- 1Marine Agriculture Research Center, Tobacco Research Institute, Chinese Academy of Agricultural Sciences, Qingdao, China
- 2School of Resources and Environment, Northeast Agricultural University, Harbin, China
- 3Weifang Engineering Vocational College, Weifang, China
Coastal soils in the Yellow River Delta (YRD) are characterized by high salinity and degraded physicochemical properties, which threaten agricultural production. Biochar has received growing interest as a sustainable soil amendment. However, the effects of biochar on coastal soil quality and the soil microbial response in the field are limited. In this study, the responses of soil properties and microbes to biochar amendment at low dosage (LBC, 18 ton/ha) and high dosage (HBC, 36 ton/ha) and no biochar treatment (CK) were investigated in a peanut field located in the YRD. The results elucidated that biochar-amended soils showed higher available nutrient (i.e., nitrogen, phosphorus, and potassium) contents and cation exchange capacity, but exhibited lower electrical conductivity. Generally, the bacterial community was more easily impacted than that of fungi in both LBC and HBC treatments. Furthermore, the LBC amendment not only improved the abundance of some beneficial bacteria (i.e., Sphingomonas and Nannocystis) but also increased the complexity, modularity index, and competitive interactions of the bacterial co-occurrence network. HBC-enriched Rozellomycota that is probably associated with peanut rot decreased the modularity index and competitive interactions, which might account for the decreased peanut yield under HBC treatment. It is encouraged to comprehensively consider the interaction among microorganisms when evaluating the effects of soil amendments on the soil environment, which plays a vital role in rhizosphere microecology and soil quality.
Introduction
Soil salinity is regarded as one of the most severe environmental stresses for crop growth, which threatens food safety (Xu et al., 2018). The coastal saline soil accounts for about half of the area of the Yellow River Delta (YRD), a typical coastal wetland in China with an area of 5.45 × 105 ha (Li et al., 2019), which is characterized by degraded physical structure (i.e., water holding capacity and hydraulic conductivity), chemical properties (i.e., exchangeable sodium and cation exchange capacity), nutrient status (i.e., available nitrogen, phosphorus, and potassium), and biological characteristics (i.e., microbial diversity and communities), ultimately inhibiting crop growth and yield (El-Akhal et al., 2013; Xu et al., 2019). Coastal saline soil might be converted into an important cultivated land resource if the soil properties were improved when amended with effective strategies (You et al., 2021).
With improving the physical, chemical, and biological properties of soil, biochar shows great potential as an effective, economic, and ecological amendment to the degraded coastal saline soil (Luo et al., 2016a). For example, biochar amendment was proved to promote plant growth by improving nutrient availability and microbial activities in YRD soil (Zheng et al., 2018). Biochar amendment can also decrease soil bulk density, and enhance soil water retention and soil aggregates (Akhtar et al., 2014). Moreover, biochar was reported to affect the mineralization of soil organic matter in the YRD (Luo et al., 2016b). Soil microorganisms involve in critical soil processes (that are, nutrient transformation and soil aggregation), which play important roles in improving soil quality (Saifullah et al., 2018). Soil microbial diversity and community composition are highly sensitive to soil environmental conditions (Zheng et al., 2018). High soil salinity could negatively affect microbial growth, activity, and diversity, and the application of a suitable type and dosage of biochar to saline soil can alleviate the toxicity of salinity to microbes and improve microbial growth and development (Bhaduri et al., 2016). For example, the abundances of phyla Cytophagaceae, Altererythrobacter, and Saprospiraceae were significantly increased, but the abundances of genera Gemmata and Flavitalea were significantly reduced in the soil amended with lignocellulosic biochar at 2.0%–2.5% (w/w) (low levels), which was opposite to the microbial response in the soils amended with 5.0%–10.0% (w/w) (high levels) of biochar (He et al., 2020). In addition, it is reported that microbial interactions and keystone taxa in the network are often pertinent to the major shifts in the whole community structure, which could be affected by biochar additions (Herren and McMahon, 2018). For example, Chen et al. (2019) found that biochar considerably increased soil pH and moisture capacity, which further shaped the composition and co-occurrence networks of the bacterial community. However, the information on the effects of biochar on the characteristics of microbial interactions of the coastal saline soil is limited, which needed to be clarified. In addition, previous research were generally conducted in with short-term pot experiments, which have not been verified in the field trials. Therefore, the response of soil microorganisms in the field is necessarily investigated, which is valuable to evaluate the actual effects of biochar on the soil properties and plant growth (Saifullah et al., 2018).
As a major source of vegetable oil and protein, peanut (Arachis hypogaea L.) is cultivated and consumed worldwide (Xu et al., 2020). In recent years, peanut with high tolerance to saline has been tried cultivated in saline-affected soil (Wan et al., 2014). However, adverse conditions (for example, nutrient deficiency) in salt-affected soil still severely limited peanut’s growth and rhizosphere microbial community (El-Akhal et al., 2013; Meena et al., 2016). Therefore, to stimulate soil microorganisms in the peanut field is of great significance for the rhizosphere environment and the growth of peanuts using appropriate soil amendments. This study aimed to evaluate the response of microbial diversity and composition in the peanut-planted soil of YRD on the biochar amendment, and explore the involved mechanism. A field trial was conducted to determine the effects of corn-straw biochar with different amounts on 1) nutrient status and physicochemical characteristics of coastal saline soil. 2) the bacterial and fungal abundances and communities of coastal saline soil; We hypothesize that an appropriate amount of biochar could improve soil physicochemical properties, and shape soil bacterial and fungal communities which benefit the productivity of coastal saline soil in YRD.
Materials and Methods
Study Area, Soil Properties, and Plot Design
A field trial was conducted in the coastal salt-affected soil of Dongying City (37°18′N, 118°37′E). The soil has a moderately salt content (2.0‰–3.0‰) that is representative of farmland for peanut production in the YRD. The typical properties of the coastal salt-affected soil are as follows: pH 7.4, electrical conductivity (EC) 561.17 μs/cm, total carbon (TC) 3.58%, total nitrogen (TN) 0.55%, Olsen-P 3.42 mg/kg, available potassium (AK) 520.40 mg/kg, organic matter 35.08 g/kg, WHC 59.10%. Corn-straw biochar produced via pyrolysis at 450°C in an oxygen-free atmosphere (Chen et al., 2019) was used in the field trials. The properties of biochar are shown in Supplementary Table S1. Peanut (Arachis hypogaea L.) seeds of Huayu-25, with relatively high salt tolerance (Zhang et al., 2020) were obtained from the Shandong Peanut Research Institute.
The biochar was added at 0, 18, and 36 t/ha (referred to as CK, LBC, and HBC) to the topsoil (0–20 cm), according to Brtnicky et al. (2021), which was mixed evenly with the soil through rotary tillage before sowing. Each treatment contained six replications (plots), and each plot in the field trial was 4 m long and 2.5 m wide. All plots in the field were randomized. Peanut seeds were mixed with pesticides (Imidacloprid), followed by sowing, and the planting density was 100 kg/ha with 30 × 15 cm plant spacing (Rahman et al., 2021) with no fertilizer application. Peanut was sowed on 05 May 2021 and harvested on 15 September 2021.
Soil Sample Collection and Analysis
Eight representative plant samples were collected from each plot at the harvest stage. Soils tightly adhering to peanut roots were sampled as rhizosphere soils using the hand-shaking method (Liu et al., 2019). The air-dried rhizosphere soils were sieved (2-mm mesh) to measure the physicochemical properties. WHC was obtained by determining the weight loss of water absorbed by the soil (He et al., 2020). Soil samples were extracted by deionized water at a ratio of 1:5 (w/v), then pH and EC were determined using a pH meter and conductivity meter (Bello et al., 2021). Soil samples were extracted by cobalt 6-aminochloride at a 7:100 (w/v) ratio, and cation exchange capacity (CEC) was calculated according to the OD472nm value (Aran et al., 2008). Soil samples were extracted by 1.0 M NH4OAc (pH 7.0) at a 1:10 (w/v) ratio, and the contents of AK and exchangeable Na (Ex-Na) were determined with a flame atomic absorption spectrophotometer (Tang et al., 2020). The exchangeable sodium percentage (ESP) (Costa-Gutierrez et al., 2020) was calculated as Ex-Na divided by CEC. Soil organic matter (SOM) content was determined according to the wet oxidation method of K2Cr2O7-H2SO4 (Xiao et al., 2020). The contents of TC and TN were measured by an element analyzer (Zhao et al., 2020). Soil samples were extracted by 1 M KCl at a 1:5 (w/v) ratio, and the contents of NH4+-N and NO3−-N were determined by an autoanalyzer (Xiao et al., 2020). Soil samples were extracted by 0.5 M NaHCO3 at a 1:20 (w/v) ratio, and the Olsen-P content was also determined by an autoanalyzer (Xiao et al., 2020).
Rhizosphere soil samples stored at −80°C were used to determine the microbial community compositions. Total DNA was extracted from 0.5 g rhizosphere soil using the E. Z.N.A.® soil DNA Kit (Omega Bio-tek, Norcross, GA, US.), which was checked by NanoDrop spectrophotometer (ND2000, Thermo Fisher Scientific) and 1.0% agarose gel electrophoresis, respectively to obtain the concentration and integrity. The V3-V4 region of the bacterial 16S rRNA genes was amplified by polymerase chain reaction (PCR) using primers of 338F (5′-ACTCCTACGGGAGGCAGCAG-3′) and 806R (5′-GGACTACHVGGGTWTCTAAT-3′) (Tang et al., 2020). Fungal rRNA genes were amplified with primer pairs of ITS1F 5′-CTTGGTCATTTAGAGGAAGTAA-3′ and ITS2R 5′-GCTGCGTTCTTCATCGATGC-3′ (Huang et al., 2021). The PCR mixtures and procedures were according to those reported by Li et al. (2017). The PCR products were checked with 2% agarose gel, AxyPrep DNA Gel Extraction Kit (Axygen Biosciences, United States), and QuantiFluor-ST (Promega, United States), respectively. The qualified genes were sequenced on the Illumina MiSeq platform by Majorbio Bio-Pharm Technology Co., Ltd. (Shanghai, China).
Moreover, the main stem height, number of lateral branches, and lateral branch length of the peanut plant were recorded. The plant samples were washed and separated into shoots, roots, and pods, followed by heating at 105°C for 30 min, and dried to a constant weight at 85°C as dry weight (Zhang et al., 2020). All pods of plants in each plot were separated from the straw and then air-dried, and the pod weight was used to calculate yield.
Statistical Analysis
Statistical analysis and figures were carried out using SPSS (20.0, IBM, United States) and Origin 2018 (OriginLab Corp., Northampton, MA, United States), respectively. One-way analysis of variance (ANOVA) with Duncan’s multiple range test was used to test the differences between treatments at p < 0.05. The quality-filtered sequences were clustered (with 97% similarity) into operational taxonomic units (OTUs) by UPARSE 7.1 (Wei et al., 2020). The alpha diversity was calculated according to the methods reported by Zeng et al. (2020). The impacts of biochar on bacterial and fungal community compositions were evaluated using principal coordinate analysis (PCoA) based on the Bray–Curtis distances. Soil bacterial and fungal networks were constructed by calculating multiple correlations and similarities with co-occurrence network (CoNet) inference. A valid co-occurrence (correlation threshold >0.9, p < 0.01) was considered a statistically robust correlation between taxa. Gephi software was used for network visualization (Chen et al., 2019). The spearman correlation heatmaps were conducted on the online platform of Majorbio i-Sanger Cloud Platform (https://cloud.majorbio.com).
Results
Effects of Biochar on Soil Physicochemical Properties
Table 1 exhibits the effects of the biochar on soil physicochemical properties and nutrient states. Compared with the control (CK), biochar applied at 18 t/ha significantly increased CEC, NH4+-N, NO3−-N, and AK by 23.10, 10.25, 15.33, and 17.32% (p < 0.05), but significantly decreased EC and Ex-Na content by 29.96 and 18.75% (p < 0.05), respectively. When biochar was applied at a high rate (36 t/ha), the CEC, TC, NH4+-N, NO3−-N, SOM, Olsen-P, and AK contents were significantly increased by 22.82, 33.82, 23.98, 29.26, 25.78, 20.93, and 41.37% (p < 0.05), but EC, Ex-Na and ESP were significantly decreased by 31.68, 34.38 and 51.88% (p < 0.05), respectively. In addition, WHC, TN, and SOM contents were increased with biochar amendments at two levels of 18 t/ha and 36 t/ha, although not significantly. The soil pH was little affected by biochar amendment at both levels.
Effects of Biochar on Soil Microbial Diversities and Community Compositions
HBC amendment significantly increased bacterial alpha diversity estimated by the Shannon index (Figure 1A) compared with CK. Fungal alpha diversity was not significantly affected by biochar amendments and was estimated by both the Shannon index (Figure 1C) and Chao1 richness (Figure 1D). The principal coordinate analysis (PCoA) of Bray–Curtis distances displayed that both bacterial and fungal community compositions did not significantly (p = 0.33 for bacteria and p = 0.45 for fungi) separate by the first and second principal coordinates under CK, LBC, and HBC treatments (Figures 1E,F).
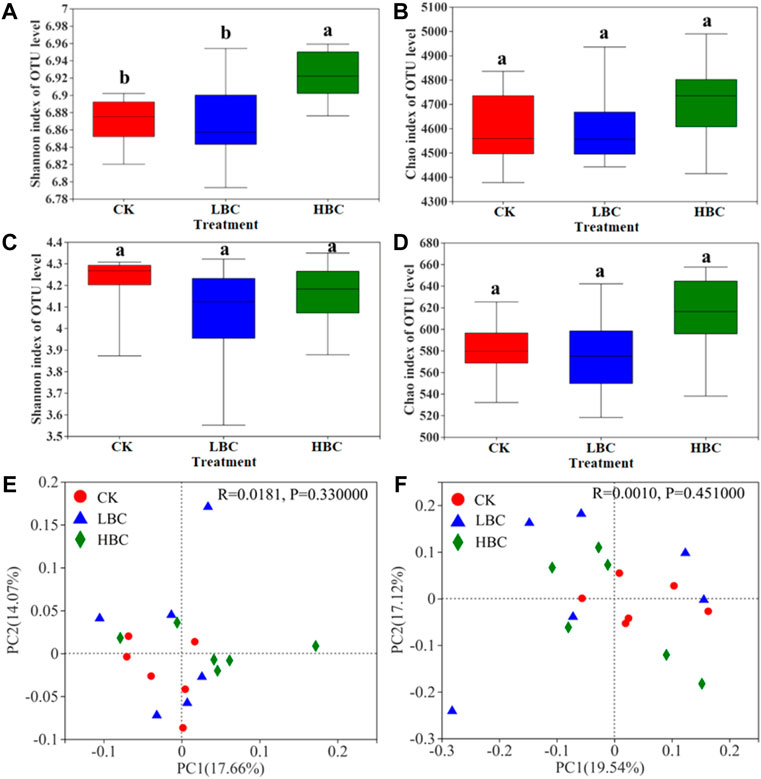
FIGURE1. Bacterial and fungal diversity (at the OUT level) of the coastal saline soil with or without biochar amendment. Alpha diversity was revealed by the Shannon index for bacteria (A) and fungi (C), and Chao one index for bacteria (B) and fungi (D). Bars (n = 6) with different letters show significant difference (p < 0.05). Beta diversity of bacteria (E) and fungi (F) was revealed by PCoA based on the Bray–Curtis distance. CK, soil without biochar amendment; LBC, soil amended with a low amount of biochar at 18 t/ha; HBC, soil amended with a high amount of biochar at 36 t/ha.
At the phylum level, the bacterial community compositions were dominated by Proteobacteria (relative abundances of 25.02%–27.03%), Actinobacteriota (20.52%–22.04%), and Acidobacteriota (13.44%–15.36%) under CK, LBC and HBC treatments (Figure 2A). Among the top 15 abundant phyla, Entotheonellaeota, Nitrospirota, and Bdellovibrionota abundances showed significant differences among the three treatments. To be specific, Nitrospirota and Bdellovibrionota abundances were enhanced under HBC treatment relative to CK and LBC treatments (Figure 2C). At the genus level, the bacterial community compositions were dominated by f__Geminicoccaceae (4.83%–5.49%), o__Vicinamibacterales (3.66%–4.26%), and f__Vicinamibacteraceae (3.37%–4.02%) under CK, LBC and HBC treatments (Figure 2B). Sphingomonas, f__Xanthobacteraceae, and Nannocystis were more abundant in biochar-amended soils than that in the CK soil, and the relative abundances of these three genera were significantly increased by HBC treatment (Figure 2D).
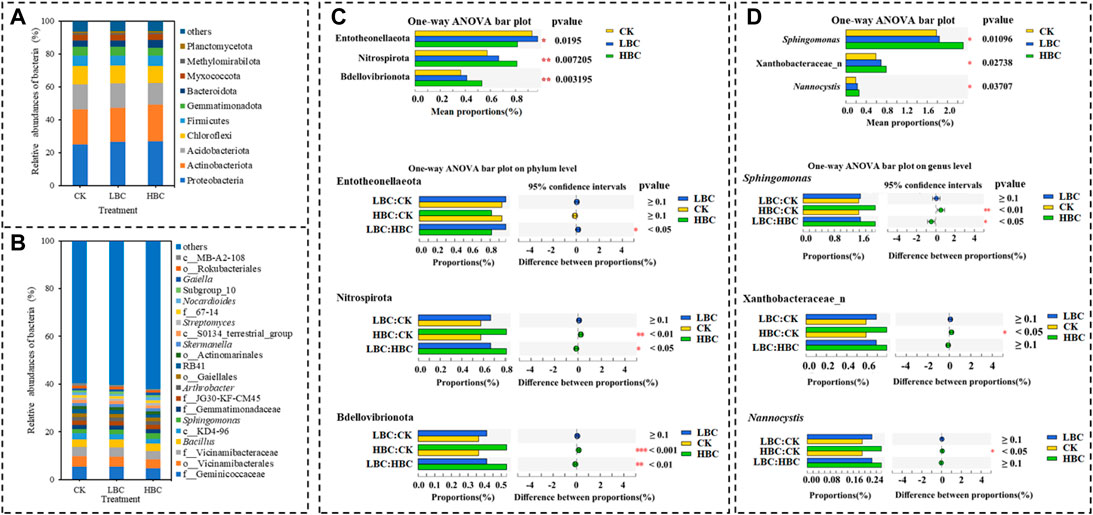
FIGURE 2. Bacterial community of the coastal saline soil with or without biochar amendment. The relative abundance of bacterial phyla (A) and genera (B), and differentially abundant bacterial phyla (C) and genera (D) according to one-way ANOVA. Others refer to bacteria and fungi with a relative abundance lower than 1%. The significances (n = 6) are marked with asterisks (*0.01 ≤ p < 0.05, **0.001 ≤ p < 0.01, ***p < 0.001). CK, soil without biochar amendment; LBC, soil amended with a low amount of biochar at 18 t/ha; HBC, soil amended with a high amount of biochar at 36 t/ha.
When considering the relative fungal abundances, Ascomycota (81.93%–83.92%), Mortierellomycota (7.76%–8.72%) and Basidiomycota (3.09%–4.68%) were the most dominant phyla in both CK and biochar-amended soils (Figure 3A), but the abundances of these phyla showed no differences among CK, LBC, and HBC treatments. Gibberella (10.13%–14.27%), Mortierella (6.92%–8.24%) and Metarhizium (5.83%–8.99%) were the most dominant genera in both CK soil and biochar-amended soils (Figure 3B). Among the top 15 abundant genera, Acrostalagmus abundance was significantly increased by HBC treatment, but Sporormiella abundance was significantly decreased by LBC and HBC treatments (Figure 3C).
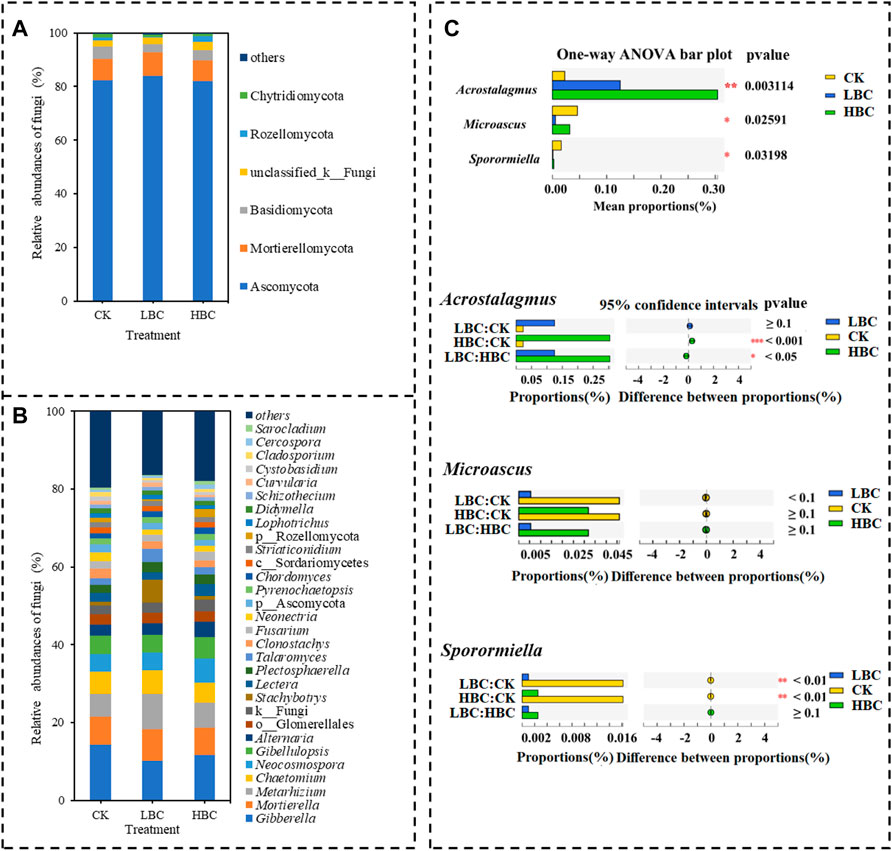
FIGURE 3. Fungal community of the coastal saline soil with or without biochar amendment. The relative abundance of fungal phyla (A) and genera (B), and differentially abundant fungal genera (C) according to one-way ANOVA. Others refer to bacteria and fungi with a relative abundance lower than 1%. The significances (n = 6) are marked with asterisks (**0.01 ≤ p < 0.05, **0.001 ≤ p < 0.01, ***p < 0.001). CK, soil without biochar amendment; LBC, soil amended with a low amount of biochar at 18 t/ha; HBC, soil amended with a high amount of biochar at 36 t/ha.
Effects of Biochar on Soil Bacterial and Fungal Co-Occurrence Networks
Biochar amendments altered the multiple topological properties of both bacterial and fungal co-occurrence patterns of networks (Supplementary Table S2 and Figures 4, 5). Biochar amendments increased positive correlations and average network distance of both bacterial and fungal networks. In the case of bacteria, other than the clustering coefficient, all the indexes were increased under LBC treatment. However, the ratios of negative correlations to positive correlations, modularity index, and clustering coefficient were decreased under HBC treatment relative to CK. In the case of fungi, the ratios of negative correlations to positive correlations, modularity index, and clustering coefficient were decreased under biochar treatment, especially LBC. Both bacterial and fungal networks were decomposed into smaller coherent modules regardless of treatments, and five dominant modules were colored. For the bacterial network, genera including Skermanella, o__CCD24 and Acidibacter under CK treatment, genera including o__Rokubacteriales, f__TRA3-20, and Sphingomonas under LBC treatment and genera including Iamia, Marmoricola, Skermanella, and f__Ilumatobacteraceae under HBC treatment were identified as the module hubs, which showed positive relationships with connected members in their individual module (Figure 4B). For the fungal network, genera including Podospora, Tausonia, Kotlabaea, and Stagonospora under CK treatment, genera Podospora under LBC treatment, and genera including Mortierella and Glomerellales under HBC treatment were designated as the module hubs, and these detected keystone taxa were positively relevant to the linked nodes in their own module (Figure 5B).
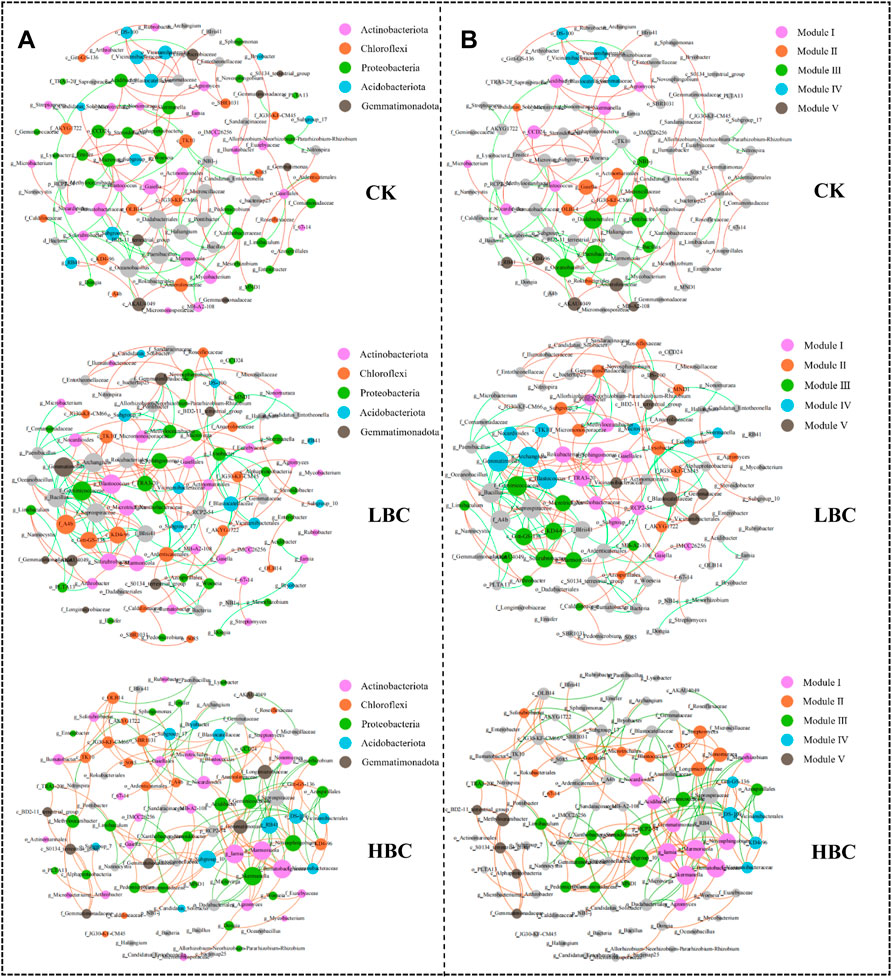
FIGURE 4. The bacterial co-occurrence networks (at the genus level) based on correlation analysis under the non-amended and biochar-amended treatments. The visualized connections reveal strong (Spearman’s p > 0.9) and significant (p value <0.01) correlations. Bacterial networks are colored by phyla (A) and modules (B), respectively. The modules I–V in the networks stand for the five clusters of closely interconnected nodes. The node size is proportional to the connection number (degree), and the connection thickness is proportional to Spearman’s correlation coefficient values. The red and green edges indicate positive and negative interactions between two nodes, respectively. The keystone taxa were identified according to the module hubs. CK, soil without biochar amendment; LBC, soil amended with a low amount of biochar at 18/t ha; HBC, soil amended with a high amount of biochar at 36 t/ha.
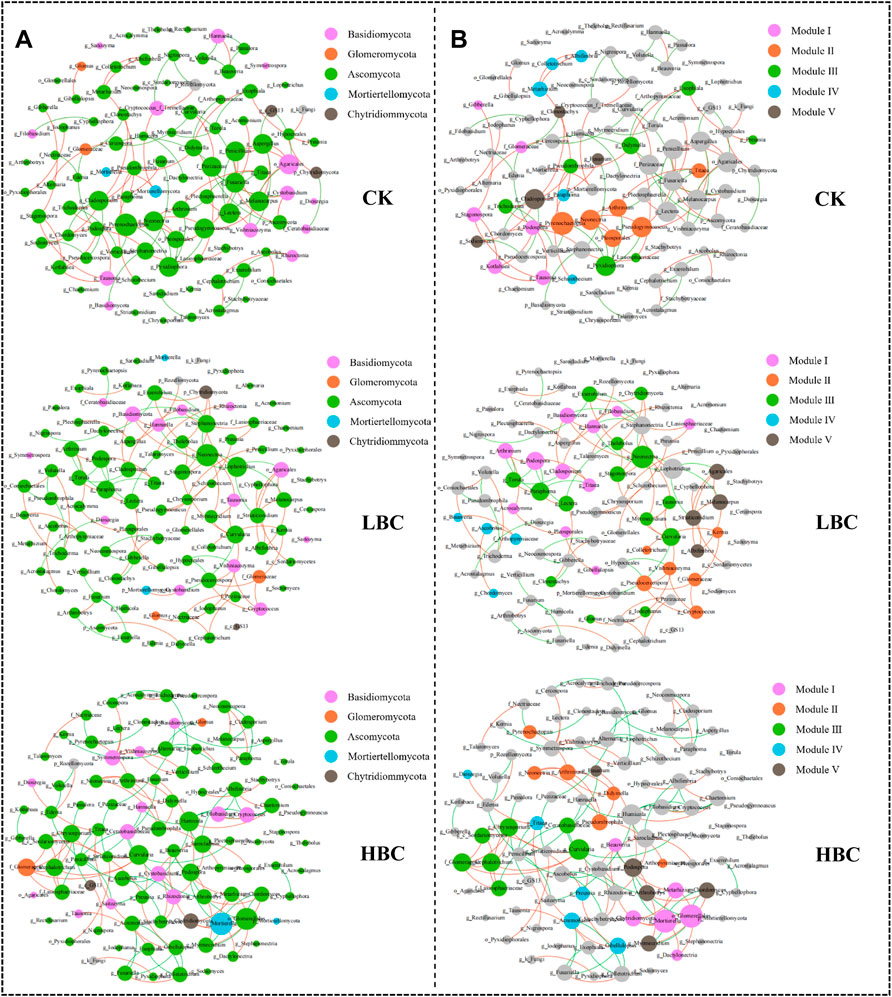
FIGURE 5. The fungal co-occurrence networks (at the genus level) based on correlation analysis. The visualized connections reveal strong (Spearman’s p > 0.9) and significant (p value <0.01) correlations. Fungal networks are colored by phyla (A) and modules (B), respectively. The modules I–V in the networks stand for the five clusters of closely interconnected nodes. The node size is proportional to the connection number (degree), and the connection thickness is proportional to Spearman’s correlation coefficient values. The red and green edges indicate positive and negative interactions between two nodes, respectively. The keystone taxa were identified according to the module hubs. CK, soil without biochar amendment; LBC, soil amended with a low amount of biochar at 18 t/ha; HBC, soil amended with a high amount of biochar at 36 t/ha.
Relationships Between Soil Microbial Communities and Soil Properties
The top 30 abundant bacterial genera were separated into two clusters based on the correlations with soil physicochemical properties (Figure 6A). One cluster contained some taxa such as Bacillus and c_AKAU4049, of which most taxa were positively correlated with EC, Ex-Na, and ESP, but were positive with TC, NO3−-N, WHC, C/N, CEC, and AK. Another cluster containing some taxa, such as f__Gemmatimonadaceae, Sphingomonas, and f__Xanthobacteraceae were negatively correlated with EC, Ex-Na, and ESP, but positive with TC, NO3−-N, WHC, C/N, CEC, and AK. In the case of fungi, no obvious clusters were observed based on the correlation analysis (Figure 6B).
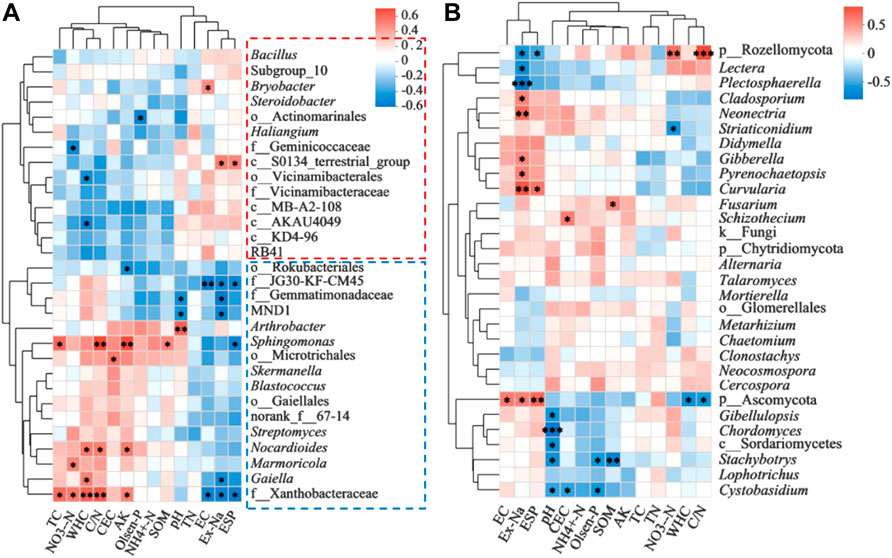
FIGURE 6. Spearman correlation heatmaps revealing the correlations of soil physicochemical properties and the relative abundances of top 30 dominant genera of bacteria (A) and fungi (B). *0.01 ≤ p < 0.05, **0.001 ≤ p < 0.01, ***p < 0.001.
Discussion
Biochar Amendments Improved Soil Physicochemical Properties and Nutrient Content
In our study, both LBC and HBC significantly decreased soil EC which could directly reflect soil salt concentration (Zhang et al., 2015). Biochar could release K+, Ca2+, and Mg2+ to the soil, which could exchange with Na+ in soil (displaced Na+ on the soil particles). Consequently, Na+ leaching and its activity in plants were reduced (Usman et al., 2016; Zheng et al., 2018). In the present study, the contents of some elements (i.e., K, Ca, Mg, Fe, and Mn) in the biochar were higher than 20 mg/kg (Supplementary Table S1), which implies that corn-straw biochar has great potential to increase the essential element contents of soil for plant growth and displace more Na+ at the same time, which decreased soil EC in the biochar-amended soils (Table 1). In the present study, biochar, especially LBC significantly increased soil CEC. On the one hand, biochar-amended soil increased the potential to trap nutrients (i.e., K+, Ca2+, and Mg2+) and reduce nutrient loss because biochar with porous structure, high surface area, abundant hydroxyl groups, and charges has strong adsorption ability of these nutrients (Zhao et al., 2020). Yuan et al. (2019) reported that about 20%–70% of CEC of soils can be contributed by SOM, because biochar has a high adsorption capacity of organic molecules in the soil, and the absorbed small organic molecules on the biochar can form organic matter via surface catalytic activity (polymerization) on the biochar surface. The generalized organic matter not only promotes the long-term fertility of the soil but also provides a certain buffer effect and delays the return of salt to the soil.
LBC and HBC significantly increased the contents of NH4+-N, NO3−-N, and AK in the coastal saline soil, which might be because biochar can directly act as fertilizer that provides these nutrients for plant growth (Agegnehu et al., 2017), or alter nutrient availability in soil (Mavi et al., 2018). For example, biochar was reported to supply high amounts of available K to plants (Xu et al., 2013). Likewise, Gul and Whalen (2016) reported that biochar increased the availability of K and other essential nutrients in the soil that are conductive to plant growth. The increased contents of NH4+-N and NO3−-N might be because biochar affected the nitrification and denitrification processes mediated by nitrifiers and de nitrifiers in soil (Wang et al., 2020). Consequently, except for physicochemical properties and nutrient states, microbial diversity and community structure that are closely correlated to soil quality and plant growth (Kamali et al., 2022) should also be analyzed.
Biochar Amendments Altered Soil Bacterial and Fungal Communities
In the present study, both LBC and HBC promoted soil nutrient contents and physicochemical properties. LBC treatment increased peanut yield by 3.03%, but HBC inhibited peanut yield by 8.58% relative to CK (Supplementary Table S3). Therefore, we speculated that peanut yields are not always positive with the improved soil physicochemical properties, and microbial response play a critical role in the peanut growth and yield. LBC might be beneficial to microbes but HBC might exert adverse effects on soil microbial diversity or communities. In the present study, the increased abundances of some beneficial bacteria such as Sphingomonas, f_Xanthobacteraceae, and Nannocystis that were associated with fixing nitrogen and inhibiting pathogens in LBC amended soil (Figure 3) might contribute to the increased peanut yield (Xu et al., 2020; Moradi et al., 2022). However, Rozellomycota was significantly enriched in HBC treatment (Supplementary Figure S1), which might account for the broken peanut shells and pods, because Rozellomycota is interpreted as an intermediate form between protists and true fungi (Corsaro et al., 2014), which is reported positively correlated with aflatoxin contamination that could induce peanut rot (Yao et al., 2020). The abundance and community structure of microorganisms can be affected by both abiotic factors (i.e., soil physical and chemical properties), and biotic factors (i.e., interactions between microorganisms) (Bello et al., 2021). According to the correlation analysis (Figure 6), we found that the abundances of dominant beneficial bacteria Sphingomonas and f__Xanthobacteraceae were negatively correlated with EC, Ex-Na, and ESP, but positive with TC, NO3−-N, WHC, C/N, CEC, and AK, indicating that these bacteria were sensitive to these soil properties. Therefore, the increased abundances of these bacteria in LBC treatment might be induced by these improved soil properties. However, in the HBC treatment, these physical and chemical properties were improved, and the abundances of these beneficial bacteria were also increased, but the peanut yield was lower than that of the control group. Therefore, we speculate that biotic factors (interactions between microorganisms) may be adversely affected by a high dose of biochar.
The network-based analysis is useful for inferring the keystone taxa of the complex networks and the microbial interactions in natural environments (Weiss et al., 2016). The topological and modular features of microbial networks showed that both LBC and HBC amendments influenced bacterial and fungal network structures (Figures 4, 5 and Supplementary Table S2). Both LBC and HBC treatments increased the average connectivity of the bacterial network, displaying more complex couplings among bacteria, which is similar to the result that biochar increased the complexity of microbial networks reported by Yu et al. (2018). The ratios of negative correlations to positive correlations of the bacterial networks were enhanced under the LBC amendment, illustrating antagonistic or competitive interactions were increased (Chen et al., 2019), but HBC treatment decreased the ratios. Moreover, the modularity index of the HBC treatment was much lower than CK. Keystone taxa can better explain microbiome compositional turnover than all taxa combined (Chen et al., 2019). Some potentially beneficial taxa (i.e., o_Rokubacteriales and f_Xanthobacteraceae) are connected to Sphingomonas (keystone taxa) in the network and constituted module I under LBC treatment (Figure 4). The order Rokubacteriales has the potential for a versatile, mixotrophic metabolism, especially for nitrogen respiration (Fan et al., 2021). About 70%–88% of members of the family Xanthobacteraceae were genus Bradyrhizobium, a critical clade of symbiotic rhizobia that can form nodulation with many kinds of leguminous plants (Chen et al., 2021). The abundance of Sphingomonas was not significantly increased by LBC, but Sphingomonas was associated as potential driver taxa of the complex and healthy bacterial network under LBC treatment, which is beneficial for peanut growth and yield. In the case of fungi, no obvious clusters for fungi were observed (Figure 6B), which might be because the relationships among the abundant bacteria are closer than that of fungi, which can also be explained by the indexes in Supplementary Table S2, where the edge number and clustering coefficient of bacteria were higher than those of fungi in both CK and biochar-amended soil samples. Consequently, the analysis of the interactions between microbial taxa might be a powerful and necessary method to understand the relationships between rhizosphere microorganisms and plant growth.
Conclusion
Both HBC and LBC treatments efficiently improved the physicochemical properties of the degraded coastal soil. The bacterial community is more sensitive to biochar amendments relative to the fungal community. HBC treatment significantly improved bacterial diversity, but decreased the competitive interactions and modularity of the bacterial co-occurrence network. On the contrary, LBC treatment increased the competitive interactions and modularity of the bacterial co-occurrence network. LBC increased peanut yield, but HBC reduced peanut yield, although not significantly. Our study highlighted the important role of the bacterial interactions in the rhizosphere bacterial community, which is critical for crop growth and yield. The interaction between microorganisms plays a non-ignorable role in rhizosphere microecology and soil quality. It is encouraged to comprehensively consider both biotic and abiotic responses when evaluating the effects of soil amendments on the soil environment.
Data Availability Statement
The original contributions presented in the study are included in the article/Supplementary Materials; further inquiries can be directed to the corresponding authors.
Author Contributions
RS and XY initiated the concept and designed the study. YC, DG, and FS have been responsible for the sampling and data analysis. BW and YL supervised the study and contributed to the article. All authors contributed to the article and approved the submitted version.
Funding
This research was funded by the Shandong Provincial Natural Science Foundation (ZR2021QD083), Postdoctoral Innovation Project of Shandong Province (202101021) and Qingdao Postdoctoral Applied Research Project, the Agricultural Science and Technology Innovation Program of China (ASTIP-TRIC06), and the Agricultural Science and Technology Innovation Program (ASTIP No. CAAS-ZDRW202201).
Conflict of Interest
The authors declare that the research was conducted in the absence of any commercial or financial relationships that could be construed as a potential conflict of interest.
Publisher’s Note
All claims expressed in this article are solely those of the authors and do not necessarily represent those of their affiliated organizations, or those of the publisher, the editors, and the reviewers. Any product that may be evaluated in this article, or claim that may be made by its manufacturer, is not guaranteed or endorsed by the publisher.
Supplementary Material
The Supplementary Material for this article can be found online at: https://www.frontiersin.org/articles/10.3389/fenvs.2022.924358/full#supplementary-material
References
Agegnehu, G., Srivastava, A. K., and Bird, M. I. (2017). The Role of Biochar and Biochar-Compost in Improving Soil Quality and Crop Performance: A Review. Appl. Soil Ecol. 119, 156–170. doi:10.1016/j.apsoil.2017.06.008
Akhtar, S. S., Li, G., Andersen, M. N., and Liu, F. (2014). Biochar Enhances Yield and Quality of Tomato under Reduced Irrigation. Agr. Water Manage. 138, 37–44. doi:10.1016/j.agwat.2014.02.016
Aran, D., Maul, A., and Masfaraud, J.-F. (2008). A Spectrophotometric Measurement of Soil Cation Exchange Capacity Based on Cobaltihexamine Chloride Absorbance. CR Geosci. 340, 865–871. doi:10.1016/j.crte.2008.07.015
Bello, A., Wang, B., Zhao, Y., Yang, W., Ogundeji, A., Deng, L., et al. (2021). Composted Biochar Affects Structural Dynamics, Function and Co-occurrence Network Patterns of Fungi Community. Sci. Total Environ. 775, 145672. doi:10.1016/j.scitotenv.2021.145672
Bhaduri, D., Saha, A., Desai, D., and Meena, H. N. (2016). Restoration of Carbon and Microbial Activity in Salt-Induced Soil by Application of Peanut Shell Biochar during Short-Term Incubation Study. Chemosphere 148, 86–98. doi:10.1016/j.chemosphere.2015.12.130
Brtnicky, M., Datta, R., Holatko, J., Bielska, L., Gusiatin, Z. M., Kucerik, J., et al. (2021). A Critical Review of the Possible Adverse Effects of Biochar in the Soil Environment. Sci. Total Environ. 796, 148756. doi:10.1016/j.scitotenv.2021.148756
Chen, L., Jiang, Y., Liang, C., Luo, Y., Xu, Q., Han, C., et al. (2019). Competitive Interaction with Keystone Taxa Induced Negative Priming under Biochar Amendments. Microbiome 7, 77. doi:10.1186/s40168-019-0693-7
Chen, W.-C., Ko, C.-H., Su, Y.-S., Lai, W.-A., and Shen, F.-T. (2021). Metabolic Potential and Community Structure of Bacteria in an Organic Tea Plantation. Appl. Soil Ecol. 157, 103762. doi:10.1016/j.apsoil.2020.103762
Corsaro, D., Walochnik, J., Venditti, D., Müller, K.-D., Hauröder, B., and Michel, R. (2014). Rediscovery of Nucleophaga Amoebae, a Novel Member of the Rozellomycota. Parasitol. Res. 113, 4491–4498. doi:10.1007/s00436-014-4138-8
Costa-Gutierrez, S. B., Raimondo, E. E., Lami, M. J., Vincent, P. A., Espinosa-Urgel, M., and de Cristóbal, R. E. (2020). Inoculation of Pseudomonas Mutant Strains Can Improve Growth of Soybean and Corn Plants in Soils under Salt Stress. Rhizosphere 16, 100255. doi:10.1016/j.rhisph.2020.100255
El-Akhal, M. R., Rincón, A., de la Peña, T. C., Lucas, M. M., El Mourabit, N., Barrijal, S., et al. (2013). Effects of Salt Stress and Rhizobial Inoculation on Growth and Nitrogen Fixation of Three Peanut Cultivars. Plant Biol. 15, 415–421. doi:10.1111/j.1438-8677.2012.00634.x
Fan, J., Jin, H., Zhang, C., Zheng, J., Zhang, J., and Han, G. (2021). Grazing Intensity Induced Alternations of Soil Microbial Community Composition in Aggregates Drive Soil Organic Carbon Turnover in a Desert Steppe. Agr. Ecosyst. Environ. 313, 107387. doi:10.1016/j.agee.2021.107387
Gul, S., and Whalen, J. K. (2016). Biochemical Cycling of Nitrogen and Phosphorus in Biochar-Amended Soils. Soil Biol. Biochem. 103, 1–15. doi:10.1016/j.soilbio.2016.08.001
He, K., He, G., Wang, C., Zhang, H., Xu, Y., Wang, S., et al. (2020). Biochar Amendment Ameliorates Soil Properties and Promotes Miscanthus Growth in a Coastal Saline-Alkali Soil. Appl. Soil Ecol. 155, 103674. doi:10.1016/j.apsoil.2020.103674
Herren, C. M., and McMahon, K. D. (2018). Keystone Taxa Predict Compositional Change in Microbial Communities. Environ. Microbiol. 20, 2207–2217. doi:10.1111/1462-2920.14257
Huang, B., Jia, H., Han, X., Gou, J., Huang, C., Wang, J., et al. (2021). Effects of Biocontrol Bacillus and Fermentation Bacteria Additions on the Microbial Community, Functions and Antibiotic Resistance Genes of Prickly Ash Seed Oil Meal-Biochar Compost. Bioresour. Technol. 340, 125668. doi:10.1016/j.biortech.2021.125668
Kamali, M., Sweygers, N., Al-Salem, S., Appels, L., Aminabhavi, T. M., and Dewil, R. (2022). Biochar for Soil Applications-Sustainability Aspects, Challenges and Future Prospects. Chem. Eng. J. 428, 131189. doi:10.1016/j.cej.2021.131189
Li, J., Huang, B., Wang, Q., Li, Y., Fang, W., Han, D., et al. (2017). Effects of Fumigation with Metam-Sodium on Soil Microbial Biomass, Respiration, Nitrogen Transformation, Bacterial Community Diversity and Genes Encoding Key Enzymes Involved in Nitrogen Cycling. Sci. Total Environ. 598, 1027–1036. doi:10.1016/j.scitotenv.2017.02.058
Li, X., Xia, J., Zhao, X., and Chen, Y. (2019). Effects of Planting Tamarix Chinensis on Shallow Soil Water and Salt Content under Different Groundwater Depths in the Yellow River Delta. Geoderma 335, 104–111. doi:10.1016/j.geoderma.2018.08.017
Liu, B., Cai, Z., Zhang, Y., Liu, G., Luo, X., and Zheng, H. (2019). Comparison of Efficacies of Peanut Shell Biochar and Biochar-Based Compost on Two Leafy Vegetable Productivity in an Infertile Land. Chemosphere 224, 151–161. doi:10.1016/j.chemosphere.2019.02.100
Luo, X., Liu, G., Xia, Y., Chen, L., Jiang, Z., Zheng, H., et al. (2016a). Use of Biochar-Compost to Improve Properties and Productivity of the Degraded Coastal Soil in the Yellow River Delta, China. J. Soils Sediments 17, 780–789. doi:10.1007/s11368-016-1361-1
Luo, X., Wang, L., Liu, G., Wang, X., Wang, Z., and Zheng, H. (2016b). Effects of Biochar on Carbon Mineralization of Coastal Wetland Soils in the Yellow River Delta, China. Ecol. Eng. 94, 329–336. doi:10.1016/j.ecoleng.2016.06.004
Mavi, M. S., Singh, G., Singh, B. P., Singh Sekhon, B., Choudhary, O. P., Sagi, S., et al. (2018). Interactive Effects of Rice-Residue Biochar and N-Fertilizer on Soil Functions and Crop Biomass in Contrasting Soils. J. soil Sci. Plant Nut. 18, 41–59. doi:10.4067/s0718-95162018005000201
Meena, H. N., Meena, M., and Yadav, R. S. (2016). Comparative Performance of Seed Types on Yield Potential of Peanut ( Arachis hypogaea L.) under Saline Irrigation. Field Crops Res. 196, 305–310. doi:10.1016/j.fcr.2016.06.006
Moradi, A., Yaghoubi-Avini, M., and Wink, J. (2022). Isolation of Nannocystis Species from Iran and Exploring Their Natural Products. Arch. Microbiol. 204, 123. doi:10.1007/s00203-021-02738-0
Rahman, M. A., Jahiruddin, M., Kader, M. A., Islam, M. R., and Solaiman, Z. M. (2021). Sugarcane Bagasse Biochar Increases Soil Carbon Sequestration and Yields of Maize and Groundnut in Charland Ecosystem. Archives Agron. Soil Sci., 1–14. doi:10.1080/03650340.2021.1892651
Saifullah, , Dahlawi, S., Naeem, A., Rengel, Z., and Naidu, R. (2018). Biochar Application for the Remediation of Salt-Affected Soils: Challenges and Opportunities. Sci. Total Environ. 625, 320–335. doi:10.1016/j.scitotenv.2017.12.257
Tang, J., Zhang, S., Zhang, X., Chen, J., He, X., and Zhang, Q. (2020). Effects of Pyrolysis Temperature on Soil-Plant-Microbe Responses to Solidago Canadensis L.-derived Biochar in Coastal Saline-Alkali Soil. Sci. Total Environ. 731, 138938. doi:10.1016/j.scitotenv.2020.138938
Usman, A. R. A., Al-Wabel, M. I., Ok, Y. S., Al-Harbi, A., Wahb-Allah, M., El-Naggar, A. H., et al. (2016). Conocarpus Biochar Induces Changes in Soil Nutrient Availability and Tomato Growth under Saline Irrigation. Pedosphere 26, 27–38. doi:10.1016/s1002-0160(15)60019-4
Wan, L., Wu, Y., Huang, J., Dai, X., Lei, Y., Yan, L., et al. (2014). Identification of ERF Genes in Peanuts and Functional Analysis of AhERF008 and AhERF019 in Abiotic Stress Response. Funct. Integr. Genomics 14, 467–477. doi:10.1007/s10142-014-0381-4
Wang, L., Yang, K., Gao, C., and Zhu, L. (2020). Effect and Mechanism of Biochar on CO2 and N2O Emissions under Different Nitrogen Fertilization Gradient from an Acidic Soil. Sci. Total Environ. 747, 141265. doi:10.1016/j.scitotenv.2020.141265
Wei, G., Li, M., Shi, W., Tian, R., Chang, C., Wang, Z., et al. (2020). Similar Drivers but Different Effects Lead to Distinct Ecological Patterns of Soil Bacterial and Archaeal Communities. Soil Biol. Biochem. 144, 107759. doi:10.1016/j.soilbio.2020.107759
Weiss, S., Van Treuren, W., Lozupone, C., Faust, K., Friedman, J., Deng, Y., et al. (2016). Correlation Detection Strategies in Microbial Data Sets Vary Widely in Sensitivity and Precision. ISME J. 10, 1669–1681. doi:10.1038/ismej.2015.235
Xiao, L., Yuan, G., Feng, L., Bi, D., and Wei, J. (2020). Soil Properties and the Growth of Wheat (Triticum aestivum L.) and Maize (Zea mays L.) in Response to Reed (Phragmites Communis) Biochar Use in a Salt-Affected Soil in the Yellow River Delta. Agric. Ecosyst. Environ. 303, 107124. doi:10.1016/j.agee.2020.107124
Xu, G., Wei, L. L., Sun, J. N., Shao, H. B., and Chang, S. X. (2013). What Is More Important for Enhancing Nutrient Bioavailability with Biochar Application into a Sandy Soil: Direct or Indirect Mechanism? Ecol. Eng. 52, 119–124. doi:10.1016/j.ecoleng.2012.12.091
Xu, Y., Yu, Z., Zhang, D., Huang, J., Wu, C., Yang, G., et al. (2018). CYSTM, a Novel Non-secreted Cysteine-Rich Peptide Family, Involved in Environmental Stresses in Arabidopsis thaliana. Plant Cell Physiol. 59, 423–438. doi:10.1093/pcp/pcx202
Xu, Y., Yu, Z., Zhang, S., Wu, C., Yang, G., Yan, K., et al. (2019). CYSTM3 Negatively Regulates Salt Stress Tolerance in Arabidopsis. Plant Mol. Biol. 99, 395–406. doi:10.1007/s11103-019-00825-x
Xu, Y., Zhang, G., Ding, H., Ci, D., Dai, L., and Zhang, Z. (2020). Influence of Salt Stress on the Rhizosphere Soil Bacterial Community Structure and Growth Performance of Groundnut (Arachis hypogaea L.). Int. Microbiol. 23, 453–465. doi:10.1007/s10123-020-00118-0
Yao, Y., Zhang, Q., Mwakinyali, S. E., Gao, S., Ding, X., Liang, S., et al. (2020). The Peanut Pods-Associated Microbiota and Their Effects on Aflatoxin Contamination. Res. Square. doi:10.21203/rs.2.21090/v1
You, X., Yin, S., Suo, F., Xu, Z., Chu, D., Kong, Q., et al. (2021). Biochar and Fertilizer Improved the Growth and Quality of the Ice Plant (Mesembryanthemum Crystallinum L.) Shoots in a Coastal Soil of Yellow River Delta, China. Sci. Total Environ. 775, 144893. doi:10.1016/j.scitotenv.2020.144893
Yu, J., Deem, L. M., Crow, S. E., Deenik, J. L., and Penton, C. R. (2018). Biochar Application Influences Microbial Assemblage Complexity and Composition Due to Soil and Bioenergy Crop Type Interactions. Soil Biol. Biochem. 117, 97–107. doi:10.1016/j.soilbio.2017.11.017
Yuan, P., Wang, J., Pan, Y., Shen, B., and Wu, C. (2019). Review of Biochar for the Management of Contaminated Soil: Preparation, Application and Prospect. Sci. Total Environ. 659, 473–490. doi:10.1016/j.scitotenv.2018.12.400
Zeng, X.-Y., Li, S.-W., Leng, Y., and Kang, X.-H. (2020). Structural and Functional Responses of Bacterial and Fungal Communities to Multiple Heavy Metal Exposure in Arid Loess. Sci. Total Environ. 723, 138081. doi:10.1016/j.scitotenv.2020.138081
Zhang, G.-c., Dai, L.-x., Ding, H., Ci, D.-w., Ning, T.-y., Yang, J.-s., et al. (2020). Response and Adaptation to the Accumulation and Distribution of Photosynthetic Product in Peanut under Salt Stress. J. Integr. Agric. 19, 690–699. doi:10.1016/s2095-3119(19)62608-0
Zhang, T., Wang, T., Liu, K., Wang, L., Wang, K., and Zhou, Y. (2015). Effects of Different Amendments for the Reclamation of Coastal Saline Soil on Soil Nutrient Dynamics and Electrical Conductivity Responses. Agric. Water Manag. 159, 115–122. doi:10.1016/j.agwat.2015.06.002
Zhao, W., Zhou, Q., Tian, Z., Cui, Y., Liang, Y., and Wang, H. (2020). Apply Biochar to Ameliorate Soda Saline-Alkali Land, Improve Soil Function and Increase Corn Nutrient Availability in the Songnen Plain. Sci. Total Environ. 722, 137428. doi:10.1016/j.scitotenv.2020.137428
Keywords: biochar, coastal soil, physicochemical properties, soil microbial community, microbial network
Citation: Sun R, You X, Cheng Y, Gan D, Suo F, Wang B and Li Y (2022) Response of Microbial Compositions and Interactions to Biochar Amendment in the Peanut-Planted Soil of the Yellow River Delta, China. Front. Environ. Sci. 10:924358. doi: 10.3389/fenvs.2022.924358
Received: 20 April 2022; Accepted: 13 May 2022;
Published: 27 June 2022.
Edited by:
Hao Zheng, Ocean University of China, ChinaReviewed by:
Guocheng Liu, Qingdao Agricultural University, ChinaZhixiang Jiang, Qingdao University, China
Xiaoyun Li, Shaanxi Normal University, China
Copyright © 2022 Sun, You, Cheng, Gan, Suo, Wang and Li. This is an open-access article distributed under the terms of the Creative Commons Attribution License (CC BY). The use, distribution or reproduction in other forums is permitted, provided the original author(s) and the copyright owner(s) are credited and that the original publication in this journal is cited, in accordance with accepted academic practice. No use, distribution or reproduction is permitted which does not comply with these terms.
*Correspondence: Xiangwei You, eW91eGlhbmd3ZWlAY2Fhcy5jbg==; Bo Wang, MTE1MDM0NDY5QHFxLmNvbQ==