habitat topsoils collected from 12 sampling sites (indicated by numbers in white circles (1–12) in Figure 1; S1–S12 in Supplementary Table S1) in Peninsular Malaysia. The X-axes are based on a logarithmic scale.
Figure 3 and Supplementary Table S4 show the EDI values in the leaves and stem for both children and adults. The values of Sb EDI for children and adults in the leaves ranged from 3.10 × 10–2 to 6.20 × 10–2 and 1.50 × 10–3 to 3.10 × 10–2, respectively. The values of Sb EDI in the stems ranged from 1.20 × 10–2 to 3.50 × 10–2 and 6.00 × 10–3 to 1.70 × 10–2, for children and adults, respectively (Supplementary Table S4). The values of As EDI for children and adults in the leaves ranged from 2.10 × 10–2 to 4.33 × 10–1 and 1.00 × 10–2 to 2.13 × 10–1, respectively. The values of As EDI in the stems ranged from 1.80 × 10–2 to 1.83 × 10–1 and 9.00 × 10–3 to 9.00 × 10–2, for children and adults, respectively (Supplementary Table S4).
FIGURE 3. Estimated dietary intake (EDI) values (µg/kg wet weight/day) of Sb and As in the leaves and stems of Centella asiatica collected from 12 sampling sites (numbers in white circles (1–12) in Figure 1; S1–S12 in Supplementary Table S1) in Peninsular Malaysia. The X-axes are based on a linear scale.
For Sb and As, the values of THQ in the leaves and stem in both children and adults are presented in Figure 4, and Supplementary Table S5. The values of Sb THQ for children and adults in the leaves ranged from 7.90 × 10–2 to 1.55 × 10–1 and 3.90 × 10–2 to 7.60 × 10–2, respectively. The values of Sb THQ for children and adults in the stems ranged from 2.90 × 10–2 to 8.80 × 10–2 and 1.40 × 10–2 to 4.30 × 10–2, respectively (Supplementary Table S5). The values of As THQ in the leaves ranged from 7.00 × 10–2 to 1.44 and 3.40 × 10–2 to 7.09 × 10–1, for children and adults, respectively. The values of As THQ in the stems ranged from 6.00 × 10–2 to 6.10 × 10–1 and 2.90 × 10–2 to 3.00 × 10–1, for children and adults, respectively (Supplementary Table S5).
FIGURE 4. Target hazard quotient (THQ) values (unitless) of As and Sb in the leaves and stems of Centella asiatica collected from 12 sampling sites (numbers in white circles (1–12) in Figure 1; S1–S12 in Supplementary Table S1) in Peninsular Malaysia. The X-axes are based on a linear scale.
Two major patterns can be identified. First, the children’s THQ values in both leaves and stems were higher than those in the adults. Second, all THQ values for Sb and As were below 1.0, except for As in children consuming the leaves collected from Kepala Batas (KBatas) in northwest Peninsular Malaysia.
Figure 5 and Supplementary Table S6 show the values of EWI for Sb and As in the leaves and stems in both children and adults. The values of Sb EWI for children and adults in the leaves ranged from 2.20 × 10–1 to 4.35 × 10–1 and 1.08 × 10–2 to 2.14 × 10–1, respectively. The values of Sb EWI for children and adults in the stems ranged from 8.10 × 10–2 to 2.47 × 10–1, and 4.00 × 10–2 to 1.22 × 10–1, respectively (Supplementary Table S6). The Sb PTWI values for a 17 and 69.2 kg body weight for children and adults in Malaysia are equivalent to 714 and 2,906 μg/week, respectively. Therefore, the EWI values for children and adults in the leaves and stems of C. asiatica were significantly lower than those of PTWI values of Sb.
FIGURE 5. Estimated weekly intake (EWI) values (µg/kg wet weight/week) of Sb and As in the leaves and stems of Centella asiatica collected from 12 sampling sites (numbers in white circles (1–12) in Figure 1; S1–S12 in Supplementary Table S1) in Peninsular Malaysia. The X-axes are based on a linear scale.
The values of As EWI for children and adults in the leaves ranged from 1.47 × 10–1 to 3.03, and 7.20 × 10–2 to 1.49, respectively. The values of As EWI for children and adults in the stems ranged from 1.26 × 10–1 to 1.28 and 6.20 × 10–2 to 6.29 × 10–1, respectively (Supplementary Table S6). The As PTWI for a 17 and 69.2 kg body weight for children and adults in Malaysia are equivalent to 255 and 1,038 μg/week, respectively. Therefore, the EWI values for children and adults in the leaves and stems of C. asiatica were significantly lower than those of PTWI values of As.
For As, the values of CR in the leaves and stems in both children and adults were presented in Figure 6 and Supplementary Table S7. The values of As CR for children and adults in the leaves ranged from 3.15 × 10–5 to 6.50 × 10–4, and 1.55 × 10–5 to 3.19 × 10–4, respectively. The values of As CR in the stems ranged from 2.70 × 10–5 to 2.74 × 10–4, and 1.33 × 10–5 to 1.35 × 10–4, for children and adults, respectively (Supplementary Table S7).
FIGURE 6. Carcinogenic risk (CR) of As in the leaves and stems of Centella asiatica collected from 12 sampling sites (numbers in white circles (1–12) in Figure 1; S1–S12 in Supplementary Table S1) in Peninsular Malaysia. The X-axes are based on a logarithmic scale.
According to USEPA methods, cancer risk lower than 1.0 × 10–6 is considered to be “negligible,” >1.0 × 10–4 is considered “unacceptable,” and in the range from 1.0 × 10–6 to 1.0 × 10–4 is considered “acceptable” (USEPA, 1989). Based on Figure 6, the As CR values of all sites were considered “acceptable” except for the 5 sites (out of 10) in children consuming stems that exceeded 1.0 × 10–4 which is considered “unacceptable,” while only 1 site exceeded the threshold limit for adults in the stem. For children consuming the leaves, six sites exceeded 1.0 × 10–4 which were considered “unacceptable” while five sites exceeded the threshold limit for adults in leaves. Therefore, these As CR values showed a public concern with 10–60% of the sampling sites exceeding the As CR threshold limit.
The levels of Sb and values of Igeo, Cf, and ER in both topsoils and mangrove sediments are presented in Figure 7 and Supplementary Table S8. The Sb concentrations (mg/kg dry weight) ranged from 2.21 to 6.61 and 0.001 to 1.72, for topsoils and mangrove sediments, respectively. Overall, the average Sb level (4.00) in the topsoils was higher than 0.97 in the mangrove sediments.
FIGURE 7. Concentrations of Sb (mg/kg dry weight), geoaccumulation index (Igeo), contamination factor (CF), and potential ecological risk index (ER) for Sb in the topsoils (numbers in white circles (1–16) in Figure 1; S1–S16 in Supplementary Table S1) and mangrove sediments (numbers in black circles (1–10) in Figure 1; M1–M10 in Supplementary Table S1) from Peninsular Malaysia. All the X-axes are based on a logarithmic scale, except for the X-axes of Igeo values which are based on a linear scale.
The Igeo ranges (2.25–3.83) of Sb in the topsoils were categorized between “moderately to strongly polluted” and “strongly polluted” while those (-0.86–1.89) of the mangrove were all categorized as “unpolluted” and “moderately polluted” (Muller, 1969). The CF ranges (7.11–21.3) of Sb in the topsoils were all under the category of “high contamination” while those (0.003–5.55) of the mangrove were all categorized as “low contamination” and “considerable contamination” (Muller, 1969).
The ER ranges (49.8–149) of Sb in the topsoils fell between “mild potential ecological risk” and “considerable potential ecological risk” while those (0.02–38.8) of the mangrove were all categorized as “minimal potential ecological risk” (Hakanson, 1980). Overall, the average Sb values of Igeo, CF, and ER in the topsoils were higher than those in the mangrove sediments.
Figure 8 and Supplementary Table S9 show the levels of As and values of Igeo, CF, and ER in both topsoils and mangrove sediments. The As concentrations (mg/kg dry weight) ranged from 9.38 to 68.2 and 8.75 to 44.6, for topsoils and mangrove sediments, respectively. Overall, the average As level (31.2) in the topsoils was higher than that (18.2) in the mangrove sediments.
FIGURE 8. Geoaccumulation index (Igeo), contamination factor (CF), and potential ecological risk index (ER) for As in the topsoils (numbers in white circles (1–16) in Figure 1; S1–S16 in Supplementary Table S1) and mangrove sediments (numbers in black circles (1–10) in Figure 1; M1–M10 in Supplementary Table S1) from Peninsular Malaysia. The X-axes are based on a logarithmic scale.
The Igeo ranges (1.64–4.51) of As in the topsoils were categorized between “moderately polluted” and “strongly to very strongly polluted” while those (1.54–3.89) of the mangrove were all categorized as “moderately polluted” and “strongly polluted” (Muller, 1969). The CF ranges (4.69–34.1) of As in the topsoils were categorized as “considerable contamination” and “high contamination” while those (4.38–22.3) of the mangrove sediments were categorized as “considerable contamination” and “high contamination” (Hakanson, 1980).
The ER ranges (46.9–341) of As in the topsoils fell between “mild potential ecological risk” and “very high ecological risk” while those (43.8–223) of the mangrove sediments were categorized as “mild potential ecological risk” and “high potential ecological risk” (Hakanson, 1980). Overall, the average As values of Igeo, CF, and ER in the topsoils was higher than those in the mangrove sediments.
The Sb values of HQing, HQinh, and HQder pathways and HI in the topsoils and sediments from Peninsular Malaysia in children and adults are presented in Figure 9 and Supplementary Table S10. In the children, the Sb values of HQing, HQinh, HQder, and overall HI in the topsoils ranged from 6.49 × 10–2 to 1.94 × 10–1, 5.20 × 10–6 to 1.56 × 10–5, 5.19 × 10–3 to 1.56 × 10–2, and 7.01 × 10–2 to 2.10 × 10–1, respectively. In the adults, the Sb values of HQing, HQinh, HQder, and overall HI in the topsoils ranged from 7.97 × 10–3 to 2.39 × 10–2, 2.14 × 10–6 to 6.42 × 10–6, 1.24 × 10–2 to 3.73 × 10–2, and 2.04 × 10–2 to 6.12 × 10–2, respectively.
FIGURE 9. Sb hazard quotient values of ingestion (HQing), inhalation (HQinh), and dermal contact (HQder) pathways and overall hazard index (HI) in the soils (numbers in white circles (1–16) in Figure 1; S1–S16 in Supplementary Table S1) and mangrove sediments (numbers in black circles (1–10) in Figure 1; M1–M10 in Supplementary Table S1) from Peninsular Malaysia, in children and adults. The X-axes are based on a logarithmic scale.
In children, the Sb values of HQing, HQinh, HQder, and overall HI in the mangrove sediments ranged from 2.94 × 10–5 to 5.06 × 10–2, 2.36 × 10–9 to 4.05 × 10–6, 2.35 × 10–6 to 4.05 × 10–3, and 3.18 × 10–5 to 5.46 × 10–2. In adults, the Sb values of HQing, HQinh, HQder, and overall HI in the mangrove sediments ranged from 3.61 × 10–6 to 6.21 × 10–3, 9.71 × 10–10 to 1.67 × 10–6, 5.64 × 10–6 to 9.70 × 10–3, and 9.25 × 10–6 to 1.59 × 10–2.
The Sb HI values based on the hazard quotients pathways of ingestion, inhalation, and dermal contact for Sb were all below 1.0 and greater in children compared to adults, and the Sb HI values in the topsoils were always higher than those in the mangrove sediments.
The As values of HQing, HQinh, HQder, and HI in the topsoils and sediments from Peninsular Malaysia in children and adults are presented in Figure 10 and Supplementary Table S11. In children, the As values of HQing, HQinh, HQder, and HI in the topsoils ranged from 3.68 × 10–1 to 2.68, 1.03 × 10–5 to 7.51 × 10–5, 1.44 × 10–3 to 1.04 × 10–2, and 3.69 × 10–1 to 2.69, respectively. There are 9 sites out of 16 where the HI values were above 1.00 via the ingestion pathway in the topsoil samples. In adults, the As values of HQing, HQinh, HQder, and HI in the topsoils ranged from 4.52 × 10–2 to 3.29 × 10–1, 4.25 × 10–6 to 3.09 × 10–5, 3.44 × 10–3 to 2.50 × 10–2, and 4.86 × 10–2 to 3.54 × 10–1, respectively.
FIGURE 10. As hazard quotient values of ingestion (HQing), inhalation (HQinh), and dermal contact (HQder) pathways in the soils (numbers in white circles (1–16) in Figure 1; S1–S16 in Supplementary Table S1) and mangrove sediments (numbers in black circles (1–10) in Figure 1; M1–M10 in Supplementary Table S1) from Peninsular Malaysia, in children and adults. The X-axes are based on a logarithmic scale.
In children, the As values of HQing, HQinh, HQder, and HI in the mangrove sediments ranged from 3.43 × 10–1 to 1.75, 9.63 × 10–6 to 4.91 × 10–5, 1.34 × 10–3 to 6.83 × 10–3, and 3.44 × 10–1 to 1.76, respectively. Only 1 site out of 10 where the HI value was above 1.00 via the ingestion pathway is the mangrove sediments. In adults, the As values of HQing, HQinh, HQder, and HI in the mangrove sediments ranged from 4.21 × 10–2 to 2.15 × 10–1, 3.97 × 10–6 to 2.02 × 10–5, 3.21 × 10–3 to 1.64 × 10–2, and 4.54 × 10–2 to 2.31 × 10–1, respectively.
Overall, there were 56% (9 out of 16 sites) in the topsoils for children with As hazard index values exceeding 1.00, in which almost all the As total HI values were contributed by the ingestion pathway. The HI values were greater in children than in adults, and the As HI values in the topsoils were always higher than those in the mangrove sediments.
The As values of CRing, CRinh, and CRder in the topsoils and sediments from Peninsular Malaysia in children and adults are presented in Figure 11 and Supplementary Table S12. In children, the As values of CRing, CRinh, and CRder in the topsoils ranged from 1.66 × 10–4 to 1.20 × 10–3, 4.67 × 10–8 to 3.40 × 10–7, and 6.46 × 10–7 to 4.70 × 10–6, respectively. In the adults, the As values of CRing, CRinh, and CRder in the topsoils ranged from 2.03 × 10–5 to 1.48 × 10–4, 1.93 × 10–8 to 1.40 × 10–7, and 1.55 × 10–6 to 1.13 × 10–5, respectively.
FIGURE 11. As carcinogenic risk (CR) values of ingestion (CRing), inhalation (CRinh), and dermal contact (CRder) pathways in the soils (numbers in white circles (1–16) in Figure 1; S1–S16 in Supplementary Table S1) and mangrove sediments (numbers in black circles (1–10) in Figure 1; M1–M10 in Supplementary Table S1) from Peninsular Malaysia, in children and adults. The X-axes are based on a logarithmic scale.
In children, the As values of CRing, CRinh, and CRder in the mangrove sediments ranged from 1.54 × 10–4 to 7.87 × 10–4, 4.36 × 10–8 to 2.22 × 10–7, and 6.03 × 10–7 to 3.07 × 10–6, respectively. In adults, the As values of CRing, CRinh, and CRder in the mangrove sediments ranged from 1.90 × 10–5 to 9.67 × 10–5, 1.80 × 10–8 to 9.16 × 10–8, and 1.44 × 10–6 to 7.36 × 10–6, respectively.
Based on USEPA methods, a cancer risk value below 1.0 × 10–6 is considered “negligible,” >1.0 × 10–4 is considered “unacceptable,” and in the range from 1.0 × 10–6 to 1.0 × 10–4 is considered “acceptable” (USEPA, 1989). Based on Figure 11, all the As children CR values for both topsoils and mangrove sediments in all sites based on the ingestion pathway exceeded 1.0 × 10–4 which is considered “unacceptable.” For As adults’ CR values of the ingestion pathway, there are four sites (out of 16 sites) that exceeded the threshold limit in the topsoil samples, while all sites in the mangrove sediments were considered “acceptable.” All the CR values for inhalation pathways in both topsoils and mangrove sediments were considered “negligible” since they are lower than 1.0 × 10–6. All the CR values for dermal contact pathways in both topsoils and mangrove sediments were considered “acceptable” since they were in the range from 1.0 × 10–6 to 1.0 × 10–4, and some are “negligible.”
The present ranges of As in the three parts of C. asiatica (0.31–1.61 mg/kg dry weight) from Peninsular Malaysia were comparable to various published research studies.
The present findings of As and Sb levels were in the order of roots > leaves > stems, which agrees with many reported studies on plants. Wilson et al. (2013) found substantially greater metalloid levels in the roots of plants compared to the sections above the ground, confirming our findings. Some researchers attributed Sb translocation from the roots to the upper part of the plants to Sb precipitation on the root membrane barriers, which hampered the transport of Sb solutions to the leaves (Tschan et al., 2009a). Tschan et al. (2009b) discovered the coherent relationship between plant Sb absorption and soluble Sb levels in the topsoil. The sites with Sb levels in C. asiatica from the present study indicated higher bioavailability of Sb in the sampling sites (Rainbow and Phillips, 1993). Plants growing near Sb pollution sources have greater Sb concentrations, according to Baroni et al. (2000).
Sb, being a non-essential element, is able to accumulate in plants (Ainsworth et al., 1991). Kabata-Pendias and Pendias, (2001) categorized plant tissue with 5–10 mg/kg of Sb as phytotoxic. Therefore, the present Sb range of 0.31–1.61 mg/kg dry weight in the three parts of Centella was not considered phytotoxic. According to Tschan et al. (2009a), the background ranges of Sb concentrations in the terrestrial plants were 0.20–50 mg/kg dry weight. This indicates that present Sb ranges were not elevated and hence can be considered the background level in the topsoils and mangrove sediments in Peninsular Malaysia. Kassem et al. (2004) reported that the Sb values ranged from 0.011 to 0.026 mg/kg dry weight in olive, eggplant, alfalfa, and cabbage leaves were grown in the Orontes River basin. Their data were significantly lower than those of the three parts in C. asiatica from the present study.
In the Pearl River Delta (PRD), South China, 112 pairs of soil and blooming cabbage were sampled from typical agricultural protection zones and vegetable-producing districts (Chang et al., 2022) to investigate the contamination levels of Sb and As in soils and harvested cabbages. Chang et al. (2022) reported that the ranges of Sb concentrations in the flowering cabbages across PRD were 0.015–0.20 mg/kg dry weight with a mean value of 0.038 mg/kg dry weight, exhibiting significant lower levels of Sb than radish leaves collected from the Xikuangshan mine area (range: 1.5–121 mg kg−1) (He, 2007). This value is also below the tolerable concentration of plants for Sb (5 mg/kg dry weight) proposed by Eikmann and Kloke, (1993), and the levels between 5 and 10 mg/kg dry weight of Sb in plant tissue were reported to be phytotoxic (Kabata-Pendias and Pendias, 1985).
The present ranges of As in the three parts of C. asiatica (0.21–20.8 mg/kg dry weight) from Peninsular Malaysia are comparatively higher than those in various published research studies.
Uddh-Söderberg et al. (2015) found that the As concentrations in lettuce ranged from 0.0022 to 4.3 mg/kg dry weight, while Bhattacharya et al. (2010) found that the total As concentrations in rice, wheat, pulses, and vegetables ranged from <0.0003 to 1.02 mg/kg dry weight in an arsenic-affected area of West Bengal (India). A reported study showed that the As concentrations measured for the lettuce, onion, radish, and bean greenhouse samples ranged from 0.00843–5.28 mg/kg wet weight (Ramirez-Andreotta et al., 2013). The present As ranges (0.2–20.8 mg/kg dry weight) in the leaves, stems, and roots of C. asiatica, in the current study, were greater than those reported in the studies mentioned above.
In Sweden and the European Union, there is no regulation for As in food (Uddh-Söderberg et al., 2015). The United Kingdom Food Standard Agency proposed a general As limit of 1.00 mg/kg in all foodstuffs (CAC, 2011). On the other hand, the Food Standard Agency of Australia and New Zealand (FSANZ, 2015) proposed a maximum level of total arsenic in cereal (1.00 mg/kg) and did not set restrictions on other food groups. The present As ranges (0.02–2.08 mg/kg wet weight) in the leaves, stems, and roots of Centella, from the present study, could be higher than the As limit by the United Kingdom Food Standard Agency (CAC, 2011) and the Food Standard Agency of Australia and New Zealand (FSANZ, 2015).
Haque et al. (2021) studied five heavy metals (including As) in the vegetables obtained from industrial, non-industrial, and As-contaminated regions and popular vegetable markets in Bangladesh. They reported that the As levels in vegetables were 0.15–0.55 mg/kg wet weight and 0.06–0.24 mg/kg wet weight for industrial and non-industrial areas, respectively. Qin et al. (2021) tested samples from vegetable plants cultivated in acidic mine water contaminated soil and found that the mean concentration of total As in the edible sections of leafy vegetables fell in the range of 0.41–7.61 mg/kg dry weight (mean: 2.39 mg/kg).
As concentrations in flowering cabbages across the PRD were reported to fall in the range of 0.021–1.20 mg/kg dry weight (mean: 0.15 mg/kg dry weight) (Chang et al., 2022). In comparison to previous reports, total As concentrations in the flowering cabbages in the current study were generally lower than those in the leafy vegetables collected near mining areas (range: 0.14–1.42 mg/kg dry weight with mean: 0.59 mg/kg dry weight for Shizhuyuan mine; range: 0.41–7.61 mg/kg dry weight with mean: 2.39 mg/kg dry weight for Dabaoshan mine) (Li L. et al., 2017; Qin et al., 2021). Furthermore, As levels in 96% of the flowering cabbages were lower than the Chinese National Standards’ (GB 2762–2017) tolerance limit (0.50 mg/kg) for pollutants in foods (Chang et al., 2022).
Zheng et al. (2022) found a wide range of total As concentration in vegetable samples (0.52–15.94 mg/kg dry weight; mean: 6.55 ± 5.18 mg/kg dry weight) from samples collected from As-polluted farmlands, which were attributed to anthropogenic activities in Guangxi province, China. The highest As concentrations were found in Romaine lettuce (15.94 mg/kg dry weight), leaf lettuce (10.48 mg/kg dry weight), and leaf mustard (11.10 mg/kg dry weight).
Varol et al. (2022) found the mean As levels in some leafy vegetables grown in Malatya province (Turkey) were the highest in purple basil (0.297 mg/kg), purslane (0.161 mg/kg), and parsley (0.1 mg/kg wet weight). However, the mean concentrations of As in all crops were lower than the permissible concentrations (MPCs) set by the United Kingdom Food Standard Agency (CAC, 2012) (1.00 mg/kg wet weight) and the Chinese Health Ministry (MHPRC, 2013) (0.50 mg/kg wet weight).
The children’s THQ values in both leaves and stems were higher than those in the adults. Second, all THQ values for Sb and As were below 1.0, except for As in children consuming the leaves collected from KBatas. This indicated that the THQ value (>1.00) of inorganic As in C. asiatica collected from KBatas exceeded the threshold value of 1, with a non-carcinogenic risk of As to the children. All EWI values for children and adults in the leaves and stems of Centella are lower than the PTWI for Sb and As which had further supported the aforementioned results of THQ values which were below 1.00.
Varol et al. (2022) reported that the As THQ of vegetables collected from Turkey was below the acceptable risk level of 1.00. Cheung et al. (2008) determined dietary exposure to three elements (including Sb) in food consumed by junior high school students in Hong Kong. The dietary intake of Sb was predicted as 0.567 μg (kg body weight) 1) week 1) for high-consumption high school students. Qin et al. (2021) reported that intake of vegetable plants cultivated on acidic mine water-polluted soils might pose considerable potential human health risk with a hazard quotient (HQ) of 2.7.
Wu et al. (2011) investigated the health risks and major routes of long-term human exposure to Sb on residents of the Tin Mine (XKS) Sb mine in China. They found that inhabitants near XKS had an inorganic dietary intake of As (107 μg/day), below the PTWI of 15 μg/kg body weight/week (equivalent to 129 μg A/day).
The As CR values are concerning as 10–60% of the studied sites of C. asiatica had higher values than the As CR threshold limit. Bhatti et al. (2013) reported that the As concentration of spinach leaves was 1.6–6.4 times greater than the maximum concentration of inorganic As in China (0.05 mg/kg fresh weight). The HI values were above 1 and CR levels were also above the highest target of 1 × 10–4, which identified the potential risk to humans from ingesting spinach leaves. Uddh-Söderberg et al. (2015) investigated the health risks from As in vegetables grown in private gardens close to 22 contaminated glass factories. They found that the increased levels of As in the soil of residential gardens were reflected in increased As levels of vegetables, and the intake of these vegetables might cause cancer risks in humans. They found that they did not exceed the threshold for chronic non-carcinogenic or acute effects; however, the reported As CR are inclusive of other non-native vegetables and total exposure.
Based on vegetables from Bangladesh, Haque et al. (2021) reported that the probabilistic risk, individual risk, and total cancer risk (TR) for As had surpassed the threshold level (1 × 10−4) and safe limit (1 × 10–6), denoting higher lifetime cancer risk due to long-term consumption of these vegetables.
The present ranges of Sb in the topsoils (2.21–6.61 mg/kg dry weight) and mangrove sediments (0.001–1.72 mg/kg dry weight) from Peninsular Malaysia are comparatively lower than those mentioned in many reported studies. Igeo, CF, and ER values for Sb are higher in the topsoils than in the mangrove sediments. The HI based on three pathways indicated no non-carcinogenic risks of Sb.
Overall, the topsoils’ mean Sb level (4.00) was higher than 0.97 in the mangrove sediments. Chang et al. (2022) reported that the total Sb contents in soils from typical agricultural protection and vegetable-producing regions of the PRD ranged from 0.20 to 7.20 mg/kg dry weight (mean: 1.60 mg/kg dry weight). This range is within the mean Sb concentration (0.54–2.93 mg/kg dry weight) in soils from various regions across China (He et al., 2012). About 91% of the soil samples were within the range of background Sb levels (0.80–3.00 mg/kg dry weight) for China soils (He, 2007). Furthermore, 95% of the total soil samples were lower than the maximum allowed Sb concentration (3.50 mg/kg dry weight) proposed for soils in the Netherlands (Crommentuijn et al., 2000).
The Sb ranges (Mean: 4.00 in the topsoils and 0.97 in the mangrove sediments) in the current study were lower than those mentioned in many reports from the mining area of Sb such as South and North Sb mines (total Sb: 3,061 mg/kg dry weight; 74.2–16,389 mg/kg dry weight) as reported by Li et al. (2014) and in the sediments of the Lianxi River (Xikuangshan Sb mine; Sb values of 57.11–7,315.7 mg/kg) (Wang et al., 2011) and an abandoned Sb ore deposit in Portugal (5,956 mg/kg dry weight; Pratas et al., 2005). In addition, the significantly higher Sb levels were also reported in the soil near the Xishan mine in Hunan province’s Lengshuijiang city (5,949 mg/kg; Qi et al., 2011a), in the surface soils of the Swiss shooting range (13,800 mg/kg dry weight; Johnson et al., 2005). Since Sb and its compounds have long-range transport properties, higher concentrations of Sb in topsoils could be attributable to air dust settlement (Tian et al., 2014).
However, the present Sb ranges were comparable to those in Xuzhou (China), with a mean of 3.46 mg/kg of Sb in urban soils (Xue et al., 2005), and 1.23 mg/kg of Sb was found in Chinese farming soils (Niu et al., 2013). Jiang et al. (2020) examined the levels of eight heavy metals (including As) in soil, groundwater, air, and locally produced grain (wheat and corn) and vegetables in a village located close to a battery factory in Xinxiang, Henan Province, China. They found that the main input of As originated from activities associated with sewage irrigation and battery plant deposition. Based on soils collected from the coal mines in the Huaibei and Huainan areas of Anhui Province, China, Qi et al. (2011a) identified that the mean Sb content in the 33 samples was 4.00 mg/kg, which is lower than that in coals from this region (6.20 mg/kg).
Based on mining-affected grassland soils, Casado et al. (2007) found that the range of total Sb contents in soils was 60–230 mg/kg, indicating a high degree of pollution of soils. Sia and Abdullah (2012) studied the concentrations of mineral-forming elements and trace elements in coal and coal ashes from Balingian coalfield, Sarawak, Malaysia, and reported an enriched content of Sb (96 ppm) in Balingian coal relative to their respective coal Clarke.
Patyar and Moghanlo, two mining regions in Iran, were studied for their soil and vegetation (Hajiani et al., 2015). In Moghanlo, the total Sb concentrations in soil were 358–3,482 mg/kg, while in Patyar, the total Sb concentrations were 284–886 mg/kg. Sb levels in plant shoots ranged from 0.8 to 287 mg/kg and 1.3 to 49 mg/kg, respectively. Achillea wilhelmsii and Matthiola farinosa were the strongest Sb accumulators among these species, with 141 and 132 mg/kg Sb in their leaves, respectively.
Based on Baoji urban soil, Li X. et al. (2017) studied seven toxic elements (including As and Sb). They found that prolonged industrial activities have enriched the Sb content (26.00 mg/kg mean value) in urban soil. Based on nine surface soil samples contaminated from lead/zinc and iron smelting operations and coal-fired power plants, Serafimovska et al. (2013) reported that the main part of total Sb (2.5–105 mg/kg dry weight) was associated with the residual fraction in all soils. Chang et al. (2022) investigated the contamination levels of Sb and As in soils and harvested cabbages across the PRD (South China) by collecting 112 pairs of soil and flowering cabbage samples from agricultural protection zones and vegetable-producing districts in the region. The levels of Sb pollution in PRD cabbages and soils were generally low, although the soils were moderately polluted by As.
The present ranges of As in the topsoils (9.38–68.2 mg/kg dry weight) and mangrove sediments (8.75–44.6 mg/kg dry weight) from Peninsular Malaysia are comparable to many reported studies in the literature. Igeo, CF, and ER values for As are also higher in the topsoils than those in the mangrove sediments. The hazard index based on three pathways indicated no non-carcinogenic risks of As. Higher and more As carcinogenic risk in the topsoils than in the mangrove sediments was observed.
Based on mining-affected grassland soils, Casado et al. (2007) found that total As contents in soils ranged from 42 to 4,530 mg/kg, denoting elevated levels of pollution in soils. Sia and Abdullah (2012) studied the concentrations of mineral-forming elements and trace elements in coal and coal ashes from Balingian coalfield, Sarawak, Malaysia, and reported an enriched content of As (as high as 181 ppm) in Balingian coal relative to their respective coal Clarke.
The mean As level (31.2) in the topsoils was higher (18.2) than in the mangrove sediments. The As levels from these two areas were higher than those (10 mg/kg dw) of the Swedish EPA (2009) generic soil guideline value for residential areas. Also, the As ranges in both areas were higher than the reported As UCC by Wedepohl (1995) of 2.00 mg/kg dry weight and close to EU recommended maximum acceptable limit for agricultural soil (20.0 mg/kg dry weight) (Rahman et al., 2007) for the mangrove sediments. Therefore, the topsoils have higher anthropogenic As sources that emptied into the coastal mangrove sediments in Peninsular Malaysia.
Chang et al. (2022) reported that the total As concentrations in soils collected from typical agricultural protection and vegetable-producing regions across the PRD ranged between 5.6 and 228 mg/kg dry weight. These values are similar to the range (15.2–174 mg/kg dry weight) reported in Dabaoshan mine and the surrounding areas (Qin et al., 2021) but much lower than those of the vegetable soils (360–1,054 mg/kg dry weight) collected from the vicinity of the Shizhuyuan mining area (Li L. et al., 2017). Furthermore, 52% of the studied soil contained As exceeding the risk screening value (40 mg/kg dry weight) proposed by the Chinese soil environmental quality risk control standard for soil contamination of agricultural land (GB15618-2018) (Chang et al., 2022).
Overall, 56% of topsoil samples (9 out of 16 sites) have As hazard index values exceeding 1.00 for children. Almost all the As total HI values are contributed by the ingestion pathway. The HI values are higher in children than in adults, and the As HI values in the topsoils are always higher than those in the mangrove sediments. All the As children CR values for both topsoils and mangrove sediments in all sites based on the ingestion pathway exceeded 1.0 × 10–4, which is considered “unacceptable.” For As adults' CR values of the ingestion pathway, 4 out of 16 sites exceeded the threshold limit in the topsoil samples, while all sites in the mangrove sediments are considered “acceptable.” All the CR values for inhalation pathways in both topsoils and mangrove sediments are “negligible” since they are lower than 1.0 × 10–6. All the CR values for dermal contact pathways in both topsoils and mangrove sediments are considered “acceptable” since they range from 1.0 × 10–6 to 1.0 × 10–4, and some are “negligible.”
Cao et al. (2014) investigated children’s health hazard risks and exposure levels to 12 elements (including As and Sb) in water, soil, dust, air, and locally produced food using field sampling and questionnaire-based surveys in China’s largest coking area. The food intake and air inhalation exposure pathways each provided about half of As exposure. They concluded that children who reside near coking operations face higher health risks from As and Sb, and that food safety is necessary to protect these children.
Total arsenic (As) concentrations in poultry feed, manure, agricultural soils, and food plants from Khyber Pakhtunkhwa Province, Pakistan were examined by Muhammad et al. (2021). In the edible sections of food plants, the total As concentrations ranged from 0.096 to 1.25 mg/kg. The mean daily intake (ADI), HQ, HI, and CR were used to determine the risk to human health. The HI was three times higher in children (2.1) than in adults (0.79), indicating that the children face non-cancer health hazards (HI index >1). For children, the CR values were somewhat higher (1.53 × 10–4) relative to USEPA and WHO limits (1 × 10–6 to 1 × 10–4), but for adults, a low CR was found from ingestion of selected food plants. They have concluded that the source of pollution in food plant was attributed to poultry manure leading to non-CR and CR in agricultural areas.
The ecological risk assessments (Igeo, CF, and ER) and health risks (HQ and HI) for Sb and As in the topsoils were higher than those in the mangrove sediments. The higher ecological–health risks of Sb and As can be explained from three perspectives.
First, the anthropogenic sources from industrial activities started far from the coastal area. Therefore, the topsoils collected near the urban and industrial areas with observable human activities had speculatively contributed to the higher levels of Sb and As indicated by the present findings. Some of the topsoils were collected near rivers adjacent to industrial areas, such as Butterworth and Kepala Batas, and nearby active agricultural areas where the use of pesticides was highly possible. Yap and Pang (2011) previously reported the sediments collected from drainage and rivers that had higher levels of heavy metals than the coastal sediments. Yap et al. (2022) recently reported that land uses played an important role of a contributing factor in the elevation of heavy metals in the topsoils of Peninsular Malaysia. These two studies clearly explain why the levels of Sb and As are higher in the topsoils than in the mangrove sediments.
Second, it has been shown that the elemental levels will decrease with increasing distance from the sources of pollution (Yap et al., 2007; Yap and Al-Mutairi, 2022), which might explain the lower levels of Sb and As in the mangrove sediments than those in the topsoils adjacent to anthropogenic sources. Various studies from other regions have reported similar findings (Qi et al., 2011a, 2011b; Hajiani et al., 2015; Qin et al., 2021).
Third, with the understanding that sediments are considered a sink of metal pollution (Szefer et al., 1995; Yap, 2018), the mangrove sediments are assumed to accumulate the highest levels of Sb and As. The present finding showed a reverse pattern indicating the mangrove forests acted as a phytoremediator of Sb and As that caused the lower levels of As and Sb in the mangrove sediments. Reasonably, the mangrove forests are effective phytoremediators of Sb and As since mangrove trees thrive in marshy ecosystems (Defew et al., 2005; Nazli and Hashim, 2010; Nirmal et al., 2011).
Concentrations of As and Sb in C. asiatica, topsoils, and mangrove sediments collected from Peninsular Malaysia were examined. The present findings indicated comparable levels of Sb in C. asiatica but comparatively higher levels of As in C. asiatica than those in previous studies. There were no non-carcinogenic risks of Sb and As in C. asiatica, although children are potentially exposed to higher risks than adults. The THQ values of As and Sb in the plant were below 1.00 for children and adult Malaysian consumers, denoting no non-carcinogenic risks of As and Sb via C. asiatica consumption, except for As in children consuming the leaves collected from the sampling site at Kepala Batas. We also found the calculated values of estimated weekly intakes below the established provisional tolerable weekly intakes of As and Sb for children and adults. However, the As CR of children in the leaves and stems of C. asiatica showed more public concern than that of adults, with 10–60% of the sampling sites exceeding the As CR threshold limit, which is considered “unacceptable.”
Risk assessments of As and Sb in topsoils and mangrove sediments indicated that the Sb ER ranges in the topsoils could reach “considerable potential ecological risk.” In contrast, those of As in the topsoils could reach “very high ecological risk.” The ER ranges of Sb in the mangrove sediments were all categorized as “minimal potential ecological risk,” while those of As in the mangrove sediments could reach “high potential ecological risk.” For the health risks of As and Sb in the topsoils and mangrove sediments, there was no non-carcinogenic risk of Sb in the topsoils and mangrove sediments. The Sb HI values were all below 1.0, although the values are higher in children than in adults. The Sb HI values in the topsoils are always higher than those in the mangrove sediments. Overall, there are 56% in the topsoils for children with As HI values exceeding 1.00. The ingestion pathway contributed almost all the As total HI values. Furthermore, a high ecological risk of As and carcinogenic risk of As in the topsoils and mangrove sediments were evident, with the HI values in children being higher than those in adults.
In conclusion, there were higher ecological–health risks of Sb and As in the topsoils than in the mangrove sediments. This indicates that anthropogenic sources of Sb and As originated from the land-based activities before reaching the mangroves. The unacceptable status based on As CR warrants future exposure research because dietary intake includes more than just C. asiatica and overall exposure results from several exposure pathways. Thus, minimizing As and Sb concentrations in vegetables is essential to safeguard human health and the environment from hazardous risks.
Therefore, establishing guidelines for Sb and As for agricultural soils and resourceful mangrove ecosystems in this region is highly recommended. This effort will ease the pollution caused by anthropogenic inputs to avoid similar disastrous chemical pollution as happened in Kim River, Southern Peninsular Malaysia (Yap et al., 2019).
The raw data supporting the conclusion of this article will be made available by the authors, without undue reservation.
Conceptualization, CKY; formal analysis, WHC; funding acquisition, CKY; investigation, KK and GHO; methodology, GHO, WHC, RH, and EC; project administration, CKY and WST; resources, WST, WMS, NAW, WHC, and MHI; supervision, CKY; validation, WST and RG; writing—original draft, CKY; writing—review and editing, CKY, WST, WHC, RN, RG, MHI, MHI, MM, HO, WC, FBE, HO, KAA, SAA, MS, MK, CFY, ARB, AB, HMHZ, TA, AN, MS, MAAR, GHO, GS, and LSW. All authors have read and agreed to the published version of the manuscript.
The present study was supported financially by the Fundamental Research Grant Scheme (FRGS), (Vote No.: 5524953) and by the Ministry of Higher Education, Malaysia.
The authors declare that the research was conducted in the absence of any commercial or financial relationships that could be construed as a potential conflict of interest.
All claims expressed in this article are solely those of the authors and do not necessarily represent those of their affiliated organizations, or those of the publisher, the editors, and the reviewers. Any product that may be evaluated in this article, or claim that may be made by its manufacturer, is not guaranteed or endorsed by the publisher.
The Supplementary Material for this article can be found online at: https://www.frontiersin.org/articles/10.3389/fenvs.2022.939860/full#supplementary-material
Ainsworth, N., Cooke, J. A., and Johnson, M. S. (1991). Biological Significance of Antimony in Contaminated Grassland. Water Air Soil Pollut. 57-58, 193–199. doi:10.1007/bf00282882
ATSDR (Agency for Toxic Substances and Disease Registry) (2007). Toxicological Profile for Arsenic. Atlanta, GA: The Agency for Toxic Substances and Disease Registry, the Public Health Service, or the U.S. Department of Health and Human Services. August 2007.
ATSDR (Agency for Toxic Substances and Disease Registry) (2019). Toxicological Profile for Antimony and Compounds. Atlanta, GA: The Agency for Toxic Substances and Disease Registry, the Public Health Service, or the U.S. Department of Health and Human Services. October 2019.
Baroni, F., Boscagli, A., Protano, G., and Riccobono, F. (2000). Antimony Accumulation in Achillea Ageratum, Plantago Lanceolata and Silene Vulgaris Growing in an Old Sb-Mining Area. Environ. Pollut. 109 (2), 347–352. doi:10.1016/s0269-7491(99)00240-7
Bhattacharya, P., Welch, A. H., Stollenwerk, K. G., McLaughlin, M. J., Bundschuh, J., and Panaullah, G. (2007). Arsenic in the Environment: Biology and Chemistry. Sci. Total Environ. 379, 109–120. doi:10.1016/j.scitotenv.2007.02.037
Bhattacharya, P., Samal, A. C., Majumdar, J., and Santra, S. C. (2010). Arsenic Contamination in Rice, Wheat, Pulses, and Vegetables: a Study in an Arsenic Affected Area of West Bengal, India. Water Air Soil Pollut. 213, 3–13. doi:10.1007/s11270-010-0361-9
Bhatti, S. M., Anderson, C. W. N., Stewart, R. B., and Robinson, B. H. (2013). Risk Assessment of Vegetables Irrigated with Arsenic-Contaminated Water. Environ. Sci. Process. Impacts 15, 1866–1875. doi:10.1039/c3em00218g
Britannica (2022). Climate of Malaysia. Available at: https://www.britannica.com/place/Malaysia/Climate (searched on May 24, 2022).
CAC (Codex Alimentarius Commission) (2011). Joint FAO/WHO Food Standards Programme Codex Committee on Contaminants in Foods, 5th Session. The Hague, NL: Codex Alimentarius Commission.
CAC (Codex Alimentarius Commission) (2012). Joint FAO/WHO Food Standards Programme Codex Committee on Contaminants in Foods, 6th Session. Maastricht: Codex Alimentarius Commission.
Cao, S., Duan, X., Zhao, X., Ma, J., Dong, T., Huang, N., et al. (2014). Health Risks from the Exposure of Children to as, Se, Pb and Other Heavy Metals Near the Largest Coking Plant in China. Sci. Total Environ. 472, 1001–1009. doi:10.1016/j.scitotenv.2013.11.124
Casado, M., Anawar, H. M., Garcia‐Sanchez, A., and Santa Regina, I. (2007). Antimony and Arsenic Uptake by Plants in an Abandoned Mining Area. Comm. Soil Sci. Plant Anal. 38 (9-10), 1255–1275. doi:10.1080/00103620701328412
Chabukdhara, M., and Nema, A. K. (2013). Heavy Metals Assessment in Urban Soil Around Industrial Clusters in Ghaziabad, India: Probabilistic Health Risk Approach. Ecotoxicol. Environ. Saf. 87, 57–64. doi:10.1016/j.ecoenv.2012.08.032
Chang, C., Li, F., Wang, Q., Hu, M., Du, Y., Zhang, X., et al. (2022). Bioavailability of Antimony and Arsenic in a Flowering Cabbage-Soil System: Controlling Factors and Interactive Effect. Sci. Total Environ. 815, 152920. doi:10.1016/j.scitotenv.2022.152920
Cheung, C. S. W., Kwong, K. P., Yau, J. C. W., and Wong, W. W. K. (2008). Dietary Exposure to Antimony, Lead and Mercury of Secondary School Students in Hong Kong. Food Addit. Contam. Part A 25 (7), 831–840. doi:10.1080/02652030701697751
Chong, K., Lee, S., Ng, S., Khouw, I., and Poh, B. (2017). Fruit and Vegetable Intake Patterns and Their Associations with Sociodemographic Characteristics, Anthropometric Status and Nutrient Intake Profiles Among Malaysian Children Aged 1-6 Years. Nutrients 9, 723. doi:10.3390/nu9080723
Clemente, R. (2013). “Antimony”, in Heavy Metals in Soils. (Springer Netherlands), 497–506. doi:10.1007/978-94-007-4470-7_18
Crommentuijn, T., Sijm, D., De Bruijn, J., Van den Hoop, M., Van Leeuwen, K., and Van de Plassche, E. (2000). Maximum Permissible and Negligible Concentrations for Metals and Metalloids in the Netherlands, Taking into Account Background Concentrations. J. Environ. Manag. 60, 121–143. doi:10.1006/jema.2000.0354
Defew, L. H., Mair, J. M., and Guzman, H. M. (2005). An Assessment of Metal Contamination in Mangrove Sediments and Leaves from Punta Mala Bay, Pacific Panama. Mar. Pollut. Bull. 50, 547–552. doi:10.1016/j.marpolbul.2004.11.047
Dubey, S., Shri, M., Misra, P., Lakhwani, D., Bag, S. K., Asif, M. H., et al. (2014). Heavy Metals Induce Oxidative Stress and Genome-wide Modulation in Transcriptome of Rice Root. Funct. Integr. Genomics 14, 401–417. doi:10.1007/s10142-014-0361-8
Ezugwu, C. K., Onwuka, O. S., Egbueri, J. C., Unigwe, C. O., and Ayejoto, D. A. (2019). Multi-criteria Approach to Water Quality and Health Risk Assessments in a Rural Agricultural Province, Southeast Nigeria. HydroResearch 2, 40–48. doi:10.1016/j.hydres.2019.11.005
Egbueri, J. C., Ukah, B. U., Ubido, O. E., and Unigwe, C. O. (2020). A Chemometric Approach to Source Apportionment, Ecological and Health Risk Assessment of Heavy Metals in Industrial Soils from Southwestern Nigeria. Int. J. Environ. Anal. Chem., 1–19. doi:10.1080/03067319.2020.1769615
Eikmann, T., and Kloke, A. (1993). “Nutzungs- und schutzgutbezogene Orientierungswerte für (Schad-)stoffe in Böden,” in Bondenschutz. Ergänzbares Handbuch der Maßnahmen und Empfehlungen für Schutz, Pflege und Sanierung von Böden Landschaft und Grundwasser. Editors D. Rosenkranz, H. Einsele, and H. M. Harreß.
FAO/WHO (Food and Agriculture Organization/World Health Organization) (1989). “Evaluation of Certain Food Additives and Contaminants,”. WHO Food Additive Report Series, No. 24 in International Programme on Chemical Safety (Geneva: World Health Organization).
FSANZ Food Standard Agency of Australia and New Zeeland (2015). Australia New Zealand Food Standards Code - Standard 1.4.1 — Contaminants and Natural Toxicants - F2015C00052. Available at: http://www.comlaw.gov.au/Details/F2015C00052/Download. (Accessed February 3, 2015).
Jamali Hajiani, N., Ghaderian, S. M., Karimi, N., and Schat, H. (2015). A Comparative Study of Antimony Accumulation in Plants Growing in Two Mining Areas in Iran, Moghanlo, and Patyar. Environ. Sci. Pollut. Res. 22 (21), 16542–16553. doi:10.1007/s11356-015-4852-5
Hakanson, L. (1980). An Ecological Risk Index for Aquatic Pollution Control. A Sedimentological Approach. Water Res. 14, 975–1001. doi:10.1016/0043-1354(80)90143-8
Haque, M. M., Niloy, N. M., Khirul, M. A., Alam, M. F., and Tareq, S. M. (2021). Appraisal of Probabilistic Human Health Risks of Heavy Metals in Vegetables from Industrial, Non-industrial and Arsenic Contaminated Areas of Bangladesh. Heliyon 7, e06309. doi:10.1016/j.heliyon.2021.e06309
He, M., Wang, X., Wu, F., and Fu, Z. (2012). Antimony Pollution in China. Sci. Total Environ. 421-422, 41–50. doi:10.1016/j.scitotenv.2011.06.009
He, M. (2007). Distribution and Phytoavailability of Antimony at an Antimony Mining and Smelting Area, Hunan, China. Environ. Geochem. Health 29, 209–219. doi:10.1007/s10653-006-9066-9
IAEA-TECDOC-1360 (2003). Collection and Preparation of Bottom Sediment Samples for Analysis of Radionuclides and Trace Elements. Vienna: IAEA. July 2003.
Jiang, Y., Ma, J., Ruan, X., and Chen, X. (2020). Compound Health Risk Assessment of Cumulative Heavy Metal Exposure: a Case Study of a Village Near a Battery Factory in Henan Province, China. Environ. Sci. Process. Impacts 22, 1408–1422. doi:10.1039/d0em00104j
Johnson, C. A., Moench, H., Wersin, P., Kugler, P., and Wenger, C. (2005). Solubility of Antimony and Other Elements in Samples Taken from Shooting Ranges. J. Environ. Qual. 34, 248–254. doi:10.2134/jeq2005.0248
Kabata-Pendias, A., and Pendias, H. (1985). Trace Elements in Soils and Plants. Boca Raton: FL: CRC Press.
Kabata-Pendias, A., and Pendias, H. (2001). Trace Elements in Soils and Plants. 3rd ed. Boca Raton, FL: CRC Press.
Kassem, A., Sarheel, A., and Al-Somel, N. (2004). Determination of Trace Elements in Soil and Plants in the Orontes Basin of Syria by Using Instrumental Neutron Activation Analysis. J. Radioanal. Nucl. Chem. 262 (3), 555–561. doi:10.1007/s10967-004-0475-x
Kawamoto, Y., and Morisawa, S. (2003). The Distribution and Speciation of Antimony in River Water, Sediment and Biota in Yodo River, Japan. Environ. Technol. 24 (11), 1349–1356. doi:10.1080/09593330309385679
Li, J., Wei, Y., Zhao, L., Zhang, J., Shangguan, Y., Li, F., et al. (2014). Bioaccessibility of Antimony and Arsenic in Highly Polluted Soils of the Mine Area and Health Risk Assessment Associated with Oral Ingestion Exposure. Ecotoxicol. Environ. Saf. 110, 308–315. doi:10.1016/j.ecoenv.2014.09.009
Li, J., Zheng, B., He, Y., Zhou, Y., Chen, X., Ruan, S., et al. (2018). Antimony Contamination, Consequences and Removal Techniques: A Review. Ecotoxicol. Environ. Saf. 156, 125–134. doi:10.1016/j.ecoenv.2018.03.024
Li, L., Hang, Z., Yang, W.-T., Gu, J.-F., and Liao, B.-H. (2017). Arsenic in Vegetables Poses a Health Risk in the Vicinity of a Mining Area in the Southern Hunan Province, China. Hum. Ecol. Risk Assess. Int. J. 23, 1315–1329. doi:10.1080/10807039.2017.1306433
Li, X., Wu, T., Bao, H., Liu, X., Xu, C., Zhao, Y., et al. (2017). Potential Toxic Trace Element (PTE) Contamination in Baoji Urban Soil (NW China): Spatial Distribution, Mobility Behavior, and Health Risk. Environ. Sci. Pollut. Res. 24, 19766–19749. doi:10.1007/s11356-017-9526-z
Mandal, P. (2017). An Insight of Environmental Contamination of Arsenic on Animal Health. Emerg. Contam. 3, 17–22. doi:10.1016/j.emcon.2017.01.004
MHPRC (Ministry of Health of the People’s Republic of China) (2013). National Food Safety Standard, Maximum Levels of Contaminants in Foods. Beijing, China. (GB2762–2012).
Mu, Z.-Q., Xu, D.-M., and Fu, R.-B. (2022). Insight into the Adsorption Behaviors of Antimony onto Soils Using Multidisciplinary Characterization. Int. J. Environ. Res. Public Health 19 (7), 4254. doi:10.3390/ijerph19074254
Muhammad, J., Xu, P., Khan, S., Su, J. Q., Sarwar, T., Nazneen, S., et al. (2021). Arsenic Contribution of Poultry Manure towards Soils and Food Plants Contamination and Associated Cancer Risk in Khyber Pakhtunkhwa, Pakistan. Environ. Geochem. Health. doi:10.1007/s10653-021-01096-6
Murphy, B. L., Toole, A. P., and Bergstrom, P. D. (1989). Health Risk Assessment for Arsenic Contaminated Soil. Environ. Geochem. Health. 11 (3-4), 163–169. doi:10.1007/BF01758667
Nazli, M. F., and Hashim, N. R. (2010). Heavy Metal Concentrations in an Important Mangrove Species, Sonneratia Caseolaris, in Peninsular Malaysia. Environ. Asia 3, 50–55.
Nirmal, I. J., Sajish, P. R., Nirmal, R., Basil, G., and Shailendra, V. (2011). An Assessment of the Accumulation Potential of Pb, Zn and Cd by Avicennia Marina (Forsk.) Vierh. In Vamleshwar Mangroves, Gujarat, India. Not. Sci. Biol. 3, 36–40. doi:10.15835/nsb315593
Niu, L., Yang, F., Xu, C., Yang, H., and Liu, W. (2013). Status of Metal Accumulation in Farmland Soils across China: from Distribution to Risk Assessment. Environ. Pollut. 176, 55–62. doi:10.1016/j.envpol.2013.01.019
Nurul Izzah, A., Aminah, A., Md Pauzi, A., Lee, Y. H., Wan Rozita, W. M., and Fatimah, S. (2012). Patterns of Fruits and Vegetable Consumption Among Adults of Different Ethnics in Selangor, Malaysia. Int. Food Res. J. 19, 1095–1107. doi:10.1177/1010539512458523
Omeka, M. E., Igwe, O., and Unigwe, C. O. (2022). An Integrated Approach to the Bioavailability, Ecological, and Health Risk Assessment of Potentially Toxic Elements in Soils within a Barite Mining Area, SE Nigeria. Environ. Monit. Assess. 194, 212. doi:10.1007/s10661-022-09856-2
Ong, G. H., Yap, C. K., Maziah, M., and Tan, S. G. (2011). Heavy Metal Accumulation in a Medicinal Plant Centella Asiatica from Peninsular Malaysia. J. Biol. Sci. 11 (2), 146–155. doi:10.3923/jbs.2011.146.155
Ong, G. H., Yap, C. K., Marziah, M., and Tan, S. (2013). Accumulation of Heavy Metals and Antioxidative Enzymes of Centella Asiatica in Relation to Metals of the Soils. Pertanika J. Trop. Agric. Sci. 36, 331–336.
Pereira, J. J., Yeap, E. B., and Ng, T. F. (1994). Application of Soil Geochemistry to the Detection of Sb-Au Mineralization in the Bufallo Reef Area, Kuala Medang, Pahang. Geo L. Soc. Malaydia Bull. 55, 125–155.
Pratas, J., Prasad, M. N. V., Freitas, H., and Conde, L. (2005). Plants Growing in Abandoned Mines of Portugal Are Useful for Biogeochemical Exploration of Arsenic, Antimony, Tungsten and Mine Reclamation. J. Geochem. Explor. 85, 99–107. doi:10.1016/j.gexplo.2004.11.003
Qi, C., Liu, G., Kang, Y., Lam, P. K. S., and Chou, C. (2011a). Assessment and Distribution of Antimony in Soils Around Three Coal Mines, Anhui, China. J. Air Waste Manag. Assoc. 61 (8), 850–857. doi:10.3155/1047-3289.61.8.850
Qi, C., Wu, F., Deng, Q., Liu, G., Mo, C., Liu, B., et al. (2011b). Distribution and Accumulation of Antimony in Plants in the Super-large Sb Deposit Areas, China. Microchem. J. 97, 44–51. doi:10.1016/j.microc.2010.05.016
Qin, J., Niu, A., Liu, Y., and Lin, C. (2021). Arsenic in Leafy Vegetable Plants Grown on Mine Water-Contaminated Soils: Uptake, Human Health Risk and Remedial Effects of Biochar. J. Hazard. Mater. 402, 123488. doi:10.1016/j.jhazmat.2020.123488
Qing, X., Yutong, Z., and Shenggao, L. (2015). Assessment of Heavy Metal Pollution and Human Health Risk in Urban Soils of Steel Industrial City (Anshan), Liaoning, Northeast China. Ecotoxicol. Environ. Saf. 120, 377–385. doi:10.1016/j.ecoenv.2015.06.019
Rahman, M. A., Hasegawa, H., Rahman, M. M., Rahman, M. A., and Miah, M. A. M. (2007). Accumulation of Arsenic in Tissues of Rice Plant (Oryza Sativa L.) and its Distribution in Fractions of Rice Grain. Chemosphere 69, 942–948. doi:10.1016/j.chemosphere.2007.05.044
Rainbow, P. S., and Phillips, D. J. H. (1993). Cosmopolitan Biomonitors of Trace Metals. Mar. Pollut. Bull. 26, 593–601. doi:10.1016/0025-326x(93)90497-8
Ramirez-Andreotta, M. D., Brusseau, M. L., Beamer, P., and Maier, R. M. (2013). Home Gardening Near a Mining Site in an Arsenic-Endemic Region of Arizona: Assessing Arsenic Exposure Dose and Risk via Ingestion of Home Garden Vegetables, Soils, and Water. Sci. Total Environ. 454-455, 373–382. doi:10.1016/j.scitotenv.2013.02.063
Reimann, C., Matschullat, J., Birke, M., and Salminen, R. (2010). Antimony in the Environment: Lessons from Geochemical Mapping. Appl. Geochem. 25 (2), 175–198. doi:10.1016/j.apgeochem.2009.11.011
Serafimovska, J. M., Arpadjan, S., Stafilov, T., and Tsekova, K. (2013). Study of the Antimony Species Distribution in Industrially Contaminated Soils. J. Soils Sedim. 13 (2), 294–303. doi:10.1007/s11368-012-0623-9
Shtangeeva, I., Bali, R., and Harris, A. (2011). Bioavailability and Toxicity of Antimony. J. Geochem. Explor. 110 (1), 40–45. doi:10.1016/j.gexplo.2010.07.003
Sia, S.-G., and Abdullah, W. H. (2012). Enrichment of Arsenic, Lead, and Antimony in Balingian Coal from Sarawak, Malaysia: Modes of Occurrence, Origin, and Partitioning Behaviour during Coal Combustion. Int. J. Coal Geol. 101, 1–15. doi:10.1016/j.coal.2012.07.005
Smichowski, P. (2008). Antimony in the Environment as a Global Pollutant: a Review on Analytical Methodologies for its Determination in Atmospheric Aerosols. Talanta 75 (1), 2–14. doi:10.1016/j.talanta.2007.11.005
Swedish EPA (2009). Riktvärden För Förorenad Mark: Modellbeskrivning Och Vägledning. Stockholm: Swedish EPA.
Szefer, P., Kusak, A., Szefer, K., Jankowska, H., WoŁowicz, M., and Ali, A. A. (1995). Distribution of Selected Metals in Sediment Cores of Puck Bay, Baltic Sea. Mar. Pollut. Bull. 30 (9), 615–618. doi:10.1016/0025-326x(95)00079-3
Tian, H., Zhou, J., Zhu, C., Zhao, D., Gao, J., Hao, J., et al. (2014). A Comprehensive Global Inventory of Atmospheric Antimony Emissions from Anthropogenic Activities, 1995-2010. Environ. Sci. Technol. 48 (17), 10235–10241. doi:10.1021/es405817u
Tschan, M., Robinson, B. H., Nodari, M., and Schulin, R. (2009a). Antimony Uptake by Different Plant Species from Nutrient Solution, Agar and Soil. Environ. Chem. 6, 144–152. doi:10.1071/en08103
Tschan, M., Robinson, B. H., and Schulin, R. (2009b). Antimony in the Soil - Plant System - a Review. Environ. Chem. 6 (2), 106–115. doi:10.1071/en08111
Tschan, M., Robinson, B., Johnson, C. A., Bürgi, A., and Schulin, R. (2010). Antimony Uptake and Toxicity in Sunflower and Maize Growing in SbIII and SbV Contaminated Soil. Plant Soil 334 (1–2), 235–245. doi:10.1007/s11104-010-0378-2
Tseng, C.-H., Chong, C.-K., Tseng, C.-P., and Centeno, J. A. (2007). Blackfoot Disease in Taiwan: Its Link with Inorganic Arsenic Exposure from Drinking Water. AMBIO A J. Hum. Environ. 36 (1), 82–84. doi:10.1579/0044-7447(2007)36[82:bditil]2.0.co;2
Uddh-Söderberg, T. E., Gunnarsson, S. J., Hogmalm, K. J., Lindegård, M. I. B. G., and Augustsson, A. L. M. (2015). An Assessment of Health Risks Associated with Arsenic Exposure via Consumption of Homegrown Vegetables Near Contaminated Glassworks Sites. Sci. Total Environ. 536 (536), 189–197. doi:10.1016/j.scitotenv.2015.07.018
Ukah, B. U., Egbueri, J. C., Unigwe, C. O., and Ubido, O. E. (2019). Extent of Heavy Metals Pollution and Health Risk Assessment of Groundwater in a Densely Populated Industrial Area, Lagos, Nigeria. Int. J. Energ Water Res. 3, 291–303. doi:10.1007/s42108-019-00039-3
USEPA (US Environmental Protection Agency) (1986). Superfund Public Health Evaluation Manual. Washington, DC, USA: U.S. Environmental Protection Agency, 1–86.
USEPA (US Environmental Protection Agency) (1989). Risk Assessment Guidance for Superfund. I: Human Health Evaluation Manual. Washington, DC: Office of Emergency and Remedial Response. EPA/540/1-89/002.
USEPA (US Environmental Protection Agency) (1997). Exposure Factors Handbook. Washington, DC, USA: National Center for Environmental Assessment, US EPA Office of Research and Development. EPA/600/P-95/002F.
USEPA (US Environmental Protection Agency) (2001). Baseline Human Health Risk Assessment Vasquez Boulevard and I-70 Superfund Site Demver. Washington, DC, USA: Co; U.S. Environmental Protection Agency.
USEPA (US Environmental Protection Agency) (2022). Human Health Risk Assessment. Regional Screening Level (RSL)—Summary Table as of May 2022. Available online: https://semspub.epa.gov/work/HQ/402369.pdf (accessed on May 24, 2022).
Varol, M., Gündüz, K., Sünbül, M. R., and Aytop, H. (2022). Arsenic and Trace Metal Concentrations in Different Vegetable Types and Assessment of Health Risks from Their Consumption. Environ. Res. 206, 112252. doi:10.1016/j.envres.2021.112252
Wang, X., He, M., Xi, J., and Lu, X. (2011). Antimony Distribution and Mobility in Rivers Around the World's Largest Antimony Mine of Xikuangshan, Hunan Province, China. Microchem. J. 97, 4–11. doi:10.1016/j.microc.2010.05.011
Wang, N., Wang, A., Kong, L., and He, M. (2018). Calculation and Application of Sb Toxicity Coefficient for Potential Ecological Risk Assessment. Sci. Total Environ. 610-611, 167–174. doi:10.1016/j.scitotenv.2017.07.268
Wedepohl, K. H. (1995). The Composition of the Continental Crust. Geochim. Cosmochim. Acta 59, 1217–1232. doi:10.1016/0016-7037(95)00038-2
WHO (World Health Organization) (2017). Guidelines for Drinking-Water Quality. Fourth edition. Geneva, Switzerland: World Health Organization. incorporating the first addendum.
Wilson, S. C., Lockwood, P. V., Ashley, P. M., and Tighe, M. (2010). The Chemistry and Behaviour of Antimony in the Soil Environment with Comparisons to Arsenic: A Critical Review. Environ. Pollut. 158 (5), 1169–1181. doi:10.1016/j.envpol.2009.10.045
Wilson, S. C., Leech, C. D., Butler, L., Lisle, L., Ashley, P. M., and Lockwood, P. V. (2013). Effects of Nutrient and Lime Additions in Mine Site Rehabilitation Strategies on the Accumulation of Antimony and Arsenic by Native Australian Plants. J. Hazard. Mater. 261, 801–807. doi:10.1016/j.jhazmat.2013.01.033
Wu, F., Fu, Z., Liu, B., Mo, C., Chen, B., Corns, W., et al. (2011). Health Risk Associated with Dietary Co-exposure to High Levels of Antimony and Arsenic in the World's Largest Antimony Mine Area. Sci. Total Environ. 409 (18), 3344–3351. doi:10.1016/j.scitotenv.2011.05.033
Xue, S. W., Yong, Q., and Shu, X. S. (2005). Accumulation and Sources of Heavy Metals in Urban Topsoils: a Case Study from the City of Xuzhou, China. Environ. Geol. 48, 101.
Yap, C. K., and Al-Mutairi, K. A. (2022). Ecological-Health Risks of Potentially Toxic Metals in Mangrove Sediments Near Estuaries after Years of Piggery Farming Bans in Peninsular Malaysia. Sustainability 14, 1525. doi:10.3390/su14031525
Yap, C. K., and Pang, B. H. (2011). Assessment of Cu, Pb, and Zn Contamination in Sediment of North Western Peninsular Malaysia by Using Sediment Quality Values and Different Geochemical Indices. Environ. Monit. Assess. 183, 23–39. doi:10.1007/s10661-011-1903-3
Yap, C. K., Ismail, A., Ching, H. L., and Tan, S. (2007). Interpretation of Copper and Zinc Contamination in the Aquatic Environment of Peninsular Malaysia with Special Reference to a Polluted River, Sepang River. Wetl. Sci. 5, 311–321.
Yap, C. K., Peng, S. H. T., and Leow, C. S. (2019). Contamination in Pasir Gudang Area, Peninsular Malaysia: What Can We Learn from Kim Kim River Chemical Waste Contamination? Int. J. Hum. Edu. Dev. 1 (2), 84–87. doi:10.22161/jhed.1.2.4
Yap, C. K., Chew, W., Al-Mutairi, K. A., Nulit, R., Ibrahim, M. H., Wong, K. W., et al. (2022). Assessments of the Ecological and Health Risks of Potentially Toxic Metals in the Topsoils of Different Land Uses: a Case Study in Peninsular Malaysia. Biology 11, 2. doi:10.3390/biology11030389
Yap, C. K. (2018). Sediment Watch: Monitoring, Ecological Risk Assessment and Environmental Management. New York, NY, USA: Nova Science Publishers.
Yeap, E. B., George, P., and Phillip, U. (1996). The Antimony Mineralization of Lalang Fault Zone, Central Sarawak. War. Geol. 22 (3), 1996.
Zhang, L., Gao, Y., Wu, S., Zhang, S., Smith, K. R., Yao, X., et al. (2020). Global Impact of Atmospheric Arsenic on Health Risk: 2005-2015. Proc. Natl. Acad. Sci. USA 117 (25), 13975–13983. doi:10.1073/pnas.2002580117
Zheng, X., Zhang, Z., Chen, J., Liang, H., Chen, X., Qin, Y., et al. (2022). Comparative Evaluation of In Vivo Relative Bioavailability and In Vitro Bioaccessibility of Arsenic in Leafy Vegetables and its Implication in Human Exposure Assessment. J. Hazard. Mater. 423, 126909. doi:10.1016/j.jhazmat.2021.126909
Keywords: ecological risk, health risk, arsenic and antimony, soil and sediment pollution, Peninsular Malaysia
Citation: Yap CK, Tan WS, Cheng WH, Syazwan WM, Azrizal-Wahid N, Krishnan K, Go R, Nulit R, Ibrahim MH, Mustafa M, Omar H, Chew W, Edward FB, Okamura H, Al-Mutairi KA, Al-Shami SA, Sharifinia M, Keshavarzifard M, You CF, Bakhtiari AR, Bintal A, Zakaly HMH, Arai T, Naji A, Saleem M, Abd Rahman MA, Ong GH, Subramaniam G and Wong LS (2022) Ecological–Health Risk of Antimony and Arsenic in Centella asiatica, Topsoils, and Mangrove Sediments: A Case Study of Peninsular Malaysia. Front. Environ. Sci. 10:939860. doi: 10.3389/fenvs.2022.939860
Received: 09 May 2022; Accepted: 22 June 2022;
Published: 08 August 2022.
Edited by:
Johnbosco C. Egbueri, Chukwuemeka Odumegwu Ojukwu University, NigeriaReviewed by:
Chinanu Unigwe, Federal University, NigeriaCopyright © 2022 Yap, Tan, Cheng, Syazwan, Azrizal-Wahid, Krishnan, Go, Nulit, Ibrahim, Mustafa, Omar, Chew, Edward, Okamura, Al-Mutairi, Al-Shami, Sharifinia, Keshavarzifard, You, Bakhtiari, Bintal, Zakaly, Arai, Naji, Saleem, Abd Rahman, Ong, Subramaniam and Wong. This is an open-access article distributed under the terms of the Creative Commons Attribution License (CC BY). The use, distribution or reproduction in other forums is permitted, provided the original author(s) and the copyright owner(s) are credited and that the original publication in this journal is cited, in accordance with accepted academic practice. No use, distribution or reproduction is permitted which does not comply with these terms.
*Correspondence: Chee Kong Yap, eWFwY2hlZUB1cG0uZWR1Lm15; Wan Hee Cheng, d2FuaGVlLmNoZW5nQG5ld2ludGkuZWR1Lm15
Disclaimer: All claims expressed in this article are solely those of the authors and do not necessarily represent those of their affiliated organizations, or those of the publisher, the editors and the reviewers. Any product that may be evaluated in this article or claim that may be made by its manufacturer is not guaranteed or endorsed by the publisher.
Research integrity at Frontiers
Learn more about the work of our research integrity team to safeguard the quality of each article we publish.