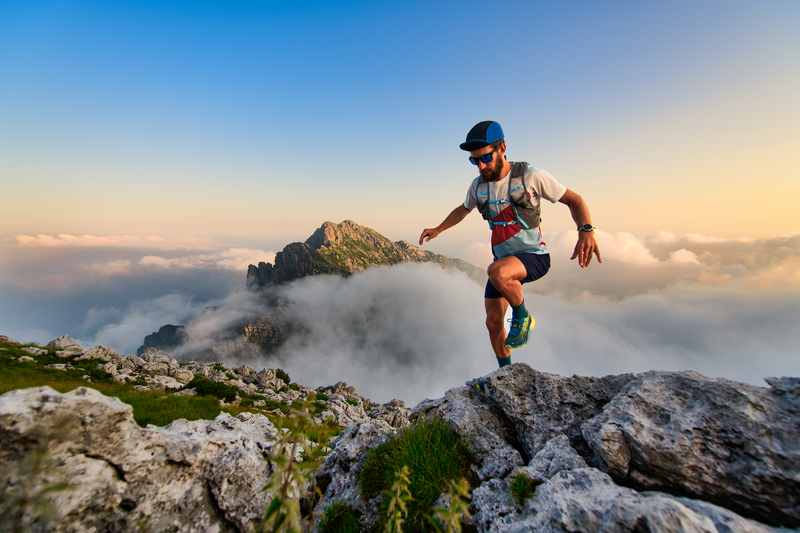
95% of researchers rate our articles as excellent or good
Learn more about the work of our research integrity team to safeguard the quality of each article we publish.
Find out more
ORIGINAL RESEARCH article
Front. Environ. Sci. , 10 November 2022
Sec. Toxicology, Pollution and the Environment
Volume 10 - 2022 | https://doi.org/10.3389/fenvs.2022.920010
This article is part of the Research Topic Exploring the Combined Effect of Climate Change and Pollution on Freshwater Ecosystems View all 5 articles
Aquatic ecosystems are exposed to pesticides through various pathways such as spray-drift, agricultural runoff, and chemical spills. Understanding the impact of pesticides on freshwater ecosystems requires not only understanding how pesticides affect aquatic organisms but also knowledge of their interactions with other stressors, such as those related to global climate change. Heatwaves are extended periods of temperature increase relative to the climatological mean. They are increasing in frequency and magnitude and pose an emerging threat to shallow freshwater ecosystems. In this study, we evaluated the single and combined effects of the herbicide terbuthylazine and a simulated heatwave on freshwater zooplankton communities using indoor microcosms. Terbuthylazine was applied at an environmentally relevant concentration (15 µg/L). The heatwave consisted of an increase of 6°C above the control temperature for a period of 7 days. When applied individually, the heatwave increased the total abundance of zooplankton by 3 times. The terbuthylazine exposure led to an indirect effect on the zooplankton community structure, reducing the relative abundance of some taxa. The combination of the heatwave and terbuthylazine had no significant impact on the zooplankton community, indicating additive effects dominated by the herbicide. The interaction between the two stressors increased chlorophyll-a concentrations and apparently changed the structure of the phytoplankton community, which may have benefitted cyanobacteria over green algae. Overall, this study shows that understanding the effects of chemical and non-chemical stressors on aquatic communities remains a challenging task. Further studies should be conducted to improve our mechanistic understanding of multiple stressor interactions at different levels of biological organisation.
With an increasing human population and food demand, pesticide use in agriculture is likely to increase in the future (Tilman et al., 2001). However, the knowledge of the environmental consequences of these often low concentrated yet widespread pesticides is scarce. In addition, the fate and effects of organic substances in the environment and their risks for aquatic communities may be influenced by global climate change (Noyes et al., 2009; Polazzo et al., 2021). Climate change is predicted to increase both the frequency and magnitude of extreme weather events, such as heatwaves (HWs) (Jentsch et al., 2007; Woolway et al., 2021). Shallow freshwater ecosystems are specifically prone to suffer from thermal extremes (Hari et al., 2006; Rasconi et al., 2017; Till et al., 2019). Yet, the knowledge of the effects of HWs in shallow lakes, wetlands, or semi-stagnant water bodies, is still scarce (Woolway et al., 2021). In lakes, HWs are predicted to increase in magnitude by 4°C and three-fold in duration (i.e., 27 days) by 2099 (Woolway et al., 2021). The stress caused by HWs on aquatic organisms and communities may increase their vulnerability to toxic chemicals and, thereby, the risk caused by them in the aquatic environment (Schiedek et al., 2007).
Freshwater planktonic communities play a key role in the metabolic balance between gross primary production and community respiration and are the major suppliers of energy for higher trophic levels (Pace and Orcutt, 1981; Hjermann et al., 2007). Field studies (Regaudie-de-Gioux and Duarte, 2012) and mesocosm experiments performed under controlled conditions (Perkins et al., 2010) have shown that the metabolic balance of freshwater and oceanic planktonic communities is temperature-dependent (Winder et al., 2012). Additionally, increasing temperature (Rasconi et al., 2015) and frequency of HWs (Moore and Folt, 1993) can alter the structure and functioning of planktonic communities by changing the abundance ratio between different functional groups and establishing different dominance patterns (Soulié et al., 2022). For example, some studies show that climate change-driven HWs may instigate smaller body sizes in phytoplankton (Moore and Folt, 1993; Zohary et al., 2021) and further impact food web functioning by, for example, favouring mixotrophic algae (Pomati et al., 2020).
Herbicides are known to pose direct toxicity to aquatic phytoplankton, which may lead to indirect effects on primary consumers such as zooplankton (Rumschlag et al., 2021). Such indirect effects include mortality (Ferrando and Andreu-Moliner, 1991), starvation, growth and reproduction inhibition (Navis et al., 2013; Oliveira dos Anjos et al., 2021), as well as the alteration of their swimming behaviour (Andrade et al., 2018), and predator scaping capacity (Hanazato, 2001). Within the group of photosynthesis inhibiting herbicides, terbuthylazine (TER) is one of the most widely used compounds (United States Environmental Protection Agency, 1995; European Food and Safety Authority, 2011). It has been considered an alternative to the more persistent herbicide atrazine (Stipičević et al., 2015), which was banned by the European Union in 2003 (Sass and Colangelo, 2006). However, due to its higher hydrophobicity, TER is less available for degrading bacteria, which may result in a higher environmental persistence and risk towards surface water ecosystems (European Food and Safety Authority, 2011). Studies have shown that TER has lethal effects on phytoplanktonic communities (using chlorophyll-a as proxy), which in turn decrease the zooplankton (i.e., copepod nauplii, rotifers, and cladocerans) abundance through food limitation and lowered dissolved oxygen levels (Pereira et al., 2017).
Currently, the potential consequences of HWs on planktonic communities that are exposed to environmentally relevant concentrations of chemicals, such as herbicides, are unknown (Polazzo et al., 2021). In this study, we assessed the single and combined effects of the herbicide TER and a heatwave (HW) on a freshwater zooplankton community representative of shallow freshwater ecosystems in the Mediterranean region. We hypothesised that: (1) TER would have an indirect negative effect on zooplankton abundance or species composition, by negatively affecting the phytoplankton community and consequently limiting the food source for zooplankton; (2) the HW alone would stress phytoplankton and zooplankton communities, changing their community structure by, e.g., decreasing the number of large-bodied zooplankton taxa or increasing the phytoplankton or cyanobacteria abundances, and that (3) the HW would accentuate the effects of TER by limiting the energy available for metabolizing and recovering from pesticide stress. Through this study, we aimed to better understand to what extent HWs influence the impact of pesticides on shallow freshwater ecosystems.
An indoor microcosm experiment was designed with 16 cylindrical glass microcosms (diameter: 20.5 cm, height: 37 cm, volume: 12 L) placed into three stainless steel water baths. The microcosms were filled with unpolluted water from an artificial lagoon at the IMDEA Water Institute (Alcalá de Henares, Spain). Zooplankton was collected from outdoor mesocosms and evenly distributed among the test microcosms. Phytoplankton species were not added individually to the test systems, but originated from the natural community in the lagoon. The introduced zooplankton was allowed to acclimatise to the environmental conditions in the microcosms for 2 weeks prior to the start of the experiment. Once per week, nutrients (NaNO3 and KH2PO4) were added to reach a concentration of 90 µg/L (total N) and 15 µg/L (total P). The N/P ratio was based on observations taken from the experimental systems where the plankton were collected and had been used in previous studies (Arenas-Sánchez et al., 2019).
The experiment included four different treatments: (1) control (no HW, no TER), (2) HW, (3) TER, and (4) HW + TER. All treatments were conducted in quadruplicates (Figure 1A). The control microcosms were kept at constant water temperature (22°C) during the experiment, which resembles the water temperature from their origin water body (outdoor mesocosms and artificial lagoon) (Polazzo et al., 2022a). For the HW treatment, heaters (wave Tropico, 300-W, AC 220V–240 V 50 Hz) were placed into the water baths to slowly (over a time period of 8 hours) heat the water of the water baths to a nominal temperature of 28°C, which resulted in a mean water temperature of 26.48°C (± 1.32) in the HW mesocosms. The HW started on day 0 and stopped 7 days later. TER was applied to the respective microcosms at a concentration of 15 µg/L. TER concentrations up to 30 µg/L have been estimated in freshwater ecosystems of Southern European agricultural areas (EC, 2007), with peak concentrations reaching 100 µg/L after spraying events (Wenneker et al., 2010). Therefore, the concentration tested here was considered to be environmentally relevant. At 15 µg/L, TER was not expected to exert direct effects on zooplankton (e.g., Daphnia magna; EC50= 21,200 µg/L) but to affect primary producers such as phytoplankton (e.g., Pseudokirchneriella subcapitata; EC50= 12 µg/L) (PPDB: Pesticide Properties DataBase, 2022).
FIGURE 1. (A) Experimental design. (B) Schematic timeline of the experiment. After an acclimatisation period of 14 days at 22°C, the experiment was started with the simultaneous application of the heatwave (HW) and the herbicide terbuthylazine (TER) on day 0. The coloured lines represent the temperature (red) and the terbuthylazine (green) dynamics in the stressed microcosms. The grey arrows mark the sampling days (i.e., day 0, 7, 14, and 21, relative to the start of the experiment).
A stock solution (180 mg/L) of TER (Sigma Aldrich; CAS No. 5915-41-3) was prepared in methanol (HiPerSolv Chromanorm, VWR International SAS). One mL of the stock solution was spiked to the microcosms that received the TER treatment to reach a concentration of 15 µg/L. Microcosms not treated with TER were spiked with 1 ml methanol, which has been demonstrated not to affect the plankton communities at the resulting water concentration (OECD, 2000). Water loss in the microcosms due to evaporation (caused by heating and regular evaporation) was compensated on a daily basis by adding deionised water via gentle pouring into the microcosms. The evaporation rate between the HW and the other treatments were not notably different. To monitor the pesticide concentrations in the microcosms, water samples were taken on days 0, 7, and 21 relative to the start of the experiment and frozen at −20°C until analysis. TER analysis was performed using a gas chromatograph (GC) system (Agilent 7890A) coupled to a mass spectrometer (MS) with a triple quadrupole analyser (Agilent 7000 GC/MS Triple Quad). The method had a limit of quantification (LOQ) between 0.1–5 µg/L (depending on the sample volume) and a recovery of 99% (analysing 3 replicates of matrix used in mesocosms, spiked at 100 ng/ml TER). Further details on the chemical analysis can be found in the supplementary material (SM).
Temperature, dissolved oxygen, electrical conductivity, salinity, pH, phycoerythrin (PE), and chlorophyll-a were measured weekly at the same time during the morning using a portable multimeter probe (Pro DSS 4-port Digital Sampling System) (Supplementary Table S3). The chlorophyll-a concentration was used as an indicator of the total phytoplankton biomass while the pigment PE was used as an indicator for the biomass of cyanobacteria (e.g., Twiss et al., 2022). Water samples were taken on days 0 and 21 and analysed for the concentration of ammonium (NH4+), nitrate (NO3−), orthophosphate (PO4−3), and total phosphorus, using a spectrophotometric method based on colourimetric analysis (American Public Health Association, 2005), to establish the same oligotrophic conditions as the sampled habitat and were maintained throughout the experiment. Total nitrogen was calculated based on the N contained in the ammonium and nitrate forms.
Zooplankton was sampled on days 0, 7, 14, and 21 relative to the start of the experiment (Figure 1B). Zooplankton sampling was done by filtering 1.5 L of microcosm water over a zooplankton net (mesh size: 55 µm). The sampled individuals were preserved with Lugol’s iodine solution (approximately 4% v/v) and stored in the dark until identification. The filtered water was returned to its original microcosm. For the identification of large zooplankton (>1 mm; e.g., Cladocera, Ostracoda, Cyclopoida), a binocular (Olympus SZX7 with objective Olympus DF PL 2X-4) was used. Smaller zooplankton individuals (<1 mm; e.g., Rotifera, Nauplii) were analysed using a microscope (Olympus CX41 and URA Technic XSZ107BN) by taking two subsamples of 1 ml, from which the mean was calculated.
Phytoplankton was not sampled in this experiment. We expect the phytoplankton species at the start of the experiment to be similar to previous studies performed with the same water source, including chlorophyta, cryptophyta, cyanobacteria, desmids, diatoms, dinophyta (Polazzo et al., 2022b; Polazzo et al., 2022a).
Zooplankton total abundance, taxa richness, and taxa diversity (Shannon-Wiener Index) were calculated with the software R (version 4.1.0 2021-05-18) using the Vegan (Oksanen et al., 2020), BiodiversityR (Kindt and Coe, 2005), and Lawstat (Hui et al., 2008) packages. Next, two-factorial ANOVAs followed by post-hoc tests (Tukey HSD) were applied to identify differences between the treatments and days. The same methods were applied to identify significant differences in chlorophyll-a and PE concentration between treatments and days. The ANOVA and Tukey HSD tests were performed with the JMP® software (Pro 15.0.0). A Principal Component Analysis (PCA) was used to visualise differences in community structure between the controls and the treatments. Differences in multivariate community composition between the treatments were assessed by means of distance-based Redundancy Analysis (dbRDA). Both PCA and dbRDA were carried out using the Vegan package (Oksanen et al., 2020). We used the function “rankindex” of the package Vegan (Oksanen et al., 2020) to evaluate which distance matrix fitted better the data. In all cases, Euclidean distances ranked higher than any other distance matrix and, thus, were used to perform the dbRDA analysis.
Measured TER concentrations corresponded closely to the intended concentrations in the TER (mean ± standard error: 15.0 ± 0.5 µg/L) and HW + TER (15.0 ± 0.4 µg/L) treatments at the start of the experiment (Supplementary Table S3). Next, concentrations of TER slightly decreased to 13.5 ± 0.3 µg/L on day 7 for TER and 14.7 ± 0.3 µg/L for HW + TER, and to 12.0 ± 0.2 µg/L and 12.8 ± 0.2 µg/L for the TER and HW + TER treatments, respectively, on day 21 (Supplementary Table S3). No traces of TER were detected in the controls nor in the HW treatments.
At the beginning of the experiment, the dissolved oxygen concentration ranged between 8.4 and 8.8 mg/L and decreased to 6.1–7.8 mg/L at the end of the experiment. The HW caused a dissolved oxygen decline on day 7, showing the lowest values for that sampling day (6.4 mg/L in the HW treatment and 6.3 mg/L in the HW + TER treatment; Supplementary Table S3). The analysed nutrient concentrations confirmed that the microcosms simulated oligotrophic systems (Baban, 1996; Dodds et al., 1998), with total N and total P concentrations ranging from <0.05 to 1.1 (± 0.6) mg/L and 0.37 (± 0.05) to 16.74 (± 6.8) µg/L, respectively (Supplementary Table S4). Chlorophyll-a concentrations in the microcosms did not differ significantly between treatments at the beginning of the experiment, with 0.45 (±0.2) µg/L in the control, 0.59 (±0.4) µg/L in HW, 0.71 (±0.6) µg/L in TER and 0.56 (±0.3) µg/L in the HW + TER treatments. The application of the HW or the TER treatment as single stressors did not result in a significant deviation in chlorophyll-a concentration relative to the controls for any of the sampling days. However, chlorophyll-a concentrations in the combined HW + TER treatment increased from 0.56 (± 0.3) µg/L at the beginning of the experiment to 1.4 (±1.1) µg/L after 14 days and showed significant differences to all other treatments over the entire period of the experiment: control (p = 0.001), HW (p = 0.029), and TER (p = 0.013) (Figure 2).
FIGURE 2. Chlorophyll-a concentrations [µg/L] measured over time in all treatments: control (blue), heatwave (HW, red), terbuthylazine (TER, green), and the combination of a heatwave and terbuthylazine (HW + TER, yellow). The shaded areas indicate the standard error from the mean. The points indicate the data points and the dashed lines connect the means of these data points. The data points were slightly shifted to avoid overlapping and allow optimal visualisation.
At the start of the experiment, PE concentrations were 8.51 (±0.3) µg/L in the control, 8.47 (±0.2) µg/L in the HW, 8.69 (±0.3) µg/L in the TER, and 8.41 (±0.1) µg/L in the HW + TER treatment, showing non-significant differences between treatments. PE concentrations dropped significantly (p < 0.001) from an average of 8.5 (±0.2) µg/L among all treatments on day 0 to an average of 2.1 (±0.8) µg/L among all treatments after the first week and remained stable in all treatments until the end of the experiment (Figure 3). Overall, the treatment HW + TER differed significantly from the control (p = 0.013), the HW (p = 0.013), and the TER treatments (p = 0.049). On day 14, the HW + TER treatment had two times higher PE concentrations compared to the other treatments (Figure 3).
FIGURE 3. Phycoerythrin concentrations [µg/L] measured over time in all treatments: control (blue), heatwave (HW, red), terbuthylazine (TER, green), and the combination of HW and TER (HW + TER, yellow). The shaded area indicates the standard error from the mean. The points indicate the data points and the dashed lines connect the means of these data points. The data points were slightly shifted to avoid overlapping and allow optimal visualisation.
The following taxonomic groups were identified in the test microcosms: Alona spp., Alonella spp., Brachionus spp., Cephalodella spp., Ceriodaphnia spp., Chydorus spp., Colurella spp., Cyclopidae spp., Daphnia longispina, Daphnia pulex, Diaphanosoma spp., Diaptomus spp., Euchlanis spp., Keratella spp., Lecanidae spp., Lepadella spp., Mytilinia spp., Nauplii, Ostracoda spp., Polyarthra spp., Simocephalus spp., and Testudinella spp. Nauplii were considered as one single group as it was difficult to differentiate species at young stage, although it could have included different taxa. Over all treatments and time points, Nauplii, Chydorus spp., Lecanidae spp., and Alona spp. were the most abundant taxa and were identified as dominant groups.
The PCA showed a separation of the HW treatment in respect to the others for the days 7 and 14, indicating that mainly the abundance of Alona spp., Chydorus spp., D. longispina, and Nauplii increased with the HW treatment on day 7 (Figure 4A). However, on day 14, most taxa (i.e., Alonella spp., Ceriodaphnia spp., Chydorus spp., D. longispina, D. pulex, Lepadella spp., Mytilinia spp., Nauplii, Testudinella spp.) showed an abundance increase in the HW treatment (Figure 4B) and decreased in the TER and the TER + HW treatments. No clear differences were found between treatments for day 0 (start of the experiment) or day 21 (recovery period).
FIGURE 4. Principal Component Analysis (PCA) indicating the relationship between the treatments (control, heatwave (HW), terbuthylazine (TER), and the combination (HW + TER)) and the individual taxa. The length and direction of the arrows indicate the affinity of each stressor for each taxon, respectively. (A) PCA of day 7 (B) PCA of day 14.
Although the HW showed an abundance increase of most taxa, the dbRDA did not show a significant effect of the HW treatment on the zooplankton community on day 0 (p = 0.582), 7 (p = 0.165), 14 (p = 0.800), or 21 (p = 0.441). In contrast to days 0, 7, and 14, there was moderate evidence (p = 0.068) on day 14 in which the TER treatment differed from the other treatments. These observations correspond with the dissimilarities in the PCA, i.e., the separation between the control and the TER samples (Figure 4B). Such dissimilarities can be mainly explained by a decrease in the abundance of the majority of zooplankton taxa in this treatment. Still, there was no evidence that HW + TER had an interactive effect on the community (p = 0.741).
At the beginning of the experiment, the total abundance in the control (167 ± 54 individuals/L) was similar to that in the rest of the treatments: HW (213 ± 62 individuals/L), TER (146 ± 60 individuals/L), and HW + TER (132 ± 58 individuals/L). The two-factorial ANOVA revealed significant differences in the overall experiment on the total abundance by the treatments (p = 0.02) and sampling day (p < 0.001). The interaction term (treatment*day) was not statistically significant (p = 0.31). The HW treatment differed significantly from the control (p = 0.03) and the TER treatment (p = 0.03). As indicated for the PCA analysis, there was an increase in the total abundance in the HW treatment from 213 (±62) individuals/L on day 0 to 546 (±281) individuals/L on day 7 (3-fold higher than control and 2-fold higher than TER). The combined treatment (HW + TER) did not significantly affect the total abundance, yet, a trend towards lower total abundance on day 14 was observed (Figure 5A). The total abundance on day 7 was significantly different to day 0 (p < 0.001), 14 (p = 0.0028), and 21 (p = 0.0008) (Figure 5A). The relative abundance changed slightly over time with a shift from cyclopoid nauplii dominance towards Chydorus spp. on day 14 throughout all treatments (Figure 6). On day 7, both HW treatments showed an increase in smaller taxa and a reduction in larger taxa (Supplementary Figure S1).
FIGURE 5. (A) Total zooplankton abundance was measured over time in all treatments: control (blue), heatwave (HW, red), terbuthylazine (TER, green), and the combination of heatwave and terbuthylazine (HW + TER, yellow). (B) Taxa richness was measured over time in all treatments: control (blue), heatwave (HW, red), terbuthylazine (TER, green), and the combination of heatwave and terbuthylazine (HW + TER, yellow). (C) Taxa diversity was measured using the Shannon-Wiener Index over time in all treatments: control (blue), heatwave (HW, red), terbuthylazine (TER, green), and the combination of heatwave and terbuthylazine (HW + TER, yellow). The shaded areas indicate the standard error from the mean. The points indicate the data points and the dashed lines connect the means of these data points. The data points were slightly shifted to avoid overlapping and allow optimal visualisation.
FIGURE 6. Relative abundance of zooplankton taxa in the treatments (control, heatwave (HW), terbuthylazine (TER), and their combination (HW + TER)) on each sampling day (i.e., 0–21).
Taxa richness on day 0 did not differ between the control (5.75 ± 1.25), HW (8 ± 1.63), TER (6 ± 0.81) or HW + TER (6.25 ± 0.5), respectively. There were overall significant effects on taxa richness by sampling day (p=<0.0001), but not by treatment level (p = 0.72) (Figure 5B). The taxa richness in the TER treatment increased by 2-fold from day 0 (6 ± 0.81) to day 7 (11.5 ± 1.7) (p = 0.005). A difference in taxa richness for HW + TER on day 14 was observed, although not statistically significant to the other treatments (control p = 0.80, HW p = 0.74, TER p = 0.97) (Figure 5B).
At the start of the experiment, the zooplankton diversity (Shannon-Wiener Index) was similar in the control (0.6 ± 0.4), HW (0.72 ± 0.1), TER (0.62 ± 0.1), and HW + TER (0.64 ± 0.3) treatments (Figure 5C). In all treatments, the taxa diversity increased over the course of the experiment resulting in a higher Shannon-Wiener Index on day 21: control (1.17 ± 0.5), HW (0.77 ± 0.2), TER (0.95 ± 0.3), and HW + TER (1.27 ± 0.4), respectively. The sampling day had an overall significant effect (p = 0.0004) on the taxa diversity. The post-hoc comparison showed that the first sampling on day 0, differed to day 7 (2-fold increase; p = 0.0006), 14 (p = 0.002), and 21 (p = 0.01), respectively. No significant differences in diversity between the treatments were identified.
Our study shows that the simulated 7-day HW affected the total chlorophyll-a concentration and resulted in an increase in the abundance of zooplankton. We assume the increase in zooplankton abundance was related to the increase of palatable phytoplankton. Smaller individuals, which usually have shorter reproductive cycles, responded faster to the metabolic increase caused by the HW (Gillooly et al., 2001; Brown et al., 2004; Dam, 2013). The observed general increase in smaller zooplankton taxa after the HW was accompanied by a reduction in large Cladocera and an increase in small Cladocera taxa compared to the control, which might be related to the fact that smaller bodied species are advantaged under warming (Daufresne et al., 2009) (Supplementary Figure S1). The temperature-driven rise in zooplankton metabolism and subsequent abundance increase may have caused the decline in chlorophyll-a on day 14 by higher feeding rates on phytoplankton. These results confirm findings from a previous microcosm study by O’Connor and colleagues in which the zooplankton biomass increased with elevated temperature and led to phytoplankton decrease due to amplified grazing pressure (O’Connor et al., 2009). However, in the present study only abundance and not biomass was measured, which might be less comparable, as abundance is not always correlated to biomass (Cardoso et al., 2012).
TER was expected to limit the food availability for the zooplankton community and, consequently, indirectly cause a decline in zooplankton abundance (Pereira et al., 2017) as the herbicide was not expected to be acutely toxic to the zooplanktonic community. These indirect effects on the zooplankton community structure particularly reduced the relative abundance of some taxa. Although we did not identify a decline in chlorophyll-a caused by TER, a significant shift in the zooplanktonic community composition and a decline in zooplankton abundance in the TER treatment was observed. A previous study described a phytoplankton community composition shift from dominant (i.e., Chloromonas angustissima, Navicula lanceolata, and Gomphonema parvulum) towards less-sensitive species (i.e., Chroococcus turgidus, Peridinium willei, and Trachelomonas spp.) when exposed to TER at concentrations that were 6 times lower than in our study (Wijewardene et al., 2021).
It seems likely that edible phytoplankton (e.g., Scenedesmus spp. or Cyclotella sp.) were replaced by more grazing-resistant phytoplankton (e.g., Anabaena or Leptolyngbya spp.). Findings from other studies (e.g., Hasenbein et al., 2017), in which a sudden change in stressor pressure resulted in a phytoplankton community shift towards more resistant phytoplankton taxa, support our hypothesis. We conclude that both the decline in zooplankton abundance and the shift in community composition were likely driven indirectly by the direct effect of TER on phytoplankton.
The combined application of both stressors (HW + TER) was expected to cause additive or synergistic effects on the zooplankton fitness, as observed in other studies assessing pesticide effects under increasing temperature scenarios (e.g., Vilas-Boas et al., 2021), owing to the cumulative energy demand caused by both stressors (Dinh et al., 2016). The combined effects were projected to be larger than the effects of the single stressors acting in isolation (Folt et al., 1999). The combination of both stressors caused a significant increase in chlorophyll-a concentration, which could be classified as a positive synergistic effect, since neither the HW nor TER influenced the chlorophyll-a levels when applied as single stressors (Piggott et al., 2015). This positive synergistic effect may have been caused by a shift in the phytoplankton community composition. Interestingly, despite the increase in chlorophyll-a, no significant effect on the zooplankton community was observed.
Cyanobacteria produce not only chlorophyll-a but also PE (Sidler, 1994). The increase in PE and chlorophyll-a in the HW + TER treatment is therefore indicative of cyanobacteria growth and may explain the chlorophyll-a increase despite the herbicide treatment. Increased temperature has been shown to advantage cyanobacteria over green algae when simultaneously exposed to photosynthesis-inhibiting herbicides (Lürling and Roessink, 2006). In contrast, lower temperatures can increase the sensitivity of cyanobacteria towards a herbicide, as shown in an experimental study where higher and lower temperatures were compared directly (Bérard et al., 1999). These findings may explain why cyanobacteria growth did not increase when TER was applied as a single stressor. Hence, we assume that cyanobacteria benefited from the HW + TER treatment and the sufficient availability of NO3- and N(NO3-) which was stable throughout the experiment (Supplementary Table S4), leading to a rapid growth in the lack of strong competition with other algae (Carey et al., 2012; Paerl and Otten, 2013). An increase in cyanobacteria may also have led to a spread of toxic bioactive compounds to prevent zooplankton grazing on them (Lyu et al., 2016), leading to lower reproduction rates (Zhou et al., 2020). We assume that the combined effect of HW and TER led to a reduction of primary productivity in green algae and to a lower relative abundance of palatable phytoplankton, as observed in comparable studies (Allen et al., 2021; Rumschlag et al., 2021), which may have caused food limitation for zooplankton, and, consequently, a decreased zooplankton abundance (Rumschlag et al., 2021).
We conclude that the positive effect of the HW expressed as zooplankton abundance increase, was eliminated when the HW was applied in combination with TER. The increase of chlorophyll-a and PE in the HW + TER treatment suggests that cyanobacteria were not affected by TER in the combined HW + TER treatment (Forlani et al., 2008), as indicated by the positive effect of the HW on cyanobacteria growth (e.g., see Calderó-Pascual et al., 2020; Urrutia-Cordero et al., 2020) potentially outweighing the negative impacts of TER. It may be that cyanobacteria even benefitted from the TER treatment (Lürling and Roessink, 2006), as cyanobacteria (e.g., Microcystis aeruginosa EC50 = 0.016 mg/L) have been demonstrated to be less sensitive toward TER than green algae (e.g., Pseudokirchneriella subcapitata EC50 = 0.012 mg/L) (European Food and Safety Authority, 2011), which strengthens the result that cyanobacteria were not significantly affected by TER.
Overall, the planktonic community used in our experiment was found to be resilient to the combined HW and TER application. Although air temperatures may not reflect water temperatures, it should be noted that the planktonic community was collected in an area with strong seasonality (−13.7°C minimum to 42.8°C maximum air temperatures in 2021, AEMET: Agencia Estatal de Meteorología, 2021), and, thus, might have been used to a wide range of temperature regimes. Further studies should investigate whether the vulnerability of zooplanktonic communities to HWs and other stressors varies between communities adapted to fluctuating temperature conditions and those collected in regions with a more stable climate.
Here we investigated the interactive effects of HWs and chemical stress events co-occurring in a freshwater experimental set-up. However, spatiotemporal variation in these stress events can yield asynchronous exposure scenarios whose impacts are challenging to predict (Jackson et al., 2021). Further research should be dedicated to assessing multiple stressor effects applied in different sequences and intensities, as well as to providing mechanistic understanding and models to quantify their propagation across different levels of biological organization.
We exposed a freshwater planktonic community to a simulated HW, the herbicide TER, and the combination of both stressors (HW + TER). The mechanistic understanding of the study outcomes was somewhat limited by not generating data on the direct impact of HW and TER on the phytoplanktonic community. Yet, our results demonstrate that the HW treatment increased the zooplankton abundance of most taxa, while TER decreased the abundance of some of them (e.g., Nauplii, Lepadella spp., Mytilinia spp.) in the long term (i.e., day 14). The indirect effects caused by TER on the zooplankton community were related to changes in the phytoplankton community structure and the proliferation of cyanobacteria. The effects caused by the combination of both stressors on zooplankton were additive and resembled the ones exerted by the TER treatment. However, HW + TER seemed to potentiate a shift in the phytoplankton community structure. Thus, future studies assessing the combination of both stressors (HW + TER) on phytoplankton and their propagation to higher trophic levels would help to better understand the consequences of multiple stressors on freshwater ecosystems.
The raw data supporting the conclusions of this article will be made available by the authors, without undue reservation.
Conceptualization: SR, AR, AS, and FP; Methodology: AR, FP, SR, AS, and AG-A; Material and logistics: AR and AS; Investigation: SR, FP, AG-A, and LC; Data analysis and interpretation: SR, FP, AR, and AS; Writing—original draft: SR, FP, AR, and AS; Writing—review and editing: all authors.
This project received funding from the EU Horizon 2020 program under the Marie Skłodowska-Curie Innovative Training Network ECORISK 2050 (grant agreement No 813124) and the CICLIC project (Smart tools and technologies to assess the environmental fate and risks of Contaminants under Climate Change), funded by the Spanish Ministry of Science, Innovation and Universities (RTI 2018_097158_A_C32). AR is supported by the Talented Researcher Support Programme—Plan GenT (CIDEGENT/2020/043) of the Generalitat Valenciana.
The authors would like to thank Melina Crettaz Minaglia for her support in zooplankton identification and Claudia Martínez Megías and Theresa Schell for their support in the experiment.
The authors declare that the research was conducted in the absence of any commercial or financial relationships that could be construed as a potential conflict of interest.
All claims expressed in this article are solely those of the authors and do not necessarily represent those of their affiliated organizations, or those of the publisher, the editors and the reviewers. Any product that may be evaluated in this article, or claim that may be made by its manufacturer, is not guaranteed or endorsed by the publisher.
The Supplementary Material for this article can be found online at: https://www.frontiersin.org/articles/10.3389/fenvs.2022.920010/full#supplementary-material
AEMET: Agencia Estatal de Meteorología (2021). Valores extremos. Available at: http://www.aemet.es/es/serviciosclimaticos/datosclimatologicos/efemerides_extremos*?w=0&k=mad&l=3175&datos=det&x=3175&m=8&v=TMX (Accessed 2, 17, 22).
Allen, J., Gross, E. M., Courcoul, C., Bouletreau, S., Compin, A., Elger, A., et al. (2021). Disentangling the direct and indirect effects of agricultural runoff on freshwater ecosystems subject to global warming: A microcosm study. Water Res. 190, 116713. doi:10.1016/j.watres.2020.116713
American Public Health Association (2005). Standard methods for the examination of water and wastewater. 21st ed. Washington, DC: American water works association/american public works association/water environment federation.
Andrade, V. S., Gutierrez, M. F., Fantón, N. I., and Gagneten, A. M. (2018). Shifts in zooplankton behavior caused by a mixture of pesticides. Water, Air, Soil Pollut. 229, 107. doi:10.1007/s11270-018-3752-y
Arenas‐Sánchez, A., López‐Heras, I., Nozal, L., Vighi, M., and Rico, A. (2019). Effects of increased temperature, drought, and an insecticide on freshwater zooplankton communities. Environ. Toxicol. Chem. 38, 396–411. doi:10.1002/etc.4304
Baban, S. M. J. (1996). Trophic classification and ecosystem checking of lakes using remotely sensed information. Hydrological Sci. J. 41, 939–957. doi:10.1080/02626669609491560
Bérard, A., Leboulanger, C., and Pelte, T. (1999). Tolerance of oscillatoria limnetica lemmermann to atrazine in natural phytoplankton populations and in pure culture: Influence of season and temperature. Arch. Environ. Contam. Toxicol. 37, 472–479. doi:10.1007/s002449900541
Brown, J. H., Gillooly, J. F., Allen, A. P., Savage, V. M., and West, G. B. (2004). Toward a metabloic theory of ecology. Ecology 85, 1771–1789. doi:10.1890/03-9000
Calderó-Pascual, M., de Eyto, E., Jennings, E., Dillane, M., Andersen, M. R., Kelly, S., et al. (2020). Effects of consecutive extreme weather events on a temperate dystrophic lake: A detailed insight into physical, chemical and biological responses. Water 12, 1411. doi:10.3390/w12051411
Cardoso, S. J., Roland, F., Loverde-Oliveira, S. M., and Huszar, V. L. de M. (2012). Phytoplankton abundance, biomass and diversity within and between Pantanal wetland habitats. Limnologica 42, 235–241. doi:10.1016/j.limno.2012.01.002
Carey, C. C., Ibelings, B. W., Hoffmann, E. P., Hamilton, D. P., and Brookes, J. D. (2012). Eco-physiological adaptations that favour freshwater cyanobacteria in a changing climate. Water Res. 46, 1394–1407. doi:10.1016/j.watres.2011.12.016
Dam, H. G. (2013). Evolutionary adaptation of marine zooplankton to global change. Ann. Rev. Mar. Sci. 5, 349–370. doi:10.1146/annurev-marine-121211-172229
Daufresne, M., Lengfellner, K., and Sommer, U. (2009). Global warming benefits the small in aquatic ecosystems. Proc. Natl. Acad. Sci. U. S. A. 106, 12788–12793. doi:10.1073/pnas.0902080106
Dinh, K. V., Janssens, L., and Stoks, R. (2016). Exposure to a heat wave under food limitation makes an agricultural insecticide lethal: A mechanistic laboratory experiment. Glob. Chang. Biol. 22, 3361–3372. doi:10.1111/gcb.13415
Dodds, W. K., Jones, J. R., and Welch, E. B. (1998). Suggested classification of stream trophic state: Distributions of temperate stream types by chlorophyll, total nitrogen, and phosphorus. Water Res. 32, 1455–1462. doi:10.1016/S0043-1354(97)00370-9
EC (2007). Draft assessment report (DAR) - initial risk assessment provided by the rapporteur member state United Kingdom for the existing active substance terbuthylazine of the third stage. Part B, article 8(2). Brussels: European Commission.
European Food and Safety Authority (2011). Conclusion on the peer review of the pesticide risk assessment of the active substance terbuthylazine. EFSA J. 9, 1969. doi:10.2903/j.efsa.2011.1969
Ferrando, M. D., and Andreu-Moliner, E. (1991). Acute lethal toxicity of some pesticides to Brachionus calyciflorus and Brachionus plicatilis. Bull. Environ. Contam. Toxicol. 47, 479–484. doi:10.1007/BF01702214
Folt, C. L., Chen, C. Y., Moore, M. V., and Burnaford, J. (1999). Synergism and antagonism among multiple stressors. Limnol. Oceanogr. 44, 864–877. doi:10.4319/lo.1999.44.3_part_2.0864
Forlani, G., Pavan, M., Gramek, M., Kafarski, P., and Lipok, J. (2008). Biochemical bases for a widespread tolerance of cyanobacteria to the phosphonate herbicide glyphosate. Plant Cell Physiol. 49, 443–456. doi:10.1093/pcp/pcn021
Gillooly, J. F., Brown, J. H., West, G. B., Savage, V. M., and Charnov, E. L. (2001). Effects of size and temperature on metabolic rate. Science 293, 2248–2251. doi:10.1126/science.1061967
Hanazato, T. (2001). Pesticide effects on freshwater zooplankton: An ecological perspective. Environ. Pollut. 112, 1–10. doi:10.1016/S0269-7491(00)00110-X
Hari, R. E., Livingstone, Da.M., Siber, R., Burkhardt-Holm, P., and Güttinger, H. (2006). Consequences of climatic change for water temperature and brown trout populations in Alpine rivers and streams. Glob. Chang. Biol. 12, 10–26. doi:10.1111/j.1365-2486.2005.001051.x
Hasenbein, S., Lawler, S. P., and Connon, R. E. (2017). An assessment of direct and indirect effects of two herbicides on aquatic communities. Environ. Toxicol. Chem. 36, 2234–2244. doi:10.1002/etc.3740
Hjermann, D., Bogstad, B., Eikeset, A. M., Ottersen, G., Gjøsæter, H., and Stenseth, N. C. (2007). Food web dynamics affect Northeast Arctic cod recruitment. Proc. R. Soc. B 274, 661–669. doi:10.1098/rspb.2006.0069
Hui, W., Gel, Y. R., and Gastwirth, J. L. (2008). Lawstat : An R package for law, public policy and biostatistics. J. Stat. Softw. 28. doi:10.18637/jss.v028.i03
Jackson, M. C., Pawar, S., and Woodward, G. (2021). The temporal dynamics of multiple stressor effects: From individuals to ecosystems. Trends Ecol. Evol. xx, 402–410. doi:10.1016/j.tree.2021.01.005
Jentsch, A., Kreyling, J., and Beierkuhnlein, C. (2007). A new generation of climate-change experiments: Events, not trends. Front. Ecol. Environ. 5, 365–374. doi:10.1890/1540-9295(2007)5[365:ANGOCE]2.0.CO;2
Kindt, R., and Coe, R. (2005). Tree diversity analysis; A manual and software for common statistical methods for ecological and biodiversity studies. Nairobi, Kenya: World Agroforestry Centre.
Lürling, M., and Roessink, I. (2006). On the way to cyanobacterial blooms: Impact of the herbicide metribuzin on the competition between a green alga (Scenedesmus) and a cyanobacterium (Microcystis). Chemosphere 65, 618–626. doi:10.1016/j.chemosphere.2006.01.073
Lyu, K., Meng, Q., Zhu, X., Dai, D., Zhang, L., Huang, Y., et al. (2016). Changes in iTRAQ-based proteomic profiling of the cladoceran Daphnia magna exposed to microcystin-producing and microcystin-free microcystis aeruginosa. Environ. Sci. Technol. 50, 4798–4807. doi:10.1021/acs.est.6b00101
Moore, M., and Folt, C. (1993). Zooplankton body size and community structure: Effects of thermal and toxicant stress. Trends Ecol. Evol. 8, 178–183. doi:10.1016/0169-5347(93)90144-E
Navis, S., Waterkeyn, A., Voet, T., De Meester, L., and Brendonck, L. (2013). Pesticide exposure impacts not only hatching of dormant eggs, but also hatchling survival and performance in the water flea Daphnia magna. Ecotoxicology 22, 803–814. doi:10.1007/s10646-013-1080-y
Noyes, P. D., McElwee, M. K., Miller, H. D., Clark, B. W., Van Tiem, L. A., Walcott, K. C., et al. (2009). The toxicology of climate change: Environmental contaminants in a warming world. Environ. Int. 35, 971–986. doi:10.1016/j.envint.2009.02.006
O’Connor, M. I., Piehler, M. F., Leech, D. M., Anton, A., and Bruno, J. F. (2009). Warming and resource availability shift food web structure and metabolism. PLoS Biol. 7, e1000178. doi:10.1371/journal.pbio.1000178
OECD (2000). Guidance document on aquatic toxicity testing of difficult substances and mixtures. Series on Testing and Assessment, No. 23. Paris, France: Organization for Economic Cooperation and Development. EnV/JM/MONO.
Oksanen, J., Blanchet, F. G., Friendly, M., Kindt, R., Legendre, P., McGlinn, D., et al. (2020). vegan: Community Ecology Package. R package version 2.5-7. Available at: https://CRAN.R-project.org/package=vegan.
Oliveira dos Anjos, T. B., Polazzo, F., Arenas‐Sánchez, A., Cherta, L., Ascari, R., Migliorati, S., et al. (2021). Eutrophic status influences the impact of pesticide mixtures and predation on Daphnia pulex populations. Ecol. Evol. 11, 4046–4057. doi:10.1002/ece3.7305
Pace, M. L., and Orcutt, J. D. (1981). The relative importance of protozoans, rotifers, and crustaceans in a freshwater zooplankton community. Limnol. Oceanogr. 26, 822–830. doi:10.4319/lo.1981.26.5.0822
Paerl, H. W., and Otten, T. G. (2013). Harmful cyanobacterial blooms: Causes, consequences, and controls. Microb. Ecol. 65, 995–1010. doi:10.1007/s00248-012-0159-y
Pereira, A. S., Cerejeira, M. J., and Daam, M. A. (2017). Toxicity of environmentally realistic concentrations of chlorpyrifos and terbuthylazine in indoor microcosms. Chemosphere 182, 348–355. doi:10.1016/j.chemosphere.2017.05.032
Perkins, D. M., McKie, B. G., Malmqvist, B., Gilmour, S. G., Reiss, J., and Woodward, G. (2010). Environmental warming and biodiversity–ecosystem functioning in freshwater microcosms: Partitioning the effects of species identity, richness and metabolism. London, UK: Academic Press by Elsevier Adv. Ecol. Res. 43, 177–209. doi:10.1016/B978-0-12-385005-8.00005-8
Piggott, J. J., Townsend, C. R., and Matthaei, C. D. (2015). Reconceptualizing synergism and antagonism among multiple stressors. Ecol. Evol. 5, 1538–1547. doi:10.1002/ece3.1465
Polazzo, F., dos Anjos, T. B. O., Arenas-Sánchez, A., Romo, S., Vighi, M., and Rico, A. (2022a). Effect of multiple agricultural stressors on freshwater ecosystems: The role of community structure, trophic status, and biodiversity-functioning relationships on ecosystem responses. Sci. Total Environ. 807, 151052. doi:10.1016/j.scitotenv.2021.151052
Polazzo, F., Marina, T., Crettaz-Minaglia, M., and Rico, A. (2022b). Food web rewiring drives long-term compositional differences and late-disturbance interactions at the community level. Proc. Natl. Acad. Sci. U. S. A. 119 (17), e2117364119. doi:10.1073/pnas.2117364119
Polazzo, F., Roth, S. K., Hermann, M., Mangold‐Döring, A., Rico, A., Sobek, A., et al. (2021). Combined effects of heatwaves and micropollutants on freshwater ecosystems: Towards an integrated assessment of extreme events in multiple stressors research. Glob. Chang. Biol. 28, 1248–1267. doi:10.1111/gcb.15971
Pomati, F., Shurin, J. B., Andersen, K. H., Tellenbach, C., and Barton, A. D. (2020). Interacting temperature, nutrients and zooplankton grazing control phytoplankton size-abundance relationships in eight Swiss lakes. Front. Microbiol. 10, 3155–3217. doi:10.3389/fmicb.2019.03155
PPDB: Pesticide Properties DataBase (2022). Terbuthylazine. Available at: https://sitem.herts.ac.uk/aeru/ppdb/en/Reports/623.htm (Accessed 9 3, 22).
Rasconi, S., Gall, A., Winter, K., and Kainz, M. J. (2015). Increasing water temperature triggers dominance of small freshwater plankton. PLoS One 10, e0140449. doi:10.1371/journal.pone.0140449
Rasconi, S., Winter, K., and Kainz, M. J. (2017). Temperature increase and fluctuation induce phytoplankton biodiversity loss - evidence from a multi-seasonal mesocosm experiment. Ecol. Evol. 7, 2936–2946. doi:10.1002/ece3.2889
Regaudie-de-Gioux, A., and Duarte, C. M. (2012). Temperature dependence of planktonic metabolism in the ocean. Glob. Biogeochem. Cycles 26. doi:10.1029/2010GB003907
Rumschlag, S. L., Casamatta, D. A., Mahon, M. B., Hoverman, J. T., Raffel, T. R., Carrick, H. J., et al. (2021). Pesticides alter ecosystem respiration via phytoplankton abundance and community structure: Effects on the carbon cycle? Glob. Chang. Biol. 28, 1091–1102. doi:10.1111/gcb.15952
Sass, J. B., and Colangelo, A. (2006). European union bans atrazine, while the United States negotiates continued use. Int. J. Occup. Environ. Health 12, 260–267. doi:10.1179/oeh.2006.12.3.260
Schiedek, D., Sundelin, B., Readman, J. W., and Macdonald, R. W. (2007). Interactions between climate change and contaminants. Mar. Pollut. Bull. 54, 1845–1856. doi:10.1016/j.marpolbul.2007.09.020
Sidler, W. A. (1994). “Phycobilisome and phycobiliprotein structures,” in The molecular biology of cyanobacteria (Dordrecht: Springer Netherlands), 139–216. doi:10.1007/978-94-011-0227-8_7
Soulié, T., Vidussi, F., Mas, S., and Mostajir, B. (2022). Functional stability of a coastal mediterranean plankton community during an experimental marine heatwave. Front. Mar. Sci. 9. doi:10.3389/fmars.2022.831496
Stipičević, S., Galzina, N., Udiković-Kolić, N., Jurina, T., Mendaš, G., Dvoršćak, M., et al. (2015). Distribution of terbuthylazine and atrazine residues in crop-cultivated soil: The effect of herbicide application rate on herbicide persistence. Geoderma 259-260, 259300–260309. doi:10.1016/j.geoderma.2015.06.018
Till, A., Rypel, A. L., Bray, A., and Fey, S. B. (2019). Fish die-offs are concurrent with thermal extremes in north temperate lakes. Nat. Clim. Chang. 9, 637–641. doi:10.1038/s41558-019-0520-y
Tilman, D., Fargione, J., Wolff, B., D’Antonio, C., Dobson, A., Howarth, R., et al. (2001). Forecasting agriculturally driven global environmental change. Science 292, 281–284. doi:10.1126/science.1057544
Twiss, M. R., Brahmstedt, E. S., Cabana, G., and Guillemette, F. (2022). Proliferation of phytoplankton along a 500 km transect of the st. Lawrence river from its outflow at lake ontario. J. Gt. Lakes. Res. 48, 336–342. doi:10.1016/j.jglr.2022.01.007
United States Environmental Protection Agency (1995). Reregistration eligibility decision ( RED ) terbuthylazine. Washington, DC: Office of Prevention, Pesticides and Toxic Substances.
Urrutia-Cordero, P., Zhang, H., Chaguaceda, F., Geng, H., and Hansson, L. (2020). Climate warming and heat waves alter harmful cyanobacterial blooms along the benthic–pelagic interface. Ecology 101, 030255–e3112. doi:10.1002/ecy.3025
Vilas-Boas, J. A., Arenas-Sánchez, A., Vighi, M., Romo, S., Van den Brink, P. J., Pedroso Dias, R. J., et al. (2021). Multiple stressors in Mediterranean coastal wetland ecosystems: Influence of salinity and an insecticide on zooplankton communities under different temperature conditions. Chemosphere 269, 129381. doi:10.1016/j.chemosphere.2020.129381
Wenneker, M., Beltman, W. H. J., De Werd, H., van Zeeland, M. G., van der Lans, A. M., and Van der Weide, R. Y. (2010). Quantifying point source entries of pesticides in surface waters. Asp. Appl. Biol. 99, 69–74.
Wijewardene, L., Wu, N., Qu, Y., Guo, K., Messyasz, B., Lorenz, S., et al. (2021). Influences of pesticides, nutrients, and local environmental variables on phytoplankton communities in lentic small water bodies in a German lowland agricultural area. Sci. Total Environ. 780, 146481. doi:10.1016/j.scitotenv.2021.146481
Winder, M., Berger, S. A., Lewandowska, A., Aberle, N., Lengfellner, K., Sommer, U., et al. (2012). Spring phenological responses of marine and freshwater plankton to changing temperature and light conditions. Mar. Biol. 159, 2491–2501. doi:10.1007/s00227-012-1964-z
Woolway, R. I., Jennings, E., Shatwell, T., Golub, M., Pierson, D. C., and Maberly, S. C. (2021). Lake heatwaves under climate change. Nature 589, 402–407. doi:10.1038/s41586-020-03119-1
Zhou, Q., Lu, N., Gu, L., Sun, Y., Zhang, L., Huang, Y., et al. (2020). Daphnia enhances relative reproductive allocation in response to toxic microcystis: Changes in the performance of parthenogenetic and sexual reproduction. Environ. Pollut. 259, 113890. doi:10.1016/j.envpol.2019.113890
Keywords: multiple stressors, heatwaves, microcosms, freshwater invertebrates, global change, terbuthylazine
Citation: Roth SK, Polazzo F, García-Astillero A, Cherta L, Sobek A and Rico A (2022) Multiple stressor effects of a heatwave and a herbicide on zooplankton communities: Implications of global climate change. Front. Environ. Sci. 10:920010. doi: 10.3389/fenvs.2022.920010
Received: 14 April 2022; Accepted: 24 October 2022;
Published: 10 November 2022.
Edited by:
Adit Chaudhary, University of California, San Diego, United StatesReviewed by:
Pablo Henrique Dos Santos Picapedra, Universidade Estadual do Oeste do Paraná, BrazilCopyright © 2022 Roth, Polazzo, García-Astillero, Cherta, Sobek and Rico. This is an open-access article distributed under the terms of the Creative Commons Attribution License (CC BY). The use, distribution or reproduction in other forums is permitted, provided the original author(s) and the copyright owner(s) are credited and that the original publication in this journal is cited, in accordance with accepted academic practice. No use, distribution or reproduction is permitted which does not comply with these terms.
*Correspondence: Sabrina K. Roth, U2FicmluYS5yb3RoQGFjZXMuc3Uuc2U=
Disclaimer: All claims expressed in this article are solely those of the authors and do not necessarily represent those of their affiliated organizations, or those of the publisher, the editors and the reviewers. Any product that may be evaluated in this article or claim that may be made by its manufacturer is not guaranteed or endorsed by the publisher.
Research integrity at Frontiers
Learn more about the work of our research integrity team to safeguard the quality of each article we publish.