- 1Department of Biology & Institute for Great Lakes Research, Central Michigan University, Mount Pleasant, MI, United States
- 2State Climate Office of North Carolina, North Carolina State University, Raleigh, NC, United States
- 3USDA-ARS Pasture Systems and Watershed Management Research Unit, University Park, PA, United States
- 4Department of Civil and Environmental Engineering, Pennsylvania State University, University Park, PA, United States
- 5Department of Biological and Environmental Engineering, Cornell University, Ithaca, NY, United States
Legacy phosphorus concentrations resulting from historic additions of phosphorus (P) to the landscape may impede rapid remediation of P pollution and achievement of water quality management goals. Herein, we hypothesized that the capacity of stream biofilms to assimilate new polyphosphate (polyP) will vary as a function of stream legacy phosphorus. To test this hypothesis, we deployed a series of in situ enrichment experiments at five sites of varying land cover in central Pennsylvania, United States. Incremental P-loading was delivered using vials fitted with porous lids, that contained agar enriched with six levels of P (as Dissolved inorganic phosphorus, dissolved inorganic P) loading with rates ranging from 0 to 1,540 µg PO4−3/day; these loading rates mimicked natural stream P loadings. Substrata were incubated at stream sites for a relatively short incubation period (12 days), to measure uptake rates; after which, biofilms growing on the lids were removed and their tissue content was analyzed for biomass (as chlorophyll) and various forms of particulate phosphorus. Polyphosphate (polyP) accumulated by stream biofilms at all sites closely tracked the release of dissolved inorganic P from experimental enrichment assays. Comparatively, biofilms accumulated relatively small amounts of Particulate inorganic phosphorus and other forms of organic P that we assume constitute a third group of P-rich biochemicals (e.g., DNA, RNA, lipids, proteins). Viewed at the watershed scale, land use appeared to affect P accumulation, where sites dominated by forest cover had a higher capacity for P storage, while sites dominated by agriculture did not; this underscores the importance of polyP storage as an indicator of legacy P pollution.
1 Introduction
Alterations in watershed nutrient loadings have been linked to changes in the water quality of receiving waters in lakes, streams, rivers, and downstream coastal ecosystems (e.g., Carpenter et al., 1998). High loadings of nitrogen (N) and phosphorus (P) to headwater streams has caused these nutrients to be of primary interest, given that both nutrients play a role in regulating primary productivity in aquatic ecosystems throughout the United States, and in the Chesapeake Bay in particular (Correll 1998; Ator and Denver 2012). Watershed sources of N and P enrichment have had a ripple effect on entire landscape segments, where N and P introduced to headwater streams have been transported throughout portions of the watershed and have ultimately enriched coastal habitats (Alexander et al., 2007; Dodds and Oakes 2008). This has been the case in the Chesapeake Bay watershed, United States, where more than 100,000 tributaries feed the Susquehanna River that drains portions of New York, Pennsylvania, and Maryland to deliver the majority of N and P to the bay (Zhang et al., 2013).
Dissolved inorganic phosphorus (DIP), which refers to unbound phosphate (PO4−3) molecules, can be readily incorporated into microbial cells and stored as electron-dense, intracellular chains of two or more phosphate molecules known as polyphosphate (PolyP; e.g., Eixler et al., 2004; Martin et al., 2014). The storage of DIP as PolyP in stream biofilms can reflect current nutrient loading events in streams (Rier et al., 2014), and possibly more chronic or P enrichment that has occurred in the past (i.e., legacy P loading effect, see Carrick and Price 2011; Price and Carrick 2014). Legacy effects exist, because internal P storage ultimately competes with carbon uptake, so that luxury consumption of P can occur (Healey 1979; Lean and Pick 1981). In the initial stages of biofilm colonization when P loading is low, algal cells tend to store new pulses of P beyond their immediate needs as intracellular PolyP bodies (e.g., Sicko-Goad and Lazinsky 1986; Saia et al., 2017). In plants, PolyP serves as a precursor to enhanced photosynthesis (Lean and Pick 1981; Jacobson and Halmann 1982) and subsequent growth of new cells as well as DNA and RNA synthesis.
In this paper, we studied the relationship between instantaneous P loading and P accumulation by enriching natural stream biofilms with N and P administered using a previously described in situ enrichment system (ISES, Carrick and Price 2011). This technique allowed us to administer P release at several, realistic levels of P loading. We hypothesized that PolyP concentration in the tissues of resident biofilms can be assayed to determine the degree of legacy P loading in a stream, and the subsequent capacity of a stream to assimilate new P. We addressed three primary research questions. First, does P accumulation by natural biofilms vary predictably with incremental P loading as delivered by the ISES experiments? Second, what form of particulate P is derived from new DIP, and do the forms of accumulated P form vary as a function of P loading? Third, does biofilm P uptake vary along a natural P loading gradient within a watershed?
2 Methods
2.1 Study Site and Stream Sampling
The study was conducted in streams located within the mid-Atlantic region (Appalachian Valley/Ridge ecoregion of Pennsylvania) in the United States (Carrick et al., 2009). Four sites studied here were located in the FD-36, a small headwater tributary to the Mahantango Creek Watershed, which has been maintained and monitored by the United States Department of Agriculture’s Agriculture Research Service (USDA-ARS) since 1968 (see USDA 2010 Figure 1). An additional single site was studied in the Aughwick Creek Watershed, which served as a control. Both watersheds share similar base geology underlain with a mix of sandstones and shale (see Geyer and Wilshusen 1982). The streams are fifth order tributaries when they discharge into the Susquehanna River, which is a tributary to the Chesapeake Bay along the Atlantic coastline of continental United States.
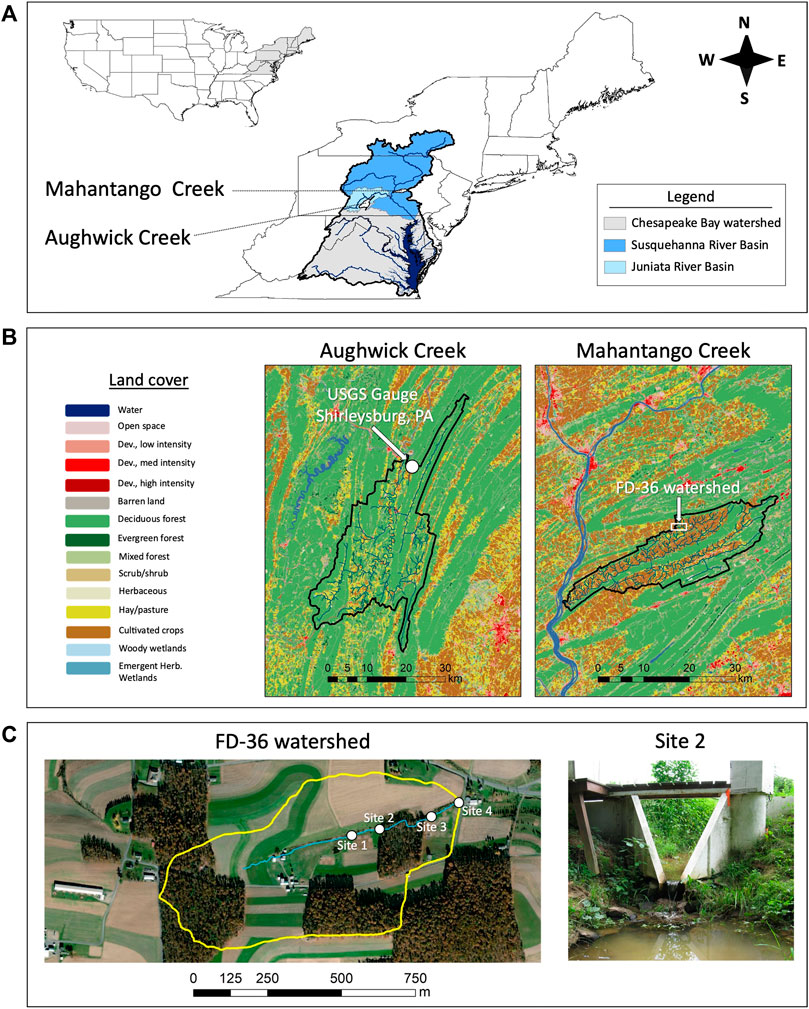
FIGURE 1. Three-panel figure showing the two study watersheds and five sampling locations. Panel (A) depicts the locations of Aughwick Creek and Mahantango Creek within the Chesapeake Bay watershed; Aughwick Creek is a tributary to the Juniata River, while Mahantango Creek is a tributary to the Susquehanna River. Panel (B) shows the land use distributions within Aughwick Creek and Mahantango Creek based on data from the 2016 National Land Cover Database (Homer et al., 2020). Also shown in Panel (B) is the location of the USGS stream gauge on Aughwick Creek near Shirleysburg, PA, which was one of the five study sites. Panel (C) provides a close-up view of the four sampling sites within the FD-36 watershed (0.4-km2 tributary of Mahantango Creek), as well as a close-up photo of one of the sites (Site two).
The FD-36 Watershed (40 ha in size; latitude 40° 40′ 20.787″, longitude -76° 39′ 56.487″, Figure 1) is composed of a mixture of agriculture (56%), forested woodlots (30%), and grasslands (14%); the watershed is located in east-central Pennsylvania (Sharpley et al., 1999; McDowell et al., 2001; Zhu et al., 2012). Several sites within this watershed have been monitored for nearly 25 years by the USDA-ARS (Buda et al., 2011; Church et al., 2011). At each station, discharge was continuously monitored (5-min intervals) and routine water quality measurements were taken during baseflow (weekly to monthly) and stormflow (flow-weighted composites during storms). Elevations ranged from 270 m at the most upstream site to 250 m at the most downstream site, and the total distance between the upper and lower sites was 400 m; distances between individual stations ranged from 100 to 150 m (Figure 1; Zhu et al., 2012).
Aughwick Creek Watershed (77,959 ha in size; latitude 40° 16′ 35.285″, longitude −77° 54′ 2.159″, Figure 1) is composed of mixed land cover (67% forest, 23% agriculture, and 7% developed) located in south-central Pennsylvania (see USGS 2016). Aughwick Creek served as a control site outside the experimental watershed. Our studies were conducted in a third order reach of Aughwick Creek; the stream is a tributary within the Juniata River Watershed, which is also a major tributary of the Susquehanna River (see Musser 2007). General stream water quality there has been monitored by the United States Geological Survey (USGS) for >30 years (since 1990).
From 30 July to 12 August 2014 (12-day period), we deployed a series of five in situ P enrichment experiments. In the FD-36 watershed, we deployed nutrient enrichment experiments at four dedicated stream gauging stations in the FD-36 watershed (see Gburek and Sharpley, 1998 and Gburek et al., 2000 for more information on these stations). In Aughwick Creek, a single nutrient enrichment experiment was deployed at the USGS gauging station near Shirleysburg, PA. These five experiments ran in simultaneously during the same incubation period.
2.2 Ambient Stream Conditions
The biogeochemical conditions in overlying stream water were evaluated from whole water samples that were collected at each of the four sites in FD-36 and the control location in Aughwick Creek (17, 23, and 30 July 2014). Water samples were collected at mid-depth in the center of each stream site and stored in 500 ml (unfixed) and 125 ml (fixed with H2SO4 to pH < 2) Nalgene bottles. Bottles were stored at 4°C for up to 28 days for total nitrogen (TN) and total phosphorus (TP) analysis. TN in the water column was determined using the Kjeldehl method where the N content of each sample was first reduced (digested with H2SO4 and HgSO4) to ammonia and then distilled and measured colorimetrically (USEPA 1997, 2002; Patton and Kryskalla 2003). TP in the water column was determined using the persulfate digestion and measured colorimetrically (USEPA 1997, 2002). Instantaneous, in-situ water temperature (°C), specific conductivity (μS/cm), pH, dissolved oxygen concentration (mg/L), and oxygen saturation (%) were collected using a YSI 6600 Sonde meter at mid-depth in center of the stream. Instruments were calibrated prior to use with analytical standards (DEP 2003). For the Aughwick Creek site, daily stream discharge was determined using data from the USGS gauging station where daily measurements were available for the 12-day period of the experiment (http://waterdata.usgs.gov). For the FD-36 sites, daily stream discharge values at each gauging station represented daily averages of the 5-min flow measurements that were taken during the 12-days experiment. In terms of meteorological air temperature was similar between Aughwick and Mantango Creek exhibited very small differences in either air temperature (average ± one SD; 19.8±1.9 versus 18.9±1.9), although some variation in cumulative rainfall was observed between the two sites (39.7 versus 53.1 mm).
At all sites, benthic biofilms were sampled from three rocks along one transect according to the USGS protocols used in the National Water-Quality Assessment Program (Moulton et al., 2002). Transects were divided into thirds (left, middle, and right); one rock was randomly sampled from each of these zones and intact stream biofilms were sampled from each rock. The stream biofilm was removed from the rock by repeated scrubbing with a modified grout brush for 60 s, rinsing with reverse osmosis (RO) water, transferring the biofilm slurry to a plastic sample container, and recording total sample volume. The slurry collected from each rock was subsampled by filtering 2 ml aliquoits for chlorophyll-a, TP, PolyP, and PIP analysis using analytical procedures stated below. All filters were stored in an ultra-low temperature freezer (−80 °C) and held for subsequent analysis. Finally, equal volumes from each of the three stream samples (left, middle, and right) were transferred into a 125-ml Nalgene bottle and preserved with 3.5% formaldehyde for subsequent algal enumeration as described below.
2.3 In Situ Enrichment System
During the period 30 July to 14 August 2014, a series of field experiments were conducted simultaneously at all five stream sites using the previously described in situ enrichment system that has been shown to deliver a range of environmentally relevant P loading rates (ISES, Carrick and Price 2011). The experimental design included enrichment with six levels of increasing P and one level of N; this was done to evaluate the storage of P within stream biofilms across a gradient of increasing P while minimizing the effects from N limitation (Carrick and Price 2011). Very briefly, 50 ml polystyrene vials (approximately 8.0 cm × 2.7 cm) were filled with ultra-pure agar (2% w/v Difco ultra-Pure Agar Noble) to avoid inadvertent enrichment with unwanted trace elements; the agar was enriched with one of six different concentrations of P (0, 0.005, 0.015, 0.045, 0.15, and 0.5 m of NaH2PO4), and of one concentration of nitrogen (0.5 m of NaNO3). This series of concentrations is denoted throughout as P0, P1, P2, P3, P4, and P5, respectively.
These nutrient additions were chosen based upon previous work showing that these concentrations produced average daily N and P loads bracketing low and high loading conditions to natural streams (Carrick and Price 2011). These treatments were administered in triplicate (6 treatments x three replicates = 18 substrata per site). Porous, porcelain crucible lids (2.6-cm diameter) were fixed to the top of the agar-filled vials using inert aquarium sealant; the agar and sealant was allowed to harden overnight. These lids provided the substrate for biofilms to colonize on as well as the point source for the P to be released from over the 2-week period (Gibeau and Miller 1969; Carrick and Price 2011). The 18 vials were then randomly arranged in a 3 × 8 metal wire rack and transported to the stream sites for deployment. The 0 M P treatment (i.e., P0) served as a proxy for the ambient phosphorus stream nutrient conditions, and thus, was used as an overall control. More specifically, this treatment was compared with the natural rocks that were collected at the sites, prior to, and following the experimental deployment.
Experiments were deployed at four sites in FD-36 on July 30 and a single site in Aughwick Creek on 30 July 2014 (Figure 1). Racks containing the experimental treatments were placed at a mid-stream location and secured into the stream bed using rebar. Experiments were incubated for 12 days at each site and run simultaneously to standardize the temporal period among streams and provide a common period for growth and P uptake by the resident biofilms. After 12 days of incubation, racks were removed from stream sites. Stream biofilms that developed on each experimental treatment were scraped from the porous caps (using the modified grout brush) and washed into 50 ml storage vials (polyethylene, 50 ml) with several RO water rinses. Storage vials were sealed and transported to the lab for chlorophyll-a, TPP, PIP, and PolyP processing within 2 h of collection. Once in the laboratory, a series of 2 ml aliquots from each of the treatment samples were concentrated onto membranes and stored at −80°C for subsequent chlorophyll-a and P analyses (see below). In addition, a core was taken from the agar remaining from each treatment replicate, and this was placed into glass vials, and stored at −80°C for subsequent P analysis described below. Finally, slurries of the three replicates for each treatment were composited and transferred to 125-ml Nalgene bottle; this sample was preserved with 3.5% formaldehyde for algal enumeration as described below.
2.4 Analytical Procedures
2.4.1 Chlorophyll Analysis
Chlorophyll concentrations were measured as a proxy for algal biomass in stream and experimental samples (Carrick et al., 1993). Subsamples were filtered (2 ml aliquots) onto glass-fiber membranes (Whatman GF/F), placed in cryo-tubes and stored at −80°C. Within 1 month, filters were removed from the freezer and placed in vessels with a 60:40 dimethylsulfoxide to 90% acetone mixture to extract chlorophyll-a present in the samples. This supernatant was removed and analyzed on a 10-AU Turner fluorometer corrected for phaeopigments and chlorophyll-b concentrations (see Welschmeyer 1994).
2.4.2 Chemical Analyses of Biofilm Phosphorus
Subsamples were retrieved from ISES treatments and natural stream rock samples and analyzed to determine the concentration of P that accumulated within stream biofilms. To evaluate key forms of P in our samples, we subdivided total particulate P (denoted TP) into three key components: polyP or inorganically bound polyphosphate, PIP or inorganic particulate P, and POP or other forms of organically bound that were determined by subtraction (e.g., RNA, DNA, lipids, proteins).
PolyP concentrations in our samples were measured using a hot water extraction procedure (Harold 1966; Carrick and Price 2011); this extraction method has been shown to provide accurate estimates of stored P in microbial assemblages (Kenney et al., 2001; Martin and Van Mooy 2013). Briefly, 2 ml aliquots were concentrated onto nitrocellulose filters (0.22 µm pore size) that were suspended in RO water. Then, these samples were autoclaved at 120°C for a 60 min thereby hydrolyzing polyP from its condensed inorganic form into DIP (Fitzgerald and Nelson 1966). The resulting DIP content of samples was analyzed using standard colorimetric methods (USEPA 1997, 2002) and measured using a model Beckman Coulter DU 700 spectrophotometer. All DIP samples were measured in duplicate with a third water subsample containing no ascorbic acid (no color change) serving as a blank.
Particulate inorganic phosphorus (PIP) was measured using a method targeting surface-adsorbed inorganic forms of P (see Asahi et al., 2014). Aliquots of 2 ml were filtered onto Whatman GF/F filters (0.70 µm pore size) to isolate sediment and other particles. The filters were then soaked in glass vials filled with 1N HCl for 12 h on a shaker table at room temperature. Following this, samples were centrifuged for 5 min and the supernatant (3 ml) was removed, neutralized with 1N NaOH, and the resulting DIP content was measured using the colorimetric procedure described above (USEPA 1997, 2002).
TP was measured in tandem on all samples; this analyte represents the collective P pool that included all organic and inorganic present in streams biofilm samples. Aliquots of 2 ml were filtered onto nitrocellulose filters (0.22 µm pore size). This material was then treated with potassium persulfate and autoclaved for 30-min standard to oxidize the P present in our samples to DIP. The resulting DIP was measured using the colorimetric procedure described above (USEPA 1997, 2002). Last, the POP component of P (other organic P) was determined mathematically, where quantities of both polyP and PIP were subtracted from TP for each of the samples.
Nutrient loading rates for the experimental treatments were determined by measuring the concentration of P present in the agar at the beginning (time 0) and end (day 12) of all five experiments. Agar cores were removed from each experimental vial (as described above) using a bore corer and the mass of the material was weighed. The agar core subsample was place in glass vials, heated on a hot-plate under low heat (until liquefied), following which a small amount of the resulting liquid was diluted in RO water (100-fold) and analyzed according to the total P procedure described above (USEPA 1997, 2002).
2.4.3 Statistical Analyses
Descriptive statistics were calculated for stream water quality, benthic biomass, and P content for each of the sites where the experiments were carried out. P-storage and P-update descriptive statistics were calculated from the mean value of replicates for site-specific experimental treatments (n = 30). A one-way analysis of variance (ANOVA) was used to compare stream discharge and dissolved nutrient (chloride, sodium, total phosphorus, etc.) measurements made during the experimental period, where stream site was considered a fixed factor. Tukey multiple means comparisons were used to isolate the pair-wise differences to give an idea of the stream site characteristics. Variation in P loading rates from the ISES treatments was assessed using a two-way ANOVA, where average loading rates were compared among two fixed factors (i.e., P treatment and site). Variation in stream biofilm biomass and P content (chlorophyll-a, PolyP, PIP, TPP) among the experimental treatments was analyzed using two-way multivariate analysis of variance (MANOVA), where both P loading and site were considered fixed factors. Post-hoc Tukey multiple means comparisons (p < 0.05) were used to evaluate sources of significant variation within individual factors. Biomass (i.e., chlorophyll-a), TPP, and PolyP content measured on natural stream rocks were analyzed using one-way MANOVA tests, where site was considered a fixed factor. To evaluate the relationship of P dynamics in the biofilms with stream productivity (see Carrick et al., 2007), P storage and P uptake determined from our experiments were regressed against stream conductivity (as a proxy for stream productivity). P uptake was determined using the slope of the PolyP concentration in biofilms vs. the experimental P loading at each site. All data were log10 transformed to meet assumptions of normality and homogeneity of variances. Normality was achieved for all ambient rock data, although normality was not achieved for any experimental data according to the Wilkes-Shapiro test; however, the residual distribution approached a normal distribution with no outliers in the data set. Thus, these data met the assumptions for normality and homogeneity of variations and allowed the use of inferential statistics (i.e., MANOVA, ANOVA, linear regressions). All statistical analyses were conducted using the statistical software SPSS v. 26.
3 Results
3.1 Biogeochemical Conditions at Stream Sites
Water chemistry was similar among the five stream sites we investigated, although some differences were evident. Aughwick Creek was characterized by modest conductivity values, circumneutral pH, and low nutrients while the four sites in FD-36 had higher nutrient levels and lower values for pH and conductivity which increased downstream (Table 1). Among all five sites, conductivity ranged from 102 to 268 μS cm−1 with an average concentration of 173 μS cm−1 and pH ranged from 6.84 to 8.99 with an average of 7.69. Stream temperature over the course of the 12-days experiment averaged 25 C (range 23.1–27.5°C). Total N levels were approximately 0.730 mg L−1 and total P levels were around 0.03 mg L−1. Stream baseflow and the mineral content of the stream water did not reveal appreciable differences among any of the stream sites, although as expected, there was an increasing trend in flow from upstream to downstream sites, with Aughwick Creek having the highest discharge. Including Aughwick Creek, where the only available water quality data were for TP and TN, there were no significant differences in total nutrient concentrations between the five sites (one-way ANOVA; F = 2.00; p = 0.151). For FD-36, where there were data for numerous chemical species (e.g., Fe, Ca, Si, S, Mg, Mn, Al, K, Na, Cl), there were few if any significant differences in water chemistry between the four sites (p > 0.05). The only difference was with Mn and S concentrations, where Site three was significantly higher and lower than the other sites, respectively. Moreover, all forms of N (ammonium, nitrate, TN) and P (DIP and TP) were consistent between the sites.

TABLE 1. Initial biogeochemical conditions at five stream sites in the Mid-Atlantic region of the United States where experiments were carried out. Four sites were located along an upstream to downstream reach (Site one to Site four) in the FD-36 watershed and one site in Aughwick Creek watershed (14 July 2014). Naturally occurring biofilms were sampled on 14 July 2014 from stream rocks and water chemistry was measured from grab samples collected on several dates (17, 23, and 30 July 2014) prior to the initiation of experiments. Discharge was measured for the entire during daily over the entire day of the experiment.
The ambient, biogeochemical conditions of intact, benthic biofilms growing on stream rocks did not exhibit marked difference among the five sites (Table 1). There was no significant difference between the sites in terms of total particulate P (one-way ANOVA; F = 1.72; p = 0.152) or polyP (F = 2.57; p = 0.430). Benthic chlorophyll did vary significantly among the five sites, where it was greater for Aughwick in comparison to the four other sites (F = 10.52; p = 0.000). The four sites in FD-36 were not different for chlorophyll, total P, and PolyP concentrations. Furthermore, ratios of Chl:TP and Chl:PP were not different among sites (F = 1.49; p = 0.273). There were significant differences in the biomass of biofilms that grew under natural stream conditions (rocks) and those exposed to experimental P enrichment (one-way ANOVA; F = 17.35; p = 0.000). As expected, polyP on the natural rocks was not different from either the P0 or the P1 treatments; however, the rocks had lower P content compared with high loading rates for P treatments (P2, P3, P4, and P5). Comparison among the study sites showed few differences, although Site two overall had a greater polyP content relative to the other sites.
3.2 Biofilm Response to Experimental P Loading
P loading rates varied by more than two orders of magnitude among the six experimental treatments and loading rates differed significantly among the six treatments (two-way ANOVA; F = 3.42; p < 0.000). The rates ranged from 0.0 μg day−1 (P0 treatment) to 1,540.7 μg day−1 (P5 treatment) with a very low standard deviation (0.00–0.147 ug day−1). That said, P loading rates were consistent, and did not vary significantly among the five experiments (F = 1.49; p = 0.216). Furthermore, there was no detectable release of P from the control treatment (P0) and no difference in P loading rates among sites (ANOVA interaction between sites and treatment, p > 0.05).
The accumulation of benthic biofilm biomass (as chlorophyll) and tissue P content as TP, PolyP, and PIP showed separate site (F = 24.46; p = 0.000) and treatment (F = 9.51; p = 0.000) effects, as well as a significant interaction between the two factors (Figure 2; two-way MANOVA, F = 1.77; p = 0.000). Blocked one-way ANOVA tested for singular, site and treatments effects (Figure 2; Table 2) showed between-subject differences for all dependent variables (chlorophyll, TP, PIP, PolyP).
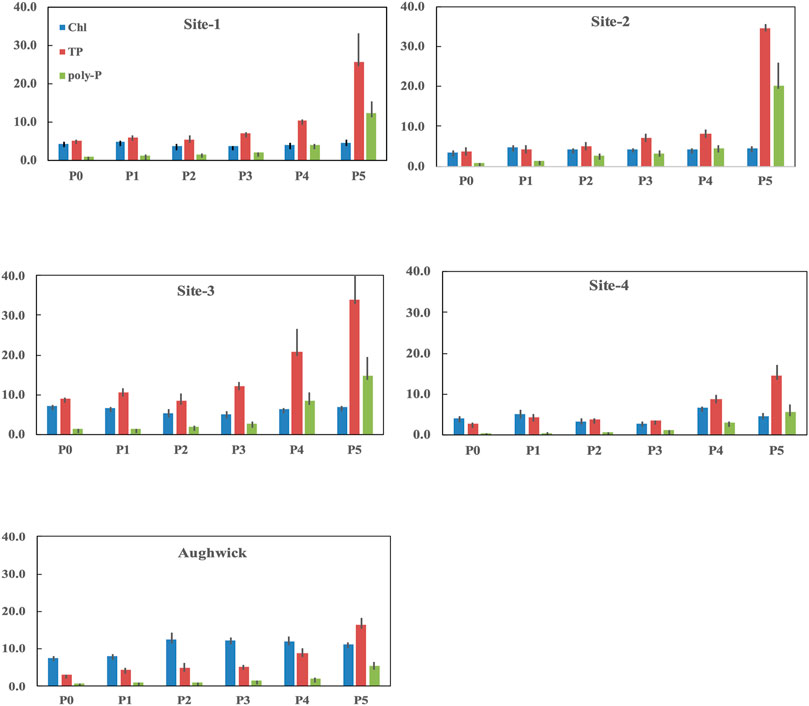
FIGURE 2. Concentrations of chlorophyll (Chl) and phosphorus (as TP, polyP) for biolfilms exposed to six levels of increasing P-loading (µg P m−2 h−1) released during in situ experiments carried out simultaneously at five streams in July 2014 (incubation times ranged from 12 days).
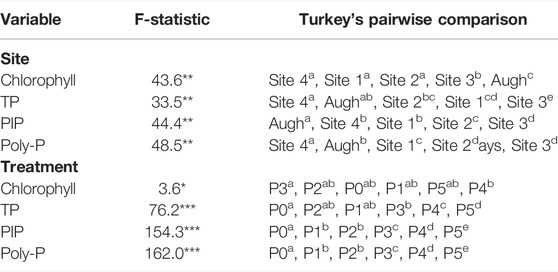
TABLE 2. Blocked one-way ANOVA and corresponding Tukey’s HSD pairwise comparisons for fixed site and treatment effects. Sites and treatments are listed in order of their means (low to high), with superscripts indicating which variables are statistically different from each other (Tukey’s comparisons p < 0.05).
In terms of singular site effects, Aughwick supported greater chlorophyll than the FD-36 sites; however, in the FD-36 watershed, Sites 1, 2, and 4 had the similar chlorophyll content, while biofilm chlorophyll at Site-3 were significantly greater. In terms of biofilm TP content, Aughwick and Sites two and four had the least amount while concentrations at Site three were greater than these three sites. PolyP storage was lowest in Site-4, followed by Aughwick and then Site 1. Sites two and three had the highest amounts of P storage. PIP showed a similar pattern with Aughwick containing the lowest amount of inorganically bound P, followed by Sites 1 and 4, then 2, with Site three having the highest amount. P loading was the same between all five sites.
In terms of singular treatment effects, there were few significant differences for chlorophyll values among P treatments (Figure 2; Table 2). That said, stream biofilm TP, PIP, and polyP concentrations increased with increasing P enrichment. For instance, concentrations of algal P were similar on P0-P3 treatment; however, all three forms of P increased with enrichment at in the P4 and P5 treatments.
3.3 Effects of P Loading on Biofilm P Mass Balance
The chemical form of P retained by benthic biofilms varied with experimentally induced P loading. TP concentrations retained by biofilms ranged from 4.74 to 25.0 mg m−2 among the six P loading treatments (average among all sites, Figure 3). P retained in biofilms identified as polyP ranged from 0.84 to 11.68 mg m−2; this P form made up a larger component of the P pool as P loading increased (one-way ANOVA; F = 161.96; p = 0.0001). The POP components (e.g., DNA/RNA or lipids) ranged from 2.64 to 4.37 mg m−2 and did not vary among P treatments (F = 0.59, p = 0.705). PIP represented a significant component of the biofilm P pool (range 0.96–8.97 mg m−2), and this component also became more important with increasing P loads (F = 154.29; p = 0.0001).
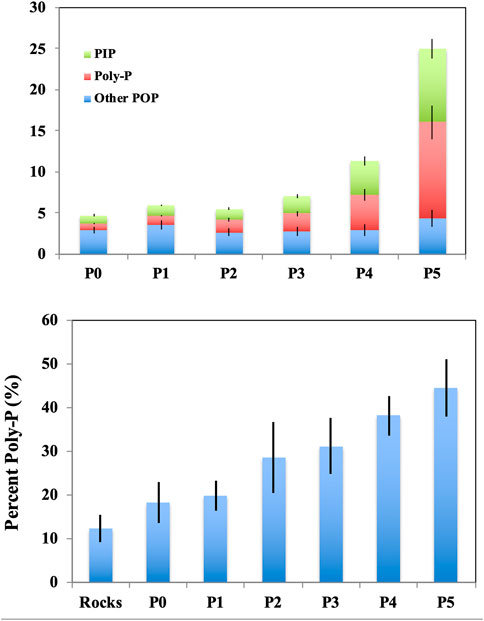
FIGURE 3. Stacked bar chart for the mass balance of biofilm P among the ISES treatments (Top panel), where sites and treatment replicates were averaged (Top panel). Bar chart depicted of the percent polyP out of TP (Bottom panel). Error bars were calculated as standard errors.
4 Discussion
4.1 P-Uptake by Biofilms as a Function of Stream P-Loading
Overall, there was a strong relationship between experimental P loading rates and PolyP accumulation by stream biofilms growing on the artificial substrata delivered by the ISES (r2 = 0.78, p = 0.000, n = 90). As such, the ISES delivered predictable loadings of DIP that promoted proportionate changes in P storage by biofilms, and this pattern was consistent among the five streams where experiments were performed (see Figure 2). The P uptake rates among experimental treatments measured here (average ± one SD: 0.111 ± 0.096, n = 30) were low compared with previous studies that evaluated P uptake using radiotracer experiments on biofilms streams from a similar region in the United States mid-Atlantic (see Price and Carrick 2013, 2014). P uptake rates measured here were likely net uptake rates, because these were based upon mass accumulations over relatively long-time periods (days, weeks). As such, the ISES experiments produce conservative rates (net) compared with more instantaneous rates (gross) estimated during short-term (minutes, hours) experiments that use radionuclide 33P tracers (see Price and Carrick 2014). That said, the range in our P uptake rates (range 0.028–0.461 μg P µg Chl−1 day−1) agreed well with other stream biofilm P uptake estimates (range 0.054–0.648 μg P µg Chl−1 day−1) that were measured similarly from mass accumulation type measurements (Steinman et al., 1991; Steinman and Boston 1993).
Interestingly, the rate of increase in P storage with P loading never reached a visible saturation point over the 12-days incubation period. This was evidenced by the exponential rate of P accumulation in the biofilms assayed here. In terms of stream ecological succession, this pattern may be attributed to the relatively short duration of the experiment, which (by design) targeted the early colonization of biofilms. Furthermore, another study found that the release rate of nutrients from agar substrates declines after 1 week (Corkum 1996). Thus, it is likely that the initial leaching of P from the vials emerged as a pulse early in the experiment, followed by decreasing loading rates after longer incubation times (Pringle and Triska 2006). The design of the ISES substrata (low surface to internal volume ratio) were such that it would minimize large, initial releases during early stages of the experiment as compared to other substrata designs with very large surface to volume configurations (Corkum 1996). Regardless, nutrient pulses that occur under natural conditions have been known to stimulate luxury P consumption (Dodds and Welch 2000; Rier et al., 2014). As a result, our experimental designed appeared to offer a reasonable representation of this common short-term, stream nutrient pulse phenomenon (Carrick and Price 2011).
Our estimates of P accumulation are likely good approximations for the capacity of resident stream biofilms to store newly available DIP through realistic stream enrichment events. The enrichment loading rates among all six treatments spanned a range from low (ambient, controls) to those experienced during high watershed run-off events (see Carrick and Price 2011). Moreover, the moderate incubation times insured that uptake and subsequent storage of P measured here were performed by biofilms occurring during an early period of algal succession (Poff et al., 1990; Sobczak and Burton 1996) compared with the longer period (>30 days) usually required to achieve late succession growth on artificial substrata (see Carrick and Lowe 2007). As such, the low stream biomass observed at all five stream sites was expected, and P uptake was also expected to be favored over carbon uptake, and carbon is usually favored when algae are actively growing (Lean and Pick 1981). We interpreted the lack of biomass differences (as chlorophyll-a) measured among the P treatments at all sites as further evidence that our experiments targeted an early colonization assemblage.
4.2 Effects of Loading on P Partitioning Within Stream Biofilms
The impact of benthic stream biofilms and associated microbes on nutrient cycling in stream ecosystems has largely been treated as a black box, particularly in the context of studies evaluating variation across long-term (seasons, years) temporal and large (watershed, ecosystem) spatial scales (e.g., Mullholland et al., 1995; Earl et al., 2006). As such, the role stream biofilms may play in altering nutrient dynamics within stream reaches has yet to be fully realized (e.g., Saia et al., 2017). As an initial step, we evaluated the P make-up of natural stream biofilms exposed to known P enrichment inputs that were delivered under in situ stream conditions along a continuum of varying degrees of legacy P loads as evidenced by the ambient TP and polyP concentrations at each experimental site. We believe our results are a reasonable representation of natural conditions because stream biofilms receiving low P enrichment (i.e., treatments P0 and P1) supported a comparable percent composition of P among chemical pools (i.e., PolyP, PIP, and POP) relative to resident stream biofilms present on rocks collected from the same location of at the end of this experiment.
In our experiments, available DIP provided through enrichment was stored as POP which we assume was composed of several relevant P-rich biochemicals (i.e., DNA, RNA, lipids, etc.) under low loading rates, whereas DIP uptake and subsequent polyP storage dominated under higher, experimental P loading rates (see Figure 3). These findings underscore the importance of polyP as a mechanism for microbes to take up, and subsequently store P from new pulses of DIP (e.g., Jensen 1968; Stevenson and Stoermer 1982). To further illustrate the impact of polyP under variable nutrient fields, Martin et al. (Martin et al., 2011; Martin et al., 2014) found that marine phytoplankton accumulated more polyP in P depleted regions of the Sargasso Sea compared to regions that were more P-rich. In freshwater streams, Rier et al. (2014) showed that biofilms growing in experimental streams readily took up new DIP during pulse enrichment events, whereby polyP formation served to store this new DIP for future use. Data from other P enrichment experiments have shown that microbial assemblages were capable of P uptake and conversion to polyP; this has been demonstrated for both freshwater lake plankton (Carrick et al., 1993) and stream biofilms (Price and Carrick 2014). As such, P enrichment studies might show promise in defining the capacity of aquatic ecosystems to assimilate new P.
We found significant concentrations of PIP in our samples (both stream baseline and experimental, see Figure 3); this form of P was likely formed as colloidal particles associated with the biofilm mat. DIP often binds with minerals such as Ca, Fe, Al within the matrix of the biofilms and is liberated by the acidic extraction procedure (Cowen and Lee 1976). Because the fraction of PIP never represented a majority of biofilm P in our study (<50% in all experimental samples), this result suggests that most of the DIP input was taken up by cells rather than being bound as more passive components of the biofilm (Ehama et al., 2016). One study on stream biofilm P contents found that PIP in lowland, hardwater (rich in calcium and magnesium carbonates) river biofilms ranged from 27–83% of TP (Jarvie et al., 2002). This percent contribution of PIP was similar those measured in coastal sediments, where the composition varied from 24–86% in the lower Chesapeake Bay (Keefe 1994) and ∼69% in the Gulf of California (Lyons et al., 2011).
Lastly, the POP fraction measured in our experiments (e.g., DNA, RNA, lipids, and proteins) was relatively consistent among the treatments. This finding supports the idea that little cell replication (i.e., growth) occurred in stream biofilms across treatments; similarly, Karpinets et al. (2006) showed that RNA:protein ratios were tightly coupled to the growth rates of microbes. This finding supports our measurements of polyP determined using the hot water extractable method, given that our estimates of polyP increased proportionately with experimental P loading while other P fractions did not exhibit this same positive relationship (see Figure 3). That said, few studies in freshwater systems have documented the occurrence of specific polyP accumulating organisms (PAOs). PAOs, as studied in enhanced biological P removal (EBPR) wastewater treatment plants, store DIP as polyP under aerobic conditions and release DIP during anaerobic conditions when polyP is hydrolyzed (Martin et al., 2011; Saia et al., 2017). One study identified known PAOs in lake sediments (Martin and Van Mooy 2013), while another observed polyP accumulation by filamentous cyanobacterial symbionts within sponges and verified the presence of ppk genes using techniques established for EBPR PAOs (Zhang et al., 2013). That said, bulk extraction hot water hydrolysis techniques, like that used here, have been successfully used to quantify polyP content in microbial stream biofilm assemblages (e.g., Price and Carrick 2013) and lake sediments (e.g., Kenney et al. 2001). More recently, enzymatic assays have been used to isolate polyP in marine plankton samples (usually 10–20% of particulate P content), although these studies were unable to reconcile a reasonable mass balance among inorganic and organic P pools (Martin et al., 2011; Martin et al., 2014). Rier et al.(2014) also used enzymatic assays to measure polyP content of stream biofilms and found it constituted ∼20% of TP, although they did not determine a mass balance for polyP relative to other inorganic and organic P pools. Finally, 31P nuclear magnetic resonance (31P-NMR) spectroscopy has been used to verify the hot water extraction method for measuring polyP content (Torres et al., 2014).
4.3 Biofilm Uptake and Stream Legacy P
In this study, we show that the capacity of stream microbes to store PolyP was dependent on DIP availability; hence, we hypothesized that their diminished capacity to take up and ultimately store new DIP as PolyP should be a useful metric of ecosystem legacy P loading. In this sense, the capacity of an ecosystem to store new DIP as PolyP may reflect the relative elasticity of the ecosystem to respond to P delivered from watershed run-off events, which we expect to become even more frequent as a function of recent changes in climate (e.g., Wagena et al., 2018). This mechanism is the basis for DIP removable from wastewater that has a long history (since the 1970s), whereby enhanced biological P removal has been optimized to use microbes know as polyphosphate accumulating organisms (PAOs) capable of taking up excess DIP relative to normal cellular levels (Yall et al., 1972; Seviour and McIlroy, 2008; Gebremariam et al., 2011).
Merely measuring the variation in DIP or other dissolved nutrients in overlying waters provides an incomplete picture of ecosystem dynamics, and more often can yield contradictory interpretations (see Saia et al., 2021). For instance, ecosystems experiencing large legacy impacts, may reduce concentrations of DIP in the overlying water simply due to the large biomass of resident biofilm which are characteristics of chronic P loadings (see Stevenson et al., 2008). These ecosystems have lost their capacity to take up (and store) any additional new P and are saturated with P, no longer buffering downstream receiving bodies from further nutrient influxes (Eriksson and Weisner 1996; Zhang et al., 2013). Similarly, N-saturation has been measured and identified in streams, although the capacity of biofilms to store N is not as great as that for P, because of the ability of microbes to use polyP as a hyper-storage mechanism (see Scanlan et al., 2015). Interestingly, a similar scenario can be imagined, where stream reaches where little to no legacy P overloading has occurred usually support biofilms with a high affinity to take up DIP. Other studies have also observed similar patterns in P retentions and cycling, where streams with high biofilm chlorophyll content will typically be saturated with polyP with a low demand for any further P (i.e., high levels of P storage per unit chlorophyll) compared with streams that experienced lower levels of chronic P loading, are under-saturated with P, and exhibit higher capacity for new P storage (see Price and Carrick 2014, 2016). Stream biofilms exposed to high DIP loadings essentially lose their ability to assimilate nutrient loads from recent P pulses (e.g., Dodds, 2010).
Given this, we believe determining the actual capacity of biofilms to assimilate DIP is a key measurement to make in the assessment of stream trophic state, health, and nutrient resiliency. Similar experiments to those done here were performed in eight mid-Atlantic ecoregions showed that P uptake rates varied predictably along a nutrient gradient (Price and Carrick, 2016), such that the development of intracellular polyP granules in stream biofilms was proportional to the degree of nutrient additions to stream ecosystems from the surrounding landscape (Price and Carrick, 2013). Along these lines, Rier et al. (2014) performed experiments in a series of artificial streams that demonstrated a causal link between storage of PolyP by stream biofilms and pulsed enrichment of overlying stream water with DIP; moreover, the stored PolyP evidently was used to fuel future microbial growth. Furthermore, in a region affected by point source nutrient inputs from a wastewater treatment plant, a significant decrease in DIP uptake efficiency might be expected downstream from a point source (Merseburger et al., 2011).
Our data here show that PolyP storage by stream biofilms in a single temperate watershed was greatest in nutrient-depleted headwater sites compared to downstream sites that were nutrient-abundant (see Figure 4). Our findings support the idea that long-term land use patterns can affect P uptake in a predictable way, as bulk percent P storage (mg m−2) measured on natural stream substrata (rocks) increased with conductivity at each site (r2 = 0.90; p = 0.01), at the same time, P uptake from our experiments decreased with conductivity (r2 = 0.70; p = 0.08, see Figure 4). Upstream sites (Sites 1–3) demonstrated significantly higher uptake capacity for DIP compared with more P enriched sites downstream (Site-4 and Aughwick Creek, see Figures 1, 2). P enriched sites tend to support higher levels of biofilm chlorophyll and stored P (as TP and polyP, see Table 1); however, these sites also exhibited lower capacity to store new sources of DIP they are more saturated with legacy P. This appears to be a typical pattern seen in streams of varying nutrient status, so that relying solely on measurement of nutrients in the water column to determine nutrient status of a stream, as is often done by researchers and managers, may not give a complete picture about the capacity of streams and river to assimilate and alter the quantity of P throughout the landscape.
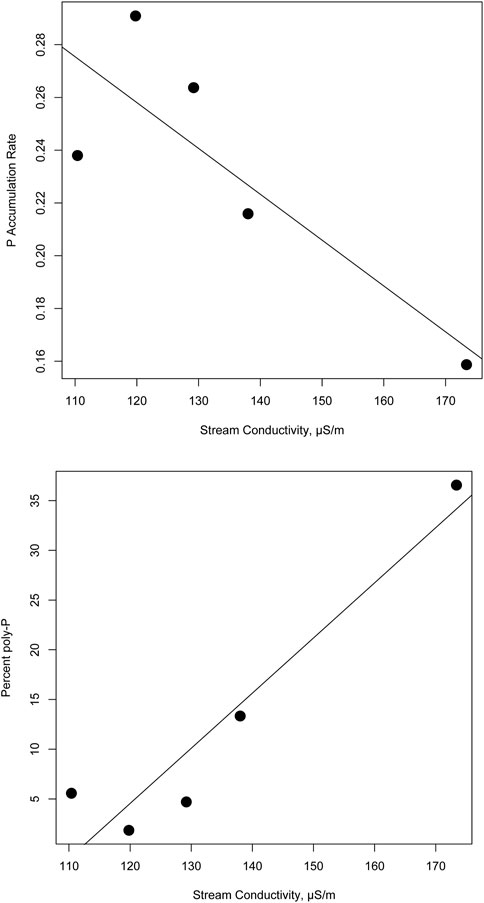
FIGURE 4. Simple linear regressions depicting the relationship between stream water conductivity (proxy for legacy nutrients in μS/cm) versus estimates percent polyP storage (Top panel), p = 0.01 on natural stream rocks and polyP uptake (Bottom panel), p = 0.08 derived from a series of in situ experiments.
Data Availability Statement
The raw data supporting the conclusions of this article will be made available by the authors, without undue reservation.
Author Contributions
ST, SS, AB, JR, MW, and HC designed the study. ST and HC collected and analyzed the data. ST, SS, PJS, JR, HC, AB, and MW interpreted the data. ST and HC drafted the manuscript. SS, AB, JR, MW, and HC provided critical revision.
Funding
The research was supported by the grant to JR, AB, HC and MW from the United States Department of Agriculture, grant number 2014-67019-21636.
Conflict of Interest
The authors declare that the research was conducted in the absence of any commercial or financial relationships that could be construed as a potential conflict of interest.
Publisher’s Note
All claims expressed in this article are solely those of the authors and do not necessarily represent those of their affiliated organizations, or those of the publisher, the editors and the reviewers. Any product that may be evaluated in this article, or claim that may be made by its manufacturer, is not guaranteed or endorsed by the publisher.
Acknowledgments
The paper was completed in partial fulfillment of the Master of Biology degree at Central Michigan University by ST; P. Hertzler and E. Linton served on her academic committee. The following people provided technical assistance in the laboratory and field: K. Carrick, A. Dart, S. Bradley, D. Daniels, M. Hurley, C. Niles, A. Stimetz, and J. Vance. This paper is contribution number 169, Institute for Great Lakes Research at Central Michigan University.
References
Alexander, R. B., Boyer, E. W., Smith, R. A., Schwarz, G. E., and Moore, R. B. (2007). The Role of Headwater Streams in Downstream Water Quality1. J. Am. Water Resour. Assoc. 43, 41–59. doi:10.1111/j.1752-1688.2007.00005.x
Asahi, T., Ichimi, K., Yamaguchi, H., and Tada, K. (2014). Horizontal Distribution of Particulate Matter and its Characterization Using Phosphorus as an Indicator in Surface Coastal Water, Harima-Nada, the Seto Inland Sea, Japan. J. Oceanogr. 70, 277–287. doi:10.1007/s10872-014-0230-z
Ator, S. W., and Denver, J. M. (2012). Estimating Contributions of Nitrate and Herbicides from Groundwater to Headwater Streams, Northern Atlantic Coastal Plain, United States. JAWRA J. Am. Water Resour. Assoc. 48, 1075–1090. doi:10.1111/j.1752-1688.2012.00672.x
Buda, A. R., Feyereisen, G. W., Veith, T. L., Folmar, G. J., Bryant, R. B., Church, C. D., et al. (2011). U.S. Department of Agriculture Agricultural Research Service Mahantango Creek Watershed, Pennsylvania, United States: Long Term Stream Discharge Database. Water Resour. Res. 47, 1–5. doi:10.1029/2010wr010059
Carpenter, S. R., Caraco, N. F., Correll, D. L., Howarth, R. W., Sharpley, A. N., and Smith, V. H. (1998). Nonpoint Pollution of Surface Waters with Phosphorus and Nitrogen. Ecol. Appl. 8, 559–568. doi:10.1890/1051-0761(1998)008[0559:nposww]2.0.co;2
Carrick, H. J., Godwin, C. M., Johnston-Greenwald, M., Rilk, C., Siefert, A., and Tzilkowski, C. J. (2007). Evaluation of Water Quality in a Spring Fed Stream (Spring Creek, Pennsylvania) Based upon Benthic Algae and Macroinvertebrates. J. Pa. Acad. Sci. 80, 71–78. Available at: https://www.jstor.org/stable/44149600.
Carrick, H. J., and Lowe, R. L. (2007). Nutrient Limitation of Benthic Algae in Lake Michigan: the Role of Silica. J. Phycol. 43, 228–234. doi:10.1111/j.1529-8817.2007.00326.x
Carrick, H. J., and Price, K. J. (2011). Determining Variation in TMDL Reduction Criteria. Final Report. Pennsylvania: Pennsylvania Department of Environmental Protection, 84.
Carrick, H. J., Scanlan, A., and Wagner, R. (2009). Use of Periphyton to Estimate TMDL End-Points. Final Report. Pennsylvania: Pennsylvania Department of Environmental Protection, 57.
Carrick, H. J., Schelske, C. L., Aldridge, F. J., and Coveney, M. F. (1993). Assessment of Phytoplankton Nutrient Limitation in Productive Waters: Application of Dilution Bioassays. Can. J. Fish. Aquat. Sci. 50, 2208–2221. doi:10.1139/f93-247
Church, C. D., Veith, T. L., Folmar, G. J., Buda, A. R., Feyereisen, G. W., Bryant, R. B., et al. (2011). U.S. Department of Agriculture Agricultural Research Service Mahantango Creek Watershed, Pennsylvania, United States: Long-Term Water Quality Database. Water Resour. Res. 47, 1–5. doi:10.1029/2010wr010060
Corkum, L. D. (1996). Patterns of Nutrient Release from Nutrient Diffusing Substrates in Flowing Water. Hydrobiologia 333, 37–43. doi:10.1007/bf00020962
Correll, D. L. (1998). The Role of Phosphorus in the Eutrophication of Receiving Waters: a Review. J. Environ. Qual. 27, 261–266. doi:10.2134/jeq1998.00472425002700020004x
Cowen, W. F., and Lee, G. F. (1976). Phosphorus Availability in Particulate Materials Transported by Urban Runoff. J. Water Pollut. Control Fed. 48, 580–591.
Dodds, W. K. K., and Welch, E. B. (2000). Establishing Nutrient Criteria in Streams. J. North Am. Benthol. Soc. 19, 186–196. doi:10.2307/1468291
Dodds, W. K., and Oakes, R. M. (2008). Headwater Influences on Downstream Water Quality. Environ. Manag. 41, 367–377. doi:10.1007/s00267-007-9033-y
Earl, S. R., Valett, H. M., and Webster, J. R. (2006). Nitrogen Saturation in Stream Ecosystems. Ecology 87, 3140–3151. doi:10.1890/0012-9658(2006)87[3140:nsise]2.0.co;2
Ehama, M., Hashihama, F., Kinouchi, S., Kanda, J., and Saito, H. (2016). Sensitive Determination of Total Particulate Phosphorus and Particulate Inorganic Phosphorus in Seawater Using Liquid Waveguide Spectrophotometry. Talanta 153, 66–70. doi:10.1016/j.talanta.2016.02.058
Eixler, S., Selig, U., and Karsten, U. (2004). Extraction and Detection Methods for Polyphosphate Storage in Autotrophic Planktonic Organisms. Hydrobiologia 533, 135–143. doi:10.1007/s10750-004-2406-9
Eriksson, P. G., and Weisner, S. E. B. (1996). Nitrogen Removal in a Wastewater Reservoir: the Importance of Denitrification by Epiphytic Biofilms on Submersed Vegetation. J. Environ. Qual. 26, 905–910.
Fitzgerald, G. P., and Nelson, T. C. (1966). Extractive and Enzymatic Analyses for Limiting or Surplus Phosphorus in Algae. J. Phycol. 2, 32–37. doi:10.1111/j.1529-8817.1966.tb04589.x
Gburek, W. J., Sharpley, A. N., and Folmar, G. J. (2000). “Critical Areas of Phosphorus Export from Agricultural Watersheds,” in Agriculture and Phosphorus Management. Editor A. N. Sharpley (Boca Raton, FL: Lewis Publishers), 83–104.
Gburek, W. J., and Sharpley, A. N. (1998). Hydrologic Controls on Phosphorus Loss from Upland Agricultural Watersheds. J. Environ. Qual. 27, 267–277. doi:10.2134/jeq1998.00472425002700020005x
Gebremariam, S. Y., Beutel, M. W., Christian, D., and Hess, T. F. (2011). Research Advances and Challenges in the Microbiology of Enhanced Biological Phosphorus Removal-A Critical Review. Water Environ. Res. 83, 195–219. doi:10.2175/106143010x12780288628534
Geyer, A. R., and Wilschusen, J. P. (1982). Engineering Characteristics of the Rocks of Pennsylvania: Environmental Geology Supplement of the State Geologic Map. United states: Department of Environmental Resources, University of Illinois.
Gibeau, G. G., and Miller, M. C. (1969). A Micro-bioassay for Epilithon Using Nutrient-Diffusing Artificial Substrata. J. Freshw. Ecol. 5, 171–176.
Harold, F. M. (1966). Inorganic Polyphosphates in Biology: Structure, Metabolism, and Function. Bacteriol. Rev. 30, 772–794. doi:10.1128/br.30.4.772-794.1966
Healey, F. P. (1979). Short-Term Responses of Nutrient-Deficient Algae to Nutrient Addition1. J. Phycol. 15, 289–299. doi:10.1111/j.0022-3646.1979.00289.x
Homer, C., Dewitz, J., Jin, S., Xian, G., Costello, C., Danielson, P., et al. (2020). Conterminous United States Land Cover Change Patterns 2001-2016 from the 2016 National Land Cover Database. ISPRS J. Photogrammetry Remote Sens. 162, 184–199. doi:10.1016/j.isprsjprs.2020.02.019
Jacobson, L., and Halmann, M. (1982). Polyphosphate Metabolism in the Blue-Green Alga Microcystis Aeru-Ginosa. J. Plankton Res. 4, 481–488. doi:10.1093/plankt/4.3.481
Jarvie, H. P., Neal, C., Warwick, A., White, J., Neal, M., Wickham, H. D., et al. (2002). Phosphorus Uptake into Algal Biofilms in a Lowland Chalk River. Sci. Total Environ. 282-283, 353–373. doi:10.1016/s0048-9697(01)00924-x
Jensen, T. E. (1968). Electron Microscopy of Polyphosphate Bodies in a Blue-Green Alga, Nostoc Pruniforme. Arch. Mikrobiol. 62, 144–152. doi:10.1007/bf00410400
Karpinets, T. V., Greenwood, D. J., Sams, C. E., and Ammons, J. T. (2006). RNA:protein Ratio of the Unicellular Organism as a Characteristic of Phosphorous and Nitrogen Stoichiometry and of the Cellular Requirement of Ribosomes for Protein Synthesis. BMC Biol. 4, 30. doi:10.1186/1741-7007-4-30
Keefe, C. W. (1994). The Contribution of Inorganic Compounds to the Particulate Carbon, Nitrogen, and Phosphorus in Suspended Matter and Surface Sediments of Chesapeake Bay. Estuaries 17, 122–130. doi:10.2307/1352561
Kenney, W. F., Schelske, C. L., and Chapman, A. D. (2001). Changes in Polyphosphate Sedimentation: a Response to Excessive Phosphorus Enrichment in a Hypereutrophic Lake. Can. J. Fish. Aquat. Sci. 58 (5), 879–887. doi:10.1139/f01-040
Lean, D. R. S., and Pick, F. R. (1981). Photosynthetic Response of Lake Plankton to Nutrient Enrichment: a Test for Nutrient Limitation. Limnol. Oceanogr. 26, 1001–1019. doi:10.4319/lo.1981.26.6.1001
Lyons, G., Benitez-Nelson, C. R., and Thunell, R. C. (2011). Phosphorus Composition of Sinking Particles in the Guaymas Basin, Gulf of California. Limnol. Oceanogr. 56, 1093–1105. doi:10.4319/lo.2011.56.3.1093
Martin, P., Dyhrman, S. T., Lomas, M. W., Poulton, N. J., and Van Mooy, B. A. S. (2014). Accumulation and Enhanced Cycling of Polyphosphate by Sargasso Sea Plankton in Response to Low Phosphorus. Proc. Natl. Acad. Sci. U.S.A. 111, 8089–8094. doi:10.1073/pnas.1321719111
Martin, P., and Van Mooy, B. A. S. (2013). Fluorometric Quantification of Polyphosphate in Environmental Plankton Samples: Extraction Protocols, Matrix Effects, and Nucleic Acid Interference. Appl. Environ. Microbiol. 79, 273–281. doi:10.1128/aem.02592-12
Martins, G., Terada, A., Ribeiro, D. C., Corral, A. M., Brito, A. G., Smets, B. F., et al. (2011). Structure and Activity of Lacustrine Sediment Bacteria Involved in Nutrient and Iron Cycles. FEMS Microbiol. Ecol. 77, 666–679. doi:10.1111/j.1574-6941.2011.01145.x
McDowell, R., Sharpley, A., and Folmar, G. (2001). Phosphorus Export from an Agricultural Watershed: Linking Source and Transport Mechanisms. J. Environ. Qual. 30, 1587–1595. doi:10.2134/jeq2001.3051587x
Merseburger, G., Martí, E., Sabater, F., and Ortiz, J. D. (2011). Point-Source Effects on N and P Uptake in a Forested and an Agricultural Mediterranean Streams. Sci. Total Environ. 409, 957–967. doi:10.1016/j.scitotenv.2010.11.014
Moulton, S. R., Kennen, J. G., Goldstein, R. M., and Hambrook, J. A. (2002). Revised Protocols for Sampling Algal, Invertebrate, and Fish Communities as Part of the National Water-Quality Assessment Program. Open File Report 02-150. Australia: National Library of Australia.
Mulholland, P. J., Marzolf, E. R., Hendricks, S. P., Wilkerson, R. V., and Baybayan, A. K. (1995). Longitudinal Patterns of Nutrient Cycling and Periphyton Characteristics in Streams: A Test of Upstream-Downstream Linkage. J. North Am. Benthol. Soc. 14, 357–370. doi:10.2307/1467202
Musser, K. (2007). Susquehanna River Watershed Map with Lower Susquehanna River Watershed Highlighted. Pennsylvania, United States: Susquehanna River Basin Commission.
O’Brien, J. M., and Dodds, W. K. (2010). Saturation of NO3- Uptake in Prairie Streams as a Function of Acute and Chronic Exposure. J. N. Am. Benthol. Soc. 29, 627–635. doi:10.1899/09-021.1
Patton, C. J., and Kryskalla, J. R. (2003). Methods of Analysis by the U.S. Geological Survey National Water Quality Laboratory: Evaluation of Alkaline Per Sulfate Digestion as an Alternative to Kjeldahl Digestion for Determination of Total and Dissolved Nitrogen and Phosphorus in Water. Water-Resources Investig. Rep.doi:10.3133/WRI034174
Poff, N. L., Voelz, N. J., Ward, J. V., and Lee, R. E. (1990). Algal Colonization under Four Experimentally-Controlled Current Regimes in High Mountain Stream. J. North Am. Benthol. Soc. 9, 303–318. doi:10.2307/1467898
Price, K., and Carrick, H. (2013). Effects of Physical Disturbance on Phosphorus Uptake in Temperate Stream Biofilms. Inland Waters 3, 321–330. doi:10.5268/iw-3.3.551
Price, K. J., and Carrick, H. J. (2016). Effects of Experimental Nutrient Loading on Phosphorus Uptake by Biofilms: Evidence for Nutrient Saturation in Mid-Atlantic Streams. Freshw. Sci. 35, 503–517. doi:10.1086/686269
Price, K. J., and Carrick, H. J. (2014). Quantitative Evaluation of Spatiotemporal Phosphorus Fluxes in Stream Biofilms. Freshw. Sci. 33, 99–111. doi:10.1086/674874
Pringle, C. M., and Triska, F. J. (2006). Effects of Nutrient Enrichment on Periphyton. Methods in Stream Ecology. Netherlands: Elsevier, 743–757.
Rier, S. T., Shirvinski, J. M., and Kinek, K. C. (2014). In Situlight and Phosphorus Manipulations Reveal Potential Role of Biofilm Algae in Enhancing Enzyme-Mediated Decomposition of Organic Matter in Streams. Freshw. Biol. 59, 1039–1051. doi:10.1111/fwb.12327
Saia, S. M., Carrick, H. J., Buda, A. R., Regan, J. M., and Walter, M. T. (2021). Critical Review of Polyphosphate and Polyphosphate Accumulating Organisms for Agricultural Water Quality Management. Environ. Sci. Technol. 55, 2722–2742. doi:10.1021/acs.est.0c03566
Saia, S. M., Sullivan, P. J., Regan, J. M., Carrick, H. J., Buda, A. R., Locke, N. A., et al. (2017). Evidence for Polyphosphate Accumulating Organism (PAO)-mediated Phosphorus Cycling in Stream Biofilms under Alternating Aerobic/anaerobic Conditions. Freshw. Sci. 36, 284–296. doi:10.1086/691439
Scanlan, A. M., Millie, D. F., Weckman, G., and Carrick, H. J. (2015). Abrupt Shifts in Stream Algal Biomass and Diatom Taxonomic Composition along a Gradient of Changing Land Use. fal 186, 153–169. doi:10.1127/fal/2015/0634
Seviour, R. J., and McIlroy, S. (2008). The Microbiology of Phosphorus Removal in Activated Sludge Processes-The Current State of Play. J. Microbiol. 46, 115–124. doi:10.1007/s12275-008-0051-0
Sharpley, A. N., Gburek, W. J., Folmar, G., and Pionke, H. B. (1999). Sources of Phosphorus Exported from an Agricultural Watershed in Pennsylvania. Agric. Water Manag. 41, 77–89. doi:10.1016/s0378-3774(99)00018-9
Sicko-Goad, L., and Lazinsky, D. (1986). Quantitative Ultrastructural Changes Associated with Lead-Coupled Luxury Phosphate Uptake and Polyphosphate Utilization. Arch. Environ. Contam. Toxicol. 15, 617–627. doi:10.1007/bf01054908
Sobczak, W. V., and Burton, T. M. (1996). Epilithic Bacterial and Algal Colonization in a Stream Run, Riffle, and Pool: a Test of Biomass Covariation. Hydrobiologia 332, 159–166. doi:10.1007/bf00031921
Steinman, A. D., and Boston, H. L. (1993). The Ecological Role of Aquatic Bryophytes in a Woodland Stream. J. North Am. Benthol. Soc. 12, 17–26. doi:10.2307/1467681
Steinman, A. D., Mulholland, P. J., and Kirschtel, D. B. (1991). Interactive Effects of Nutrient Reduction and Herbivory on Biomass, Taxonomic Structure, and P Uptake in Lotic Periphyton Communities. Can. J. Fish. Aquat. Sci. 48, 1951–1959. doi:10.1139/f91-232
Stevenson, R. J., Hill, B. H., Herlihy, A. T., Yuan, L. L., and Norton, S. B. (2008). Algae-P Relationships, Thresholds, and Frequency Distributions Guide Nutrient Criterion Development. J. North Am. Benthol. Soc. 27, 783–799. doi:10.1899/07-077.1
Stevenson, R. J., and Stoermer, E. F. (1982). Luxury Consumption of Phosphorus by Five Cladophora Epiphytes in Lake Huron. Trans. Am. Microsc. Soc. 101, 151–161. doi:10.2307/3225768
Torres, I. C., Turner, B. L., and Ramesh Reddy, K. (2014). The Chemical Nature of Phosphorus in Subtropical Lake Sediments. Aquat. Geochem 20, 437–457. doi:10.1007/s10498-014-9228-9
U.S. Department of Agriculture (2010). Agricultural Research Service Mahantango Creek Watershed, Pennsylvania, United States: Physiography and History. Water Resour. Res. 47. 5p. doi:10.1029/2010WR010056
U.S. Environmental Protection Agency (2002). National Water Quality Inventory: 2000. Report. Washington, D.C: U.S. Environmental Protection Agency Report EPA-841-R-02-001.
U.S. Environmental Protection Agency (1997). Volunteer Stream Monitoring: A Methods Manual. Washington, D.C: U.S. Environmental Protection Agency Report EPA-841-R-02-001. EPA-841-B-97-003.
Wagena, M. B., Collick, A. S., Ross, A. C., Najjar, R. G., Rau, B., Sommerlot, A. R., et al. (2018). Impact of Climate Change and Climate Anomalies on Hydrologic and Biogeochemical Processes in an Agricultural Catchment of the Chesapeake Bay Watershed, USA. Sci. Total Environ. 637-638, 1443–1454. doi:10.1016/j.scitotenv.2018.05.116
Welschmeyer, N. A. (1994). Fluorometric Analysis of Chlorophyll a in the Presence of Chlorophyll B and Pheopigments. Limnol. Oceanogr. 39, 1985–1992. doi:10.4319/lo.1994.39.8.1985
Logical removal of phosphates Yall, L., Boughton, B. H., Roinestad, S. A., and Sinclair, N. A. (1972). Applications of New Concepts of Physical Chemical Waste Water Treatment. Nashville, Tennessee: Vanderbilt University.
Zhang, Q., Brady, D. C., and Ball, W. P. (2013). Long-term Seasonal Trends of Nitrogen, Phosphorus, and Suspended Sediment Load from the Non-tidal Susquehanna River Basin to Chesapeake Bay. Sci. Total Environ. 452-453, 208–221. doi:10.1016/j.scitotenv.2013.02.012
Zhang, X., Liu, Z., Gulati, R. D., and Jeppesen, E. (2013). The Effect of Benthic Algae on Phosphorus Exchange between Sediment and Overlying Water in Shallow Lakes: a Microcosm Study Using 32P as a Tracer. Hydrobiologia 710, 109–116. doi:10.1007/s10750-012-1134-9
Keywords: legacy p, polyphosphate, phosphorus enrichment, watershed, biofilms
Citation: Taylor S, Saia SM, Buda AR, Regan JM, Walter MT and Carrick HJ (2022) Polyphosphate Accumulation Tracks Incremental P-Enrichment in a Temperate Watershed (Pennsylvania, United States) as an Indicator of Stream Ecosystem Legacy P. Front. Environ. Sci. 10:920733. doi: 10.3389/fenvs.2022.920733
Received: 15 April 2022; Accepted: 16 June 2022;
Published: 06 July 2022.
Edited by:
Zhongqi He, United States Department of Agriculture, United StatesReviewed by:
Paulo Pagliari, University of Minnesota Twin Cities, United StatesNi Maofei, Guizhou Minzu University, China
Copyright © 2022 Taylor, Saia, Buda, Regan, Walter and Carrick. This is an open-access article distributed under the terms of the Creative Commons Attribution License (CC BY). The use, distribution or reproduction in other forums is permitted, provided the original author(s) and the copyright owner(s) are credited and that the original publication in this journal is cited, in accordance with accepted academic practice. No use, distribution or reproduction is permitted which does not comply with these terms.
*Correspondence: Hunter J. Carrick, aHVudGVyLmNhcnJpY2tAY21pY2guZWR1