- 1School of Environment and Sustainability, University of Saskatchewan, Saskatoon, SK, Canada
- 2Global Institute for Water Security, University of Saskatchewan, Saskatoon, SK, Canada
- 3Agriculture and Agri-Food Canada, Swift Current Research and Development Centre, Swift Current, SK, Canada
Understanding sediment phosphorus (P) compounds is essential to managing P in lake sediments because P speciation will determine bioavailability and reactivity. Little is known about organic P (Po) in hardwater eutrophic lakes in the North American Great Plains, or the role of metals in Po cycling. Sediment cores (0–12 cm deep) collected from four lakes from the Qu’Appelle chain in Saskatchewan, Canada, were sectioned by depth and analyzed by solution P nuclear magnetic resonance spectroscopy to characterize P forms. Concentrations and pools of calcium (Ca), magnesium (Mg), iron (Fe), manganese (Mn), and aluminum (Al) were also determined. A range of P compounds was detected with significant interactions between lakes and depth for orthophosphate, phytate and DNA, and significant differences among lakes or with depth for polyphosphates and phosphonates. The main class of Po compounds identified in all lakes was orthophosphate diesters, including phospholipids and DNA, typical of living biota, suggesting that P immobilized by microbes and algae is an important pool in the sediments of these lakes. There were significant differences in metal concentrations among the lakes. In three lakes, Ca concentrations were high, and P was tightly bound with Ca compounds of low solubility. In the fourth lake with lower Ca concentrations, P appeared to be loosely bound to Al and Fe compounds. Our study indicates that there were significant differences in P compounds and the factors controlling their cycling among these four lakes in the same chain, which has implications for P management and water quality control.
Introduction
Phosphorus (P) is an essential nutrient to organisms for energy and growth. As a widely used fertilizer, P plays a crucial role in global food security (Jarvie et al., 2015). In freshwater ecosystems, P enrichment from external loads can degrade water quality by causing cultural eutrophication and triggering harmful algal blooms (Schindler, 2012). Anthropogenic activities can enhance external loads; this can contribute to a build-up of legacy P in lakes (Carey and Migliaccio, 2009), which in turn can be biogeochemically recycled via internal sediment P loading. Phosphorus in lakes can be operationally characterized into particulate and dissolved pools based on retention on filters, and chemically defined as inorganic and organic P. Inorganic P (Pi) forms include phosphates (H2PO4− or HPO42−, at environmentally relevant pH), pyrophosphate (two phosphates linked by anhydride bonds) and polyphosphates (three or more linked phosphates). Organic P (Po) compounds contain P groups linked to C groups, and are grouped by bonding type into orthophosphate monoesters (ROPO32−; e.g., phytate, a storage compound in terrestrial plants), orthophosphate diesters [R1O(RO)PO2−, which are components of living cells, e.g., deoxyribonucleic acid (DNA), phospholipids], and phosphonates [RP(O)(OH)2, with a direct C-P bond, e.g., ciliatine in cilia of protozoa or the herbicide glyphosate; Condron et al., 2005].
Lake sediments are deposited from allochthonous, autochthonous, and decaying organic matter (OM) particulates in the overlying water, and undergo diagenetic changes that control P distribution and cycling, creating a complex matrix of organic and inorganic materials with varied P compounds reflective of point and non-point sources and sediment chemistry (Carpenter et al., 1998; Correll, 1998). Phosphorus forms vary in chemical characterization, which affects their retention or release within sediments and their bioavailability. Phosphorus retention processes for both Pi and Po compounds include sedimentation, direct uptake and biological assimilation, sorption, and precipitation (Boström et al., 1988; Reddy and Delaune, 2008a), which are important sediment P control mechanisms. However, there is considerable scientific interest in sediment P mobilization processes due to their essential roles in sediment nutrient budgets and influence on lake trophic status and recovery from eutrophication. The P-control mechanism of the sediment iron (Fe III)-P complex is a significant factor in many lakes (Mortimer, 1941), but Fe is not the only metal cation controlling phosphate release at the sediment-water interface (SWI) in lakes. Phosphorus compounds can react with dissolved compounds in sediments, including (hydr)oxides of iron, manganese (Mn) and aluminum (Al), and carbonates of calcium (Ca) and magnesium (Mg; Mortimer, 1941; Hupfer and Lewandowski, 2008; Reddy and Delaune, 2008a) and can be incorporated into OM or bridged to OM by metals (Williams et al., 1976; Søndergaard et al., 2001). The precipitation or adsorption of P forms to Al, Fe or Mn tends to form unstable amorphous compounds, which can stabilize to crystalline or organically-complexed compounds (Reddy and Delaune, 2008a). In calcareous sediments, P compounds adsorb and precipitate with Ca carbonates (CaCO3) and Mg-calcite, which are affected by changes in temperature and pH (Boström et al., 1988). In lakes with high Fe and Mn concentrations, sediment P cycling tends to be controlled by oxidation-reduction reactions (Nürnberg, 1988). In all lakes, P cycling is strongly influenced by microbial activities, temperature, and pH (Otsuki and Wetzel, 1972; Reddy and Delaune, 2008b; Hamilton et al., 2009; Read et al., 2014).
Most studies measuring P in lakes have used simple colorimetric analyses of total P (TP) and soluble reactive Pi or operationally-defined P pools, but have not examined the distribution and cycling of chemically-defined Pi and Po species in sediments (Condron and Newman, 2011). Detailed information on sediment P composition can be gained from advanced analytical spectroscopic techniques, such as solution 31P nuclear magnetic resonance (P-NMR) spectroscopy, which has been used to characterize P in lake sediments in Europe (Hupfer et al., 2004; Ahlgren et al., 2005; Paraskova et al., 2015), Asia (Bai et al., 2009; Dong et al., 2012; Shinohara et al., 2012), Australia (Baldwin, 1996), the United States (Amirbahman et al., 2013; Giles et al., 2015), and Eastern Canada (Audette et al., 2018; O’Connell et al., 2020). However, there have been no P-NMR studies of lake sediments in the Northern Great Plains to date. The Northern Great Plains region in the United States and Canadian Prairie Ecozone has relatively flat grasslands with a large agricultural land base, important for global food security (Tollerud et al., 2018; Baulch et al., 2019). In the Canadian Prairies, lakes are alkaline (Hammer, 1986), and several lakes show historical evidence of eutrophication through nutrient loading (Barica, 1993; Quinlan, 2000; Patoine and Leavitt, 2006). High internal sediment P loading can occur in sediments of reservoirs (North et al., 2015; D’Silva, 2017; Doig et al., 2017) and lakes (Allan and Roy, 1980; Shaw and Prepas, 1990; Orihel et al., 2017) of the region.
The unique conditions on the Canadian Prairies, including shallow, often eutrophic lakes, large predominantly agricultural watersheds, and potentially high rates of internal P loading create a critical situation for water quality in this region (Schindler and Donahue, 2006; Orihel et al., 2017). Within the Qu’Appelle River Watershed, the lakes are P-rich, dissolved reactive P concentrations are high (0.38–3.85 mg L−1) and increase in downstream lakes (Hammer, 1971). These high P concentrations affect algal productivity (Vogt et al., 2015), contributing to recurrent blooms. Sediment P concentrations in these lakes are comparable to other Canadian Prairie lakes, and can be high to depths of 15 cm (Allan and Williams, 1978). Phosphorus forms associated with redox-sensitive metal cations in lake sediments within the watershed constitute a significant P fraction that can release phosphate under changing redox conditions and increased duration of thermal stratification (Allan and Williams, 1978; Doig et al., 2017). However, our current knowledge is limited regarding composition, bioavailability, and reactivity of P species in lake sediments at different depths, and the broader role of sediment metals on P cycling in hardwater lakes in the Northern Great Plains. Increased knowledge of sediment P forms will improve understanding of internal P loading, to better manage P in lake sediments (Shinohara et al., 2012; Giles et al., 2015), especially in eutrophic bloom-affected lakes. This study addresses the research questions: What are the major forms of P present in eutrophic, hardwater lakes in the prairies? Are there differences in sediment P biogeochemistry across lakes? And, what factors are associated with these differences? Specifically, our objectives were to characterize and quantify P forms at different depths in sediments from several prairie lakes located within the Qu’Appelle River watershed using P-NMR, and to examine geochemical factors influencing these P forms. Lakes along the Qu’Appelle chain differ in their inputs (Saskatchewan Water Security Agency, 2018), morphometry, and ecology (Hammer, 1971; Hall et al., 1999), hence we hypothesized that the lakes would show 1) significant differences in sediment P species and sediment metal cation concentrations; and 2) sediment P species and associated metal cation concentrations will vary with depth, based on diagenetic processes.
Materials and methods
Study area
The Canadian Prairies have semi-arid conditions in the south and west with frigid winters and warm summers with low precipitation coupled with high evaporation (Hammer, 1971; Sauchyn et al., 2002). Snowmelt is the primary source of runoff sustaining most streams, lakes, and wetlands in this region. Drought can lead to cessation of surface runoff while snowmelt and spring rain occasionally cause extreme flood events (Sauchyn et al., 2002; Pomeroy et al., 2005; Wheater and Gober, 2013). The Qu’Appelle River Watershed in southern Saskatchewan, Canada, drains more than 53,000 km2 of semi-arid agricultural land, and contains reservoirs, and lakes (McGowan et al., 2005) providing important ecosystem services including hydroelectricity, recreation and drinking water (Hall et al., 1999). The Qu’Appelle lakes are a chain of polymictic, hardwater, and naturally eutrophic prairie lakes in the lower Qu’Appelle River Watershed that range in depth (maximum depth 4.6–21.9 m) with short water residence times (0.05–0.7 years; Rawson and Moore, 1944; Hammer, 1971; Barica, 1993; Supplementary Table S1).
Four lakes from the Qu’Appelle lake chain were selected for this research (Figure 1; Supplementary Table S1). The first, Wascana Lake, is situated in the middle of the city of Regina, upstream of the Regional Regina Wastewater Treatment Plant (RRWTP). It was formed in the 1880s by damming an agriculturally-drained tributary, Wascana Creek. The lake was drained, dredged, and deepened in 1931 and 2003–2004 (Riddell, 1992; Hughes, 2004). Wascana Lake receives water flow directly from Wascana Creek and urban storm runoff from Regina, including road construction and residential development (Riddell, 1992). Pasqua Lake, Echo Lake, and Crooked Lake are in a series connected by the Qu’Appelle River and flow into each other. Pasqua Lake is the first, Echo Lake is second, connected to Pasqua Lake by a narrow, shallow channel, and Crooked Lake is further downstream and receives water from other lakes not included in this study. These three lakes are downstream of the RRWTP. Control structures along the Qu’Appelle River moderate water flow downstream so that Crooked Lake may receive less flow than the other lakes of this study (Pomeroy et al., 2005).
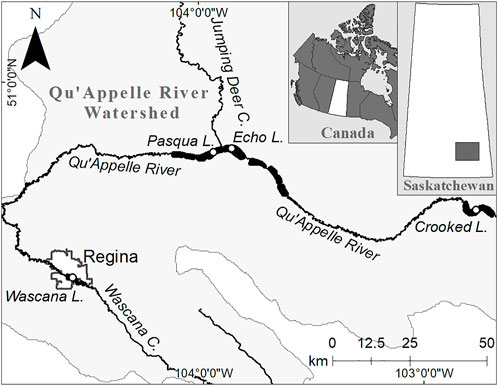
FIGURE 1. The four study lakes in the Lower Qu’Appelle River Watershed; insets show (i) Saskatchewan, Canada, and (ii) the Qu’Appelle River Watershed in southern Saskatchewan. C, Creek; L, Lake. Map source: Government of Canada open data at open.canada.ca.
Sample collection and processing
Vertical lake profile measurements, including oxygen, temperature, pH, and specific conductance, were measured using YSI 556 Multi-Probe System (YSI Environmental, OH) to assess the ambient water conditions when samples were collected. Dissolved oxygen concentrations indicated that the lakes were well-oxygenated from the water surface to 8 m, the conductivity levels were homogenous, and the pH for the water column (8.39–8.74) was alkaline (Supplementary Table S2). Undisturbed sediment cores were collected within a 48-h period in September 2018 using a Glew gravity corer, John Glew, Kingston ON, Canada (Glew et al., 2001). Four replicate sediment cores were collected from two to four random sub-sites within 5 m of the deepest spots in each lake. Two sediment cores were designated as main lake cores, and the other two as duplicate lake cores. All samples were placed on ice and held upright during transport to the laboratory, where they were stored and refrigerated at 4°C in the dark until processing. Sediment cores were sectioned in 1-cm intervals for the first 5 cm of each core, then one section each for the 5–10-cm and 10–12-cm increments. After sectioning, identical depths from each set of cores were combined to give duplicate samples for each depth for each lake. Sediments were then frozen, lyophilized using a Freezone Freeze-Dryer (Labconco, MO) and stored in the dark until analysis.
Geochemical analyses
A subset of sediment samples was submitted to the Analytical Chemistry Services Laboratory (ACS Lab) in Victoria, BC, for geochemical analyses. One 5–10-cm sample (from all but Pasqua Lake, where a 0–1-cm sample was used), was analyzed for particle size using the hydrometer method (Kroetsch and Wang, 2008) to determine percentages of silt, clay, and sand, and for pH with a 1:1 sediment: water ratio (Hendershot et al., 2008). Also at the ACS Lab, samples from all depths of one core per lake were analyzed for 1) total concentrations of major metal cations and P by Vaporized High Pressure (VHP) closed vessel microwave acid digestion followed by inductively coupled plasma mass spectrometry (ICP-MS; EPA 3051A; Agilent 7900 ICP-MS); and 2) extractable Al, Mn and Fe concentrations in separate extractions using sodium pyrophosphate (SP), acid ammonium-oxalate (AAO), and dithionite-citrate (DC), followed by inductively coupled plasma optical emission spectrometry analysis (Courchesne and Turmel, 2008; Teledyne Leeman Prodigy ICP-OES). Metal cations extracted with SP were considered to be organically-complexed (o-Al, o-Fe, o-Mn), the difference between AAO and SP concentrations was considered to represent amorphous metals (a-Al, a-Fe, a-Mn), and the difference between DC and AAO concentrations was considered to represent crystalline metals (c-Fe, c-Mn; Reddy and Delaune, 2008a; Jan et al., 2013). The concentrations of Fe, Mn, and Al in each extracted metal pool were converted to percentages of the total concentrations for each metal cation. At the Swift Current Research and Development Centre (SCRDC), samples from all depths for both cores from all lakes were analyzed for total Po (TPo) concentrations by the ignition method (Saunders and Williams, 1955; O'Halloran and Cade-Menun, 2008), calculated as the difference in P concentrations of extracts of ignited and unignited samples after colorimetric analysis (Murphy and Riley, 1962). Samples were weighed before and after ignition, before extraction, for loss on ignition to estimate OM (%), while the total P concentrations were estimated (eTP) by extraction and colorimetric analysis of ignited samples. Linear regression showed a positive and significant correlation (p < 0.001, R2 = 0.87, y = 0.69x + 0.32) between the TP concentrations determined by digestion and the eTP concentrations determined by ignition; thus, only eTP concentrations are reported.
Solution phosphorus-31 nuclear magnetic resonance spectroscopy
At the SCRDC, a revised version of the Cade-Menun and Preston (1996) protocol was used to extract samples from all depths in both cores for all lakes for P-NMR analysis. The extractant solution was 0.5 M NaOH plus 0.1 M Na2EDTA (NaOH-EDTA) with an extractant: sediment (dry weight) ratio of 25 ml: 2.5 g, extracted for 5 h at 20°C and 180 rpm in the dark, followed by centrifugation (20 min at 10,000 × g). A 1-ml aliquot of each supernatant, diluted to 10 ml with water, was analyzed by ICP-OES (Thermo Scientific ICAP 6300 Duo) for NaOH-EDTA-extracted total P (TPNE), Fe, and Mn concentrations. The TPNE concentration was compared to the eTP concentration to determine the percentage of recovered P (Recovered TP). In the remaining supernatants, pH was adjusted to near neutral with 6 M HCl (Cade-Menun et al., 2006), and then they were frozen and lyophilized. Lyophilized extracts were re-dissolved using D2O (0.6 ml), water (0.6 ml), 10 M NaOH (0.8 ml) and the NaOH-EDTA extraction solution (0.8 ml), vortex-mixed (5 min; Johns Scientific Thermolyne Corp. United States), centrifuged (20 min at 3,260 × g; Thermo Scientific Sorval ST8, United States) and transferred to 10-mm NMR tubes. After preparation, samples were stored in a refrigerator for no more than 24 h before analysis with a Bruker Avance III HD 500 MHz spectrometer equipped with a 10-mm broadband observe probe. Experimental parameters were: 202.4 MHz; 45° pulse angle; 20°C; 0.4 s acquisition time; no proton decoupling; and 1,526–4,164 scans. Recycle delay times were determined as 4 times T1, with T1 values estimated for each sample from the P: (Fe + Mn) ratio in each extract (McDowell et al., 2006; Cade-Menun and Liu, 2014) and ranged from 3 to 10 s. Spiking experiments to confirm peak identifications for myo-inositol hexakisphosphate (myo-IHP, phytate), DNA, pyrophosphate, α- and β-glycerophosphate, and adenosine monophosphate (AMP) were conducted after initial P-NMR analysis (Cade-Menun, 2015).
Spectra were processed with Acorn NMR Utility Transform Software (NUTS), using 7 Hz line broadening for the whole spectrum and 2 Hz line-broadening for specific regions. Chemical shifts were determined relative to an 85% phosphoric acid standard, and the orthophosphate peak in each spectrum was standardized to 6 ppm during processing. Peaks were identified from the literature (Cade-Menun, 2015) and confirmed from spiking experiments. The calculations for total orthophosphate monoesters and total orthophosphate diesters were corrected by subtracting the percentages of orthophosphate diester degradation products (α-glycerophosphate, β-glycerophosphate and mononucleotides) from the orthophosphate monoester region and adding these percentages to the orthophosphate diester region per spectrum (Schneider et al., 2016). Peak area percentages were multiplied by TPNE concentrations for each sample to convert to mg P kg−1 sediment. Examples of P-NMR spectra are shown in Supplementary Figures S2–S4 and P compound chemical shifts are shown in Supplementary Table S4.
Statistical analyses
Geochemical data were tested for normality with the Shapiro Wilks test and were log-transformed as needed. The P-NMR data were normalized by centred-log ratio transformation (Abdi et al., 2015). All statistical analyses were completed using R statistical software (R Core Team, 2020), with a significance level of α < 0.05. Two-way Analysis of Variance (ANOVA) tests were conducted to evaluate relationships between depths and lakes (Zar, 1999). Tukey HSD post-hoc tests were performed when the ANOVA results were statistically significant. Kruskal-Wallis tests followed by a pairwise Wilcoxon test with Bonferroni correction were conducted for data sets that did not normalize after log-transformations (e.g., OM, TPo). Pearson correlation analyses with Bonferroni correction were used to evaluate relationships among the P compounds determined by P-NMR, sediment metal cations, and other geochemical data.
Results
Sediment texture, pH, organic matter, total organic phosphorus and estimated total phosphorus
Sediment pH was 7.53–7.93 (Supplementary Table S1). Wascana Lake sediments were higher in clay than the other lakes, while Pasqua and Echo Lake sediments had more silt and sand than Wascana and Crooked Lake sediments (Supplementary Table S1). For OM, eTP and TPo concentrations, the depth × lake interaction in two-way ANOVAs was not significant, but there were highly significant differences (p < 0.001) among lakes (Supplementary Table S3). Mean OM, eTP, and TPo concentrations were significantly higher in Echo Lake and Crooked Lake sediments than in Pasqua Lake and Wascana Lake (Table 1). There were also significant differences (p < 0.05) in TPo concentrations with sediment depth, which were significantly higher at 0–1-cm than at 5–10-cm (Figure 2), using Kruskal-Wallis tests. There were highly significant differences (p < 0.001) among lakes for concentrations of TPNE and Recovered TP (Supplementary Table S3) using Kruskal-Wallis tests. Mean TPNE concentrations were significantly lower in Pasqua Lake sediments than the other lakes, while the greatest proportion of Recovered TP was from Wascana Lake sediments, and the lowest was from Echo Lake sediments (Table 1).
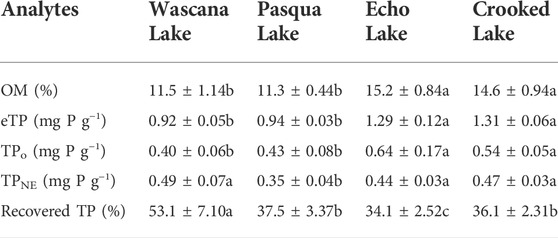
TABLE 1. Organic matter (OM), estimated concentrations per dry weight of total P (eTP), total organic P (TPo), NaOH-EDTA-extracted TP (TPNE) and the proportion of eTP recovered in TPNE extracts (Recovered TP) of sediments from the sampled Qu’Appelle lakes, Saskatchewan, Canada. Values are the concentration means (±SD); n = 14 (7 depths, duplicate sediment core samples that were evenly mixed per depth). Different letters indicate significant differences (p < 0.05) in concentrations of the nutrients, determined using Kruskal-Wallis tests followed by pairwise Wilcoxon tests with Bonferroni correction.
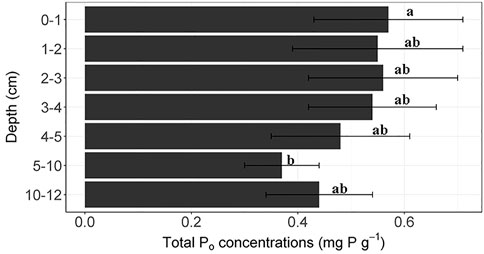
FIGURE 2. Total organic P (TPo) concentrations (mg P g−1 dry weight) in sediments by depth. Values are means (±SD); n = 8 (4 lakes, duplicate cores). Different letters indicate significant differences (p < 0.05) in the Po concentrations among the depths and lakes, determined by Kruskal-Wallis tests followed by pairwise Wilcoxon tests with Bonferroni correction.
Sediment metals
Sediment total Ca concentrations were generally highest in Echo Lake (110–120 mg g−1) and lowest in Wascana Lake (22.0–26.0 mg g−1) and generally increased with sediment depth (Figure 3). Sediment total Mg concentrations (11.0–14.0 mg g−1) were generally constant across depths among the Qu’Appelle lakes with 1 mg g−1 fluctuations for Echo and Crooked Lakes (Figure 3). Total Al concentrations generally increased with depth for all lakes (Figure 3), with the highest concentrations in Wascana Lake (44.0–48.0 mg g−1), followed by Crooked Lake (27.0–28.0 mg g−1), then Pasqua Lake (18.0–21.0 mg g−1), and Echo Lake (13.0–18.0 mg g−1). Approximately 2.4%–6.9% of the total sediment Al extracted from the sediments of the Qu’Appelle lakes was o-Al (Figure 4), with no detected a-Al. Wascana Lake sediments had the greatest concentrations of extracted o-Al of the four studied lakes, and the concentrations increased with depth from 5.1% at 4–5-cm to 6.9% at 10–12-cm.
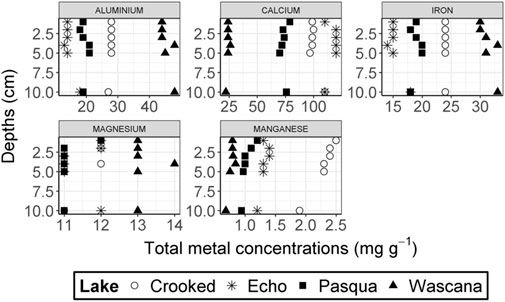
FIGURE 3. Concentrations of total sediment metal cations (mg g−1) plotted against depth from the sampled Qu’Appelle lakes, Saskatchewan, Canada; n = 6 sediment samples per lake (no duplicates were analyzed). Each point corresponds to 0–1-cm, 1–2-cm, 2–3-cm, 3–4-cm, 4–5-cm, and 10–12-cm depth increments.
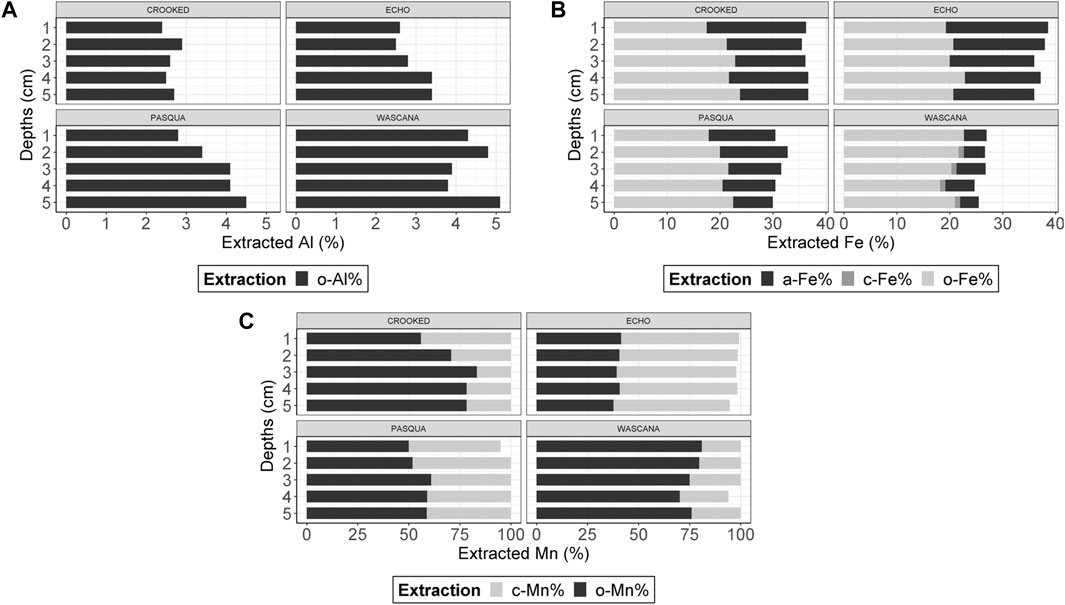
FIGURE 4. Extracted metal cation phase concentrations of (A) organically-complexed aluminum (o-Al) as a percentage of the total Al; (B) organically-complexed iron (o-Fe), amorphous Fe (a-Fe) and crystalline Fe (c-Fe) as percentages of the total Fe; and (C) organically-complexed manganese (o-Mn) and crystalline Mn (c-Mn) as percentages of the total Mn in sediments by depth (ranging from 0–5-cm); n = 5 depth samples per lake, (no duplicates were analyzed).
Total Fe concentrations in sediments generally increased with depth (Figure 3), and the highest concentrations were observed in Wascana Lake (30–33 mg g−1) followed by Crooked Lake (with constant concentrations of 24 mg g−1 from 0–1-cm to 10–12-cm), then Pasqua Lake (18–20 mg g−1) and Echo Lake (14–18 mg g−1). Approximately 15.0%–23.8% of the total Fe extracted was o-Fe, which increased with depth (Figure 4), a-Fe was a smaller proportion of total Fe (3.5%–19.3%) and decreased with depth, and c-Fe was the smallest proportion of total Fe (0.3%–0.6%). Percentages of a-Fe were similar in Crooked Lake (12.9–18.8%) and Echo Lake (10.0%–19.3%) and lower in Wascana Lake (3.5%–5.5%). However, Wascana Lake had more o-Fe (18.2%–22.7%) and was the only lake with c-Fe from 1–2-cm to 10–12-cm depths. Sediment total Mn concentrations were highest in Crooked Lake (1.90–2.50 mg g−1; Figure 3), similar in Echo Lake and Pasqua Lake (1.20–1.40 mg g−1 and 0.94–1.20 mg g−1), and lowest in Wascana Lake (0.68–0.84 mg g−1), and were generally higher at the sediment surface. Of the total Mn, o-Mn was 37.7%–83.3%, c-Mn was 16.7%–58.6%, and a-Mn was not detected (Figure 4). The largest percentage of c-Mn (58.6%) and the lowest percentage of o-Mn (39.3%) were observed in Echo Lake (Figure 4).
Solution phosphorus-31 nuclear magnetic resonance analysis
The P-NMR results for Pi compounds will be presented first, followed by those for Po compounds.
Orthophosphate, pyrophosphate, and polyphosphates
There was a significant lakes × depth interaction in two-way ANOVAs for orthophosphate, significant differences with both lakes and depths but no interactions for total polyphosphates and pyrophosphate, and significant differences with depth for polyphosphate (Supplementary Table S5). Orthophosphate concentrations at all depths in Crooked and Wascana Lake sediments were significantly (p < 0.05) higher than in the other lakes, and were significantly lower at 0–1-cm and 2–3-cm in Echo and Pasqua Lake sediments than all other depths among the four lakes (Table 2). Recovered TP was 34.1 (±2.52) to 53.1 (±7.10) % of eTP (Table 1). Sediment P not extracted with NaOH-EDTA is likely insoluble mineral-bound orthophosphate (Chen et al., 2021), which accounted for 46.9%–65.9% of sediment P. Pyrophosphate concentrations in Wascana Lake sediments were significantly (p < 0.01) higher than those in Crooked Lake (Table 3), and pyrophosphate (p < 0.01) and polyphosphate (p < 0.001) concentrations at 0–1-cm and 1–2-cm were significantly higher than those at 10–12-cm (Table 4). Total polyphosphate concentrations (pyrophosphate and polyphosphates combined) were significantly (p < 0.001) lower in Crooked Lake sediments compared to the other lakes (Figure 5), and concentrations at 0–1-cm were significantly higher (p < 0.001) than at 5–10-cm and 10–12-cm (Table 4).
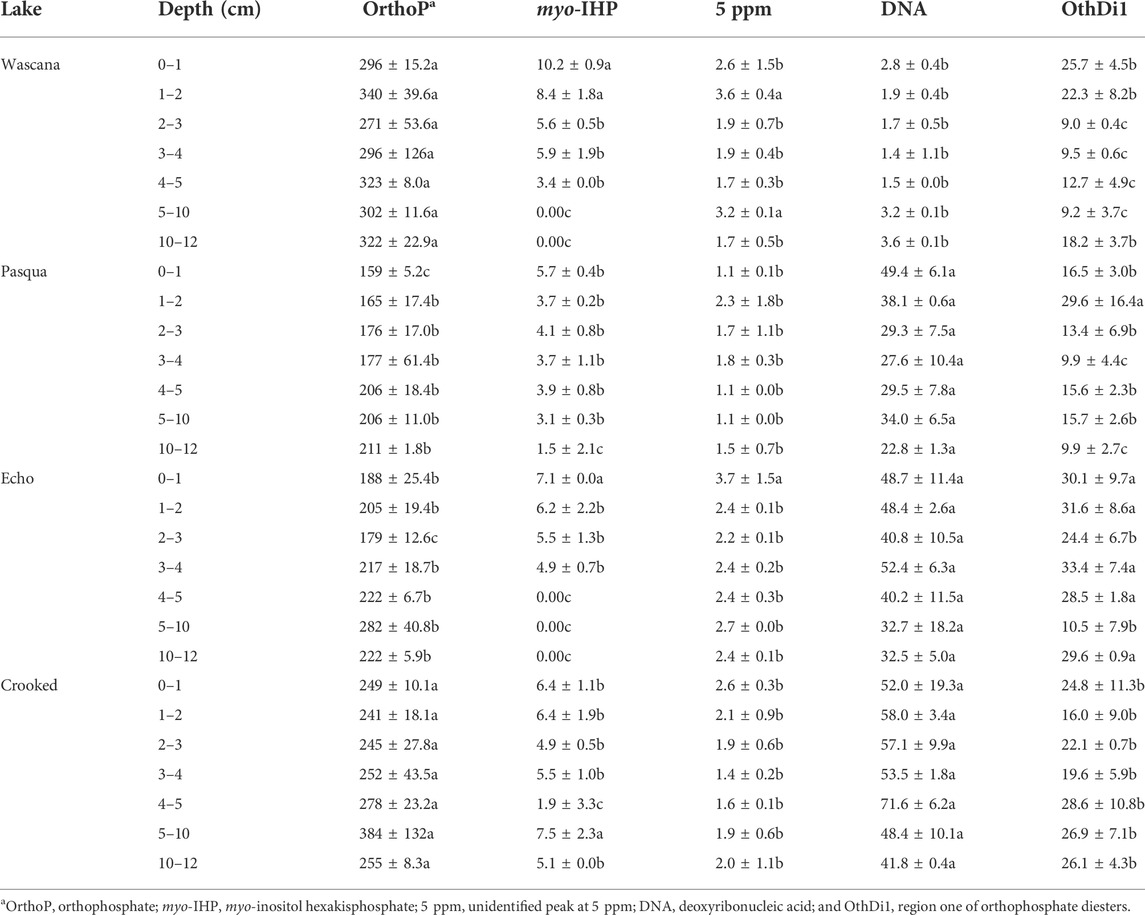
TABLE 2. Concentrations (mg P kg−1 dry weight) of orthophosphate and organic P compounds in NaOH-EDTA extracts of sediments analyzed by P-NMR spectroscopy. Values are means (±SD); n = 2 (duplicate cores) per depth. Different letters indicate significant (p < 0.05) differences in concentrations among depths and lakes for each P species, determined by two-way ANOVAs followed by Tukey HSD post-hoc tests using centred-log ratio data.

TABLE 3. Concentrations (mg P kg−1 dry weight) of sediment P species in NaOH-EDTA extracts of sediments analyzed by P-NMR spectroscopy. Values are means (±SD); n = 14 depths (7 depths, duplicate cores per lake). Different letters indicate significant (p < 0.05) differences in concentrations of P species among lakes, determined by two-way ANOVAs followed by Tukey HSD post-hoc tests using centred-log ratio data.
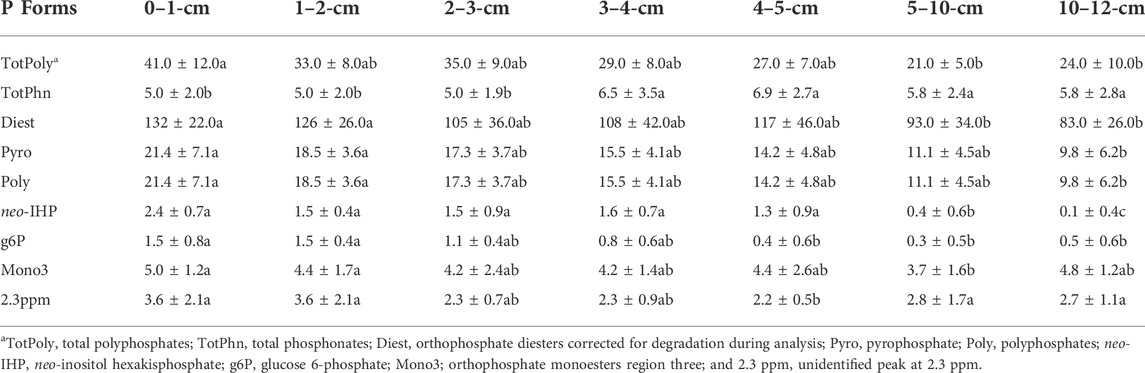
TABLE 4. Concentrations (mg P kg−1 dry weight) of sediment P compound classes and P species in NaOH-EDTA extracts of sediments analyzed by P-NMR spectroscopy. Values are means (±SD); n = 8 (4 lakes, duplicate cores). Different letters indicate significant (p < 0.05) differences in concentrations of P compound class and P species among depths, determined by two-way ANOVAs followed by Tukey HSD post-hoc tests using centred-log ratio data.
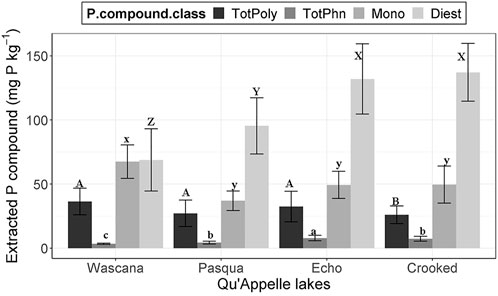
FIGURE 5. Concentrations of P compound classes (mg P kg−1 dry weight) in NaOH-EDTA extracts of sediments analyzed by P-NMR spectroscopy. Values are means (±SD); n = 14 (7 depths, duplicate cores). The bar plots show TotPoly, total polyphosphates; TotPhn, total phosphonates; Mono, total orthophosphate monoesters; and Diest, total orthophosphate diesters corrected for degradation during analysis on the y-axis and the sampled Qu’Appelle lakes are on the x-axis. Different groups of capital and common letters for each P compound indicate significant (p < 0.05) differences in P compound class concentrations among lakes, determined by two-way ANOVAs followed by Tukey HSD post-hoc tests.
Orthophosphate monoesters
There were differences among lakes in two-way ANOVAs for total orthophosphate monoesters (Supplementary Table S5). Concentrations of total orthophosphate monoesters were significantly (p < 0.001) higher in Wascana Lake sediments than in all other lakes (Figure 5). There were significant lakes × depths interactions in two-way ANOVAs for myo-IHP, significant differences among lakes for scyllo-IHP and D-chiro-IHP, and significant differences with depth for neo-IHP (Supplementary Table S5). Concentrations of myo-IHP were significantly (p < 0.01) higher at 0–1-cm in Echo Lake sediment, at 0–1-cm and 1–2-cm in Wascana Lake, and at 5–10-cm in Crooked Lake than all other depths among the lakes (Table 2). Concentrations of scyllo-IHP were significantly (p < 0.05) higher in Wascana Lake sediments than the other lakes (Table 3). Concentrations of D-chiro-IHP were significantly (p < 0.01) higher in Echo Lake and Pasqua Lake sediments and lowest in Crooked Lake (Table 3), while concentrations of neo-IHP were significantly lower (p < 0.01) at 5–10-cm and 10–12-cm than at all other depths, and significantly higher at 5–10-cm than at 10–12-cm (Table 4). There were significant lakes × depths interactions for the unidentified peak at 5 ppm (Supplementary Table S5), concentrations of which were significantly higher (p < 0.01) at 0–1-cm in Echo Lake sediment and at 1–2-cm and 5–10-cm in Wascana Lake than all other depths among the four lakes (Table 2). There were significant differences with depth for g6P concentrations, which were significantly (p < 0.05) higher at 0–1-cm and 1–2-cm than at all other depths (Table 4; Supplementary Table S5). There were significant differences with depth for the orthophosphate monoester three region (Mono 3; Supplementary Table S5), with significantly (p < 0.001) higher concentrations at 0–1-cm and 1–2-cm than at 5–10-cm (Table 4).
Orthophosphate diesters
There were significant differences with both lakes and depths, but no interactions in two-way ANOVAs for total orthophosphate diester concentrations (Supplementary Table S5), which were significantly (p < 0.001) higher in Crooked and Echo Lakes than in the other lakes (Figure 5) and were significantly (p < 0.001) higher at 0–1-cm and 1–2-cm than all other depths (Table 4). The only orthophosphate diester specifically identified in these sediments was DNA. There were significant lakes × depths interactions in two-way ANOVAs for DNA concentrations (Supplementary Table S5), which were significantly (p < 0.01) higher in Pasqua, Echo and Crooked Lakes than in Wascana Lake and generally decreased with depth among the four lakes (Table 2). There were significant differences with both lakes and depths, but no interactions, for concentrations of the unidentified peak at 2.3 ppm (Supplementary Table S5), which were significantly (p < 0.01) higher in Crooked and Echo Lakes than in Wascana Lake (Table 3), and were significantly (p < 0.001) lower at 2–3-cm, 3–4-cm, and 4–5-cm (Table 4). There were significant lakes × depth interactions in two-way ANOVAs for concentrations in the general orthophosphate diester one region (OthDi1; Supplementary Table S5), which were significantly (p < 0.01) higher at all depths in Echo Lake than the other lakes, except at 2–3-cm and 5–10-cm, and 1–2-cm in Pasqua Lake (Table 2).
Phosphonates
There were significant differences with both lakes and depths, but no interactions in two-way ANOVAs for total phosphonates (Supplementary Table S5). Total phosphonates were the lowest concentrations of all the P compound classes among the lakes, were significantly higher (p < 0.001) in Echo Lake and lowest in Wascana Lake sediments (Figure 5), and were significantly (p < 0.05) lower at 0–1-cm, 1–2-cm, and 2–3-cm than in the other depths among the four lakes (Table 4).
Relationships of phosphorus compounds to sediment organic matter, total organic phosphorus, and metals
Organic matter was significantly (p < 0.01) and positively correlated to TPo, both of which were significantly and positively correlated to total phosphonates, total orthophosphate diesters and DNA, but negatively correlated to o-Al across depths among the lakes (Supplementary Table S6). Orthophosphate concentrations were significantly (p < 0.01) and positively correlated to total Al, total Fe, o-Al, and o-Fe (Supplementary Table S7). Total phosphonates were significantly (p < 0.01) and positively correlated to total Ca and c-Mn, but were negatively correlated to total Mg (Supplementary Table S7). Total orthophosphate monoester concentrations were significantly (p < 0.01) and positively correlated to total Al and Mg, and o-Fe and o-Al (Supplementary Table S7). Total orthophosphate diester concentrations were significantly (p < 0.01) and positively correlated to total Ca and Mn, and a-Fe and c-Mn, but were negatively correlated to c-Fe and o-Al (Supplementary Table S7). Concentrations of DNA were significantly (p < 0.01) and positively correlated to total Ca and Mn, and a-Fe, o-Mn, and c-Mn, but were negatively correlated to total Al, Fe and Mg, and c-Fe and o-Al (Supplementary Table S7).
Discussion
Sediment chemistry
The mean sediment eTP concentrations ranged from 0.92 (±0.05) to 1.31 (±0.06) mg P g−1 across the Qu’Appelle lakes used for this study, which is comparable to historical sediment TP concentrations in Pasqua Lake and Echo Lake (0.96–1.05 and 1.35 mg g−1, respectively; Allan and Williams, 1978), and to TP concentrations of other prairie lakes, such as mesotrophic-eutrophic Lake Diefenbaker, a river-valley reservoir in the same watershed in Saskatchewan (0.91–1.24 mg g−1; Doig et al., 2017). Although slightly lower, the study lakes sediment TP concentrations are also comparable to concentrations (0.19–2.86 mg g−1) reported for Lake Erie in Ontario (Williams et al., 1976), Lake Simcoe (0.90–1.10 mg g−1; Audette et al., 2018), and polymictic Nakamun Lake in Alberta (2.12 mg g−1; Orihel et al., 2015). However, they are much lower than the surface sediment TP concentrations for the artificially P-amended Lake 227 in the Experimental Lake Area of Ontario (180–210 μmol g−1, which is 5.6–6.5 mg g−1; O’Connell et al., 2020). The mean TPo concentrations [0.40 (±0.06) to 0.64 (±0.17) mg P g−1] across the studied Qu’Appelle lakes are comparable to sediment TPo concentrations (0.10–0.42 mg P g−1) in seven mesotrophic-eutrophic lakes in China (Zhang et al., 2009), but were higher than 48 different sites in Lake Erie in Canada (<0.01–0.29 mg P g−1; Williams et al., 1976).
Although sediment carbon is not the focus of this study, it is worth noting that the high OM in Echo Lake and Crooked Lake sediments may be due to higher concentrations of OM settling from the water column, or other drivers, such as lower mineralization rates. Although sediment accumulation rates reported across three of the lakes are high relative to much of Canada (Baud et al., 2021), they are fairly similar across the studied Qu’Appelle lakes (Pasqua, 5.7 ± 0.1 mm yr−1; Echo, 4.3 ± 0.1 mm yr−1; and Crooked Lakes, 5.72 ± 0.1 mm yr−1; Dixit et al., 2000; Quinlan et al., 2002). Mineralization has not been directly studied in the lake chain; however, previous research showed that deep lakes have lower sediment OM mineralization than those with shallower depths (e.g., Canadian Shield lakes in Quebec; Den-Heyer and Kalff, 1998). Echo and Crooked Lakes are deeper than Wascana and Pasqua Lakes (Supplementary Table S1), suggesting lake depth effects on mineralization rates may be one driver of these differences. Lake trophic status may also be associated with OM in lake sediments (Ahlgren et al., 2005). Mean sediment OM percentages of 11.3 (±0.44) to 14.6 (±0.94) across the studied Qu’Appelle lakes are comparable to the top 10 cm of seven eutrophic lakes in China with sediment OM of 5.3%–16.9% (Zhang et al., 2009), but are much lower than the sediment OM at depths of 0–2-cm and 32–24-cm in mesotrophic Lake Simcoe in Canada (27.4%; Audette et al., 2018), at 0–5-cm and 5–10-cm depths of seven dimictic lakes from the United States and Canada (23.3%–62.5%; Nürnberg, 1988), and in the top 15 cm of mesotrophic Lake Erken, Sweden (17.2%–21.3%; Reitzel et al., 2007). All of the Qu’Appelle lakes studied here are considered eutrophic; however, productivity is expected to vary across lakes and years, which may affect organic matter deposition.
Calcium was the dominant metal in the sediments of Echo, Crooked and Pasqua Lakes, but not Wascana Lake, the only dredged lake. Previous studies have shown differences in Ca-related mineralogy (calcite vs. dolomite) in water bodies of this lake chain, and have shown that calcite can precipitate from supersaturated water columns to increase sediment Ca (Ghebre-Egziabhier and Arnaud, 1983; Last and Last, 2012). Calcium dominance is not uncommon among Canadian lakes not located on the Canadian Shield; Ca compounds dominated the sediments of Lake Simcoe in Ontario, with total Ca concentrations >65 mg g−1 (Audette et al., 2018), but lower sediment total Ca concentrations were reported for Nakamun Lake (25.4 mg g−1; Orihel et al., 2015). Sediment total Mg concentrations were higher in the study lakes than in Nakamun Lake (6.94 mg g−1; Orihel et al., 2015) and Lake Simcoe (4.5–5.1 mg g−1; Audette et al., 2018). Elevated sediment Al concentrations that were noted in Wascana Lake were also reported for one site in the downstream region of Buffalo Pound Lake (an upstream Qu’Appelle lake), which resulted from long-term alum release (D’Silva, 2017). However, we are not aware of any alum release or application to Wascana Lake. Total Fe concentrations in Echo and Pasqua Lake are comparable to sediment Fe concentrations (17.1 mg g−1) in Nakamun Lake (Orihel et al., 2015) and Lake Simcoe (13.3–14.8 mg g−1; Audette et al., 2018). Low sediment total Mn concentrations in Wascana Lake were comparable to those in Nakamun Lake (0.81 mg g−1; Orihel et al., 2015) and Lake Simcoe (0.47–0.84 mg g−1; Audette et al., 2018). Total Al and Fe concentrations were generally greater across the lakes than Mn. Iron concentrations were generally greater in Wascana Lake and Mn concentrations were greater in Crooked Lake, suggesting that sediment P in these two lakes may be more susceptible to changes in reduction-oxidation processes because of these redox-sensitive metals (Boström et al., 1988; Reddy and Delaune, 2008b). Higher Al and Fe and lower Mn concentrations in Wascana Lake are likely due to the higher clay content (Reddy and Delaune, 2008a), which may be linked to dredging in this lake. Wascana Lake had higher Fe + Mn: Ca concentration ratios, while the other three lakes had higher Ca: Fe + Mn concentration ratios (Supplementary Figure S1).
Phosphorus forms and cycling in lakes
Orthophosphate was the dominant extracted and reactive Pi form in sediments at all depths among the studied Qu’Appelle lakes, which concurred with results for mesotrophic-eutrophic Lake Erken (Ahlgren et al., 2005), Lake Simcoe (Audette et al., 2018), and eutrophic Lake Champlain at the USA-Canada border (Giles et al., 2015). High polyphosphate and pyrophosphate concentrations at the sediment surface in our study lakes are consistent with previous research on sediments of Lake Erken (Ahlgren et al., 2005), Lake Champlain (Giles et al., 2015), and eutrophic Lake Baldegg and oligo-mesotrophic Lake Lucerne in Switzerland (Hupfer et al., 1995). Functions of pyrophosphate and polyphosphates include energy and P storage, stress response, and enzyme activities in several organisms (Hupfer et al., 2007; Raven, 2015). These complex sediment Pi compounds were shown to have a short half-life (∼1 decade) relative to Po in Swedish lakes (Ahlgren et al., 2005; Reitzel et al., 2007) and are hydrolyzed to phosphates during early diagenesis via secreted phosphatases (Hupfer et al., 1995; Hupfer et al., 2004). Additionally, Mn4+ has a high electron affinity to sorb phosphates, can be more readily reduced to Mn2+ under low redox conditions at the SWI to desorb or solubilize phosphates (Mortimer, 1941; Reddy and Delaune, 2008b), and can catalyze the hydrolysis of polyphosphates to orthophosphate in the presence of different Mn oxides (e.g., α-MnO2) and Ca2+ cations (Wan et al., 2019).
Orthophosphate monoesters identified in our study include inositol hexakisphosphates (IHP; with six phosphate groups; Giles et al., 2011), glucose 6-phosphate (g6P), choline phosphate, α- and β-glycerophosphate and mononucleotides (degradation products of phospholipids and RNA, respectively), and unidentified peaks either identified by their chemical shift (unknown 5 ppm) or grouped into general regions of the overall monoesters region of spectra as Monoesters 1, 2, and 3 (Cade-Menun, 2015; Supplementary Table S4) similar to Lake Kvie (Denmark; Paraskova et al., 2015), Lake Champlain (Giles et al., 2015), and Chaohu Lake (China; Zhang et al., 2017). Previous studies have reported that sediment Po was predominantly orthophosphate monoesters in seven different lakes in China (Zhang et al., 2009) and a reservoir in Poland (Młynarczyk et al., 2013). However, these studies did not correct for orthophosphate diester degradation to orthophosphate monoesters during P-NMR extraction and analysis, which will overestimate the total orthophosphate monoester concentrations and underestimate total orthophosphate diester concentrations in the original sediment samples (Zhang et al., 2017).
Four IHP stereoisomers were identified in these lake sediments (myo, scyllo, neo, and D-chiro), similar to previous work in Lake Champlain (Giles et al., 2015). The scyllo-IHP concentrations among the study lakes were consistent with previous work in Danish lake sediments, where it was one of the dominant orthophosphate monoesters (Jørgensen et al., 2011). Phytate (myo-IHP) is a P storage compound in higher plants (Raboy, 2003; Raven, 2015), but the origin of the other stereoisomers is unknown. In soils, the other stereoisomers are reported to be synthesized by microbes de novo or by the transformation of myo-IHP (Giles et al., 2011), but it is unclear if this is also true for aquatic sediments. All four stereoisomers have been identified in Saskatchewan soils, in both native grasslands and agriculturally-managed soils (Liu et al., 2018; Chen et al., 2021); therefore, they may have entered lakes via soil erosion (Giles et al., 2015). Decreases in IHP stereoisomers with depth suggest they may be mineralized by phytase in lake sediments (Giles et al., 2011), consistent with results in Lake Champlain (Giles et al., 2015). Further investigation is warranted to understand the origins and turnover of these important P compounds in Qu’Appelle lake sediments. The specific Po compounds in the monoester 1, 2, and 3 regions are unknown, but they may include lower inositol phosphates and sugar phosphates (Giles et al., 2015). Glucose 6-phosphate is a sugar phosphate that functions in intermediate biochemical reactions (Raven, 2015). The origin and function of the P compound represented by the peak at 5 ppm are unknown.
Orthophosphate diesters were the predominant sediment Po in our study after correction for degradation during analysis, consistent with Chaohu Lake (Zhang et al., 2017), Lake Simcoe (Audette et al., 2018), and Lake 227 (O’Connell et al., 2020). High sediment surface concentrations of DNA among the study lakes contrasted to Lake Champlain where DNA increased with depth (Giles et al., 2015). Bacteria can be a major contributor to DNA in lake sediments (Jørgensen and Jacobsen, 1996; Ishii et al., 1998; Zhang et al., 2009). In the studied Qu’Appelle lakes, DNA may have been extracted from intact microbes (algae and bacteria in these high productivity systems) or OM, but may also have been extracted from DNA sorbed to minerals (Giles et al., 2015; O’Connell et al., 2020). Intracellular and extracellular nucleases, bacteriovore grazing, and OM decomposition are responsible for mineralizing DNA in aquatic environments (Paul et al., 1987). Under algal breakdown and sedimentation, DNA and other biologically-derived P compounds are readily mineralized and released from sediment (Ahlgren et al., 2005) to supply phosphate to the water column, as may be the case in our studied Qu’Appelle lakes, Chaohu Lake (Zhang et al., 2017), and Lake Erken and eutrophic Lake Sønderby (Reitzel et al., 2006).
The unidentified peak at 2.3 ppm was separately quantified because it was prominent and present in most samples, and it may originate from lipoteichoic acid (Cade-Menun, 2015). The OthDi 1 region contains lipoteichoic acids, phospholipids, and RNA not degraded during extraction and NMR analysis (Cade-Menun, 2015; Giles et al., 2015), while the compounds producing peaks in the OthDi 2 region are unknown. As noted for the orthophosphate monoesters above, degradation products of phospholipids and RNA (α- and β-glycerophosphate and mononucleotides) were detected in these lake sediments, consistent with past work (Jørgensen et al., 2011; Giles et al., 2015; Audette et al., 2018). Phospholipids and lipoteichoic acids are contained in cell membranes and are thought to represent microbial communities in sediments (Watts et al., 2002; Cade-Menun, 2015).
Low phosphonate concentrations at the sediment surface in our studied Qu’Appelle lakes agreed with several different southwestern and river channel lakes in China (Zhang et al., 2009) and mountain lakes in Sweden (Ahlgren et al., 2006). Phosphonates have very stable bonds that resist thermal, photolytic, and hydrolytic mineralization (Quinn et al., 2007; Kamat and Raushel, 2013; Sosa et al., 2019), and they exist in natural (e.g., ciliatine, 2-aminoethyl phosphonate) and synthetic forms, including herbicides (e.g., glyphosate), antibiotics (phosphomycin, 1, two epoxypropyl phosphonic extracted from Streptomyces sp.), and detergent additives (Hilderbrand, 1983; Cade-Menun, 2015). The specific source of phosphonates in these lakes is unknown. However, snail shells were observed in Echo Lake sediments, and snails are known to synthesize phosphonates (Miceli et al., 1980), which may explain the higher phosphonate concentrations in that lake. Further investigation is warranted to determine if the phosphonates in these lakes come from natural or synthetic sources.
Sediment metal–P correlations
The positive and negative correlations between P compounds and metal cations presented in the results of this study indicate that metal associations play an important role in retaining P forms in sediments (Reddy and Delaune, 2008a). The correlations of sediment orthophosphate, Al and Fe concentrations among the studied Qu’Appelle lakes were consistent with past work in sediments of eutrophic constructed lakes in China (Cai et al., 2020) and eutrophic lakes in Denmark and Sweden (Reitzel et al., 2006). Total IHP did not correlate to any mineral pools in the studied lake sediments, and neither did any of the IHP stereoisomers, which contrasted with previous sediment studies reporting IHP sorption and precipitation with Ca, Mg, Al, and Fe (Jørgensen et al., 2011; Paraskova et al., 2015). Sediment DNA is the only orthophosphate diester that sorbs (Giles et al., 2015), although sorption is strongest below pH 5 (Condron et al., 2005), well below the pH in these sediments. Phosphonates can adsorb or precipitate to soluble Ca compounds (Hilderbrand, 1983). They can also sorb to o-Fe and o-Al (Sprankle et al., 1975), so we expected to see a correlation of phosphonates to Fe and Al pools, but this was not significant in our study.
Conclusion
Currently, knowledge is limited regarding P species in sediments of hardwater lakes on the North American Great Plains and the factors that control their cycling. This study is the first to use P-NMR to characterize lake sediment P forms in this region. In four lakes from the Qu’Appelle lakes chain in Saskatchewan, Canada, significant differences in sediment P forms were observed that were not detected by measurements of sediment total P and total organic P concentrations alone. The main class of organic P compounds identified by P-NMR in extracts from all four lakes was orthophosphate diesters, typical of living biota, suggesting that P immobilized by microbes and algae is an important pool in these sediments of hardwater eutrophic lakes. Decreases in the concentrations of orthophosphate diesters and other organic P compounds with depth suggest that biological uptake and assimilation, decomposition and mineralization via enzyme hydrolysis and cell lysis appear to be important diagenetic processes at the SWI in these study lakes, either simultaneous with, or sequentially to redox processes and sedimentation. Significant differences were detected among the lakes for pools and concentrations of metal cations, suggesting there may be different processes controlling P cycling in these lakes. Of the total metal cation concentrations in these lakes, concentrations of Ca were greatest in Pasqua, Echo, and Crooked Lakes, while the concentrations of Al and Fe were greater in Wascana Lake. Crooked Lake had greater total Al and Fe concentrations than Pasqua and Echo Lakes, and the greatest total Mn concentrations of the lakes. In all four lakes, Al, Mn, and Fe were predominantly organically complexed. These metal cations appear to have considerable influence on the inorganic P forms (which formed 47%–66% of the eTP). Although total P and total organic P concentrations were highest in Echo and Crooked Lakes, the recovery of total P in extracts for P-NMR was low for these lakes and greatest for Wascana Lake. This suggests that P was loosely bound to Al and Fe compounds in Wascana Lake, but more tightly bound with Ca compounds of low solubility in the lakes with greater total Ca concentrations.
Significant differences were observed for P species among the lakes. Orthophosphate and orthophosphate monoester concentrations were greater in Wascana Lake than in the other lakes, while orthophosphate diesters were greater in Echo and Crooked Lakes. Differences with depth and some lakes and depths interactions were also observed, including increased orthophosphate concentrations and decreased orthophosphate diester concentrations with depth. Factors that may be responsible for the differences among these lakes include P retention by metal cations (including redox-sensitive processes) and variations in inputs, decomposition, and mineralization. It is not clear which of these processes most controls P in each lake, but it is clear that there are differences in factors controlling P cycling even within these four lakes, although they are all part of the same lake chain. These factors must be taken into consideration because they could affect P management and water quality control measures in these and other lakes within the Great Plains.
Data availability statement
The original contributions presented in the study are included in the article/Supplementary Material; further inquiries can be directed to the corresponding authors.
Author contributions
MW-T: Conceptualization, Project funding, Analytical work, Data curation, Methodology, Writing original draft; BC-M: Conceptualization, Analytical work, Methodology, NMR interpretation, Writing original draft; HB: Conceptualization, Project funding, Methodology, Writing original draft. All authors have read and approved the final version of this manuscript prior to submission.
Funding
This study was funded by the Organization of American States (OAS), Environment Canada–Environmental Damages Fund, Natural Sciences and Engineering Research Council of Canada- Collaborative Research and Training Experience (NSERC–CREATE) program, NSERC Discovery grant to Helen Baulch, School of Environment and Sustainability (SENS) at the University of Saskatchewan, including the Centennial Enhancement Chair (CEC) Program, and the Global Institute for Water Security (GIWS).
Conflict of interest
The authors declare that the research was conducted in the absence of any commercial or financial relationships that could be construed as a potential conflict of interest.
Publisher’s note
All claims expressed in this article are solely those of the authors and do not necessarily represent those of their affiliated organizations, or those of the publisher, the editors and the reviewers. Any product that may be evaluated in this article, or claim that may be made by its manufacturer, is not guaranteed or endorsed by the publisher.
Acknowledgments
We are thankful to Lukas Smith for his help with the map, Jianfeng Zhu (Saskatchewan Structural Sciences Centre, University of Saskatchewan), staff at the Swift Current Research and Development Centre, Agriculture and Agri-Food Canada (SCRD, AAFC), and Kim Gilmour, Katy Nugent and Cameron Hoggarth at the Saskatchewan Water Chemistry and Ecology (SaskWatChe) Laboratory for laboratory and field assistance.
Supplementary material
The Supplementary Material for this article can be found online at: https://www.frontiersin.org/articles/10.3389/fenvs.2022.928824/full#supplementary-material
References
Abdi, D., Cade-Menun, B. J., Ziadi, N., and Parent, L. É. (2015). Compositional statistical analysis of soil 31P-NMR forms. Geoderma 257–258, 40–47. doi:10.1016/j.geoderma.2015.03.019
Ahlgren, J., Reitzel, K., Danielsson, R., Gogoll, A., and Rydin, E. (2006). Biogenic phosphorus in oligotrophic mountain lake sediments: differences in composition measured with NMR spectroscopy. Water Res. 40 (20), 3705–3712. doi:10.1016/j.watres.2006.09.006
Ahlgren, J., Tranvik, L., Gogoll, A., Waldebäck, M., Markides, K., Rydin, E., et al. (2005). Sediment depth attenuation of biogenic phosphorus compounds measured by 31P-NMR. Environ. Sci. Technol. 39, 867–872. doi:10.1021/es049590h
Allan, R. J., and Roy, M. (1980). Lake water nutrient chemistry and chlorophyll a in Pasqua, Echo, mission, katepwa, crooked and round lakes on the Qu’Appelle River, saskatchewan. Sci. Ser. 112, 37.
Allan, R. J., and Williams, J. D. H. (1978). Trophic status related to sediment chemistry of Canadian prairie lakes. J. Environ. Qual. 7 (1), 99–106. doi:10.2134/jeq1978.00472425000700010020x
Amirbahman, A., Lake, B. A., and Norton, S. A. (2013). Seasonal phosphorus dynamics in the surficial sediment of two shallow temperate lakes: a solid-phase and pore-water study. Hydrobiologia 701 (1), 65–77. doi:10.1007/s10750-012-1257-z
Audette, Y., O’Halloran, I. P., Nowell, P. M., Dyer, R., Kelly, R., Voroney, R. P., et al. (2018). Speciation of phosphorus from agricultural muck soils to stream and lake sediments. J. Environ. Qual. 47 (4), 884–892. doi:10.2134/jeq2018.02.0068
Bai, X., Ding, S., Fan, C., Liu, T., Shi, D., Zhang, L., et al. (2009). Organic phosphorus species in surface sediments of a large, shallow, eutrophic lake, Lake Taihu, China. Environ. Pollut. 157 (8–9), 2507–2513. doi:10.1016/j.envpol.2009.03.018
Baldwin, D. S. (1996). The phosphorus composition of a diverse series of Australian sediments. Hydrobiologia 335, 63–73. doi:10.1007/BF00013684
Barica, J. (1993). Boundaries of ecological sustainability of prairie lakes and reservoirs. Can. Water Resour. J. 18 (3), 291–297. doi:10.4296/cwrj1803291
Baud, A., Jenny, J. P., Francus, P., and Gregory-Eaves, I. (2021). Global acceleration of lake sediment accumulation rates associated with recent human population growth and land-use changes. J. Paleolimnol. 66 (4), 453–467. doi:10.1007/s10933-021-00217-6
Baulch, H. M., Elliott, J. A., Cordeiro, M. R. C., Flaten, D. N., Lobb, D. A., Wilson, H. F., et al. (2019). Soil and water management: opportunities to mitigate nutrient losses to surface waters in the northern Great Plains. Environ. Rev. 27 (4), 447–477. doi:10.1139/er-2018-0101
Boström, B., Andersen, J. M., Fleischer, S., and Jansson, M. (1988). Exchange of phosphorus across the sediment-water interface. Hydrobiologia 170, 229–244. doi:10.1007/bf00024907
Cade-Menun, B. J. (2015). Improved peak identification in 31P-NMR spectra of environmental samples with a standardized method and peak library. Geoderma 257–258, 102–114. doi:10.1016/j.geoderma.2014.12.016
Cade-Menun, B. J., and Liu, C. W. (2014). Solution phosphorus-31 nuclear magnetic resonance spectroscopy of soils from 2005 to 2013: a review of sample preparation and experimental parameters. Soil Sci. Soc. Am. J. 78, 19–37. doi:10.2136/sssaj2013.05.0187dgs
Cade-Menun, B. J., Navaratnam, J. A., and Walbridge, M. R. (2006). Characterizing dissolved and particulate phosphorus in water with 31P nuclear magnetic resonance spectroscopy. Environ. Sci. Technol. 40, 7874–7880. doi:10.1021/es061843e
Cade-Menun, B. J., and Preston, C. M. (1996). A comparison of soil extraction procedure for 31P-NMR spectroscopy. Soil Sci. 161 (11), 770–785.
Cai, O., Xiong, Y., Yang, H., Liu, J., and Wang, H. (2020). Phosphorus transformation under the influence of aluminum, organic carbon, and dissolved oxygen at the water-sediment interface: a simulative study. Front. Environ. Sci. Eng. 14 (3), 50. doi:10.1007/s11783-020-1227-z
Carey, R. O., and Migliaccio, K. W. (2009). Contribution of wastewater treatment plant effluents to nutrient dynamics in aquatic systems: a review. Environ. Manage. 44, 205–217. doi:10.1007/s00267-009-9309-5
Carpenter, S. R., Caraco, N. F., Correll, D. L., Howarth, R. W., Sharpley, A. N., Smith, V. H., et al. (1998). Nonpoint pollution of surface waters with phosphorus and nitrogen. Ecol. Appl. 8 (3), 559–568. doi:10.1890/1051-0761(1998)008[0559:nposww]2.0.co;2
Chen, S., Cade-Menun, B. J., Bainard, L. D., Luce, St.M., Hu, Y., Chen, Q., et al. (2021). The influence of long-term N and P fertilization on soil P forms and cycling in a wheat/fallow cropping system. Geoderma 404 (June), 115274. doi:10.1016/j.geoderma.2021.115274
Condron, L. M., and Newman, S. (2011). Revisiting the fundamentals of phosphorus fractionation of sediments and soils. J. Soils Sediments 11, 830–840. doi:10.1007/s11368-011-0363-2
Condron, L. M., Turner, B. L., and Cade-Menun, B. J. (2005). Chemistry and dynamics of soil organic phosphorus. Phosphorus agri. Environ. 46, 87–121. doi:10.2134/agronmonogr46.c4
Correll, D. L. (1998). The role of phosphorus in the eutrophication of receiving waters: a review. J. Environ. Qual. 27 (2), 261–266. doi:10.2134/jeq1998.00472425002700020004x
Courchesne, F., and Turmel, M. -C. (2008). “Soil reaction and exchangeable acidity,” in Soil sampling and methods of analysis. Editors M. R. Carter, and E. G. Gregorich. 2nd edition (Boca Raton, FL, USA: Canadian Society of Soil Science), 307–315.
Den-Heyer, C., and Kalff, J. (1998). Organic matter mineralization rates in sediments: a within-and among-lake study. Limnol. Oceanogr. 43 (4), 695–705. doi:10.4319/lo.1998.43.4.0695
Dixit, A. S., Hall, R. I., Leavitt, P. R., Quinlan, R., and Smol, J. P. (2000). Effects of sequential depositional basins on lake response to urban and agricultural pollution: a palaeoecological analysis of the Qu’Appelle Valley, Saskatchewan, Canada. Freshw. Biol. 43 (3), 319–337. doi:10.1046/j.1365-2427.2000.00516.x
Doig, L. E., North, R. L., Hudson, J. J., Hewlett, C., Lindenschmidt, K. E., Liber, K., et al. (2017). Phosphorus release from sediments in a river-valley reservoir in the northern Great Plains of North America. Hydrobiologia 787, 323–339. doi:10.1007/s10750-016-2977-2
Dong, L., Yang, Z., Liu, X., and Liu, G. (2012). Investigation into organic phosphorus species in sediments of Baiyangdian Lake in China measured by fractionation and 31P NMR. Environ. Monit. Assess. 184 (9), 5829–5839. doi:10.1007/s10661-012-2550-z
D’Silva, L. (2017). Biological and physicochemical mechanisms affecting phosphorus and arsenic efflux from prairie reservoir sediment, Buffalo Pound Lake, SK, Canada. M. Sc. Thesis. Saskatoon, SK, Canada: University of Saskatchewan.
Ghebre-Egziabhier, K., and Arnaud, St.R. J. (1983). Carbonate mineralogy of lake sediments and surrounding soils. 2. The Qu’Appelle lakes ( Canada). Can. J. Soil Sci. 63 (2), 259–269. doi:10.4141/cjss83-026
Giles, C. D., Cade-Menun, B. J., and Hill, J. E. (2011). The inositol phosphates in soils and manures: abundance, cycling, and measurement. Can. J. Soil Sci. 91 (3), 397–416. doi:10.4141/cjss09090
Giles, C. D., Lee, L. G., Cade-Menun, B. J., Hill, J. E., Isles, P. D. F., Schroth, A. W., et al. (2015). Characterization of organic phosphorus form and bioavailability in lake sediments using P nuclear magnetic resonance and enzymatic hydrolysis. J. Environ. Qual. 44, 882–894. doi:10.2134/jeq2014.06.0273
Glew, J. R., Smol, J. P., and Last, W. M. (2001). “Sediment core collection and extrusion,” in Tracking environmental change in lake sediments. Vol 1, basin analysis, coring and chronological techniques. Editors W. M. Last, and J. P. Smol (Dordrecht: Kluwer Academic Publishers), 73–105.
Hall, R. I., Leavitt, P. R., Quinlan, R., Dixit, A. S., and Smol, J. P. (1999). Effects of agriculture, urbanization, and climate on water quality in the northern Great Plains. Limnol. Oceanogr. 44 (3), 739–756. doi:10.4319/lo.1999.44.3_part_2.0739
Hamilton, S. K., Bruesewitz, D. A., Horst, G. P., Weed, D. B., and Sarnelle, O. (2009). Biogenic calcite–phosphorus precipitation as a negative feedback to lake eutrophication. Can. J. Fish. Aquat. Sci. 66, 343–350. doi:10.1139/F09-003
Hammer, U. T. (1971). Limnological studies of the lakes and streams of the upper Qu’Appelle river system, Saskatchewan, Canada, I. chemical and physical aspects of the lakes and drainage System. Hydrobiologia 37 (3-4), 473–507. doi:10.1007/bf00018815
Hammer, U. T. (1986). Saline lake resources of the Canadian prairies. Can. Water Resour. J. 11 (1), 43–57. doi:10.4296/cwrj1101043
Hendershot, W. H., Lalonde, H., and Duquette, M. (2008). “Soil reaction and exchangeable acidity,” in Soil sampling and methods of analysis. Editors M. R. Carter, and E. G. Gregorich. 2nd edition (Boca Raton, FL, USA: Canadian Society of Soil Science), 173–178.
Hilderbrand, R. (1983). The role of phosphonates in living systems. 1st edition. Boca Raton, FL, USA: CRC Press.
Hughes, B. (2004). The big dig: The miracle of Wascana Centre. Regina, Saskatchewan, Canada. Regina, SK, Canada: Centax Books, a division of PrintWest Communications Ltd.
Hupfer, M., Gachter, R., and Ruegger, H. (1995). Polyphosphate in Lake sediments: 31P- NMR spectroscopy as a tool for its identification. Limnol. Oceanogr. 40 (3), 610–617. doi:10.4319/lo.1995.40.3.0610
Hupfer, M., Gloess, S., and Grossart, H. P. (2007). Polyphosphate-accumulating microorganisms in aquatic sediments. Aquat. Microb. Ecol. 47 (3), 299–311. doi:10.3354/ame047299
Hupfer, M., and Lewandowski, J. (2008). Oxygen controls the phosphorus release from Lake sediments – A long-lasting paradigm in limnology. Int. Rev. Hydrobiol. 93 (4–5), 415–432. doi:10.1002/iroh.200711054
Hupfer, M., Rube, B., and Schmieder, P. (2004). Origin and diagenesis of polyphosphate in Lake sediments: a 31P-NMR study. Limnol. Oceanogr. 49 (1), 1–10. doi:10.4319/lo.2004.49.1.0001
Ishii, N., Kawabata, Z., Nakano, S. I., Min, M. G., and Takata, R. (1998). Microbial interactions responsible for dissolved DNA production in a hypereutrophic pond. Hydrobiologia 380 (1–3), 67–76. doi:10.1023/a:1003475000195
Jan, J., Borovec, J., Kopáček, J., and Hejzlar, J. (2013). What do results of common sequential fractionation and single-step extractions tell us about P binding with Fe and Al compounds in non-calcareous sediments? Water Res. 47 (2), 547–557. doi:10.1016/j.watres.2012.10.053
Jarvie, H. P., Sharpley, A. N., Flaten, D., Kleinman, P. J. A., Jenkins, A., Simmons, T., et al. (2015). The pivotal role of phosphorus in a resilient water–energy–food security nexus. J. Environ. Qual. 44, 1049–1062. doi:10.2134/jeq2015.01.0030
Jørgensen, C., Jensen, H. S., Andersen, F., Egemose, S., and Reitzel, K. (2011). Occurrence of orthophosphate monoesters in Lake sediments: significance of myo- and scyllo-inositol hexakisphosphate. J. Environ. Monit. 13 (8), 2328. doi:10.1039/c1em10202h
Jørgensen, N. O. G., and Jacobsen, C. S. (1996). Bacterial uptake and utilization of dissolved DNA. Aquat. Microb. Ecol. 11 (3), 263–270. doi:10.3354/ame011263
Kamat, S. S., and Raushel, F. M. (2013). The enzymatic conversion of phosphonates to phosphate by bacteria. Curr. Opin. Chem. Biol. 17 (4), 589–596. doi:10.1016/j.cbpa.2013.06.006
Kroetsch, D., and Wang, C. (2008). “Particle size distribution,” in Soil sampling and methods of analysis. Editors M. R. Carter, and E. G. Gregorich. 2nd edition (Boca Raton, FL, USA: Canadian Society of Soil Science), 173–725.
Last, F. M., and Last, W. M. (2012). Lacustrine carbonates of the northern Great Plains of Canada. Sediment. Geol. 277–278, 1–31. doi:10.1016/j.sedgeo.2012.07.011
Liu, J., Cade-Menun, B. J., Yang, J., Hu, Y., Liu, C. W., Tremblay, J., et al. (2018). Long-term land use affects phosphorus speciation and the composition of phosphorus cycling genes in agricultural soils. Front. Microbiol. 9 (Jul), 1643. doi:10.3389/fmicb.2018.01643
McDowell, R. W., Stewart, I., and Cade-Menun, B. J. (2006). An examination of spin–lattice relaxation times for analysis of soil and manure extracts by liquid state phosphorus-31 nuclear magnetic resonance spectroscopy. J. Environ. Qual. 35, 293–302. doi:10.2134/jeq2005.0285
McGowan, S., Patoine, A., Graham, M. D., and Leavitt, P. R. (2005). Intrinsic and extrinsic controls on lake phytoplankton synchrony as illustrated by algal pigments. Verh. Intern. Ver. Limnol. 29, 794–798. doi:10.1080/03680770.2005.11902787
Miceli, M., Henderson, T., and Myers, T. (1980). 2-Aminoethylphosphonic acid metabolism during embryonic development of the planorbid snail Helisoma. Science 209, 1245–1247. doi:10.1126/science.209.4462.1245
Młynarczyk, N., Bartoszek, M., Polak, J., and Sułkowski, W. W. (2013). Forms of phosphorus in sediments from the Goczałkowice Reservoir. Appl. Geochem. 37, 87–93. doi:10.1016/j.apgeochem.2013.07.008
Mortimer, C. H. (1941). The exchange of dissolved substances between mud and water in lakes. J. Ecol. 29 (2), 147. doi:10.2307/2256691
Murphy, J., and Riley, J. P. (1962). A modified single solution method for the determination of phosphate in natural waters. Anal. Chim. Acta X. 27, 31–36. doi:10.1016/s0003-2670(00)88444-5
North, R. L., Johansson, J., Vandergucht, D. M., Doig, L. E., Liber, K., Lindenschmidt, K. E., et al. (2015). Evidence for internal phosphorus loading in a large prairie reservoir (Lake Diefenbaker, Saskatchewan). J. Gt. Lakes. Res. 41, 91–99. doi:10.1016/j.jglr.2015.07.003
Nürnberg, G. K. (1988). Prediction of phosphorus release rates from total and reductant-soluble phosphorus in anoxic lake sediments. Can. J. Fish. Aquat. Sci. 45, 453–462. doi:10.1139/f88-054
O’Connell, D. W., Ansems, N., Kukkadapu, R. K., Jaisi, D., Orihel, D. M., Cade-Menun, B. J., et al. (2020). Changes in sedimentary phosphorus burial following artificial eutrophication of Lake 227, Experimental Lakes Area, Ontario, Canada. J. Geophys. Res. Biogeosci. 125, e2020JG005713. doi:10.1029/2020JG005713
O’Halloran, I. P., and Cade-Menun, B. J. (2008). “Total and organic phosphorus,” in Soil sampling and methods of analysis. Editors M. R. Carter, and E. G. Gregorich. 2nd edition (Boca Raton, FL, USA: Canadian Society of Soil Science), 265–291.
Orihel, D. M., Baulch, H. M., Casson, N. J., North, R. L., Parsons, C. T., Seckar, D. C. M., et al. (2017). Internal phosphorus loading in Canadian fresh waters: a critical review and data analysis. Can. J. Fish. Aquat. Sci. 74 (12), 2005–2029. doi:10.1139/cjfas-2016-0500
Orihel, D. M., Schindler, D. W., Ballard, N. C., Graham, M. D., O’Connell, D. W., Wilson, L. R., et al. (2015). The “nutrient pump:” iron-poor sediments fuel low nitrogen-to-phosphorus ratios and cyanobacterial blooms in polymictic lakes. Limnol. Oceanogr. 60, 856–871. doi:10.1002/lno.10076
Otsuki, A., and Wetzel, R. G. (1972). Coprecipitation of phosphate with carbonates in marl lakes. Hickory Corners, 49060: Notes for W. K. Kellogg Biological Station, Michigan State University, 763–767.
Paraskova, J. V., Jørgensen, C., Reitzel, K., Pettersson, J., Rydin, E., Sjöberg, P. J. R., et al. (2015). Speciation of inositol phosphates in lake sediments by ion-exchange chromatography coupled with mass spectrometry, inductively coupled plasma atomic emission spectroscopy, and 31P NMR spectroscopy. Anal. Chem. 87, 2672–2677. doi:10.1021/ac5033484
Patoine, A., and Leavitt, P. R. (2006). Century-long synchrony of fossil algae in a chain of Canadian prairie lakes. Ecology 87 (7), 1710–1721. doi:10.1890/0012-9658(2006)87[1710:csofai]2.0.co;2
Paul, J. H., Jeffrey, W. H., and DeFlaun, M. F. (1987). Dynamics of extracellular DNA in the marine environment. Appl. Environ. Microbiol. 53 (1), 170–179. doi:10.1128/aem.53.1.170-179.1987
Pomeroy, J. W., De Boer, D., and Martz, L. W. (2005). Hydrology and Water Resources of Saskatchewan, Centre for Hydrology: Report #1. Centre for Hydrology, 117 science place. Saskatoon, SK Canada: University of Saskatchewan.
Quinlan, R. (2000). Fossil chironomids as indicators of water quality changes in south-central Ontario and Qu’Appelle Valley (Saskatchewan) lakes. Ph. D. thesis. Kingston, ON (Canada): Queen’s University.
Quinlan, R., Leavitt, P. R., Dixit, A. S., Hall, R. I., and Smol, J. P. (2002). Landscape effects of climate, agriculture, and urbanization on benthic invertebrate communities of Canadian prairie lakes. Limnol. Oceanogr. 47 (2), 378–391. doi:10.4319/lo.2002.47.2.0378
Quinn, J. P., Kulakova, A. N., Cooley, N. A., and MGrath, J. W. (2007). New ways to break an old bond: the bacterial carbon-phosphorus hydrolases and their role in biogeochemical phosphorus cycling. Environ. Microbiol. 9 (10), 2392–2400. doi:10.1111/j.1462-2920.2007.01397.x
R Core Team (2020). R: A language and environment for statistical computing. Vienna, Austria: R Foundation for Statistical Computing. Available at: https://www.R-project.org/
Raboy, V. (2003). myo-Inositol-1, 2, 3, 4, 5, 6-hexakisphosphate. Phytochemistry 64 (6), 1033–1043. doi:10.1016/S0031-9422(03)00446-1
Raven, J. A. (2015). “Interactions between nitrogen and phosphorus metabolism,” in Annual plant reviews,Phosphorus metabolism in plants. 1st Edn, Editors W. C. Plaxton, and H. Lambers (New Jersey, USA: John Wiley & Sons, Ltd.), Chap. 7, Vol. 48, 187–214. doi:10.1002/9781118958841.ch7
Rawson, D. S., and Moore, J. E. (1944). The saline lakes of Saskatchewan. Can. J. Res. 22, 141–201. doi:10.1139/cjr44d-011
Read, E. K., Ivancic, M., Hanson, P., Cade-Menun, B. J., and McMahon, K. D. (2014). Phosphorus speciation in a eutrophic lake by 31P NMR spectroscopy. Water Res. 62, 229–240. doi:10.1016/j.watres.2014.06.005
Reddy, R., and Delaune, R. D. (2008b). “Iron and manganese,” in Biogeochemistry of wetlands, science and applications. 1st edition (Boca Raton: Taylor & Francis Group, LLC), 405–445.
Reddy, R., and Delaune, R. D. (2008a). “Phosphorus,” in Biogeochemistry of wetlands, science and applications. 1st edition (Boca Raton: Taylor & Francis Group, LLC), 325–404.
Reitzel, K., Ahlgren, J., DeBrabandere, H., Waldebäck, M., Gogoll, A., Tranvik, L., et al. (2007). Degradation rates of organic phosphorus in lake sediment. Biogeochemistry 82 (1), 15–28. doi:10.1007/s10533-006-9049-z
Reitzel, K., Ahlgren, J., Gogoll, A., Jensen, H. S., and Rydin, E. (2006). Characterization of phosphorus in sequential extracts from lake sediments using 31P nuclear magnetic resonance spectroscopy. Can. J. Fish. Aquat. Sci. 63 (8), 1686–1699. doi:10.1139/F06-070
Riddell, W. A. (1992). The origin and development of Wascana Centre. Regina, SK. Canada: Wascana Centre Authority.
Saskatchewan Water Security Agency (2018). Qu’Appelle nutrient mass balance report 2013-2016. Water quality & habitat assessment services report WQ201812-01. Available at: https://www.wsask.ca/wp-content/uploads/2021/03/The-QuAppelle-Nutrient-Mass-Balance-Report.pdf. (Accessed Dec 20, 2019).
Sauchyn, D. J., Barrow, E. M., Hopkinson, R. F., and Leavitt, P. R. (2002). Aridity on the Canadian Plains. Géographie Phys. Quat. 56 (2–3), 247–259. doi:10.7202/009109ar
Saunders, W. M. H., and Williams, E. G. (1955). Observations on the determination of total organic phosphorus in soils. J. Soil Sci. 6 (2), 254–267. doi:10.1111/j.1365-2389.1955.tb00849.x
Schindler, D. W., and Donahue, W. F. (2006). An impending water crisis in Canada’s western prairie provinces. Proc. Natl. Acad. Sci. U. S. A. 103 (19), 7210–7216. doi:10.1073/pnas.0601568103
Schindler, D. W. (2012). The dilemma of controlling cultural eutrophication of lakes. Proc. R. Soc. B 279, 4322–4333. doi:10.1098/rspb.2012.1032
Schneider, K. D., Cade-Menun, B. J., Lynch, D. H., and Voroney, R. P. (2016). Soil phosphorus forms from organic and conventional forage fields. Soil Sci. Soc. Am. J. 80 (2), 328–340. doi:10.2136/sssaj2015.09.0340
Shaw, J. F. H., and Prepas, E. E. (1990). Relationships between phosphorus in shallow sediments and in the trophogenic zone of seven Alberta lakes. Water Res. 24 (5), 551–556. doi:10.1016/0043-1354(90)90186-A
Shinohara, R., Imai, A., Kawasaki, N., Komatsu, K., Kohzu, A., Miura, S., et al. (2012). Biogenic phosphorus compounds in sediment and suspended particles in a shallow eutrophic lake: a 31P-nuclear magnetic resonance (31P-NMR) study. Environ. Sci. Technol. 46 (19), 10572–10578. doi:10.1021/es301887z
Søndergaard, M., Jensen, P. J., and Jeppesen, E. (2001). Retention and internal loading of phosphorus in shallow, eutrophic lakes. Sci. World J. 1, 427–442. doi:10.1100/tsw.2001.72
Sosa, O. A., Repeta, D. J., DeLong, E. F., Ashkezari, M. D., and Karl, D. M. (2019). Phosphate-limited ocean regions select for bacterial populations enriched in the carbon–phosphorus lyase pathway for phosphonate degradation. Environ. Microbiol. 21 (7), 2402–2414. doi:10.1111/1462-2920.14628
Sprankle, P., Meggitt, W., and Penner, D. (1975). Adsorption, mobility, and microbial degradation of glyphosate in the soil. Weed Sci. 23 (3), 229–234. doi:10.1017/s0043174500052929
Tollerud, H., Brown, J., Loveland, T., Mahmood, R., and Bliss, N. (2018). Drought and land-cover conditions in the Great Plains. Earth Interact. 22 (17), 1. doi:10.1175/EI-D-17-0025.1
Vogt, R. J., Sharma, S., and Leavitt, P. R. (2015). Decadal regulation of phytoplankton abundance and water clarity in a large continental reservoir by climatic, hydrologic and trophic processes. J. Gt. Lakes. Res. 41 (Suppl. 2), 81–90. doi:10.1016/j.jglr.2014.11.007
Wan, B., Huang, R., Diaz, J. M., and Tang, Y. (2019). Manganese oxide catalyzed hydrolysis of polyphosphates. ACS Earth Space Chem. 3 (11), 2623–2634. doi:10.1021/acsearthspacechem.9b00220
Watts, E. E., Dean, P. A. W., and Martin, R. R. (2002). 31P Nuclear magnetic resonance study of sediment microbial phospholipids. Can. J. Anal. Sci. Spectrosc. 47 (5), 127–133.
Wheater, H., and Gober, P. (2013). Water security in the Canadian Prairies: science and management challenges. Phil. Trans. R. Soc. A 371, 20120409. doi:10.1098/rsta.2012.0409
Williams, J. D. H., Jaquet, J.-M., and Thomas, R. L. (1976). Forms of phosphorus in the surficial sediments of Lake Erie. J. Fish. Res. Bd. Can. 33 (3), 413–429. doi:10.1139/f76-063
Zar, J. H. (1999). Biostatistical analysis. 4th edition. Upper Saddle River, New Jersey, USA: Prentice-Hall.
Zhang, R., Wu, F., He, Z., Zheng, J., Song, B., Jin, L., et al. (2009). Phosphorus composition in sediments from seven different trophic lakes, China: a phosphorus-31 NMR study. J. Environ. Qual. 38 (1), 353–359. doi:10.2134/jeq2007.0616
Keywords: P-NMR, metals, calcium, hardwater lakes, organic P, P biogeochemistry
Citation: Wauchope-Thompson MS, Cade-Menun BJ and Baulch HM (2022) Phosphorus forms by depth in sediments from the Qu’Appelle lakes, Saskatchewan, Canada. Front. Environ. Sci. 10:928824. doi: 10.3389/fenvs.2022.928824
Received: 26 April 2022; Accepted: 30 June 2022;
Published: 26 August 2022.
Edited by:
Jun Hou, Hohai University, ChinaReviewed by:
Nathalie Gassama, Université de Tours, FranceJeng-Wei Tsai, China Medical University, Taiwan
Copyright © 2022 Wauchope-Thompson, Cade-Menun and Baulch. and Her Majesty the Queen in Right of Canada, as represented by the Minister of Agriculture and Agri-Food Canada for the contribution of Barbara J. Cade-Menun. This is an open-access article distributed under the terms of the Creative Commons Attribution License (CC BY). The use, distribution or reproduction in other forums is permitted, provided the original author(s) and the copyright owner(s) are credited and that the original publication in this journal is cited, in accordance with accepted academic practice. No use, distribution or reproduction is permitted which does not comply with these terms.
*Correspondence: Michelle S. Wauchope-Thompson, bWljaGVsbGV3YXVjaG9wZUBnbWFpbC5jb20=; Barbara J. Cade-Menun, YmFyYmFyYS5jYWRlLW1lbnVuQGFnci5nYy5jYQ==
†ORCID: Helen M. Baulch, orcid.org/0000-0003-4391-3718