- 1Department of Oceanography and Coastal Sciences, Louisiana State University, Baton Rouge, LA, United States
- 2Department of Zoology, Weber State University, Ogden, UT, United States
- 3Department of Biological Sciences, University of North Carolina at Charlotte, Charlotte, NC, United States
- 4Baruch Marine Field Laboratory, University of South Carolina, Georgetown, SC, United States
The estuarine environments surrounding coastal Louisiana create favorable conditions for microbially mediated mercury (Hg) methylation and subsequent bioaccumulation by biota. In 2010, the Deepwater Horizon (DWH) oil spill released large amounts of oil which, despite having low Hg concentrations, had the potential to influence methylmercury (MeHg) bioavailability in the coastal zone. To explore this possibility, we assessed Hg concentrations and trophodynamics in the coastal Louisiana food web prior to and immediately following the DWH oil spill and compared these metrics with an adjacent coastal ecosystem in the northern Gulf of Mexico. We found no differences in MeHg concentrations between oysters collected in years prior to the spill (1986–2007) and those collected during or in the months immediately after the spill (May to December 2010). When comparing tissue MeHg concentrations and carbon and nitrogen stable isotope values across 13 species of bivalves, shrimp, crabs, fishes, and birds we found evidence of significant biomagnification within the coastal Louisiana food web driven by species’ trophic position and their use of differing basal carbon sources. In addition, Hg trophodynamics also differed between two adjacent coastal ecosystems, post-spill coastal Louisiana (2010) and pre-spill coastal Alabama (2008–2009). While there was a higher trophic magnification factor in coastal Louisiana relative to coastal Alabama, food web baseline MeHg concentrations were higher in coastal Alabama. The high degree of biomagnification in coastal Louisiana, and significant regional variation, underscores the need to monitor Hg trophodynamics over space and time to better evaluate the short and long-term ecological consequences of events like the DWH oil spill.
Introduction
Resulting from both natural and anthropogenic activities, mercury (Hg) has gained notoriety as a global pollutant and a threat to human health (Boening, 2000). The cycling of Hg in the environment is complex and difficult to measure, as it is present in several different chemical forms (Liu et al., 2011). While both organic and inorganic forms of Hg can be taken up actively or passively by the surrounding biota (Golding et al., 2002; Pickhardt and Fisher, 2007), methylmercury (MeHg) is particularly concerning due to its potential to bioaccumulate in organisms and biomagnify in food webs (Bloom, 1992; Boening, 2000; Bank et al., 2007). Biomagnification occurs when contaminant concentrations in consumers exceed the concentrations found in their prey (Borgå et al., 2012). Soil microbes that are generally associated with the reduction of sulfate and iron are primarily responsible for the transformation of Hg into a bioavailable form (i.e., MeHg) where it enters the food web (Compeau and Bartha, 1985; Golding et al., 2002). Larger organisms cannot readily take up MeHg directly from the water column or sediment, but are largely exposed to MeHg via their diet (Hall et al., 1997; Liu et al., 2011). In fish for example, MeHg tends to be sequestered in muscle tissue, and studies have demonstrated that nearly 60–100% of total Hg (THg) in fish muscle tissue is in the organic, MeHg, form (Cappon and Smith, 1981; Bloom, 1992; Harris, 2003). The lipophilic and protein-binding properties of MeHg make it difficult for organisms to eliminate MeHg, and chronic exposure can lead to the accumulation of MeHg concentrations high enough to have severe toxicological effects (Wiener and Spry, 1996; Leaner and Mason, 2001). Moreover, the relatively long residence time of MeHg in consumer tissues makes it more likely to biomagnify with trophic level, as higher trophic position predators must consume more prey biomass to satisfy their greater metabolic requirements (Harris, 2003; Chumchal et al., 2011).
Advances in the ecological use of stable isotopes as tracers of organic matter and nutrients through food webs now allow for the quantification of Hg and other contaminant dynamics and biomagnification in complex ecosystems (Jardine et al., 2006). This is because stable isotope analysis provides a reliable method of quantifying trophic positions and habitat usage, and, in turn, the trophodynamics of Hg and other heavy metals as well as organic contaminants and other lipophilic pollutants (Hallanger et al., 2011; Borgå et al., 2012; Masset et al., 2019). Carbon (13C/12C) and nitrogen (15N/14N) stable isotopes occur naturally and become assimilated in the tissues of organisms via the metabolic processing of nutrients from the organism’s diet. As there is minimal fractionation of carbon stable isotope values (δ13C) from the basal carbon source to primary and subsequent consumers (∼0.3‰) it can be a reliable tracer for consumer’s habitat and/or basal resource use (Peterson and Fry, 1987). While δ13C values are used in biomagnification studies less frequently than nitrogen stable isotope values (δ15N), several studies have found δ13C values in consumers to be related to the location and/or sources of Hg accumulation (Power et al., 2002; Kidd et al., 2003; Cardona-Marek et al., 2009). δ15N values are commonly used as a proxy for trophic position due a general increase in tissue δ15N values with each trophic step (Pinnegar and Polunin, 1999). Therefore, δ15N value-based estimates of trophic position are often combined with contaminant data to quantify a food web’s trophic magnification factor (TMF) which is a measure of the average diet-to-consumer transfer of contaminants in cases where diet is the main route of exposure and consumers cannot readily eliminate contaminants from their tissue (Borgå et al., 2012). This TMF approach has been successfully applied to studies of Hg biomagnification across a wide range of aquatic, estuarine, and marine food webs (Bisi et al., 2012; Lavoie et al., 2013; Rumbold et al., 2018; Fonseca et al., 2019).
The sub-tropical, estuarine environment surrounding the Mississippi River delta produces an abundance of economically important species, such as oysters, crab, menhaden, shrimp, and drum (Day et al., 1982). However, it also creates a favorable environment for microbially mediated MeHg production and bioaccumulation (Delaune et al., 2008; Jonsson et al., 2014; Taylor et al., 2019). Coastal Louisiana is also an active area for oil exploration, refining, and petrochemical industries, which are known sources of heavy metals (Pardue et al., 1992; Delaune et al., 2008). Between April and July 2010, the Deepwater Horizon (DWH) oil spill released approximately 779 million liters of oil into Louisiana’s coastal zone, in addition to the approximately 7.9 million liters of lipophilic chemical oil dispersants used in mitigation efforts (Kujawinski et al., 2011; McNutt et al., 2012). DWH oil itself had low concentrations of Hg (Wilhelm et al., 2007; Paris et al., 2012) and a study of coastal sediments bracketing the spill from 2009 to 2011 reported a mean THg concentration of 0.15 ± 0.03 parts per million (ppm) with no difference among years (Steffy et al., 2013). However, it is possible that oil and dispersants may have influenced Hg bioavailability at the base of coastal food chains via changes in sedimentation rates, microbial activity, and/or water chemistry (Perrot et al., 2019). In addition, in late April 2010, the State of Louisiana opened freshwater diversion structures in the Barataria Bay and Breton Sound basins to prevent oil from entering coastal marshes (Martínez et al., 2012). This practice, along with concurrent large rain events from multiple tropical cyclones, altered sea surface temperatures, river flux anomalies, and the quantity of fresh water entering the estuarine environment, had the potential to further influence the deposition of atmospheric Hg, MeHg production, and Hg bioaccumulation and biomagnification in Louisiana’s coastal zone (Pickhardt and Fisher, 2007; Black et al., 2012; Kleindienst et al., 2015; Hastings et al., 2016; O'Connor et al., 2016).
Elevated Hg levels in marine and freshwater fishes have been reported in several economically important species across Louisiana which are regularly monitored for safe seafood consumption (O'Connor, 1998; Kongchum et al., 2006; Katner et al., 2010; Apeti et al., 2012). In addition, past studies in coastal Louisiana have examined abiotic cycling of Hg in sediments and the water column, bioaccumulation in select species, and/or biomagnification in estuarine fishes (Bank et al., 2007; Katner et al., 2010; Black et al., 2012; Fry and Chumchal, 2012; Jonsson et al., 2014). The goal of this research was to build on these prior studies and provide a holistic assessment of Hg concentrations and Hg biomagnification through the coastal Louisiana food web prior to and immediately following the 2010 DWH oil spill. Specifically, our first objective was to assess the immediate effect DWH had on the amount of Hg incorporated into taxa at the base of a coastal Louisiana food web by comparing Hg concentrations of oysters collected after the oil spill (May–December 2010) to oysters collected by the Mussel Watch Program prior to the spill (1986–2007). Second, we assessed Hg concentrations and biomagnification potential across a coastal Louisiana food web following the DWH oil spill, from bivalves to seabirds, and identified drivers of Hg concentration using stable isotope analysis. Finally, we compared metrics of Hg trophodynamics and food web biomagnification between two adjacent coastal ecosystems in the northern Gulf of Mexico, specifically coastal Louisiana immediately following DWH oil spill (2010) and costal Alabama prior to the DWH oil spill (2008 and 2009).
Materials and methods
Oyster sampling in Louisiana
Following the Deepwater Horizon oil spill in 2010, the Natural Resource Damage Assessment (NRDA) collected eastern oysters (Crassostrea virginica; n = 38) from Upper and Lower Barataria Bay, Louisiana in September and November 2010 (Figure 1). Whole bodies of shucked oysters were homogenized and stored frozen (−20°C) prior to analysis. In addition to this NRDA dataset, we obtained pre-spill total Hg (THg) concentrations of eastern oysters from NOAA’s National Status and Trends Mussel Watch Program (MWP; NCCOS 2016). The MWP annually monitors pollutants in invertebrates across the Gulf of Mexico. We selected data collected from 1986 to 2007 from two monitoring sites in Barataria Bay: Bayou Saint Denis (Upper Barataria Bay) and Middle Bank (Lower Barataria Bay; Figure 1). Composite samples (∼20 individuals) were analyzed for THg in dry weight. Detailed information regarding sample collection and laboratory analysis can be found in Apeti et al. (2012).
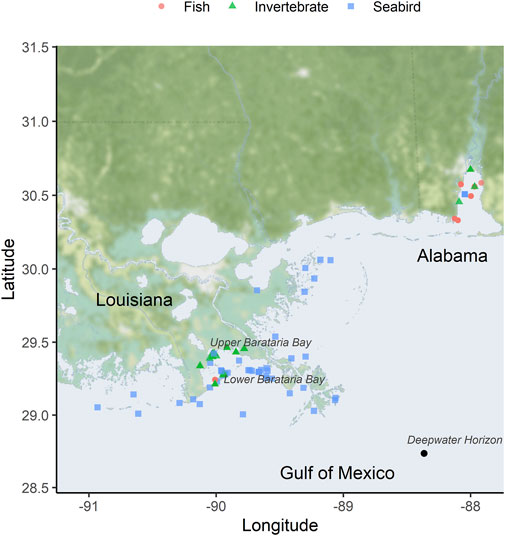
FIGURE 1. Study sampling locations in the northern Gulf of Mexico. Invertebrates, fishes, and seabirds around the Louisiana coast were collected in the aftermath of the 2010 Deepwater Horizon oil spill. Historic oyster samples were collected from the two locations in Barataria Bay by the Mussel Watch Program from 1986 to 2007. Upper Barataria Bay corresponds to Bayou St. Denis and Lower Barataria Bay corresponds to the Middle Bank. Alabama samples were collected by Showalter (2010) in 2008 and 2009.
Food web sampling in Louisiana
In addition to oysters, specimens of fish, crab, shrimp and adult seabird were collected from multiple locations in coastal Louisiana centered around Barataria Bay between May and December 2010 as part of NRDA and other DWH response efforts (Figure 1). Species collected included: blue crab (Callinectes sapidus), white shrimp (Litopenaeus setiferus), flathead grey mullet (also known as striped mullet; Mugil cephalus), white mullet (Mugil curema), Gulf menhaden (Brevoortia patronus), Atlantic croaker (Micropogonias undulatus), pinfish (Lagodon rhomboides), sand seatrout (Cynoscion arenarius), brown pelican (Pelecanus occidentalis), royal tern (Thalasseus maximus), black skimmer (Rynchops niger) and laughing gull (Leucophaeus atriclla). No collected individuals were visibly oiled, and all specimens were frozen (−20°C) prior to analysis.
Hg and stable isotope analysis of Louisiana samples
Homogenized whole-body tissue of oysters and crabs (excluding shell and carapace), white muscle tissue of shrimp and fish, and breast muscle tissue of seabirds were rinsed in deionized water, lyophilized for 24 h, ground into a homogenous powder and weighed in preparation for Hg and stable isotope analysis. We prioritized muscle tissue when possible because Hg has a strong affinity for the sulfhydryl groups attached to proteins when methylated and is sequestered into muscle tissues where it accumulates over time (Cappon and Smith, 1981; Bloom, 1992; Hall et al., 1997; Liu et al., 2011). In addition, it has a longer isotopic turnover time relative to other tissues (e.g., blood, liver, etc.) and generally reflects a consumer’s diet assimilated over a longer period of time (Post, 2002), which may vary from weeks to months depending on species.
Individual samples analyzed for total Hg (THg) concentrations were measured using a Nippon MA-3000 direct mercury analyzer following the US EPA Method 7473 (US EPA, 2007) at Southeast Missouri University. Approximately 10–20 mg of dried, homogenized tissue was analyzed per sample, with duplicate samples run every 10 samples to test for sample homogeneity. Two samples of standard reference materials (TORT-3) were run at the beginning of each run and after every 20 samples throughout the analysis. The mean percent recovery for TORT-3 was 98 ± 2%, and the method detection limit was 0.001. THg concentrations are reported in parts per million (ppm), dry weight.
THg concentration is commonly used as proxy of the bioavailable form (i.e., MeHg) in both applied and basic research. For example, the Louisiana Department of Environmental Quality’s Mercury Initiative regularly monitors THg levels in fish muscle to develop and implement consumption advisories (LDEQ, 1993). In addition, studies of Hg across a wide range of aquatic (e.g., reviewed by Eagles-Smith et al., 2016) and avian (e.g., reviewed by Ackerman et al., 2016) taxa regularly rely on THg and/or convert between THg and MeHg as needed to explore spatial and temporal patterns in Hg concentrations and biomagnification. This includes prior studies specific to mercury bioaccumulation in Louisiana’s estuarine food webs (Fry and Chumchal, 2012).
Therefore, we compiled data from prior studies that validated relationships between THg and MeHg concentrations in the tissues of the taxa examined in our study. For example, Apeti et al. (2012) found that tissue THg and MeHg concentrations in bivalves collected from the Central Gulf of Mexico sub-region had a strong positive correlation (r = 0.92, p < 0.001). Specifically, in Baritaria Bay they found that on average 46% of tissue THg was MeHg (Apeti et al., 2012; Table 1). Similarly, we compiled research that validated the percentage of THg that is MeHg in muscle tissue for blue crabs, penaeid shrimp, fishes, gulls, terns, and pelicans reflecting the taxa we sampled in Louisiana in 2010 (Table 1). Similar to Apeti et al. (2012), these studies of crustaceans (Kannan et al., 1998; Adams and Engel, 2014), fish (Bebbington et al., 1977; Kannan et al., 1998; Nilson et al., 2001; Senn et al., 2010; Xu et al., 2013), and seabirds (Kim et al., 1996; Ruelas-Inzunza et al., 2009) reported strong positive relationships between tissue THg and MeHg concentrations. The percentages of THg that is MeHg were then averaged for each consumer type: bivalves (46%), crustaceans (84%), fish (76%), and seabirds (91%; Table 1). These average percentages were used as conservative estimates to convert all THg concentrations to MeHg concentrations. This conversion was implemented as MeHg is the toxic species of Hg that bioaccumulates leading to deleterious organismal effects (Liu et al., 2011) and better satisfies the assumptions of TMF models (Borgå et al., 2012).
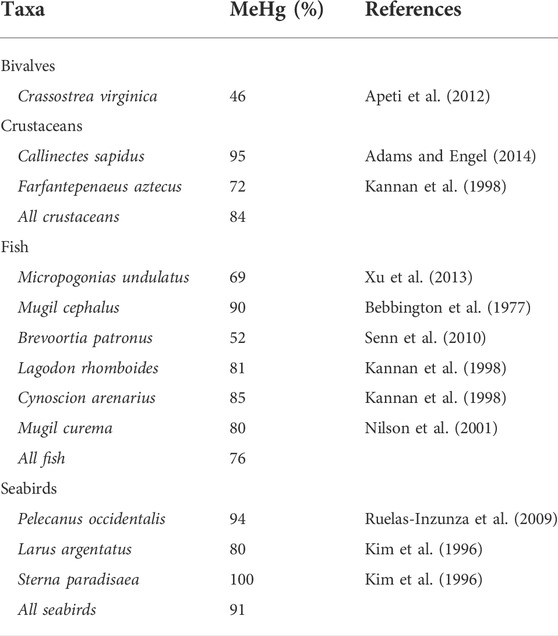
TABLE 1. Taxa-specific literature values of the average percentage of measured total mercury (THg) in dry muscle tissue that is present in the form of Methylmercury (MeHg). The percentages of THg that is MeHg averaged for each consumer type: bivalves (46%), crustaceans (84%), fish (76%), and seabirds (91%) were then used to convert all THg concentrations to MeHg concentrations.
Individual samples were analyzed for carbon (δ13C) and nitrogen (δ15N) stable isotope values using a Costech ECS 4010 elemental analyzer interfaced with a Delta XP continuous-flow stable isotope ratio mass spectrometer. Approximately 0.600 ± 0.025 mg of dried, homogenized tissue was analyzed per sample. Glutamic acid reference materials (USGS-40 and USGS-41) with high and low values were used to normalize the raw d values on a two-point scale. The analytical precision, based on standard deviations of repeated reference materials, were 0.1 and 0.2‰ for δ13C and δ15N, respectively. Reported in d notation in per mil units (‰), stable isotope ratios were calculated by using the following equation:
where X is 13C or 15N, R is the ratio of heavy/light isotope respectively (13C/12C, 15N/14N). The Rstandard values were based on the Vienna PeeDee Belemnite (VPDB) for δ13C and atmospheric N2 for δ15N. Samples with large C:N ratios (C:N > 3.5) were normalized for lipid content using Post et al.’s (2007) recommended mathematical correction:
Hg and stable isotope analysis of Alabama samples
Hg and stable isotope data were obtained from a food web study conducted in coastal Alabama by Showalter (2010) for comparison with our data collected from Louisiana. Only species common to both regions were included in this comparison, except for rangia clams (Rangia cuneata) and eastern oysters, which represent common filter-feeding, primary consumers in Alabama and Louisiana, respectively. The resulting dataset included muscle tissue THg concentrations and δ13C and δ15N values for rangia clams (n = 10), white shrimp (n = 2), sand seatrout (n = 11), Gulf menhaden (n = 5), flathead grey mullet (n = 5), Atlantic croaker (n = 19), adult brown pelicans (n = 5), and adult laughing gulls (n = 7) collected in Mobile Bay and Mississippi Sound between June–October 2008 and May–July 2009 (Figure 1; Table 2). Detailed collection and analysis protocols for this dataset are found in Showalter (2010). THg concentration in the Alabama dataset were converted from wet weight (wt.) to dry wt. using the average moisture content in the muscle tissue of each species in the Louisiana study and then converted to MeHg concentrations using the method listed above (Table 1).
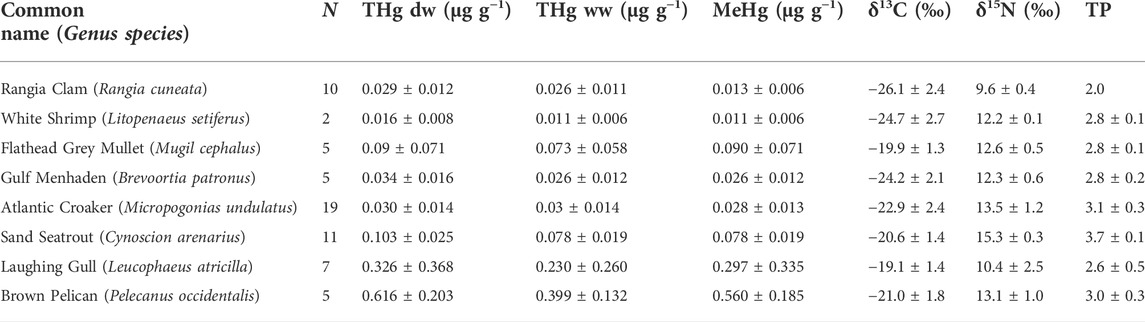
TABLE 2. Stable isotope values and Hg concentrations of consumers from coastal Alabama derived from Showalter (2010). Hg concentrations were converted from the original wet wt. measurement to dry wt. using moisture percentages estimated from moisture content measured in Louisiana species. Trophic position (TP) estimates were estimated using δ15N of bivalves as the isotopic baseline to compare subsequent consumers. MeHg concentrations were estimated using literature values averaged across groups of seabirds, fishes, and species-specific invertebrates (Table 1). The total number (N) of individuals sampled is reported for each species along with mean ±1 standard deviation for each variable.
Trophic position calculations
Borgå et al. (2012) recommends converting δ15N to relative trophic position (TP) when comparing TMFs across space and time to account for regional baseline differences. In accordance, we used the following single-baseline equation, from Post (2002), to estimate the TP of individual fish, invertebrates, and seabirds:
where δ15Nbase represents the mean value of primary consumers, oysters in Louisiana and rangia clams in Alabama, and are assumed to have a TP of 2, represented as λ. Secondary and subsequent consumer values are represented as δ15Nconsumer. The trophic enrichment factor, δ15Nfoodweb, corrects for the fractionation that occurs when energy is transferred between trophic levels. We assumed a trophic enrichment factor of 3.4‰, as this value has been found to be robust across multiple food webs and is suggested when there is a lack of prior knowledge Post (2002).
Statistical analysis
To achieve our first objective, we compared THg concentrations in oysters collected before the oil spill (1986–2007) by MWP to those collected immediately following the spill in 2010 by NRDA. For these comparisons we pooled MWP THg concentrations from all years to collectively represent the pre-spill group. For this specific analysis THg concentrations were not converted to MeHg, because we were comparing the same organism and the relative difference in bioaccumulative potential for each species of Hg was assumed to be constant. Three t-tests were conducted with one to compare THg between areas (Upper and Lower Barataria Bay) with pre- and post-spill samples pooled, and two more to compare pre- and post-spill THg but in separate areas (Upper and Lower Barataria Bay). For this analysis and subsequent analyses, we opted to use Bayesian estimation over frequentist or other estimation routines. Bayesian methods in general provide numerous advantages, such as exact inferences, robust small-sample size estimation, and incorporation of prior information. Furthermore, Bayesian probabilistic interpretation allows for understanding the magnitude of the effect rather than simply rejecting or failing to reject the binary outcome of a p-value. t-tests were run in JAGS that used a normal distribution for the THg response variable and normal vague priors for both groups and a vague uniform prior for the variance. Three MCMC (Markov chain Monte Carlo) chains were run each for 10,000 iterations with a burn-in of 2,000 iterations and thinning rate of three. Pairwise comparison between location (Lower vs. Upper) and time period (pre- vs. post-spill) were calculated as the posterior probability that mean estimates of THg concentration in one group are different in a one-way comparison with another group.
As part of our second objective, we used means parameterized one-way ANOVAs run in JAGS to compare MeHg, stable isotope values, and TP across all species collected in Louisiana. Descriptions of MCMC sampling and convergence were the same as those presented above for the t-tests. Pairwise differences were modeled as derived quantities and evaluated using the same criteria of difference described for the t-test above. Pairwise comparisons between species were calculated as the posterior probability that mean estimates of MeHg, stable isotope values and/or TP concentrations in one group are different in a one-way comparison with another group.
Next, we ran a linear regression in JAGS with the response variable of log (MeHg) concentrations regressed with two covariates (δ13C and δ15N values) to aid in identifying the ecological drivers of variation in MeHg within the coastal Louisiana food web. To directly compare the effect of each covariate, the covariates were standardized by mean centering and dividing by one standard deviation. Such standardizing allows for direct comparison of the estimated coefficients. Descriptions of MCMC sampling and convergence were the same as those presented above for the t-tests. δ13C and δ15N values were weakly correlated (r = 0.36), which allowed them both to be used in the model.
TP was not included in the above model given its inherent correlation with δ15N values (r = 0.97). However, we did use TP values to examine biomagnification in the coastal Louisiana food web by calculating a TMF based on the equations outlined in Borgå et al. (2012). Specifically, TMF was calculated by taking the anti-log of slope coefficient estimated by a linear regression of log (MeHg) concentrations as the dependent variable and TP as the independent variable. To aid in the biological interpretation of the regression intercept TP was transformed prior to modeling by subtracting 1 from all TP estimates, such that the model intercept represents log (MeHg) at a TP of 1. For this model we used all available samples collected from coastal Louisiana in 2010. Borgå et al. (2012) suggests that positive slopes that produce TMFs >1 indicate the occurrence of biomagnification up the food web. Therefore, we concluded that biomagnification was occurring if 0 was not contained within the 95% credible interval of posterior β. Descriptions of MCMC sampling and convergence were the same as those presented above for the t-tests.
Finally, to achieve our third objective, we calculated and compared TMFs between coastal Louisiana immediately following DWH oil spill (2010) and costal Alabama prior to the DWH oil spill (2008 and 2009). However, several species were not sampled in both food webs and species common to both food webs had different sample sizes. Given that ANOVA results suggested differences in MeHg concentration among species, if a species with high THg concentrations were overrepresented in one state, the resulting TMF may disproportionately reflect the presence or weight (i.e., sample size) of that species. Therefore, we first modeled and compared TMFs between Louisiana and Alabama after we subsampled each food web for only overlapping (i.e., common) species, with rangia clams and eastern oysters considered as trophic position analogs. To complement this primary analysis, we conducted a secondary comparison of TMFs after further sub-setting these common species so that species had the same sample sizes in each food web. This was accomplished by randomly selecting the same number of species observations from each state for each species. This iterative approach allowed us to conservatively quantify TMFs while also assessing the potential influences that differences in species and/or sample sizes might have when comparing biomagnification between food webs.
As noted above, TMFs were calculated using linear regression between TP and log (MeHg) with model intercepts representing the log (MeHg) at a TP of 1. Although a factor of interest, state (Louisiana and Alabama), makes sense as a random effect that would unify the models, we wanted to avoid running a model with a random effect that included only two levels. Specifically, the expected coefficient shrinkage in a model with only two levels of a random effect could dampen any estimates, in addition to the fact that we are hypothesizing that Alabama and Louisiana samples may be different and not necessarily representative of random effects (in this case). To assess if our random subsampling approach influenced model estimates we ran 20 model iterations each with different random samples. Although the numerical coefficient estimates varied slightly with each iteration, they never changed in their significance and never overlapped with each other supporting the validity of this approach. Pairwise comparisons for both the “common species” and “common species/sample size” models between location (Louisiana and Alabama) were calculated as the posterior probability that mean estimates of intercept, slope, and TMF from one location are different in a one-way comparison with the other location.
Results
Oyster Hg: Pre- vs. post- spill
All t-test models converged (based on
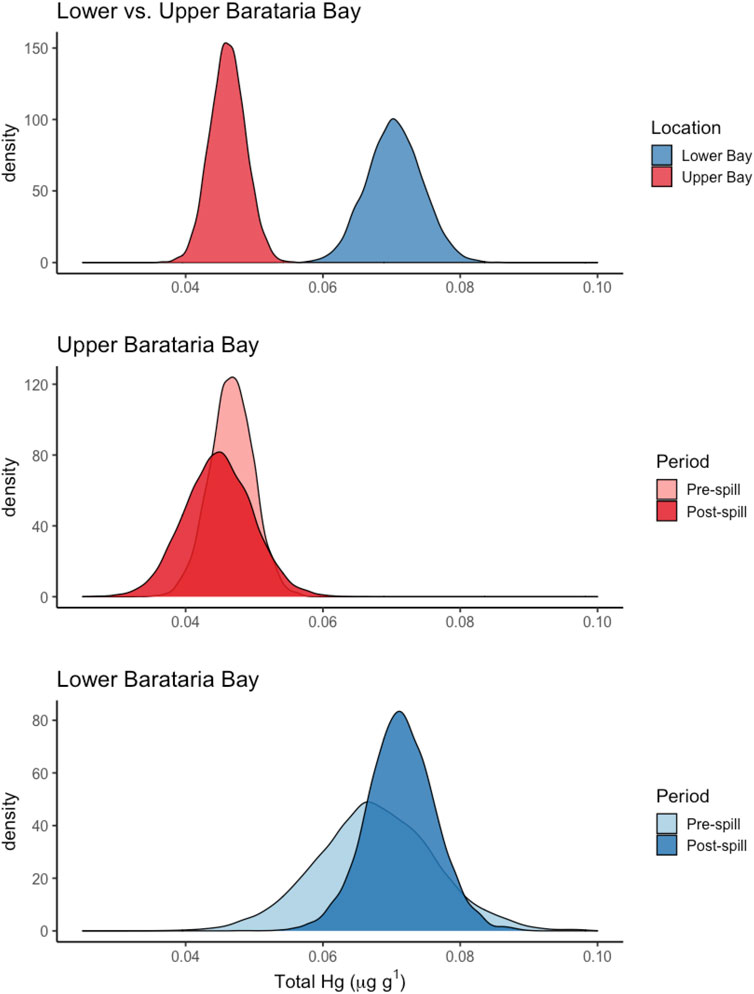
FIGURE 2. Posterior distributions estimated from Bayesian t-tests to compare THg dry wt. concentrations in oysters at two different locations in Barataria Bay before (pre-spill) and after (post-spill) the 2010 Deepwater Horizon oil spill.
Hg and stable isotope values in coastal Louisiana
All ANOVA models converged (based on
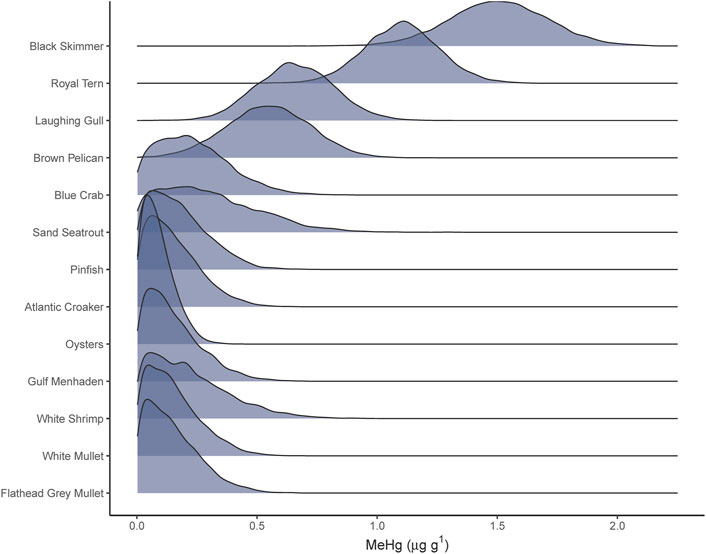
FIGURE 3. Posterior distributions estimated from Bayesian ANOVA to compare MeHg dry wt. concentrations across 13 species of bivalves, crustaceans, fish and seabirds in the aftermath of the 2010 Deepwater Horizon oil spill.
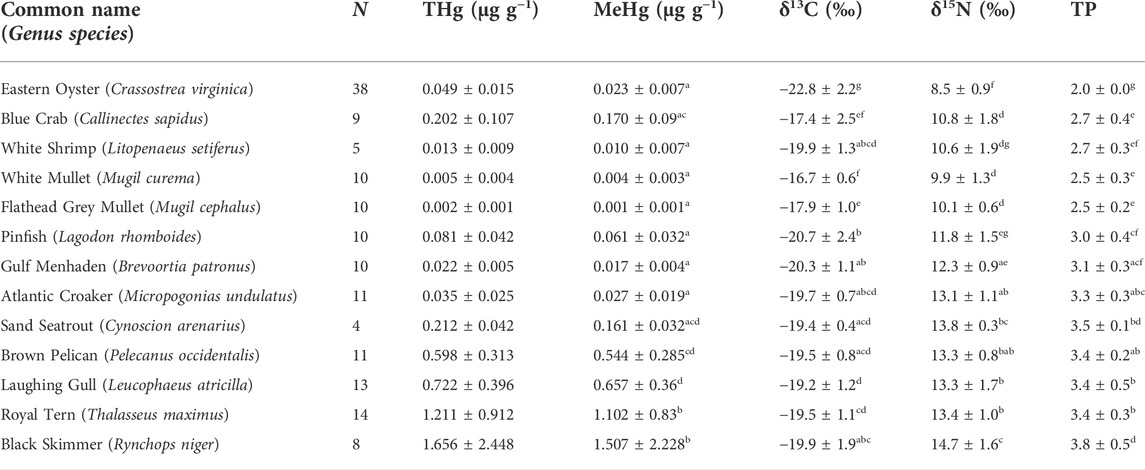
TABLE 3. Stable isotope values and Hg concentrations measured in tissues of consumers collected in coastal Louisiana following 2010 Deepwater Horizon oil spill. Trophic position (TP) estimates were estimated using δ15N of bivalves as the isotopic baseline to compare subsequent consumers. MeHg concentrations were estimated using literature values averaged across groups of seabirds, fishes, and species-specific invertebrates (Table 1). The total number (N) of individuals sampled is reported for each species along with mean ±1 standard deviation for each variable. Results of post hoc comparisons of each variable across species are represented by lettered superscripts. Species that do not share the same letter within the same column have pairwise comparisons with posterior probabilities ≥95%.
The linear regression that included δ13C values (habitat proxy) and δ15N values (trophic position proxy) converged (based on
Trophic magnification factors in coastal Louisiana
The slope of the relationship between log (MeHg) and TP in the coastal Louisiana food web was estimated to have a mean value of 0.786 with an intercept of −2.757 (Figure 4). This intercept equates to a mean MeHg concentration of 0.063 μg g−1 (95% CI: 0.043, 0.093) at a TP of 1. The 95% credible interval around the slope estimate (0.588–0.989) excludes 0, indicating a significantly non-zero slope. The mean antilog of the slope for the coastal Louisiana food web (or TMF) was 6.288 (95% CI: 3.865, 9.560), which is greater than the commonly used biomagnification threshold of 1.
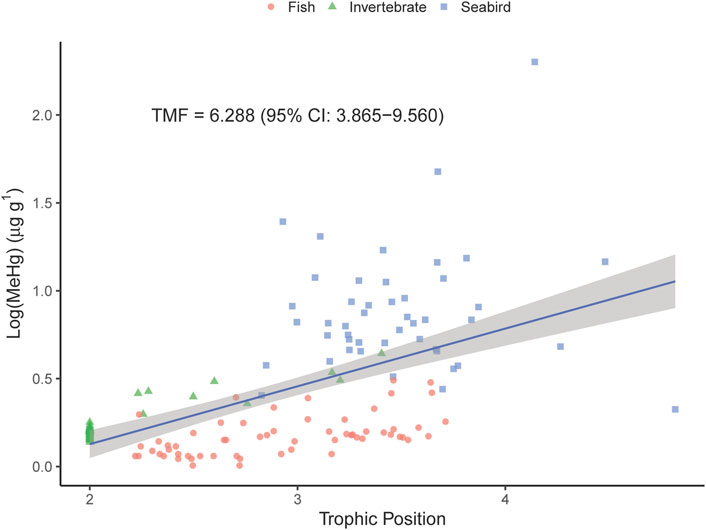
FIGURE 4. The relationship between consumers’ MeHg (log-transformed) burden and trophic positions in Louisiana. The overall fit of the line is represented by the grey shaded area. Trophic magnification factors (TMF) are derived from the anti-log of the slope coefficient and indicate biomagnification is occurring when values exceed 1.
Trophic magnification factors comparisons between Louisiana and Alabama
All regression models for Alabama and Louisiana TMF estimates converged (based on
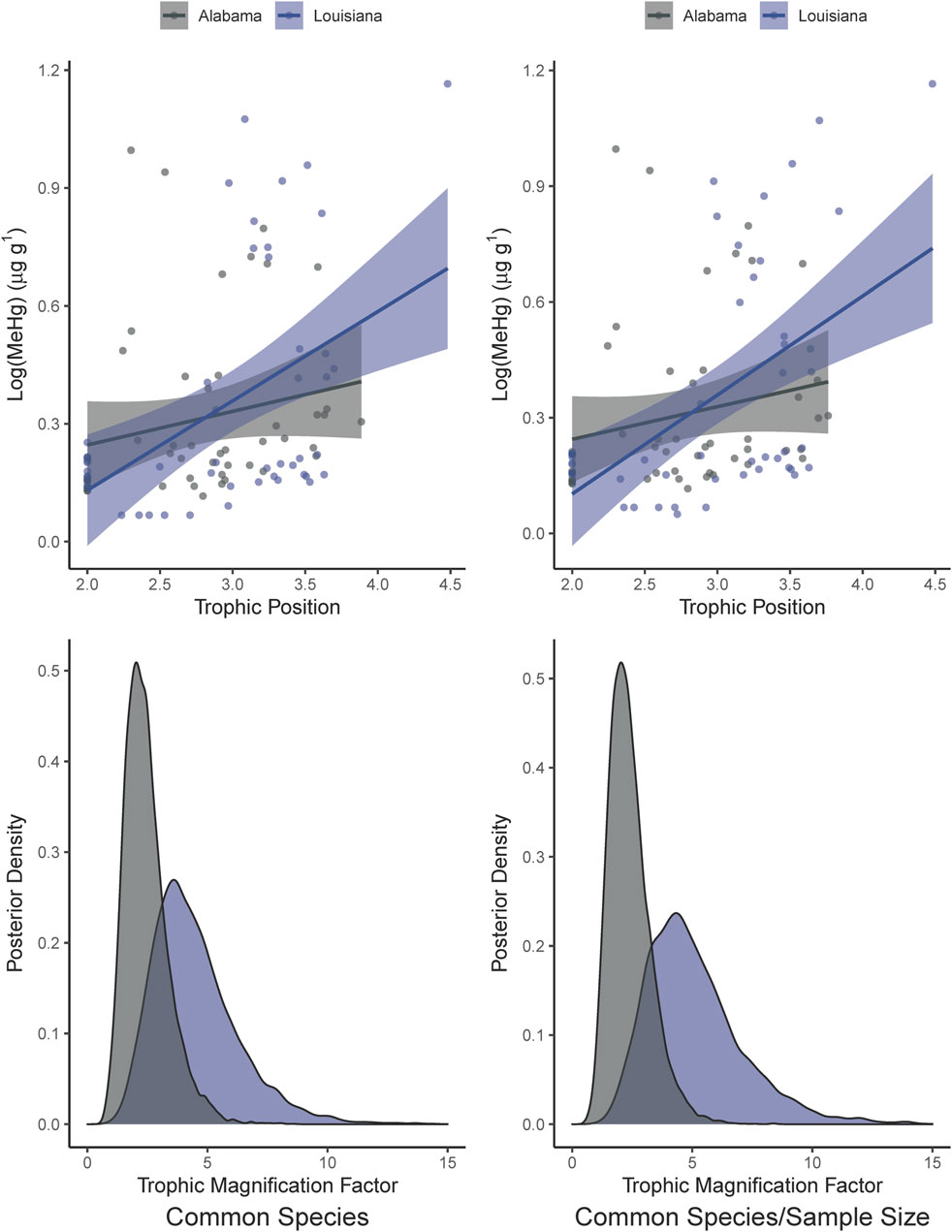
FIGURE 5. The relationship between consumers’ MeHg (log-transformed) burden and trophic positions and estimated posterior distributions of trophic magnification factors (TMF) in Louisiana and Alabama using common species but differing samples sizes or common species and standardized samples sizes.
For models that included common species and balanced sample sizes the intercept, slope, and TMF mean estimates were 2.01 (95% CI: 2.56, –1.44), 0.37 (95% CI: 0.06, 0.67), and 2.305 (95% CI: 1.108, 4.501), respectively for Alabama (Figure 5). The Alabama regression intercept equates to a mean MeHg concentration of 0.143 (95% CI: 0.081, 0.255) μg g−1 at a TP of 1. The mean intercept, slope, and TMF estimates for Louisiana were −2.63 (95% CI: 3.22, −2.01), 0.63 (95% CI: 0.33, 0.92), and 4.973 (95% CI: 2.002, 10.460), respectively (Figure 5). The Louisiana regression intercept equates to a mean MeHg concentration of 0.072 (95% CI: 0.036, 0.144) μg g−1 at a TP of 1. Pairwise comparisons of model parameters indicated that intercept estimates were generally higher in Alabama relative to Louisiana (posterior probability: 92%), while slope and TMF estimates were higher in Louisiana relative to Alabama (posterior probabilities: 90%; Figure 5).
Discussion
When comparing samples collected before and after the DWH oil spill we found little evidence to suggest that the DWH event, either directly or indirectly, led to changes in the concentration of Hg assimilated into the tissues of consumers at the base of the coastal Louisiana food web. Even so, our results indicate that there was significant biomagnification of Hg within the coastal Louisiana food web, driven by the consumers’ trophic position and their use of differing basal carbon sources. Regional comparisons between Louisiana and Alabama further identified differing concentrations of MeHg and THg at the base of the food web as well as evidence of higher trophic biomagnification of Hg in the coastal Louisiana, relative to Alabama.
Mercury in Louisiana oysters
We found that spatial variation in oyster THg concentration within Barataria Bay, Louisiana was greater than temporal variation in THg prior to (1986–2007) and immediately after (2010) the DWH oil spill. Specifically, oysters collected from Lower Barataria Bay consistently had credibly higher concentrations of THg in their tissues relative to oysters collected from Upper Barataria Bay. Our results agree with Apeti et al. (2012), who prior to the spill in 2006 reported higher Hg concentrations in oyster tissue from Lower Barataria Bay (Middle Bank: THg: 0.047 ppm; MeHg 0.023) relative to Upper Barataria Bay (Bayou Saint Dennis: THg: 0.026 ppm; MeHg 0.011). Surface sediment THg concentrations in 2016 were also higher in Lower Barataria Bay (0.054 ppm) relative to Upper Barataria Bay (0.048 ppm) and MeHg concentrations were low (≤0.005 ppm) in both areas of Barataria Bay (Apeti et al., 2012). Following the spill in January 2011, surface sediment THg concentrations reported across four sites within Barataria Bay (0.043 ± 0.015 ppm; Keevan, 2012) were broadly similar to pre-spill THg concentrations reported by Apeti et al. (2012). These prior studies support the conclusions of our analyses, which found spatial differences in oyster THg within Barataria Bay both before and after the DWH oil spill and no credible difference in THg concentration between oysters collected from 1986 to 2007 and oysters collected in 2010.
Multiple factors had the potential to alter Hg deposition and dynamics in coastal Louisiana following the DWH oil spill. For example, while DWH oil itself had a low Hg concentration (Paris et al., 2012), harmful algal blooms formed from the lack of copepod grazing could have aided evasion of Hg into the atmosphere (Pandey et al., 2009; Walsh et al., 2015). Excessive rain events potentially increased the deposition of atmospheric Hg into the coastal ecosystem where dramatic changes in microbial activity and assemblages were occurring in response to the presence of hydrocarbons and dispersant substrates (Beazley et al., 2012; Scalise, 2013; Joye et al., 2014; Kleindienst et al., 2015; Engel et al., 2017). The increased flux of fresh water and dissolved organic carbon from the river could also have increased the amount of Hg in the coastal system (Aiken et al., 2003; Ravichandran, 2004; Wang et al., 2004; Taylor et al., 2019). Even so, our results indicate that these factors had no measurable effect on the amount of Hg incorporated into a common primary consumer (i.e., oysters) at the base of the coastal Louisiana food web directly after the DWH oil spill, at least at the spatial and temporal scope examined in this study.
The time needed for Hg to move through the food web along with the spatial separation among the site of the oil spill, our sample sites, and the location of river diversion may have also influenced our results. Hg deposited from the DWH oil and/or subsequent environmental conditions was most likely in the inorganic form and had to undergo microbial transformation before becoming bioavailable in the food web (Knightes et al., 2007). In addition, oyster tissues reflect longer-term assimilation of diet and Hg (Cunningham and Tripp, 1973). Thus, a potential temporal lag between Hg deposition, methylation, and tissue assimilation may have contributed to the lack of differences found between oysters collected before and after DWH oil spill. The offshore location (64 km southeast off the Louisiana coast) and depth of the spill (1,525 m) further decrease the likelihood that the spill had any immediate influence on Hg burden in primary consumers in the coastal Louisiana food web (McNutt et al., 2012). In addition, both the upper and lower sampling sites in Barataria Bay may have been too distant from the Davis Pond, LA river diversion to have experienced significant trophic responses to punctuated salinity changes following DWH (Fry and Chumchal, 2012). However, because there was no change in Hg concentrations in oysters at the bottom of the food chain, it is reasonable to assume the Hg burdens in subsequent higher trophic position consumers were not also influenced by DWH, and thus these data may be used as baseline values of Hg for this and future studies. However, it is important to note that pathways for accumulation and exposure in higher trophic predators could have varied if predators had to prey shift as a result of loss of preferred prey following the spill.
Mercury dynamics in the Louisiana coastal food web
We found that both a consumer’s trophic position (i.e., δ15N) and, to a lesser extent, basal carbon source use (i.e., δ13C) were predictors of the tissue Hg concentration across species in the Louisiana coastal food web. With the exception of seabirds, none of the organisms sampled in Louisiana had an Hg value larger than the FDA advisory limit of 1.0 ppm nor the LDEQ limit of 0.500 ppm (Katner et al., 2010), which are consistent with concentrations of Hg found in fish species sampled from the same area by Fry and Chumchal (2012). Blue crabs, which are a commonly consumed species, had similar Hg burdens to blue crabs from the US Atlantic coast (Adams and Engel, 2014). The observed relationship between tissue Hg concentration, δ15N values, and trophic position is not unexpected as multiple past studies have found biomagnification of Hg in upper trophic level aquatic predators which generally consume a larger biomass than that of its prey to sustain metabolic function (Lavoie et al., 2013). Most consumers cannot efficiently eliminate MeHg from tissues, so chronic consumption of contaminated prey (even at low levels) may cause the MeHg to accumulate in consumers and may lead to deleterious health consequences (Liu et al., 2011).
Despite their potential as bioindicators of ecosystem health, few studies have captured the long-term effects of chronic exposure to sub-lethal Hg concentrations in long-lived endotherms such as seabirds along the northern Gulf of Mexico coast because of their complex life histories, spatial movements, and species-specific metabolisms (Monteiro and Furness, 1995; Kim et al., 1996). The Hg burden in brown pelicans from this study was less than those measured in brown pelican tissues in both southeastern California and North Carolina, which were not associated with any severe adverse effects (Ruelas-Inzunza et al., 2009; Newtoff and Emslie, 2017). While there remains a disconnect between how the Hg burden directly relates to the health, and considering the emerging links between atmospheric pollutants and neurodegenerative diseases, monitoring Hg dynamics and toxicological effects should remain a priority (Levesque et al., 2011; Bondy, 2016).
In contrast to δ15N values and trophic position, only a weak negative effect of a δ13C values on MeHg concentrations was observed. Consumer δ13C values in marine systems are often used to infer the importance terrestrial, nearshore/benthic, and offshore/pelagic resource use (France, 1995). For example, in coastal systems C4 marsh grasses and benthic macroalgae generally have lower δ13C values relative to coastal phytoplankton (Peterson et al., 1985; Peterson and Fry, 1987). Our results indicate consumers that assimilate higher proportions of terrestrial and/or benthic carbon sources are more likely to have lower Hg concentrations relative to species consuming water column/pelagic resources. This could be due to higher Hg bioavailability and/or biomagnification in the water column relative to the benthos or marsh platform. Similar conclusions have been drawn in Hg studies in other estuarine and lake systems, where the effect of basal carbon source use was stronger than the one in this study (Chen et al., 2014; Karimi et al., 2016).
In addition to differences in trophic position and basal resource use, the observed variability in Hg concentrations among coastal Louisiana consumers may also correspond with species-specific feeding strategies, residence time in coastal habitats, and tissue-specific differences in Hg. In Louisiana the two mullet species had the lowest MeHg concentrations (0.004 ± 0.003 and 0.001 ± 0.001 μg g−1) relative to the other fishes (Table 3). In addition, the Hg concentrations found in the planktivorous small mullet in this study were lower than those found in past studies of Baritaria Bay (Fry and Chumchal, 2012). Moreover, our results indicate a range of variation in Hg concentrations among organisms with similar basal resource and/or trophic position. For example, δ13C values, δ15N values, and trophic position were generally similar between benthivorous blue crabs and mullet species, however Hg concentration was relatively higher in blue crabs. Evans et al. (2000) observed a similar trend between blue crabs and another benthic consumer, the pink shrimp (Farfantepenaeus duorarum), and concluded that the differentiating factor could be the residence time each consumer spent in contaminated areas. Alternatively, the higher concentrations observed in blue crabs could be due to the use of homogenized whole bodies including the hepatopancreas, which, as the primary organ responsible for elimination, is known to accumulate larger concentrations of Hg relative to other tissues (Adams and Engel, 2014). When compiling prior research that validated the relationships between MeHg and THg we endeavored to obtain data for the specific species and/or taxa we sampled in Louisiana (Table 1). While multiple studies have found strongly positive correlations between tissue MeHg and THg concentrations in these species (e.g., Kannan et al., 1998; Apeti et al., 2012; Adams and Engel, 2014), deviations from these assumed relationships may contribute to additional unexplained variation in the MeHg concentrations calculated for blue crab and other taxa in our study. It is of note that blue crabs sampled in Louisiana from this study had higher Hg burdens relative to those sampled by Adams and Engel (2014) on the Atlantic coast of Florida. While it lies beyond the scope of this study, elevated levels of Hg in blue crabs may have implications for long-term consumption by humans (Lincoln et al., 2011).
Trophic magnification of Hg in coastal Louisiana and Alabama
Based on findings from prior studies on select coastal and estuarine invertebrate and fish species, we expected that Hg would biomagnify up the coastal Louisiana food web to meso- and top predators (Bank et al., 2007; Fry and Chumchal, 2012). However, we did not anticipate the large magnitude of Hg transferred between trophic positions. Other studies that have utilized TMFs as biomagnification metrics generally have slopes closer to 1, even when the system is biomagnifying the contaminant (Bisi et al., 2012). Most of these studies, however, include only ectothermic species or rely solely on δ15N values as a proxy for trophic position and have stronger linear fits (Rumbold et al., 2018; Fonseca et al., 2019). It is possible that these results could be influenced by the assumed trophic enrichment factor (3.4‰) we used in the TP calculations. Previous studies have demonstrated that observed trophic enrichment factors can in some cases be lower than the commonly assumed Post (2002) value (3.4‰) (Kobayashi et al., 2019). In addition, the uncertainty in the fitted linear regression could be a consequence of sampling across a large region with varying environmental conditions created by natural gradients imposed by riverine and marine processes. Regardless, these results provide new insight into the trophic dynamics of Hg in the coastal Louisiana food web with consideration of coastal seabirds. This biomagnification model establishes a baseline that may be used to inform future studies and monitoring efforts.
While both Louisiana and Alabama had TMF values greater than 1, Louisiana had a higher TMF. This result implies that MeHg is potentially biomagnifying at relatively higher concentrations per trophic transfer in the coastal Louisiana food web. On the contrary, Alabama had a higher baseline concentration of MeHg. One possible explanation for this differing MeHg baseline may be that the Alabama sample came from locations within Mobile Bay, which is directly influenced by the Mobile and Tensaw rivers. In contrast, Barataria Bay in Louisiana does not have a direct hydrological connection to the Mississippi River, and it has been suggested that the basin has historically less contamination than other coastal areas in Louisiana (Katner et al., 2010). Even so, Showalter (2010) found significant correlation between log [THg] and δ15N values when considering all species in their study, which agrees with our results indicating Hg biomagnification in the Alabama coastal food web.
Our analyses indicate that variation in species composition and sample sizes have the potential to influence estimates of TMFs as well as comparisons of biomagnification between food webs. For example, while 95% CI overlapped across methods, mean estimates of TMF varied when using our full Louisiana dataset (6.288), Louisiana species also found in Alabama (2.305), and Louisiana species also found in Alabama at standardized sample sizes (4.973). In addition, standardizing both species and sample sizes between Louisiana and Alabama reduced the posterior probability that TMF differed (90%) relative to standardizing only by species (98%). This suggests that sub-setting species and/or sample sizes may be an important approach to consider when seeking to standardize TMF comparisons between food webs. Regardless, it is important to recognize that restricting/reducing species and sample sizes also has the potential to mask meaningful biological differences between food webs. Therefore, we recommend researchers calculate TMFs using multiple approaches (e.g., full datasets vs. standardized datasets) to allow for more comprehensive assessments when seeking to compare biomagnification between food webs.
Conclusion
This research provides an assessment of Hg concentrations and biomagnification through the coastal Louisiana food web prior to and immediately following the 2010 DWH oil spill. We found no evidence to suggest that the DWH event influenced Hg concentrations in oysters, a common indicator species at the base of the coastal Louisiana food web. However, we did observe significant biomagnification of Hg within the coastal Louisiana food web. Specifically, variation in Hg concentration among species was related to trophic position and the use of differing carbon sources. Regional comparisons between Louisiana and Alabama found different baseline concentrations of MeHg and THg at the base of the food web, along with evidence of higher trophic biomagnification of Hg in the coastal Louisiana food web, relative to Alabama. Differences at the regional level underscore the need for development of trophic and contaminant baselines in consumers at multiple trophic levels over space and time in order to adequately evaluate short- and long-term ecological consequences of environmental stressors.
Data availability statement
The datasets presented in this study can be found in online repositories. The names of the repository/repositories and accession number(s) can be found below: https://data.gulfresearchinitiative.org/data/R6.x808.000:0071.
Ethics statement
Ethical review and approval was not required for the animal study because analyses were conducted on vertebrate tissue samples previously collected for other research projects and government monitoring efforts not associated with this research.
Author contributions
KL and MP designed the study; KL, MP, MK, and PL-D collected, collated, and processes samples; KL and RB analyzed samples; KL, SM, and RB analyzed data; KL and MP wrote the manuscript; KL, MP, and SM designed the graphics; MP and PL-D supervised the study; and all authors contributed to previous versions and approved the final manuscript.
Funding
This research was made possible by a grant from The Gulf of Mexico Research Initiative to the Coastal Waters Consortium. The funders had no role in study design, data collection and analysis, decision to publish, or preparation of the manuscript.
Acknowledgments
Thank you to D. Shark, N. Rothman, P. Tuttle, and others from USFWS and NRDA for sample access. Thank you to T. Mauney, M. Brady, V. Chua, D. Dittmann, S. Cardiff, and students from the Fall 2016 semester Biology of Marine Vertebrate course at LSU for assistance with sample preparation and analyses. Thank you to L. Lamb, J. Watkins, and P. Watkins for the unwavering support and love they provided to Katelyn.
Conflict of interest
The authors declare that the research was conducted in the absence of any commercial or financial relationships that could be construed as a potential conflict of interest.
Publisher’s note
All claims expressed in this article are solely those of the authors and do not necessarily represent those of their affiliated organizations, or those of the publisher, the editors and the reviewers. Any product that may be evaluated in this article, or claim that may be made by its manufacturer, is not guaranteed or endorsed by the publisher.
References
Ackerman, J. T., Eagles-Smith, C. A., Herzog, M. P., Hartman, C. A., Peterson, S. H., Evers, D. C., et al. (2016). Avian mercury exposure and toxicological risk across Western North America: A synthesis. Sci. Total Environ. 568, 749–769. doi:10.1016/j.scitotenv.2016.03.071
Adams, D. H., and Engel, M. E. (2014). Mercury, lead, and cadmium in blue crabs, Callinectes sapidus, from the atlantic coast of Florida, USA: A multipredator approach. Ecotoxicol. Environ. Saf. 102, 196–201. doi:10.1016/j.ecoenv.2013.11.029
Aiken, G., Haitzer, M., Ryan, J. N., and Nagy, K. (2003). Interactions between dissolved organic matter and mercury in the Florida Everglades. J. Phys. IV Fr. 107, 29–32. doi:10.1051/jp4:20030235
Apeti, D. A., Lauenstein, G. G., and Evans, D. W. (2012). Recent status of total mercury and methyl mercury in the coastal waters of the northern Gulf of Mexico using oysters and sediments from NOAA's mussel watch program. Mar. Pollut. Bull. 64 (11), 2399–2408. doi:10.1016/j.marpolbul.2012.08.006
Bank, M. S., Chesney, E., Shine, J. P., Maage, A., and Senn, D. B. (2007). Mercury bioaccumulation and trophic transfer in sympatric snapper species from the Gulf of Mexico. Ecol. Appl. 17, 2100–2110. doi:10.1890/06-1422.1
Beazley, M. J., Martinez, R. J., Rajan, S., Powell, J., Piceno, Y. M., Tom, L. M., et al. (2012). Microbial community analysis of a coastal salt marsh affected by the Deepwater Horizon oil spill. PloS one 7, e41305. doi:10.1371/journal.pone.0041305
Bebbington, G. N., Mackay, N. J., Chvojka, R., Williams, R. J., Dunn, A., and Auty, E. H. (1977). Heavy metals, selenium and arsenic in nine species of Australian commercial fish. Mar. Freshw. Res. 28 (3), 277–286. doi:10.1071/mf9770277
Bisi, T. L., Lepoint, G., Azevedo, A., Dorneles, P. R., Flach, L., Das, K., et al. (2012). Trophic relationships and mercury biomagnification in Brazilian tropical coastal food webs. Ecol. Indic. 18, 291–302. doi:10.1016/j.ecolind.2011.11.015
Black, F. J., Poulin, B. A., and Flegal, A. R. (2012). Factors controlling the abiotic photo-degradation of monomethylmercury in surface waters. Geochimica Cosmochimica Acta 84, 492–507. doi:10.1016/j.gca.2012.01.019
Bloom, N. S. (1992). On the chemical form of mercury in edible fish and marine invertebrate tissue. Can. J. Fish. Aquat. Sci. 49, 1010–1017. doi:10.1139/f92-113
Boening, D. W. (2000). Ecological effects, transport, and fate of mercury: A general review. Chemosphere 40, 1335–1351. doi:10.1016/s0045-6535(99)00283-0
Bondy, S. C. (2016). Anthropogenic pollutants may increase the incidence of neurodegenerative disease in an aging population. Toxicology 341-343, 41–46. doi:10.1016/j.tox.2016.01.007
Borgå, K., Kidd, K. A., Muir, D. C. G., Berglund, O., Conder, J. M., Gobas, F. A. P. C., et al. (2012). Trophic magnification factors: Considerations of ecology, ecosystems, and study design. Integr. Environ. Assess. Manag. 8, 64–84.
Cappon, C. J., and Smith, J. C. (1981). Mercury and selenium content and chemical form in fish muscle. Arch. Environ. Contam. Toxicol. 10 (3), 305–319. doi:10.1007/bf01055632
Cardona-Marek, T., Knott, K. K., Meyer, B. E., and O'Hara, T. M. (2009). Mercury concentrations in southern beaufort sea polar bears: Variation based on stable isotopes of carbon and nitrogen. Environ. Toxicol. Chem. 28, 1416–1424. doi:10.1897/08-557.1
Chen, C. Y., Borsuk, M. E., Bugge, D. M., Hollweg, T., Balcom, P. H., Ward, D. M., et al. (2014). Benthic and pelagic pathways of methylmercury bioaccumulation in estuarine food webs of the Northeast United States. PloS one 9. doi:10.1371/journal.pone.0089305
Chumchal, M. M., Rainwater, T. R., Osborn, S. C., Roberts, A. P., Abel, M. T., Cobb, G. P., et al. (2011). Mercury speciation and biomagnification in the food web of Caddo Lake, Texas and Louisiana, USA, a subtropical freshwater ecosystem. Environ. Toxicol. Chem. 30 (5), 1153–1162. doi:10.1002/etc.477
Compeau, G. C., and Bartha, R. (1985). Sulfate-reducing bacteria: Principal methylators of mercury in anoxic estuarine sediment. Appl. Environ. Microbiol. 50, 498–502. doi:10.1128/aem.50.2.498-502.1985
Cunningham, P. A., and Tripp, M. R. (1973). Accumulation and depuration of mercury in the American oyster Crassostrea virginica. Mar. Biol. 20, 14–19. doi:10.1007/bf00387669
Day, J. W., Hopkinson, C. S., and Conner, W. H. (1982). “An analysis of environmental factors regulating community metabolism and fisheries production in a Louisiana estuary,” in Estuarine comparisons. Editor V. S. Kennedy (Gleneden Beach, OR: Academic Press), 121–136. doi:10.1016/b978-0-12-404070-0.50014-4
Delaune, R. D., Gambrell, R. P., Jugsujinda, A., Devai, I., and Hou, A. (2008). Total Hg, methyl Hg and other toxic heavy metals in a northern Gulf of Mexico estuary: Louisiana pontchartrain basin. J. Environ. Sci. Health, Part A 43, 1006–1015. doi:10.1080/10934520802059839
Eagles-Smith, C. A., Ackerman, J. T., Willacker, J. J., Tate, M. T., Lutz, M. A., Fleck, J. A., et al. (2016). Spatial and temporal patterns of mercury concentrations in freshwater fish across the Western United States and Canada. Sci. Total Environ. 568, 1171–1184. doi:10.1016/j.scitotenv.2016.03.229
Engel, A. S., Liu, C., Paterson, A. T., Anderson, L. C., Turner, R. E., and Overton, E. B. (2017). Salt marsh bacterial communities before and after the Deepwater Horizon oil spill. Appl. Environ. Microbiol. 83, e00784. doi:10.1128/aem.00784-17
Evans, D. W., Kathman, R. D., and Walker, W. W. (2000). Trophic accumulation and depuration of mercury by blue crabs (Callinectes sapidus) and pink shrimp (Penaeus duorarum). Mar. Environ. Res. 49, 419–434. doi:10.1016/s0141-1136(99)00083-5
Fonseca, V. F., França, S., Duarte, B., Caçador, I., Cabral, H. N., Mieiro, C. L., et al. (2019). Spatial variation in mercury bioaccumulation and magnification in a temperate estuarine food web. Front. Mar. Sci. 6, 117. doi:10.3389/fmars.2019.00117
France, R. L. (1995). Carbon-13 enrichment in benthic compared to planktonic algae:foodweb implications. Mar. Ecol. Prog. Ser. 124, 307–312. doi:10.3354/meps124307
Fry, B., and Chumchal, M. M. (2012). Mercury bioaccumulation in estuarine food webs. Ecol. Appl. 22 (2), 606–623. doi:10.1890/11-0921.1
Golding, G. R., Kelly, C. A., Sparling, R., Loewen, P. C., Rudd, J. W. M., and Barkay, T. (2002). Evidence for facilitated uptake of Hg(II) by Vibrio anguillarum and Escherichia coli under anaerobic and aerobic conditions. Limnol. Oceanogr. 47, 967–975. doi:10.4319/lo.2002.47.4.0967
Hall, B. D., Bodaly, R. A., Fudge, R. J. P., Rudd, J. W. M., and Rosenberg, D. M. (1997). Food as the dominant pathway of methylmercury uptake by fish. Water, Air, & Soil Pollut. 100, 13–24. doi:10.1023/a:1018071406537
Hallanger, I. G., Warner, N. A., Ruus, A., Evenset, A., Christensen, G., Herzke, D., et al. (2011). Seasonality in contaminant accumulation in Arctic marine pelagic food webs using trophic magnification factor as a measure of bioaccumulation. Environ. Toxicol. Chem. 30, 1026–1035. doi:10.1002/etc.488
Harris, H. H., Pickering, I. J., and George, G. N. (2003). The chemical form of mercury in fish. Science 301, 1203. doi:10.1126/science.1085941
Hastings, D. W., Schwing, P. T., Brooks, G. R., Larson, R. A., Morford, J. L., Roeder, T., et al. (2016). Changes in sediment redox conditions following the BP DWH blowout event. Deep Sea Res. Part II Top. Stud. Oceanogr. 129, 167–178. doi:10.1016/j.dsr2.2014.12.009
Jardine, T. D., Kidd, K. A., and Fisk, A. T. (2006). Applications, considerations, and sources of uncertainty when using stable isotope analysis in ecotoxicology. Environ. Sci. Technol. 40, 7501–7511. doi:10.1021/es061263h
Jonsson, S., Skyllberg, U., Nilsson, M. B., Lundberg, E., Andersson, A., and Björn, E. (2014). Differentiated availability of geochemical mercury pools controls methylmercury levels in estuarine sediment and biota. Nat. Commun. 5, 4624. doi:10.1038/ncomms5624
Joye, S. B., Teske, A. P., and Kostka, J. E. (2014). Microbial dynamics following the Macondo oil well blowout across Gulf of Mexico environments. Bioscience 64, 766–777. doi:10.1093/biosci/biu121
Kannan, K., Jr., R. G., Lee, R. F., Windom, H. L., Heitmuller, P. T., Macauley, J. M., et al. (1998). Distribution of total mercury and methyl mercury in water, sediment, and fish from south Florida estuaries. Archives Environ. Contam. Toxicol. 34 (2), 109–118. doi:10.1007/s002449900294
Karimi, R., Chen, C. Y., and Folt, C. L. (2016). Comparing nearshore benthic and pelagic prey as mercury sources to lake fish: The importance of prey quality and mercury content. Sci. Total Environ. 565, 211–221. doi:10.1016/j.scitotenv.2016.04.162
Katner, A., Sun, M.-H., and Suffet, M. (2010). An evaluation of mercury levels in Louisiana fish: Trends and public health issues. Sci. total Environ. 408, 5707–5714. doi:10.1016/j.scitotenv.2010.08.021
Keevan, J. (2012). Assessing transformation of trace metals and crude oil in Mississippi and Louisiana coastal wetlands in response to the Deepwater Horizon oil spill. Auburn University. M.S. Thesis.
Kidd, K. A., Bootsma, H. A., Hesslein, R. H., Lyle Lockhart, W. L., and Hecky, R. E. (2003). Mercury concentrations in the food web of lake Malawi, east africa. J. Gt. Lakes. Res. 29, 258–266. doi:10.1016/s0380-1330(03)70553-x
Kim, E. Y., Murakami, T., Saeki, K., and Tatsukawa, R. (1996). Mercury levels and its chemical form in tissues and organs of seabirds. Arch. Environ. Contam. Toxicol. 30 (2), 259–266. doi:10.1007/bf00215806
Kleindienst, S., Paul, J. H., and Joye, S. B. (2015). Using dispersants after oil spills: Impacts on the composition and activity of microbial communities. Nat. Rev. Microbiol. 13, 388–396. doi:10.1038/nrmicro3452
Knightes, C. D., Sunderland, E. M., Barber, M. C., Johnston, J. M., and RobertAmbrose, B. J. (2007). Application of ecosystem scale fate and bioaccumulation models to predict fish mercury response times to changes in atmospheric deposition. Environ. Toxicol. Chem. Int. J. 28 (4), 881–893. doi:10.1897/08-242.1
Kobayashi, J., Yoshimoto, M., Yamada, K., Okamura, K., and Sakurai, T. (2019). Comparison of trophic magnification factors of PCBs and PBDEs in Tokyo Bay based on nitrogen isotope ratios in bulk nitrogen and amino acids. Chemosphere 226, 220–228. doi:10.1016/j.chemosphere.2019.03.133
Kongchum, M., Devai, I., DeLaune, R. D., and Jugsujinda, A. (2006). Total mercury and methylmercury in freshwater and salt marsh soils of the Mississippi river deltaic plain. Chemosphere 63, 1300–1303. doi:10.1016/j.chemosphere.2005.09.024
Kujawinski, E. B., Kido Soule, M. C., Valentine, D. L., Boysen, A. K., Longnecker, K., and Redmond, M. C. (2011). Fate of dispersants associated with the Deepwater Horizon oil spill. Environ. Sci. Technol. 45, 1298–1306. doi:10.1021/es103838p
Lavoie, R. A., Jardine, T. D., Chumchal, M. M., Kidd, K. A., and Campbell, L. M. (2013). Biomagnification of mercury in aquatic food webs: A worldwide meta-analysis. Environ. Sci. Technol. 47, 13385–13394. doi:10.1021/es403103t
LDEQ (1993). Project WQ1994001: Mercury contaminant levels in Louisiana biota. Avaiable at: https://waterdata.deq.louisiana.gov/Projects/WQ1994001.
Leaner, J. J., and Mason, R. P. (2001). The effect of thiolate organic compounds on methylmercury accumulation and redistribution in sheepshead minnows,Cyprinodon variegatus. Environ. Toxicol. Chem. 20, 1557–1563. doi:10.1002/etc.5620200720
Levesque, S., Surace, M. J., McDonald, J., and Block, M. L. (2011). Air pollution & the brain: Subchronic diesel exhaust exposure causes neuroinflammation and elevates early markers of neurodegenerative disease. J. Neuroinflammation 8, 105. doi:10.1186/1742-2094-8-105
Lincoln, R. A., Shine, J. P., Chesney, E. J., Vorhees, D. J., Grandjean, P., and Senn, D. B. (2011). Fish consumption and mercury exposure among Louisiana recreational anglers. Environ. Health Perspect. 119, 245–251. doi:10.1289/ehp.1002609
Liu, G., Cai, Y., and O'Driscoll, N. (2011). Environmental chemistry and toxicology of mercury. Hoboken, NJ: John Wiley & Sons.
Martínez, M. L., Feagin, R. A., Yeager, K. M., Day, J., Costanza, R., Harris, J. A., et al. (2012). Artificial modifications of the coast in response to theDeepwater horizonoil spill: Quick solutions or long-term liabilities? Front. Ecol. Environ. 10, 44–49. doi:10.1890/100151
Masset, T., Frossard, V., Perga, M. E., Cottin, N., Piot, C., Cachera, S., et al. (2019). Trophic position and individual feeding habits as drivers of differential PCB bioaccumulation in fish populations. Sci. Total Environ. 674, 472–481. doi:10.1016/j.scitotenv.2019.04.196
McNutt, M. K., Chu, S., Lubchenco, J., Hunter, T., Dreyfus, G., Murawski, S. A., et al. (2012). Applications of science and engineering to quantify and control the Deepwater Horizon oil spill. Proc. Natl. Acad. Sci. U.S.A. 109, 20222–20228. doi:10.1073/pnas.1214389109
Monteiro, L. R., and Furness, R. W. (1995). Seabirds as monitors of mercury in the marine environment. Water Air Soil Pollut. 80, 851–870. doi:10.1007/bf01189736
Newtoff, K. N., and Emslie, S. D. (2017). Mercury exposure and diet in Brown pelicans (Pelecanus occidentalis) in North Carolina, USA. Waterbirds 40, 50–57. doi:10.1675/063.040.0107
Nilson, S. A., Costa, M., and Akagi, H. (2001). Total and methylmercury levels of a coastal human population and of fish from the Brazilian northeast. Environ Sci Pollut Res 8 (4), 280–284. doi:10.1007/bf02987408
O'Connor, B. S., Muller-Karger, F. E., Nero, R. W., Hu, C., and Peebles, E. B. (2016). The role of Mississippi River discharge in offshore phytoplankton blooming in the northeastern Gulf of Mexico during August 2010. Remote Sens. Environ. 173, 133–144. doi:10.1016/j.rse.2015.11.004
O'Connor, T. P. (1998). The NOAA national status and trends program. Mar. Pollut. Bull. 37, 3–5. doi:10.1016/s0025-326x(98)00124-6
Pandey, S. K., Kim, K.-H., Yim, U.-H., Jung, M.-C., and Kang, C.-H. (2009). Airborne mercury pollution from a large oil spill accident on the west coast of Korea. J. Hazard. Mater. 164, 380–384. doi:10.1016/j.jhazmat.2008.07.126
Pardue, J. H., DeLaune, R. D., and Patrick, W. H. (1992). Metal to aluminum correlation in Louisiana coastal wetlands: Identification of elevated metal concentrations. J. Environ. Qual. 21, 539–545. doi:10.2134/jeq1992.00472425002100040003x
Paris, C. B., Hénaff, M. L., Aman, Z. M., Subramaniam, A., Helgers, J., Wang, D.-P., et al. (2012). Evolution of the macondo well blowout: Simulating the effects of the circulation and synthetic dispersants on the subsea oil transport. Environ. Sci. Technol. 46, 13293–13302. doi:10.1021/es303197h
Perrot, V., Landing, W. M., Grubbs, R. D., and Salters, V. J. M. (2019). Mercury bioaccumulation in tilefish from the northeastern Gulf of Mexico 2 years after the Deepwater Horizon oil spill: Insights from Hg, C, N and S stable isotopes. Sci. Total Environ. 666, 828–838. doi:10.1016/j.scitotenv.2019.02.295
Peterson, B. J., and Fry, B. (1987). Stable isotopes in ecosystem studies. Annu. Rev. Ecol. Syst. 18, 293–320. doi:10.1146/annurev.es.18.110187.001453
Peterson, B. J., Howarth, R. W., and Garritt, R. H. (1985). Multiple stable isotopes used to trace the flow of organic matter in estuarine food webs. Science 227, 1361–1363. doi:10.1126/science.227.4692.1361
Pickhardt, P. C., and Fisher, N. S. (2007). Accumulation of inorganic and methylmercury by freshwater phytoplankton in two contrasting water bodies. Environ. Sci. Technol. 41, 125–131. doi:10.1021/es060966w
Pinnegar, J. K., and Polunin, N. V. C. (1999). Differential fractionation of δ 13 C and δ 15 N among fish tissues: Implications for the study of trophic interactions. Funct. Ecol. 13, 225–231. doi:10.1046/j.1365-2435.1999.00301.x
Post, D. M., Layman, C. A., Arrington, D. A., Takimoto, G., Quattrochi, J., and Montaña, C. G. (2007). Getting to the fat of the matter: Models, methods and assumptions for dealing with lipids in stable isotope analyses. Oecologia 152, 179–189. doi:10.1007/s00442-006-0630-x
Post, D. M. (2002). Using stable isotopes to estimate trophic position: Models, methods, and assumptions. Ecology 83, 703–718. doi:10.1890/0012-9658(2002)083[0703:usitet]2.0.co;2
Power, M., Klein, G. M., Guiguer, K. R. R. A., and Kwan, M. K. H. (2002). Mercury accumulation in the fish community of a sub-Arctic lake in relation to trophic position and carbon sources. J. Appl. Ecol. 39 (5), 819–830. doi:10.1046/j.1365-2664.2002.00758.x
Ravichandran, M. (2004). Interactions between mercury and dissolved organic matter--a review. Chemosphere 55, 319–331. doi:10.1016/j.chemosphere.2003.11.011
Ruelas-Inzunza, J., Hernández-Osuna, J., and Páez-Osuna, F. (2009). Organic and total mercury in muscle tissue of five aquatic birds with different feeding habits from the SE Gulf of California, Mexico. Chemosphere 76 (3), 415–418. doi:10.1016/j.chemosphere.2009.03.042
Rumbold, D. G., Lange, T. R., Richard, D., DelPizzo, G., and Hass, N. (2018). Mercury biomagnification through food webs along a salinity gradient down-estuary from a biological hotspot. Estuar. Coast. Shelf Sci. 200, 116–125. doi:10.1016/j.ecss.2017.10.018
Scalise, K. (2013). Atmospheric distributions of polycyclic aromatic hydrocarbons (PAHs) in coastal Northern Gulf of Mexico, USA, associated with the deepwater horizon oil spill. East Carolina University. M.S. Thesis.
Senn, D. B., Chesney, E. J., Blum, J. D., Bank, M. S., Maage, A., and Shine, J. P. (2010). Stable isotope (N, C, Hg) study of methylmercury sources and trophic transfer in the northern Gulf of Mexico. Environ. Sci. Technol. 44 (5), 1630–1637. doi:10.1021/es902361j
Showalter, L. M. (2010). Mercury bioaccumulation in the biota of mobile Bay. Alabama: University of Alabama. M.S. Thesis.
Steffy, D. A., Nichols, A. C., Morgan, L. J., and Gibbs, R. (2013). Evidence that the Deepwater Horizon oil spill caused a change in the nickel, chromium, and lead average seasonal concentrations occurring in sea bottom sediment collected from the eastern Gulf of Mexico continental shelf between the years 2009 and 2011. Water, Air, & Soil Pollut. 224 (11), 1–11. doi:10.1007/s11270-013-1756-1
Taylor, V. F., Buckman, K. L., Seelen, E. A., Mazrui, N. M., Balcom, P. H., Mason, R. P., et al. (2019). Organic carbon content drives methylmercury levels in the water column and in estuarine food webs across latitudes in the Northeast United States. Environ. Pollut. 246, 639–649. doi:10.1016/j.envpol.2018.12.064
US EPA (2007). Method 7473: Mercury in solids and solutions by thermal decomposition, amalgamation, and atomic absorption spectrophotometry. Available at: https://www.epa.gov/sites/production/files/2015-07/documents/epa-7473.pdf.
Walsh, J. J., Lenes, J. M., Darrow, B. P., Parks, A. A., Weisberg, R. H., Zheng, L., et al. (2015). A simulation analysis of the plankton fate of the Deepwater Horizon oil spills. Cont. Shelf Res. 107, 50–68. doi:10.1016/j.csr.2015.07.002
Wang, X.-C., Chen, R. F., and Gardner, G. B. (2004). Sources and transport of dissolved and particulate organic carbon in the Mississippi River estuary and adjacent coastal waters of the northern Gulf of Mexico. Mar. Chem. 89, 241–256. doi:10.1016/j.marchem.2004.02.014
Wiener, J. G., and Spry, D. J. (1996). “Toxicological significance of mercury in freshwater fish,” in Environmental contaminants in wildlife: Interpreting tissue concentrations. Editors W. N. Beyer, G. H. Heinz, and A. W. Redmon (Boca Raton, Florida, USA: CRC), 297–339.
Wilhelm, S. M., Liang, L., Cussen, D., and Kirchgessner, D. A. (2007). Mercury in crude oil processed in the United States (2004). Environ. Sci. Technol. 41 (13), 4509–4514. doi:10.1021/es062742j
Keywords: methylmercury, Hg, stable isotope analysis, trophic magnification factor, trophodynamics
Citation: Lamb KJ, Midway SR, Brasso RL, López-Duarte PC, Kimball ME and Polito MJ (2022) Mercury biomagnification in a coastal Louisiana food web following the 2010 Deepwater Horizon oil spill. Front. Environ. Sci. 10:937124. doi: 10.3389/fenvs.2022.937124
Received: 05 May 2022; Accepted: 01 August 2022;
Published: 24 August 2022.
Edited by:
Shaoda Liu, Beijing Normal University, ChinaReviewed by:
Jianfeng Feng, Nankai University, ChinaFei Dang, Institute of Soil Science (CAS), China
Copyright © 2022 Lamb, Midway, Brasso, López-Duarte, Kimball and Polito. This is an open-access article distributed under the terms of the Creative Commons Attribution License (CC BY). The use, distribution or reproduction in other forums is permitted, provided the original author(s) and the copyright owner(s) are credited and that the original publication in this journal is cited, in accordance with accepted academic practice. No use, distribution or reproduction is permitted which does not comply with these terms.
*Correspondence: Michael J. Polito, bXBvbGl0b0Bsc3UuZWR1