- 1School of Environment, Resources and Sustainability, University of Waterloo, Waterloo, ON, Canada
- 2Department of Natural Resource Sciences, McGill University, Sainte-Anne-de-Bellevue, QC, Canada
- 3Ecohydrology Research Group, Department of Earth and Environmental Sciences and Water Institute, University of Waterloo, Waterloo, ON, Canada
In temperate cold regions, the gradual resurgence of soil microbial activity during spring freeze-thaw events is frequently associated with greenhouse gas emissions. Enhanced greenhouse gas fluxes during spring freeze-thaw are related to the mineralization of bioavailable substrates, which may be elevated when soil is amended with organic residues (e.g., biobased residues such as compost, digestate, biosolids). The objective of this study was to determine the impact of biobased residues, compared to urea fertilizer, on greenhouse gas emissions during spring freeze-thaw events. The field treatments included urea (170 kg N ha−1 y−1), composted food waste (240 kg N ha−1 y−1), hydrolyzed biosolids (215 kg N ha−1 y−1), and anaerobic digestate (231 kg N ha−1 y−1). Headspace gases were sampled from a closed static chamber in each replicate plot (n = 4) and categorized with three transient spring freeze-thaw phases (waterlogged, wet, and dry). Among the treatments, nitrous oxide (N2O) flux was significantly different (p < 0.05) where compost had the highest emission and digestate lowest while carbon dioxide (CO2) and methane (CH4) fluxes were not significantly different (p > 0.05). The greenhouse gas fluxes were significantly different among the freeze-thaw events (p < 0.05) likely due to intense microbial activity and anaerobic conditions. The CO2 and CH4 emissions were related to N2O emission (p < 0.05), and soil temperature strongly correlated with CO2 fluxes. This suggested that soil warming driven by ambient conditions as well as the type and quantity of carbon input influenced soil microbial activity, leading to greenhouse gases production. Therefore, soil amended with biobased residues may either increase or reduce greenhouse gas fluxes during spring freeze-thaw events depending on the source and production method of the organic material.
Introduction
In temperate cold regions, spring freeze-thaw events contribute to the production of greenhouse gases, which further exacerbates climate change (Pelster et al., 2013; Natali et al., 2019). Greenhouse gas production during spring freeze-thaw account for 29–73% of the annual N2O (Hung et al., 2021), 5–50% of the annual CO2 (Kurganova et al., 2007), and ∼80% of the annual CH4 (Song et al., 2012). In temperate agroecosystems, greenhouse gas fluxes peak during spring freeze-thaw events due to carbon (C) and reactive nitrogen (N) remaining in the soil from the previous crop season (i.e., legacy C and N) (Wagner-Riddle et al., 2017). Accordingly, amending the soil with organic residues, high in C and N, increases the production and fluxes of greenhouse gases considerably during spring freeze-thaw events (Kariyapperuma et al., 2012; Chantigny et al., 2016). Furthermore, intermittent greenhouse gas fluxes during freeze-thaw events are also related to temporal changes in soil biophysical conditions influenced by snow melting, waterlogging, and gradual warming and drying (Congreves et al., 2018).
Biobased residues are biological wastes that include, but are not limited to, compost (e.g., food waste), biosolids, and anaerobic digestate. Biobased residues are comprised of readily accessible organic C and N, thereby enhancing soil microbial activity and greenhouse gas productions (Thangarajan et al., 2013). Furthermore, organic matter, nutrient concentration, as well as physical characteristics (e.g., composts are solid, biosolids are semi-liquid and anaerobic digestates are liquid) can vary substantially among biobased residue types (Bertora et al., 2008; Grave et al., 2018; Badewa and Oelbermann, 2020). Differences in biobased residue composition also influence the soil C and N dynamics and microbial activity, which regulates greenhouse gas production (Miura et al., 2019; Rosinger et al., 2022).
Since spring freeze-thaw influences the soil’s biophysical conditions, the presence of readily available C and N substrates to the microbial community from biobased residues can cause nitrification, incomplete denitrification or methanogenesis, resulting in the generation of greenhouse gases (Zhu-Barker et al., 2015; Brenzinger et al., 2018). Additionally, temperature fluctuation and moisture variation (waterlogged, wet, and dry) during spring freeze-thaw, where the soil has minimal or no snow cover, causes frequent and intense episodes of freezing and thawing coupled with physical deformation of soil aggregates (Miao et al., 2014; Wu et al., 2017; Wu et al., 2021). Furthermore, substantial soil warming during the thaw phase increases the microbial activity and triggers greenhouse gas emissions (Henry, 2008; Henry, 2013; Chantigny et al., 2019).
Although multiple studies determined the impact of biobased residues on greenhouse gas productions (Thangarajan et al., 2013; Insam et al., 2015; Roman-Perez et al., 2021), our knowledge on the impact of biobased residues on spring freeze-thaw events and resultant greenhouse gas fluxes remains limited. However, knowing the contribution of biobased residues to greenhouse gas fluxes is critical since biological and physicochemical properties, in addition to soil management (e.g., the rate and timing of biobased residue application, soil cultivation) and environmental characteristics (e.g., soil type, weather), vary at the local and regional scale (Dambreville et al., 2008; Eckard et al., 2010; Grave et al., 2018). Although some studies found that freeze-thaw events can cause a shift in soil physical conditions and how biobased residues influence this shift and respond to greenhouse gas production, but they have only been evaluated under laboratory conditions and with inconsistent results (Sahin et al., 2008; Liu et al., 2016; Fu et al., 2019; Pupko, 2019; Hou et al., 2020). Under field conditions, Roman-Perez et al. (2021) found that soil amended with contrasting biosolids types (composted, anaerobic digested, alkaline stabilized) varied in their production of N2O. Roman-Perez et al. (2021) also found that N2O fluxes from biobased residues were higher compared to those from urea. Consequently, knowledge on the impact of biobased residues on greenhouse gas production during spring freeze-thaw events in a field setting is warranted (Hung et al., 2021).
The objectives of our study were to quantify and compare greenhouse gas fluxes from soil amended with biobased residues and N fertilizer during the spring freeze-thaw events. We hypothesized that N2O, CO2 and CH4 fluxes from soil amended with biobased residues will be greater compared to soil amended with N fertilizer (urea) during spring freeze-thaw since a greater quantity of C and N are available from the biobased residues than from urea. We also hypothesized that N2O and CH4 fluxes will be greater during the waterlogged and wet phase due to denitrification and methanogenesis caused by anaerobioses, whereas the CO2 flux will be greater during the drying phase due to enhanced microbial activity caused by a rising soil temperature. We also assumed that the CO2 flux is strongly related to soil temperature throughout the spring freeze-thaw.
Materials and Methods
Site Description and Field Experiment
The field site was located at Elora Research Station (43o45′N, 80o21′W), Elora, Ontario, Canada. The mean annual temperature at this site is 6.8°C, with mean annual precipitation of 989 mm and 6 cm snow depth, based on a 16-year data (2004–2019) from the Environment and Climate Change Canada (2021). At this field site, soils undergo spring freeze-thaw from March to April when snow begins to melt. This creates an environment with transitory waterlogging, a partial ice layer, and wet and drying conditions (Supplementary Table S1). Daily ambient temperature fluctuations during the spring cause repeated freeze thaw cycles, and the daily average maximum and minimum temperatures in this site were 6.9 to −3.2°C between March 1 and April 30 from 2004 to 2020 (Environment and Climate Change Canada, 2021). Soil at the site is classified as a grey brown Luvisol, with a silt loam approximately 29% sand, 52% silt and 19% clay. The soil (0–20 cm depth) has 25 g soil organic C kg−1, 2.4 g total N kg−1, and pH of 7.9.
The field experiment was established with four different treatments including urea, compost, biosolid, and digestate and applied in a completely randomized block design. Urea was a commercial granular urea fertilizer that contained 46% N applied at a rate of 170 kg N ha−1. Compost was composted food waste applied at 12 t ha−1 (240 kg N ha−1) (Table 1). Biosolid (LysteGro®) was hydrolyzed biosolid slurry applied at 28,000 L ha−1 (215 kg N ha−1). Digestate was liquid anaerobic digestate of farm green plant applied at 42,000 L ha−1 (231 kg N ha−1). Biobased residues and urea were applied in May of each cropping season. All the treatment plots received 27 kg P2O5 ha−1 using triple superphosphate (0-46-0) and 55 kg K2O ha−1 potassium chloride (0-0-60) according to the local agronomic requirement (Table 1). The site was under a maize (Zea mays L.)—soybean (Glycine max) rotation, where maize was seeded in 2018 and 2020 and soybean in 2019. Crop grain was removed and the remaining aboveground biomass (stalks and leaves) and belowground residues (roots) were incorporated to a 15 cm with an offset disc harrow. However, for this study, we focused on the impact of spring thaw event on the greenhouse gas emission of the four different treatments applied in May 2020 during the spring of 2021 (March specifically). The effects of biobased residues on greenhouse gas emissions during growing and non-growing seasons is explored further in a related manuscript (Badewa et al., unpublished).
The effect of ambient temperature, snow depth, and precipitation were obtained from a weather station located at the research station. Soil temperature at ∼10 cm depth was measured by temperature sensors buried in one replicate of each treatment (n = 4). Air and soil temperature followed a similar pattern from October to mid-November but were distinctly different from the end of November to mid-March when the soil was covered with snow for 95 days (Supplementary Figure S1). During this period, the snow cover (∼49 cm) and crop residues left on the soil insulated and regulated the soil temperature (0–10 cm depth) to ∼0°C with precipitation ranging from ∼29 mm (Supplementary Figure S1).
Greenhouse Gas Fluxes
Greenhouse gas fluxes were measured from 11 March to 24 March 2021 using closed static chambers. The static chamber bottom (15 cm long and 56 cm diameter) was constructed of a light grey colored polyvinyl chloride pipe and inserted into the soil depth of 10 cm, leaving 5 cm headspace above the soil surface. Chamber caps consisted of a 1 cm diameter opening fitted with rubber septa for gas sampling and a 10 cm long vent tube (9 mm inner diameter) to account for pressure differences during sample collection (Parkin and Venterea, 2010). After fitting the bottom and top cap, chamber volume was 30.2 L, with a 0.08 m2 cross-sectional area. One permanent base was placed randomly in soil for each treatment replicate in October 2020 before soil freezing. The first measurement occurred on 11 March 2021, when the chamber bottom in the treatment plots were visible—not covered with snow and had <5 cm of snow. The final measurement occurred on 24 March 2021, 7 days after the snow and ice melted disappears. We were not able to record moisture due to freezing soil state and data logger issues, but we categorized our measurement days into three freeze-thaw phases using the visual moisture state observed during greenhouse gas measurement (Supplementary Table S1). First freeze-thaw phase (waterlogged) occurred when the soil was covered with melting snow and saturated. The second freeze-thaw phase (wet) occurred immediately after the snow and ice melted and when mostly wet, followed by third freeze-thaw phase (dry) that was mostly dry with wet patches (Supplementary Table S1).
Gas samples were extracted daily from each treatment replicate. To control for sinusoidal diurnal temperature variations, sampling was conducted between 10:00-h and 16:00-h (Smith et al., 2003). Gas sampling (30 ml) was carried out at 0, 10, 20 and 30 min after placing the chamber cap and transferred into pre-evacuated 12 ml glass Exetainers (LabCorp Ltd., Lampeter, Wales, United Kingdom). The collected gas samples were analyzed on a Bruker 450 gas chromatograph (Bruker Corporation, Billerica, MA, United States) fitted with a thermal conductivity detector for CO2, a flame ionization detector for CH4, and an electron capture detector for N2O analysis. The gas concentration (ppm) measured by gas chromatograph was converted to mass-based concentration with the Ideal Gas Law using the relative molecular mass of C (12 g mol−1) or N (28 g mol−1) (Pedersen, 2017). The fluxes (µg m−2 min−1) were then estimated using the HMR package in R (version 4.1.2). Cumulative emission of the gases and the global warming potential over 100 years (GWP) during the spring freeze-thaw events (11 March to 24 March 2021) were calculated using Eqs. 1, 2.
Where CE is the cumulative emission of soil greenhouse gas (µg m−2/mg m−2) and Fi is the greenhouse gas flux of the ith sampling time (µg m−2 h−1). The GWP (kg CO2 eq ha−1) was calculated using:
Where
Statistical Analysis
All data processing and analysis were carried out in R v.4.2.1 (R Core Team, 2020). Data did not meet assumption of normality, so data were analyzed by non-parametric statistics. The effect of treatment and the freeze thaw phases on greenhouse gas flux and emission was determined with a Kruskal wallis test and the treatment comparison was done with a Dunn test (p < 0.05). The relationship between greenhouse gas emission and soil or air temperature was determined using Pearson correlation coefficients (r). The relationship between greenhouse gas fluxes and soil temperature was evaluated using Pearson correlation coefficients (r) and exponential model.
Results
Temperature Conditions and Greenhouse Gas Fluxes
Soil temperature among treatments was identical (p > 0.05) during the spring freeze-thaw (Figure 1). Soil and air temperature varied among the freeze thaw phases (p < 0.05) with average soil temperature during greenhouse gas sampling highest for dry (7°C), followed by wet (2°C) and lowest for waterlogged (0°C) (Figure 1). Cumulative N2O emission were different among treatments (p = 0.020) while cumulative CO2 and CH4 emissions were not different among treatments (p > 0.05) (Table 2). When comparing N2O emission among treatments, the average loss was highest in compost (669 µg N2O-N m2), followed by urea (419 µg N2O-N m2), biosolids (259 µg N2O-N m2), and digestate (105 µg N2O-N m2) (p < 0.05). The global warming potential varied among treatments but was not significantly different (p > 0.05), where urea had the highest (127 kg CO2 ha−1) and digestate the lowest (55 kg CO2 ha−1) warming potential (Table 2). The greenhouse gas emission varied significantly among the freeze thaw phases (p < 0.05) with peak N2O and CO2 fluxes during the wet, and peak CH4 flux during the dry (Figure 2). N2O flux ranged from −388 to 454 µg N2O-N m−2 h−1, CO2 flux from −211 to 565 mg CO2-C m−2 h−1, and CH4 flux −1285–216 µg CH4-C m−2 h−1. However, mean N2O flux was highest during the wet (31 µg N2O m−2 h−1) and lowest during waterlogged (−2 µg N2O-N m−2 h−1), mean CO2 flux was highest flux during the dry (34 mg CO2 -C m−2 h−1) and lowest during waterlogged (5 mg CO2 -C m−2 h−1) while mean CH4 flux was highest during the wet (−11 µg CH4-C m−2 h−1) and lowest during waterlogged (−24 µg CH4-C m−2 h−1).
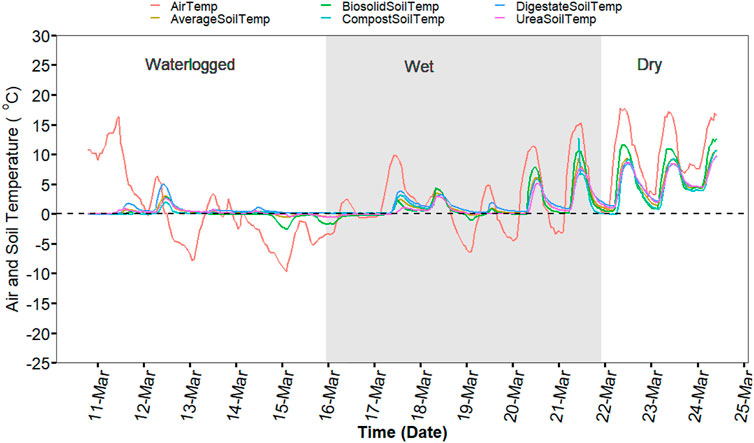
FIGURE 1. Hourly soil temperature from the four treatment plots (∼10 cm) and air temperature during the experiment period (from 11 March 2020 to 24 March 2021).

TABLE 2. Cumulative greenhouse gas emission and global warming potential from soils amended with urea and biobased residues during the 2021 spring freeze-thaw event.
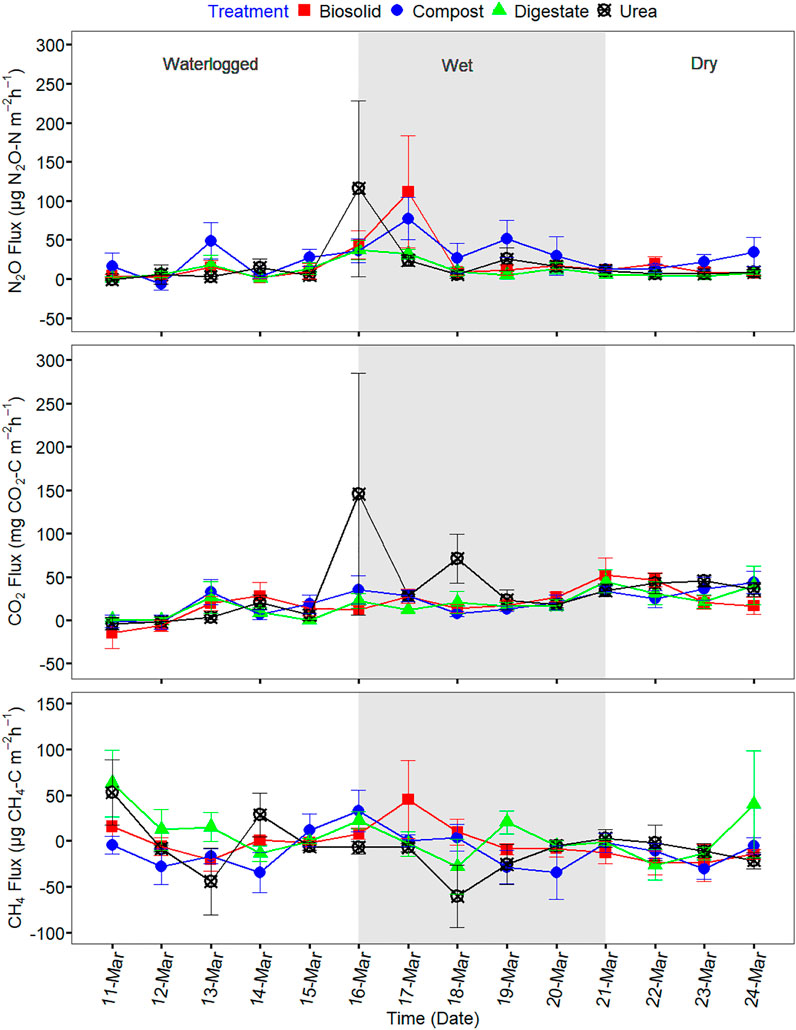
FIGURE 2. Greenhouse gas fluxes of N2O, CO2, and CH4 during the spring freeze-thaw event of 11 March to 24 March 2021.
Relationship Between Greenhouse Gas Emissions, Air, and Soil Temperature
N2O emission had a moderate positive correlation with CO2 emission (p = 0.000), weak positive correlation with CH4 emission (p = 0.006) but weak non-significant correlation with soil, or air temperature (p > 0.05) (Table 3). CO2 emission had a weak positive correlation with soil temperature (p = 0.032), weak positive correlation with air temperature (p = 0.042) but was not significantly correlated with CH4 emission (p > 0.05) (Table 3). CH4 emission was not significantly correlated to CO2 emission, and soil and air temperature (p > 0.05) except CH4 emission (p = 0.006) (Table 3). Relationship between greenhouse gas fluxes and soil temperature were estimated using exponential model (Figure 3; Table 4) (Luo et al., 2013; Natali et al., 2019). The CO2 flux was positively correlated with soil temperature for compost, biosolid, and digestate and when averaging all four treatments (p < 0.05) (Figure 3A). However, N2O and CH4 fluxes were generally not significantly correlated with soil temperature (p > 0.05) (Figures 3B,C).
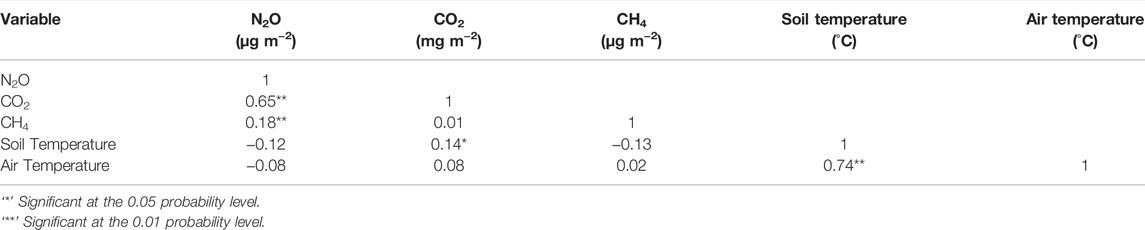
TABLE 3. Correlation values for the greenhouse gas fluxes and emissions and soil and ambient temperature during the spring freeze-thaw events (n = 224).
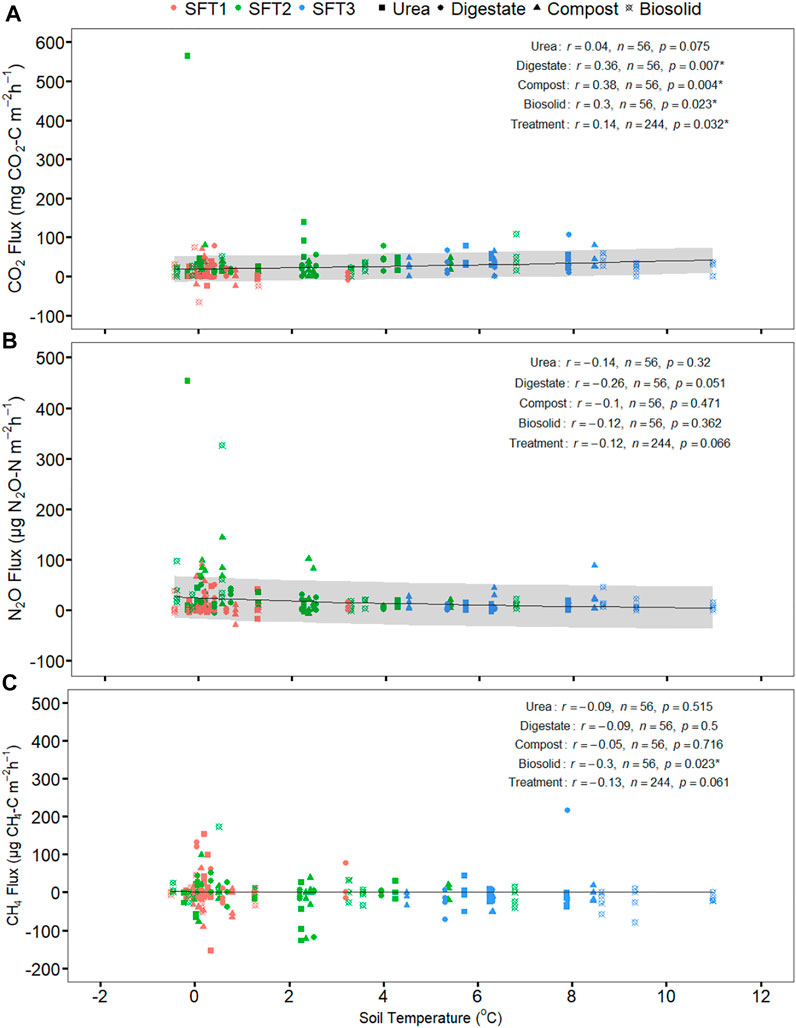
FIGURE 3. The effect of soil temperature (∼10 cm) on (A) CO2 (B) N2O (C) CH4 fluxes release from the soil amended with urea and biobased residues during the spring freeze thaw event on 11 March to 24 March 2021. The graph presented the exponential model fitted (solid gray line) and the standard deviation of an exponential model fitted (gray shading) between greenhouse gas flux per treatment and soil temperature during the spring freeze thaw event (note: CH4 flux standard deviation was not achieved due to iterative error caused by the many negative integers). The Pearson correlation coefficients (r) and the significant level (p) are shown for the treatments. SFT1 is the first spring freeze-thaw phase (waterlogged), SFT2 is the second spring freeze-thaw phase (wet) and SFT3 is the third spring freeze-thaw phase (dry). * Shows p-value < 0.05.
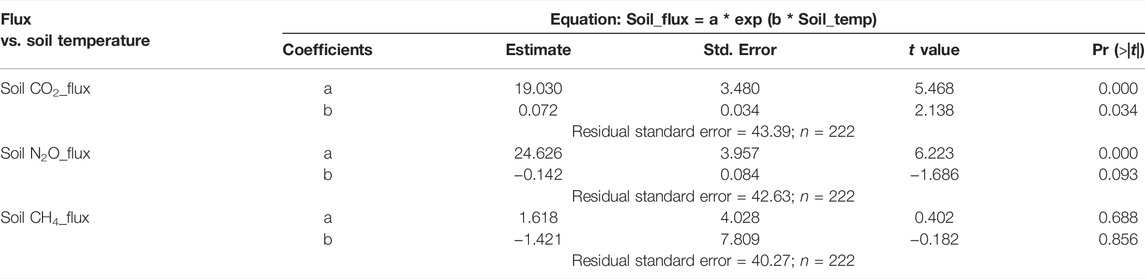
TABLE 4. Relationship between greenhouse gas fluxes and soil temperature (∼10 cm) using exponential model during the 2021 spring freeze-thaw events.
Discussion
Influence of Soil Amendments on Greenhouse Gas Fluxes During Spring Freeze-Thaw
Nitrous oxide emission from compost amended soil was notably different from that of biosolid and digestate. This was likely due to the variation in C and N availability (Table 1), and therefore microbial activity among the different biobased residues evaluated in our study. We found that compost had a high N2O flux compared to the other treatments, although this biobased residue has the lowest N mineralization potential (Table 1). This possibly occurred because compost has the highest application rate that supplied more C and N for N2O production, and highest organic C and lowest N composition which takes the longest to fully mineralized. Thus, compost-treated plot has the highest residual C and N in the next year spring when the experiment was carried out. Similarly, Kariyapperuma et al. (2012) found also that the variable composition of composted swine influenced N2O emissions during non-growing season that undergoes spring freeze thaw. Generally, composts with higher organic C and available N content cause greater N2O fluxes and as the compost C:N ratio increases, N2O fluxes decreases (Santos et al., 2021) suggesting chemical composition of compost could regulate soil N2O fluxes.
The lower than expected N2O flux for anaerobic digestate in our study (Table 2) was due to the presence of readily degradable C and the physical quality (i.e., liquid state of this residue type). Our findings were also consistent with other studies that found lower N2O fluxes with digested materials that were in a liquid state compared to either undigested materials (e.g., solid state) or mineral fertilizer (Petersen 1999; Börjesson and Berglund, 2007; Chantigny et al., 2007; Collins et al., 2011; Rodhe et al., 2015; Baral et al., 2017). It has been shown that the low soluble organic C in anaerobic digestate leads to a lower source of energy for denitrifiers causing a reduction in the production of N2O (Vallejo et al., 2006; Nkoa, 2014). Furthermore, digestates contain high soluble N that is readily available for plant uptake (De Vries et al., 2012; Baral et al., 2017). In our study, we found that ∼98% of the anaerobic digestate was in the mineralized form and was probably taken-up by the maize crop planted in the 2020 growing season (data not shown).
Some previous studies showed that during biosolid production and throughout the first month of its application to agricultural soil, volatile N (e.g., ammonia) is lost from the soil (e.g., Yoshida et al., 2015; Roman-Perez et al., 2021). In our study, lower N2O flux observed was likely caused by volatilization of ammonia from the biosolid treatment. Furthermore, the biosolid used in our study was obtained from Lystek that uses a thermal hydrolysis process that disintegrates the cell walls of microbes and hydrolyses complex macromolecules into simpler compounds (Lystek, 2021). The use of heat, shearing, and alkalinity during thermal hydrolysis can cause the cell walls to lyse and it reduces the abundance of denitrifying bacteria, while C substrates persist and contribute to CO2 and/or CH4 emissions (Knowles, 1982; Murray et al., 2019). Results from our study also show that the N mineralization rate of the Lystek biosolid was ∼50% and indicate that most of the available N was taken-up by the maize during growing season (data not shown).
We also found a positive relationship between N2O versus CO2 and CH4 emission among treatments which indicated that C input from biobased residues and urea stimulated the production of N2O (Table. 3). Faubert et al. (2019) also found a positive relationship between N2O and CO2 emission and suggested that C input stimulated N2O emission either by reducing soil oxygen availability through increased soil respiration, or by the direct use of C from biobased residues as a source of energy for hetrotophic denitrifiers (Wrage-Mönnig et al., 2018). Since biobased residues can be distinctly different due to variation in their feedstock and/or production process, the quality of C (e.g., lignin content) and its N mineralization rate also varies (Ejack and Whalen, 2021). However, Roman-Perez et al. (2021) found that regardless of biobased residue type, the processes of C mineralization is similar, whereby increased C availability enhances microbial respiration and raises CO2 and CH4 fluxes (Roman-Perez et al., 2021). Furthermore, in our study N mineralization likely increased available N which was transformed to N2O via nitrification and complete denitrification (Grant et al., 2020).
Although the cumulative CO2 and CH4 emissions, and the global warming potential did not differ among treatments (p > 0.05), we observed a wide range in their values among treatments (Table 2). This was due to differences in nutrient availability, especially the availability of C during the spring freeze-thaw, among treatments (Adviento-Borbe et al., 2007; Brenzinger et al., 2018). Our results also supported the growing view among researchers that treatment comparisons should be explained using variability and magnitude effects and not only be limited to statistical significances (Wasserstein et al., 2019; King et al., 2021). Furthermore, results from our study that showed variation in greenhouse gas fluxes among treatments during the spring freeze-thaw, also highlights the importance of incorporating negative greenhouse gas fluxes. Since most studies in temperate environments do not account for greenhouse gas fluxes during winter and early spring (Yan et al., 2016; Kim et al., 2019; Natali et al., 2019), our study showed the importance of accounting for negative fluxes, especially in fertilized agroecosystems, since soil produces and consumes greenhouse gases (Hung et al., 2021).
Freeze Thaw Phases and Temperature Influence on Greenhouse Gas Fluxes
We found that soil freezing, and thawing combined with changes in air and soil temperature caused a high degree of variability in CO2 fluxes in all treatments. This indicated that soil warming regulated and enhanced microbial respiration during the spring freeze-thaw (Byun et al., 2021; King et al., 2021; Rafat et al., 2021). We found that CO2 fluxes were different among freeze-thaw phases where the dry phase had highest flux compared to the wet and waterlogged phases. This was due to the variation in soil microbial respiration, which was enhanced when the soil became warmer (Ganjurjav et al., 2016; Gao et al., 2020). Furthermore, we found that soil temperature was lowest during the waterlogged phase (∼−0°C) compared to the wet (∼2°C) and dry (∼7°C) phases. Natali et al. (2019) also found that warming during freeze-thaw allowed for the short-term mineralization of organic residues which caused the release of CO2.
In addition, the intensity of soil respiration during freeze-thaw is positively dependent on soil temperature and moisture (Schipper et al., 2014; Byun et al., 2021). Since no crops or plants were present over the winter, the CO2 flux quantified in our study was a direct measurement of soil microbial activity (Hung et al., 2021). Although we were not able to measure moisture in our study, we found that the significant relationship between temperature and CO2 flux was a measure of the intensity of microbial metabolism even when temperature drop close to zero (−1–2°C) during the waterlogged and wet phase (Schaefer and Jafarov, 2016). This is because temperature controls a range of biogeochemical processes that regulate soil C cycling (Flanagan et al., 2013). In a temperate forest ecosystem, Yan et al. (2016) found a significant and positive relationship between soil CO2 flux and soil temperature during the spring freeze-thaw.Furthermore, Zou et al. (2018) summarized that multiple field studies found a significant and positive relationship between soil respiration intensity and soil temperature and soil moisture. However, to our knowledge, our study is the first to quantify the relationship between CO2 flux and soil temperature from various biobased residues during the spring freeze-thaw under field conditions.
We also found that soil freezing, and thawing caused a high degree of variability in N2O and CH4 fluxes indicating anaerobiosis caused by soil moisture state of the phases contributed to the variability of the fluxes. The freeze thaw phases in our study were determined with the visual moisture state and the N2O flux during the wet phase was significantly highest compared to the waterlogged and dry phase. This was likely due to the release of trapped N2O in soil pores during the wet phase. The waterlogged phase causes soil pores with transient liquid water and ice to enable microbes to undergo denitrification that produces N2O (Kim et al., 2012; Congreves et al., 2018). The N2O produced could however be trapped because of high tortuosity created by water and ice in the pores (Hung et al., 2021; King et al., 2021) and gradually release when the waterlogged phase transitioned to the wet phase where ice seal was removed. In addition, CH4 flux during the wet phase was different and higher compared to dry but not the waterlogged phase. This suggests that the anaerobic and wet condition encourage microbial production of CH4 through methanogenesis (Luo et al., 2013). Further research should consider in-depth evaluation of the simultaneous contribution of spatial and temporal soil temperature and soil moisture measurements on NO2 and CH4 fluxes during spring freeze thaw in temperate agricultural land.
Conclusion
We evaluated the impact of biobased residues on greenhouse gas fluxes during the spring freeze-thaw. Greenhouse gas fluxes from biobased residues is not different compared to urea except for N2O emission from compost. There was a significant relationship between soil temperature and CO2 flux but no relationship with N2O and CH4 fluxes during the spring freeze thaw. Among the freeze thaw phases, the greenhouse gas fluxes were different. We found that the dry phase during freeze-thaw caused intensified CO2 fluxes compared to the wet and waterlogged freeze-thaw phase due to enhanced warming. We found that greenhouse gas fluxes from treatments with biobased residues and urea were controlled by the availability of C and N that regulated microbial processes of C mineralization and nitrification/denitrification. We propose that future studies should incorporate molecular techniques to elucidate the specific organisms responsible for C and N transformations during spring freeze-thaw events especially in the waterlogged and drying phases. This information will help to further understand the role of biobased residues versus N fertilizers in greenhouse gas fluxes and aid with management decisions with respect to the type and timing of biobased residue application.
Data Availability Statement
The original contributions presented in the study are included in the article/Supplementary Material, further inquiries can be directed to the corresponding authors.
Author Contributions
EB: design of experiment, data acquisition, data analysis, data interpretation, and drafting of manuscript. CY: data interpretation and revision of draft. FR: data interpretation and revision of draft. JW: design of experiment, data interpretation, and revision of draft. MO: design of experiment, data interpretation, and revision of draft.
Funding
Funding was provided by the Quebec-Ontario Cooperation for Agri-Food Research (project SR9323) supported by the Quebec Ministry of Agriculture, Fisheries and Food and the Ontario Ministry of Agriculture, Food and Rural Affairs.
Conflict of Interest
The authors declare that the research was conducted in the absence of any commercial or financial relationships that could be construed as a potential conflict of interest.
Publisher’s Note
All claims expressed in this article are solely those of the authors and do not necessarily represent those of their affiliated organizations, or those of the publisher, the editors and the reviewers. Any product that may be evaluated in this article, or claim that may be made by its manufacturer, is not guaranteed or endorsed by the publisher.
Acknowledgments
We thank Lystek International for providing Lystegro biosolid, AIM Environmental for providing the composted food waste, and BioEn for providing the anaerobic digestate. We also thank the Canadian Foundation for Innovation (CFI), the University of Waterloo and McGill University for providing research infrastructure. We thank Hicham Benslim (McGill University) for greenhouse gas sample analysis.
Supplementary Material
The Supplementary Material for this article can be found online at: https://www.frontiersin.org/articles/10.3389/fenvs.2022.909683/full#supplementary-material
References
Adviento-borbe, M. A. A., Haddix, M. L., Binder, D. L., Walters, D. T., and Dobermann, A. (2007). Soil Greenhouse Gas Fluxes and Global Warming Potential in Four High-Yielding Maize Systems. Glob. Change Biol. 13 (9), 1972–1988. doi:10.1111/j.1365-2486.2007.01421.x
Badewa, E., and Oelbermann, M. (2020). Achieving Soil Security Through Biobased Residues. World J. Agri. & Soil Sci. 5 (2).
Baral, K. R., Labouriau, R., Olesen, J. E., and Petersen, S. O. (2017). Nitrous Oxide Emissions and Nitrogen Use Efficiency of Manure and Digestates Applied to Spring Barley. Agric. Ecosyst. Environ. 239, 188–198. doi:10.1016/j.agee.2017.01.012
Bertora, C., Alluvione, F., Zavattaro, L., van Groenigen, J. W., Velthof, G., and Grignani, C. (2008). Pig Slurry Treatment Modifies Slurry Composition, N2O, and CO2 Emissions after Soil Incorporation. Soil Biol. Biochem. 40 (8), 1999–2006. doi:10.1016/j.soilbio.2008.03.021
Börjesson, P., and Berglund, M. (2007). Environmental Systems Analysis of Biogas Systems-Part II: The Environmental Impact of Replacing Various Reference Systems. Biomass Bioenergy 31 (5), 326–344. doi:10.1016/j.biombioe.2007.01.004
Brenzinger, K., Drost, S. M., Korthals, G., and Bodelier, P. L. E. (2018). Organic Residue Amendments to Modulate Greenhouse Gas Emissions from Agricultural Soils. Front. Microbiol. 9, 3035. doi:10.3389/fmicb.2018.03035
Byun, E., Rezanezhad, F., Fairbairn, L., Slowinski, S., Basiliko, N., Price, J. S., et al. (2021). Temperature, Moisture and Freeze-Thaw Controls on CO2 Production in Soil Incubations from Northern Peatlands. Sci. Rep. 11 (1), 1–15. doi:10.1038/s41598-021-02606-3
Chantigny, M. H., Angers, D. A., Rochette, P., Bélanger, G., Massé, D., and Côté, D. (2007). Gaseous Nitrogen Emissions and Forage Nitrogen Uptake on Soils Fertilized with Raw and Treated Swine Manure. J. Environ. Qual. 36 (6), 1864–1872. doi:10.2134/jeq2007.0083
Chantigny, M. H., Rochette, P., Angers, D. A., Goyer, C., Brin, L. D., and Bertrand, N. (2016). Nongrowing Season N2O and CO2 Emissions—Temporal Dynamics and Influence of Soil Texture and Fall-Applied Manure. Can. J. Soil Sci. 97 (3), 452–464. doi:10.1139/cjss-2016-0110
Chantigny, M. H., Bittman, S., Larney, F. J., Lapen, D., Hunt, D. E., Goyer, C., et al. (2019). A Multi-Region Study Reveals High Overwinter Loss of Fall-Applied Reactive Nitrogen in Cold and Frozen Soils. Can. J. Soil. Sci. 99 (2), 126–135. doi:10.1139/cjss-2018-0151
Collins, H. P., Alva, A. K., Streubel, J. D., Fransen, S. F., Frear, C., Chen, S., et al. (2011). Greenhouse Gas Emissions from an Irrigated Silt Loam Soil Amended with Anaerobically Digested Dairy Manure. Soil Sci. Soc. Am. J. 75 (6), 2206–2216. doi:10.2136/sssaj2010.0360
Congreves, K. A., Wagner-Riddle, C., Si, B. C., and Clough, T. J. (2018). Nitrous Oxide Emissions and Biogeochemical Responses to Soil Freezing-Thawing and Drying-Wetting. Soil Biol. Biochem. 117, 5–15. doi:10.1016/j.soilbio.2017.10.040
Dambreville, C., Morvan, T., and Germon, J. (2008). N2O Emission in Maize-Crops Fertilized with Pig Slurry, Matured Pig Manure or Ammonium Nitrate in Brittany. Agric. Ecosyst. Environ. 123 (1-3), 201–210. doi:10.1016/j.agee.2007.06.001
De Vries, J. W., Vinken, T. M. W. J., Hamelin, L., and De Boer, I. J. M. (2012). Comparing Environmental Consequences of Anaerobic Mono- and Co-digestion of Pig Manure to Produce Bio-Energy - A Life Cycle Perspective. Bioresour. Technol. 125, 239–248. doi:10.1016/j.biortech.2012.08.124
Eckard, R. J., Grainger, C., and De Klein, C. A. M. (2010). Options for the Abatement of Methane and Nitrous Oxide from Ruminant Production: A Review. Livest. Sci. 130 (1-3), 47–56. doi:10.1016/j.livsci.2010.02.010
Ejack, L., and Whalen, J. K. (2021). Freeze-thaw Cycles Release Nitrous Oxide Produced in Frozen Agricultural Soils. Biol. Fertil. Soils 57 (3), 389–398. doi:10.1007/s00374-020-01537-x
Environment and Climate Change Canada (2021). Daily Meteorological Data Report for Elora RCS Ontario. Available at: https://climate.weather.gc.ca/climate_data/daily_data_e.html?timeframe=2&Year=2018&Month=9&Day=27&hlyRange=2003-09-09%7C2018-09-27&dlyRange=2003-10-01%7C2018-09-26&mlyRange=2003-10-01%7C20061201&StationID=41983&Prov=ON&urlExtension=_e.html&searchType=stnProx&optLimit=yearRange&StartYear=2016&EndYear=2018&selRowPerPage=25&Line=3&txtRadius=25&optProxType=city&selCity=43%7C27%7C80%7C29%7CKitchener&selPark= (Accessed date: December 1, 2021).
Faubert, P., Bélisle, C. L., Bertrand, N., Bouchard, S., Chantigny, M. H., Paré, M. C., et al. (2019). Land Application of Pulp and Paper Mill Sludge May Reduce Greenhouse Gas Emissions Compared to Landfilling. Resour. Conservation Recycl. 150, 104415. doi:10.1016/j.resconrec.2019.104415
Flanagan, L. B., Sharp, E. J., and Letts, M. G. (2013). Response of Plant Biomass and Soil Respiration to Experimental Warming and Precipitation Manipulation in a Northern Great Plains Grassland. Agric. For. Meteorology 173, 40–52. doi:10.1016/j.agrformet.2013.01.002
Fu, Q., Yan, J., Li, H., Li, T., Hou, R., Liu, D., et al. (2019). Effects of Biochar Amendment on Nitrogen Mineralization in Black Soil with Different Moisture Contents under Freeze-Thaw Cycles. Geoderma 353, 459–467. doi:10.1016/j.geoderma.2019.07.027
Ganjurjav, H., Gao, Q., Gornish, E. S., Schwartz, M. W., Liang, Y., Cao, X., et al. (2016). Differential Response of Alpine Steppe and Alpine Meadow to Climate Warming in the Central Qinghai-Tibetan Plateau. Agric. For. Meteorology 223, 233–240. doi:10.1016/j.agrformet.2016.03.017
Gao, Y., Li, T., Fu, Q., Li, H., Liu, D., Ji, Y., et al. (2020). Biochar Application for the Improvement of Water-Soil Environments and Carbon Emissions under Freeze-Thaw Conditions: An In-Situ Field Trial. Sci. Total Environ. 723, 138007. doi:10.1016/j.scitotenv.2020.138007
Grant, R. F., Lin, S., and Hernandez-Ramirez, G. (2020). Modelling Nitrification Inhibitor Effects on N<sub>2</sub>O Emissions after Fall- and Spring-Applied Slurry by Reducing Nitrifier NH<sub>4</sub><sup>+</sup> Oxidation Rate. Biogeosciences 17 (7), 2021–2039. doi:10.5194/bg-17-2021-2020
Grave, R. A., Nicoloso, R. d. S., Cassol, P. C., da Silva, M. L. B., Mezzari, M. P., Aita, C., et al. (2018). Determining the Effects of Tillage and Nitrogen Sources on Soil N 2 O Emission. Soil Tillage Res. 175, 1–12. doi:10.1016/j.still.2017.08.011
Henry, H. A. L. (2008). Climate Change and Soil Freezing Dynamics: Historical Trends and Projected Changes. Clim. Change 87 (3), 421–434. doi:10.1007/s10584-007-9322-8
Henry, H. A. L. (2013). “Soil Freezing Dynamics in a Changing Climate: Implications for Agriculture,” in Plant and Microbe Adaptations to Cold in a Changing World (New York, NY: Springer), 17–27. doi:10.1007/978-1-4614-8253-6_2
Hou, R., Li, T., Fu, Q., Liu, D., Li, M., Zhou, Z., et al. (2020). Effects of Biochar and Straw on Greenhouse Gas Emission and its Response Mechanism in Seasonally Frozen Farmland Ecosystems. Catena 194, 104735. doi:10.1016/j.catena.2020.104735
Hung, C.-Y., Ejack, L., and Whalen, J. K. (2021). Fall-applied Manure with Cover Crop Did Not Increase Nitrous Oxide Emissions during Spring Freeze-Thaw Periods. Appl. Soil Ecol. 158, 103786. doi:10.1016/j.apsoil.2020.103786
Insam, H., Gómez-Brandón, M., and Ascher, J. (2015). Manure-based Biogas Fermentation Residues - Friend or Foe of Soil Fertility? Soil Biol. Biochem. 84, 1–14. doi:10.1016/j.soilbio.2015.02.006
Kariyapperuma, K. A., Furon, A., and Wagner-Riddle, C. (2012). Non-growing Season Nitrous Oxide Fluxes from an Agricultural Soil as Affected by Application of Liquid and Composted Swine Manure. Can. J. Soil. Sci. 92 (2), 315–327. doi:10.4141/cjss2011-059
Kim, D.-G., Vargas, R., Bond-Lamberty, B., and Turetsky, M. R. (2012). Effects of Soil Rewetting and Thawing on Soil Gas Fluxes: a Review of Current Literature and Suggestions for Future Research. Biogeosciences 9 (7), 2459–2483. doi:10.5194/bg-9-2459-2012
Kim, S. U., Ruangcharus, C., Kumar, S., Lee, H. H., Park, H. J., Jung, E. S., et al. (2019). Nitrous Oxide Emission from Upland Soil Amended with Different Animal Manures. Appl. Biol. Chem. 62 (1), 1–8. doi:10.1186/s13765-019-0409-5
King, A. E., Rezanezhad, F., and Wagner-Riddle, C. (2021). Evidence for Microbial rather Than Aggregate Origin of Substrates Fueling Freeze-Thaw Induced N2O Emissions. Soil Biol. Biochem. 160, 108352. doi:10.1016/j.soilbio.2021.108352
Kurganova, I., Teepe, R., and Loftfield, N. (2007). Influence of Freeze-Thaw Events on Carbon Dioxide Emission from Soils at Different Moisture and Land Use. Carbon Balance Manage 2 (1), 1–9. doi:10.1186/1750-0680-2-2
Liu, X., Wang, Q., Qi, Z., Han, J., and Li, L. (2016). Response of N2O Emissions to Biochar Amendment in a Cultivated Sandy Loam Soil during Freeze-Thaw Cycles. Sci. Rep. 6 (1), 1–9. doi:10.1038/srep35411
Luo, G. J., Kiese, R., Wolf, B., and Butterbach-Bahl, K. (2013). Effects of Soil Temperature and Moisture on Methane Uptake and Nitrous Oxide Emissions across Three Different Ecosystem Types. Biogeosciences 10 (5), 3205–3219. doi:10.5194/bg-10-3205-2013
Lystek (2021). Lystek THP-How it Works. Available at: https://lystek.com/technology/lystek-thp/.
Miao, S., Qiao, Y., Han, X., Brancher Franco, R., and Burger, M. (2014). Frozen Cropland Soil in Northeast China as Source of N2O and CO2 Emissions. PLoS One 9 (12), e115761. doi:10.1371/journal.pone.0115761
Miura, M., Jones, T. G., Hill, P. W., and Jones, D. L. (2019). Freeze-thaw and Dry-Wet Events Reduce Microbial Extracellular Enzyme Activity, but Not Organic Matter Turnover in an Agricultural Grassland Soil. Appl. Soil Ecol. 144, 196–199. doi:10.1016/j.apsoil.2019.08.002
Murray, R., Tien, Y.-C., Scott, A., and Topp, E. (2019). The Impact of Municipal Sewage Sludge Stabilization Processes on the Abundance, Field Persistence, and Transmission of Antibiotic Resistant Bacteria and Antibiotic Resistance Genes to Vegetables at Harvest. Sci. Total Environ. 651, 1680–1687. doi:10.1016/j.scitotenv.2018.10.030
Natali, S. M., Watts, J. D., Rogers, B. M., Potter, S., Ludwig, S. M., Selbmann, A.-K., et al. (2019). Large Loss of CO2 in Winter Observed across the Northern Permafrost Region. Nat. Clim. Chang. 9 (11), 852–857. doi:10.1038/s41558-019-0592-8
Nkoa, R. (2014). Agricultural Benefits and Environmental Risks of Soil Fertilization with Anaerobic Digestates: a Review. Agron. Sustain. Dev. 34 (2), 473–492. doi:10.1007/s13593-013-0196-z
Oelbermann, M., and Oelbermann, M. (2020). Achieving Soil Security through Biobased Residues. World J. Agric. Soil Sci. 5 (2), 2020. doi:10.33552/WJASS.2020.05.000607
Parkin, T. B., and Venterea, R. T. (2010). “Sampling Protocols—Chapter 3: Chamber-Based Trace Gas Flux Measurements,” in Sampling Protocols. Editor R. F. Follett (Fort Collins, Collorado, USA: USDA-ARS), 1–39. Available at: www.ars.usda.gov/research/GRACEnet.
Pelster, D. E., Chantigny, M. H., Rochette, P., Angers, D. A., Laganière, J., Zebarth, B., et al. (2013). Crop Residue Incorporation Alters Soil Nitrous Oxide Emissions during Freeze-Thaw Cycles. Can. J. Soil. Sci. 93 (4), 415–425. doi:10.4141/cjss2012-043
Petersen, S. O. (1999). Nitrous Oxide Emissions from Manure and Inorganic Fertilizers Applied to Spring Barley. J. Environ. Qual. 28 (No. 5), 1610–1618. doi:10.2134/jeq1999.00472425002800050027x
Pupko, J. M. (2019). Nitrous Oxide Release from Agricultural Soils under Different Management Practices during Freeze-Thaw Cycles. Thesis. Burlington: University of Vermont. ISSN: 2576-7550. Available at: https://scholarworks.uvm.edu/hcoltheses/292.
Rafat, A., Rezanezhad, F., Quinton, W. L., Humphreys, E. R., Webster, K., and Van Cappellen, P. (2021). Non-growing Season Carbon Emissions in a Northern Peatland Are Projected to Increase under Global Warming. Commun. Earth Environ. 2 (1), 1–12. doi:10.1038/s43247-021-00184-w
R Core Team (2020). “R: A Language and Environment for Statistical Computing,” in R Foundation for Statistical Computing (Vienna, Austria). Available at: https://www.R-project.org/
Rodhe, L. K. K., Ascue, J., Willén, A., Persson, B. V., and Nordberg, Å. (2015). Greenhouse Gas Emissions from Storage and Field Application of Anaerobically Digested and Non-digested Cattle Slurry. Agric. Ecosyst. Environ. 199, 358–368. doi:10.1016/j.agee.2014.10.004
Roman-Perez, C. C., Hernandez-Ramirez, G., Kryzanowski, L., Puurveen, D., and Lohstraeter, G. (2021). Greenhouse Gas Emissions, Nitrogen Dynamics and Barley Productivity as Impacted by Biosolids Applications. Agric. Ecosyst. Environ. 320, 107577. doi:10.1016/j.agee.2021.107577
Rosinger, C., Clayton, J., Baron, K., and Bonkowski, M. (2022). Soil Freezing-Thawing Induces Immediate Shifts in Microbial and Resource Stoichiometry in Luvisol Soils along a Postmining Agricultural Chronosequence in Western Germany. Geoderma 408, 115596. doi:10.1016/j.geoderma.2021.115596
Sahin, U., Angin, I., and Kiziloglu, F. M. (2008). Effect of Freezing and Thawing Processes on Some Physical Properties of Saline-Sodic Soils Mixed with Sewage Sludge or Fly Ash. Soil Tillage Res. 99 (2), 254–260. doi:10.1016/j.still.2008.03.001
Santos, C., Fonseca, J., Coutinho, J., Trindade, H., and Jensen, L. S. (2021). Chemical Properties of Agro-Waste Compost Affect Greenhouse Gas Emission from Soils through Changed C and N Mineralisation. Biol. Fertil. Soils 57 (6), 781–792. doi:10.1007/s00374-021-01560-6
Schaefer, K., and Jafarov, E. (2016). A Parameterization of Respiration in Frozen Soils Based on Substrate Availability. Biogeosciences 13 (7), 1991–2001. doi:10.5194/bg-13-1991-2016
Schipper, L. A., Hobbs, J. K., Rutledge, S., and Arcus, V. L. (2014). Thermodynamic Theory Explains the Temperature Optima of Soil Microbial Processes and High Q 10 Values at Low Temperatures. Glob. Change Biol. 20 (11), 3578–3586. doi:10.1111/gcb.12596
Smith, K. A., Ball, T., Conen, F., Dobbie, K. E., Massheder, J., and Rey, A. (2003). Exchange of Greenhouse Gases between Soil and Atmosphere: Interactions of Soil Physical Factors and Biological Processes. Eur. J. Soil Sci. 54, 779–791. doi:10.1046/j.1351-0754.2003.0567.x
Song, C., Xu, X., Sun, X., Tian, H., Sun, L., Miao, Y., et al. (2012). Large Methane Emission upon Spring Thaw from Natural Wetlands in the Northern Permafrost Region. Environ. Res. Lett. 7 (3), 034009. doi:10.1088/1748-9326/7/3/034009
Thangarajan, R., Bolan, N. S., Tian, G., Naidu, R., and Kunhikrishnan, A. (2013). Role of Organic Amendment Application on Greenhouse Gas Emission from Soil. Sci. Total Environ. 465, 72–96. doi:10.1016/j.scitotenv.2013.01.031
Vallejo, A., Skiba, U., Garciatorres, L., Arce, A., Lopezfernandez, S., and Sanchezmartin, L. (2006). Nitrogen Oxides Emission from Soils Bearing a Potato Crop as Influenced by Fertilization with Treated Pig Slurries and Composts. Soil Biol. Biochem. 38 (9), 2782–2793. doi:10.1016/j.soilbio.2006.04.040
Wagner-Riddle, C., Congreves, K. A., Abalos, D., Berg, A. A., Brown, S. E., Ambadan, J. T., et al. (2017). Globally Important Nitrous Oxide Emissions from Croplands Induced by Freeze-Thaw Cycles. Nat. Geosci. 10 (4), 279–283. doi:10.1038/ngeo2907
Wasserstein, R. L., Schirm, A. L., and Lazar, N. A. (2019). Moving to a World beyond "p. Am. Statistician 73 (Suppl. 1), 1–19. doi:10.1080/00031305.2019.1583913
Wrage-Mönnig, N., Horn, M. A., Well, R., Müller, C., Velthof, G., and Oenema, O. (2018). The Role of Nitrifier Denitrification in the Production of Nitrous Oxide Revisited. Soil Biol. Biochem. 123, A3–A16. doi:10.1016/j.soilbio.2018.03.020
Wu, H., Xu, X., Cheng, W., Fu, P., and Li, F. (2017). Antecedent Soil Moisture Prior to Freezing Can Affect Quantity, Composition and Stability of Soil Dissolved Organic Matter during Thaw. Sci. Rep. 7 (1), 1–12. doi:10.1038/s41598-017-06563-8
Wu, H., Xu, X., Fu, P., Cheng, W., and Fu, C. (2021). Responses of Soil WEOM Quantity and Quality to Freeze-Thaw and Litter Manipulation with Contrasting Soil Water Content: A Laboratory Experiment. Catena 198, 105058. doi:10.1016/j.catena.2020.105058
Yan, G., Xing, Y., Xu, L., Wang, J., Meng, W., Wang, Q., et al. (2016). Nitrogen Deposition May Enhance Soil Carbon Storage via Change of Soil Respiration Dynamic during a Spring Freeze-Thaw Cycle Period. Sci. Rep. 6 (1), 1–9. doi:10.1038/srep29134
Yoshida, H., Nielsen, M. P., Scheutz, C., Jensen, L. S., Christensen, T. H., Nielsen, S., et al. (2015). Effects of Sewage Sludge Stabilization on Fertilizer Value and Greenhouse Gas Emissions after Soil Application. Acta Agric. Scand. Sect. B - Soil & Plant Sci. 65 (6), 506–516. doi:10.1080/09064710.2015.1027730
Zhu-Barker, X., Doane, T. A., and Horwath, W. R. (2015). Role of Green Waste Compost in the Production of N2O from Agricultural Soils. Soil Biol. Biochem. 83, 57–65. doi:10.1016/j.soilbio.2015.01.008
Keywords: cold regions, organic amendment, nitrogen transformation, greenhouse gas emissions, biobased residues
Citation: Badewa EA, Yeung CC, Rezanezhad F, Whalen JK and Oelbermann M (2022) Spring Freeze–Thaw Stimulates Greenhouse Gas Emissions From Agricultural Soil. Front. Environ. Sci. 10:909683. doi: 10.3389/fenvs.2022.909683
Received: 31 March 2022; Accepted: 31 May 2022;
Published: 27 June 2022.
Edited by:
Jehangir Bhadha, University of Florida, United StatesReviewed by:
Haohao Wu, Nanjing Institute of Geography and Limnology (CAS), ChinaYuchuan Fan, University of Florida, United States
Copyright © 2022 Badewa, Yeung, Rezanezhad, Whalen and Oelbermann. This is an open-access article distributed under the terms of the Creative Commons Attribution License (CC BY). The use, distribution or reproduction in other forums is permitted, provided the original author(s) and the copyright owner(s) are credited and that the original publication in this journal is cited, in accordance with accepted academic practice. No use, distribution or reproduction is permitted which does not comply with these terms.
*Correspondence: Emmanuel A. Badewa, ZWJhZGV3YUB1d2F0ZXJsb28uY2E=; Maren Oelbermann, bW9lbGJlcm1hbm5AdXdhdGVybG9vLmNh