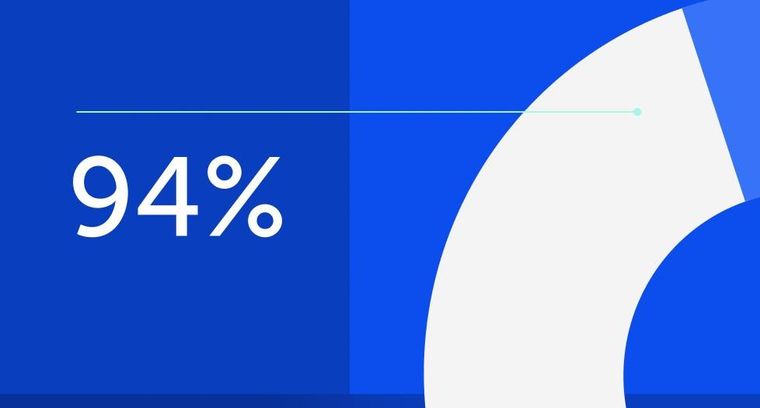
94% of researchers rate our articles as excellent or good
Learn more about the work of our research integrity team to safeguard the quality of each article we publish.
Find out more
REVIEW article
Front. Environ. Sci., 01 September 2022
Sec. Environmental Systems Engineering
Volume 10 - 2022 | https://doi.org/10.3389/fenvs.2022.907562
Landfill methane currently represents the largest global source of greenhouse gas emissions from the solid waste sector. Emissions are expected to increase due to increasing waste generation, particularly in countries still landfilling biodegradable wastes. As a complementary measure to gas extraction with subsequent flaring or energy conversion, or for emissions reduction from old landfills or from landfills containing wastes with a low gas potential, microbial methane oxidation systems (MMOS) are considered a promising technology. Numerous studies relating to controlling factors and enhancement of microbial methane oxidation in biocovers, biowindows or biofilters, both in laboratory and in large scale field settings, have been published. The design of optimized MMOS requires thorough understanding of the involved processes, specifically the biological ones and of those related to the transport of gas and water in porous media, and of the impact of material properties and external environmental factors on these processes. Consequently, the selection of materials that are suitable from a biogeochemical and from a geotechnical point of view, meeting the required water and gas transport properties, are key aspects in the design process. This paper reviews the scientific background of the relevant concepts and processes dictating MMOS performance, and provides guidance on layout and design steps, including choice of materials and quality control. Further, a decision tree to support the choice of MMOS is proposed. This paper provides the scientific foundation for upcoming technical guidance documents.
Anaerobic degradation of organic waste matter in landfills leads to the production of landfill gas, which contains mainly methane (CH4; around 60%) and carbon dioxide (CO2; around 40%). The global warming potential (GWP) of CH4 is 28 over a 100 years observation period; however, within a period of 20 years, the GWP of CH4 amounts to 84 (Aghdam et al., 2019). On a global perspective, CH4 emissions from landfills represent the largest direct source of GHG emissions from the solid waste sector. According to the fifth IPCC assessment report, landfill contribution to greenhouse gases amounts to approximately 630 Mt CO2eq (Fischedick et al., 2018) Global landfill emissions are expected to rise, since waste generation per capita is expected to increase, particularly in developing nations, who landfill large quantities of biodegradable organic matter (Heroux et al., 2010). Given the high short-term GWP, the nature of landfills as a point source and the magnitude of GHG emissions from landfills, an effective reduction of emissions will contribute significantly to reducing the source term in the greenhouse gas balance. Furthermore, prior to implementation of more recent stringent regulations, old (already closed) landfills in the past received great quantities of biodegradable organic waste. These old landfills can still release significant amounts of methane.
In the European Union (EU), where waste management is strictly regulated (Waste Framework Directive, http://data.europa.eu/eli/dir/2008/98/2018-07-05; Landfill Directive, http://data.europa.eu/eli/dir/1999/31/2018-07-04 and their respective implementation in the EU member states), there are diverse options for reducing CH4 emissions from landfills (Kjeldsen and Scheutz, 2018). These range from measures prior to landfilling (e.g., separate collection of biogenic waste for composting or for anaerobic digestion; or reduction of biodegradable organic content by mechanical-biological or thermal pre-treatment of wastes before landfilling) to procedures during or after landfilling of waste. These include landfill gas extraction, in-situ techniques to reduce the amount of already deposited biodegradable waste (e.g. in-situ aeration), and implementation of microbial methane oxidation systems (MMOS), which are the focus of this paper.
MMOS are considered key technologies for landfill emissions abatement and are especially applicable when gas extraction becomes technically or economically unfeasible but CH4 generation is still expected to continue for decades. MMOS have been listed in the 4th IPCC Assessment Report as promising mitigation strategies to reduce greenhouse gas emissions from the waste sector (Bogner et al., 2007). This recommendation was based on the work of international research groups that conducted detailed investigations on the enhancement of microbial methane oxidation in diverse lab- and field-scale settings on landfills (Streese and Stegman, 2003; Huber-Humer, 2004a; Barlaz et al., 2004; Gebert and Groengroeft, 2006a, b; Powelson et al., 2006; Haubrichs and Widmann, 2006). Since then, numerous investigations of the performance of - more or less engineered - biocovers, biowindows or biofilters have been carried out (Huber-Humer et al., 2008a, b; Abichou et al., 2009; Bogner et al., 2010; Bohn et al., 2011; Dever et al., 2011; Scheutz et al., 2011a; Roncato and Cabral, 2012; Scheutz et al., 2014; Roewer et al., 2016a, b; Cassini et al., 2017; Scheutz et al., 2017). These MMOS differ particularly with respect to geometric characteristics (e.g., volume and surface area available for the enhanced microbial oxidation process), CH4 supply (actively or passively vented), and type of selected substrate (e.g., sand and/or soil mixtures, waste-based materials like compost, wood chips). The terms “passive methane oxidation biosystems (PMOB)” or “biofiters,” “biowindows” and “biocovers” have also been adopted to refer to MMOS (e.g., Cabral et al., 2010b; Ahoughalandari et al., 2018).
A first review on microbial CH4 oxidation processes and technologies for landfill CH4 emission abatement was published by members of the international working group CLEAR (Consortium for Landfill Emissions Abatement Research, a task group of the International Waste Working Group, IWWG; Huber-Humer, 2004b) in 2009 (Scheutz et al., 2009a) presenting the state-of-the-science of the fundamental processes controlling CH4 oxidation in soils and landfill settings, and reporting the results of small/lab-scale experiments and the first engineered field systems for mitigating landfill CH4 emissions. Since then, further process knowledge has been generated and large-scale field trials and full-scale applications of MMOS have been implemented in several countries. A few countries have developed brief technical information (Campbell and Robinson, 2017) or guidelines for biocovers and biowindows (e.g. ÖVA, 2008; Kjeldsen and Scheutz, 2016; LAGA Ad hoc AG Deponietechnik, 2016a; LAGA Ad hoc AG Deponietechnik, 2016b, see Table 1 in Supplementary Material S1) and biofilters (NSW, 2019). One can find, for example, general recommendations on quality criteria to select suitable materials for the construction of methane oxidation layers. However, science-based guidance on design, construction and quality control of MMOS is still missing. Such guidance should take into account all physical and biological processes and their controls, including those related to the transport of gas and water in porous media, to the impact of material properties and to external environmental factors, for example, as determined by the local climatic conditions.
Generally, the most relevant environmental factors and conditions are interdependent; for example, moisture – a key factor dictating gas transport properties and thus the performance of MMOS – depends on prevailing climatic conditions, on the geometry of the MMOS, and on the texture and compaction of the material constituting the methane oxidation layer (MOL). The porosity of the MOL material, its water holding capacity after placement, and its gas permeability have a decisive effect on the performance of the MMOS. Therefore, selection of suitable materials and how they are compacted represent key design aspects (Gebert et al., 2011a; Ahoughalandari et al., 2018; Van Verseveld and Gebert, 2020). Another challenging design concern is ensuring uniform spatial distribution of the CH4 load to the biosystem. Spatial inhomogeneity of landfill gas can be caused by unequal distribution at the base of the MMOS (potentially associated with moisture blocking upward flow of landfill gas), by the formation of secondary macropores (cracks, fissures, animal burrows) within the MMOS, or from spatially uneven compaction or moisture content of the MMOS material. Along these preferential pathways, the CH4 load likely exceeds the oxidation capacity of the medium; and excessive advective methane bottom flux through these pathways hinders the diffusive influx of oxygen from the surface (Gebert et al., 2011b). This results in “hotspots,” i.e. areas of high CH4 fluxes or uncontrolled emissions, at the surface of the landfill cover. Ensuring a more even distribution of the CH4 loading greatly improves emissions abatement, with CH4 oxidation rates attaining nearly 100% of the applied loading (Huber-Humer, 2004a; Bogner et al., 2007; Roncato and Cabral, 2012; Ndanga et al., 2015; Ndanga et al., 2016).
The fundamental factors impacting the microbial process of CH4 oxidation in landfill settings have been covered in various publications, e.g., by Huber-Humer et al. (2008b), Scheutz et al. (2009a), Spokas and Bogner (2011) and will not be presented herein. The scope of this paper is to review technology relevant processes and to reflect the current knowledge regarding technical design of microbial methane oxidation systems, acting as basis for future science-based, technical guidance documents on MMOS design. Moreover, this paper provides guidance on when to implement MMOS, and which system would be the most appropriate under specific condition and proposes possible approaches for the monitoring and performance assessment of MMOS (Supplementary Material S3), including a proposal for an action plan that addresses unwanted developments of MMOS. Further, recommendations on methods for the quality control of candidate materials are given (Supplementary Material S4). This paper, therefore, provides a comprehensive state-of-knowledge basis for further research regarding optimization of MMOS as well as guidance to landfill operators, MMOS designers and permitting authorities, supporting choice of the adequate emission abatement strategy to curb fugitive emissions during the operational years of a landfill, and for residual emissions during the after-care period.
The main characteristics and functions of the three to four layers typically making up a methane oxidation system are as follows (and summarized in Tables 1, 2):
(1) Foundation layer (MMOS bed)
When building a MMOS below-grade (biowindows and some types of biofilters), i.e. within the existing landfill top cover, the foundation layer will probably be the existing cover soil, or any soil layer that was placed immediately upon the waste mass. When building an above-grade MMOS (biofilters and biocovers) (Table 2), the foundation layer is the base of the structure supporting the MOL, which needs to be impervious to gas migration. On landfills where no cover was placed, a layer of inert wastes can be compacted, profiled and levelled and therefore serve as a foundation for a MMOS.
(2) Gas distribution layer (GDL)
The fundamental functions of the gas distribution layer are the following: distribute the CH4 loading (or landfill gas loading) as uniformly as possible to the base of the overlying MOL; and drain away water that seeped through the MMOS. Uniform distribution of CH4 loading is a critical design issue. It ensures that the system is not overloaded, thereby preventing the creation of hotspots. In below-grade MMOS, the GDL intercepts landfill gas fluxes from the upper boundary of the waste body or introduced through a dedicated gas supply pipe, whereas in above-grade MMOS the CH4 loading is introduced into the GDL through dedicated gas supply pipes.
The materials constituting the GDL must not be prone to cracking due to differential settlement to prevent upward gas flow in spatially concentrated zones. Moreover, as will be discussed in detail later, the gas conductivity (kGas) and hydraulic conductivity (k) of the GDL material must be adequate to maximize horizontal flow of gas in the gas distribution layer (therefore ensuring uniform distribution at the base of the MOL) and minimize the effect of capillary barrier that naturally occurs at the interface of materials with contrasting hydraulic properties. Optimization of spatial gas distribution means herein that kGas of GDL material must be significantly higher than that of the overlying MOL material. The required difference depends on the landfill gas pressure and the number of either designed and constructed or naturally present gas inlets into the GDL (see also Pairing of GDL and MOL materials regarding spatial homogenization of CH4 load).
(3) Filter layer (FL)
Since the GDL is typically constructed with coarse-grained material, whereas the MOL may be constituted of relatively finer-textured material, it may be necessary to include a filter layer between them. One of the filter’s main functions is preventing particle migration from the MOL material into the GDL, which might otherwise cause mechanical clogging at the interface between the MOL and GDL, redirecting flow elsewhere and possibly leading to hotspots. Particles sizes of GDL, FL and MOL need to observe Terzaghi’s filter rules to effectively prevent particle migration. The second relevant function of the filter layer is to reduce the hydraulic contrast between GDL and MOL. This contrast leads to the formation of a capillary barrier, i.e. a zone where the degree of water saturation in the MOL is high enough to cause occlusion of the pores by water at its lower boundary (Cassini et al., 2017; Ahoughalandari et al., 2018), again blocking gas flow and eventually inducing preferential flow. Reduced contrast as well as a sloped interface reduce the negative impact that the capillary barrier effect may have on gas migration. The necessity for a filter layer depends on the contrast between the unsaturated hydraulic conductivity of the gas distribution and the methane oxidation layer. Examples of MMOS in which a filter layer was implemented are given by Gebert et al. (2003), two biowindows (1,400 and 2,100 m2; Gebert et al., 2015; Gebert et al., 2016; Gebert et al., 2017), three passive MMOS constructed at the St-Nicéphore landfill experimental plot (Cabral et al., 2010b; Capanema and Cabral, 2012; Roncato and Cabral, 2012) and a recent above-grade MMOS constructed over the old Kitchener landfill, in Kitchener, Ontario, Canada (Nelson et al., 2021); however, there are also successful examples that did not require the construction of a FL, such as the MMOS using well-structured compost as substrate for MOL reported by Huber-Humer (2004a), Scheutz et al. (2011c), Cassini et al. (2017), and Huber-Humer et al. (2017).
(4) Methane Oxidation Layer (MOL)
The MOL must be protected from adverse conditions that may reduce its ability to oxidize CH4, such as excessive heat or cold, excessive moisture or lack thereof, and rapid changes in any of the preceding. In the case of mineral soils, the MOL can be constructed in two sublayers: the vegetated top part (usually composed of humic topsoil, constituting the main rooting zone) and the subsoil. Examples of an topsoil-subsoil layering are given in Gebert et al. (2003), biofilter; Huber-Humer et al. (2009), biocover test cell; Bour et al. (2015), laboratory column study; Gebert et al. (2015), biowindow; Röwer et al. (2016a), biocover and Ndanga et al. (2015); laboratory and field tests of several biofilters. The CH4 oxidation front (depth) may vary over time, depending on the depth of ingress of atmospheric air, the composition and height of the accruing landfill gas, and the environmental conditions that drive the microbial process (e.g., temperature, water tension).
The key services of and requirements on the MOL in biofilters, biowindows or biocovers are the following (relevant phenomena are explained in Choice of Materials):
(1) Permit advective and diffusive gas transport up (CH4 and CO2) and down (O2) to maintain the CH4 oxidation capacity, so that CH4 surface emissions are brought down to the desired levels.
(2) Provide enough water retention to sustain activity of microorganisms and plants.
(3) Offer a suitable physical environment for the attachment of methanotrophic and cohabitating bacteria.
(4) Offer a sufficient insulation effect to maintain temperature within suitable range for methanotrophic activity.
(5) Offer a suitable geochemical environment for methanotrophic activity.
(6) Supply nutrients to sustain activity of microorganisms and plants.
One of the main challenges, insofar as design is concerned, is to balance the requirements on gas diffusivity (1) and water retention (2), as they pose potentially conflicting demands on the material’s pore space. Table 1 summarises the five key services, their associated aims, the parameters by which each service can be assessed, and the soil property associated with or affecting these parameters.
Depending on the operational status of a landfill (open, closed, after-care), the magnitude of the production of landfill gas, whether the existing cover is final or interim, and the presence of other relevant infrastructure such as surface sealing (e.g., clay liner, geomembrane or geosynthetics clay liners) and gas extraction system, different microbial methane oxidation systems are applicable. The three most common types of MMOS are methane oxidation filters (also called “biofilters”), methane oxidation windows (also called “biowindows”) and methane oxidation covers (also called “biocovers”). A summary of these system’s applications and operational conditions are shown in Table 2, followed by details pertaining to each. Further information can be found in Huber-Humer et al. (2008b) and Kjeldsen and Scheutz (2018).
Biofilters are self-contained fixed-bed reactors in which landfill CH4 is oxidized by methanotrophic bacteria colonizing the filter material. They can be operated in combination with conventional surface sealing systems and require an active or passive gas supply system through which the landfill gas is routed to the filter. The gas supply or gas extraction system can also be used to quantify the expected (before dimensioning) and operational loads, and hence aid appropriate dimensioning of the biofilter and CH4 load monitoring. Table 2 illustrates two open systems configurations: one below-grade and one above-grade. Biofilters can be operated either as open (Gebert et al., 2003; Gebert et al., 2004; Powelson et al., 2006; Cabral et al., 2010b; Roncato and Cabral, 2012) or closed (Streese and Stegmann, 2003; Melse and Van der Werf, 2005; Haubrichs and Widmann, 2006) reactors. In the former, the ingress of atmospheric oxygen occurs by diffusion from the atmosphere. In closed reactors, oxygen must be either admixed prior to loading the gas mixture to the filter (Streese and Stegmann, 2003; Stegmann et al., 2006) or supplied through aeration of the filter bed (Haubrichs and Widmann, 2006), unless the loaded gas mixture already contains enough oxygen. This occurs when treating landfill gas from a landfill under in situ aeration (Meza et al., 2021).
Open filter systems are particularly suited for passive operation, where gas flow through the filter follows the pressure gradient between the waste mass and the atmosphere, i.e. the gas is not actively extracted and pumped into the MMOS. Operational conditions (e.g. temperature, humidity) of such open systems are controlled by the local weather and are thus subject to seasonal variability. Operational scenarios should be reproduced – numerically or otherwise – during the design process. For example, when using unsaturated flow modelling to observe the hydraulic behaviour of several MMOS scenarios, one can adopt different weather conditions, hydraulic parameters varying with time (to account for settlement, for example), etc. In contrast, in the encased filter systems, temperature, pressure and humidity can be controlled to maximize CH4 oxidation. Consequently, open filters influenced by ambient conditions and encased filters operated under controlled conditions require different dimensions and different factors of safety to treat a given CH4 load. They also comprise different capital and operational costs. In open biofilters, the vegetation layer provides protection against erosion through wind and precipitation, and buffers temperature extremes. It may also enhance aeration through the formation of secondary macropores by the root system. Further examples of design, construction and operation of methane oxidation filters can be found in Zeiss (2006), Dever et al. (2011) and Parker et al. (2013).
Biowindows are open compartments integrated into the (often fine-grained, cohesive) landfill top cover that do not require connection to a gas extraction system. They are either planned from the outset or inserted later to remediate a hotspot which developed, for example, due to preferential pathways in the cover soil such as cracks caused by settlement or desiccation, or by differences in degree of compaction of the cover soil, by cracks in the transition zone between waste and cover soil (Table 2), or by an elevated gas flow within the waste body at the respective location. If planned, the landfill gas flows to the engineered window due to its higher permeability compared to that of the surrounding landfill cover. As with open-bed biofilters, atmospheric oxygen is supplied by diffusion via the window surface. Biowindows are particularly suited for old landfills lacking gas extraction systems and already covered with soil. If a geosynthetic liner is present in the final cover system, existing gas wells can be used to route the landfill gas through the liner into the window. Possibly, further perforations in the liner are needed to feed the necessary number of windows. Biowindows can replace gas wells if the active gas extraction system on closed landfills is to be turned off. While biofilters can be operated based on known CH4 loads (measured in the gas supply pipe), the CH4 load reaching a biowindow needs to be estimated. For the remediation of hotspots, emission measurements in combination with an estimate of the oxidized share (for example using the carbon balance method; Christophersen et al., 2001; Gebert et al., 2011c; Geck et al., 2016a, Geck et al., 2016b) is the appropriate method of determining the CH4 load.
Examples of construction and operational details of methane oxidation windows can be found in Jugnia et al. (2008), Philopoulos et al. (2008), Ait-Benichou et al. (2009), Bogner et al. (2010), Scheutz et al., 2011a), Röwer I. U. et al., 2011), Pecorini et al. (2013), Röwer (2014) and Huber-Humer et al. (2017). The remediation of hotspots using biowindows was investigated by Röwer et al. (2011b).
Biocovers, schematically illustrated in Table 2, are designed to oxidize landfill CH4 over large tracts of the interim or final cover. They reduce residual emissions after closure of the gas extraction system while also serving the general functions of the final soil cover and can be planned and designed as part of the regular landfill design. Biocovers can also be considered when many biofilters or biowindows are required to treat the CH4 load. Further cases of potential retro-fitted biocovers include old landfills whose final covers do not prevent the occurrence of high surface CH4 concentrations. In biocovers, landfill gas migrates into the gas distribution layer either directly from the underlying waste or from the waste through the (partly excavated) former cover (Table 2). If an impermeable liner or existing layer of compacted soil caps the waste mass gas supply pipes must be installed to feed the gas into the GDL. For landfills with an active gas extraction system, it is a feasible option to connect the existing gas drainage (collection) system with its piping infrastructure to the biocover’s GDL. Uniformity of spatial distribution needs to be achieved on a known projectable scale.
In contrast to biofilters and biowindows, the great advantage of biocovers lies in the large area available for biological gas treatment, and hence the lower spatial CH4 load compared to biofilters and biowindows. However, a major challenge with biocovers is ensuring spatially uniform distribution of the CH4 load, especially when the locations where landfill gas escapes from the waste into the gas distribution layer and their respective contribution to the total flow are unknown. The latter will be the case if the cover is placed directly on top of the waste mass. Also, from the perspective of moisture distribution, there are considerable difficulties in attaining uniform loading over great lengths due to potential accumulation of moisture at the interface between the GDL and the MOL, particularly in downslope areas. This can be addressed by insertion of drainage spots at certain intervals along the interface to evacuate accumulated moisture, as has been applied for engineered capillary barriers (e.g. von Der Hude et al., 1999) or by a sloped interface between these layers. Alternatively, choice of substrate and construction practice must include higher methane loadings, therefore higher oxidation capacity in the upslope area.
In general, landfill covers must fulfil diverse tasks: they must serve as recultivation layer, as a water balance layer, and must have suitable properties for whatever after-use is envisaged. Biocovers hence may have to meet the demands on water balance, gas balance, and possibly on geotechnical requirements for the physical support of the specific after-use. Biocover materials must possess enough water retention capacity and high enough gas conductivity (kGas) and gas diffusivity (Deff); such a combination significantly narrows down the spectrum of suitable soil or substrate textures. Moreover, for the construction of an entire landfill cover, large amounts of suitable materials are required, which can become a considerable cost factor or even a limiting issue concerning availability.
Further examples of design, construction and operation of biocovers or corresponding test cells can be found in Barlaz et al. (2004), Huber-Humer (2004a), Huber-Humer et al. (2008b), Einola et al. (2009), Geck et al. (2016a), Röwer et al. (2016a, b), and Scheutz et al. (2017).
The transport of gas and water through the layered system of the MMOS dictates its capacity to abate CH4 emissions and hence deserves attention during the design phase. Soil texture and compaction level govern a soil’s pore size distribution and thereby its water retention characteristics and associated diffusive and advective gas transport capacity (Arya and Paris, 1981; Aberg, 1992, 1996; Arya et al., 1999; Poulsen and Blendstrup, 2008; Gebhard et al., 2009; Gebert et al., 2011a; Smith et al., 2018; Van Verseveld and Gebert, 2020). Its heat transfer characteristics also influence diffusive flow.
The following aspects are fundamental in MMOS design: the water retention curve (WRC; relationship between soil moisture and soil suction, also yielding a soil’s pore-size distribution), the hydraulic conductivity function (kfct; permeability to water as a function of soil suction), and the air/gas permeability function (kG-fct; the relationship between the soil’s gas permeability and the volumetric air porosity or air-filled porosity) of the MOL. This section briefly reviews the corresponding fundamental processes and mechanisms that form the basis for proper design. The associated steps are presented in detail in Design Steps Based on Hydraulic Considerations.
The water retention curve (WRC) and hydraulic permeability function (k-fct) are fundamental in any problem related to unsaturated flow of water, which directly affects transport of gas. In MMOS design, they are applied to 1) optimise for the MOL base area receiving the gas load, using the air-filled porosity at the point of occlusion of pores with water (θa_occ) and the resulting length of unrestricted gas migration (LUGM) on a slope (Design Steps Based on Hydraulic Considerations), and the difference in gas conductivity between methane oxidation and gas distribution layer (Pairing of GDL and MOL materials regarding spatial homogenization of CH4 load), and to 2) optimise for the ingress of atmospheric oxygen into the methane oxidation layer, using the soil’s effective diffusivity (Diffusive Gas Transport).
The WRC (Figure 1) is the constitutive relationship between moisture in the soil (be it the gravimetric water content, w, the volumetric water content, θw, or the degree of saturation, Sr), and the negative water pressure (soil suction, ψ) caused by capillarity (Barbour, 1998). Its main features are the suction at air entry value (ψAEV), or the suction value at which air starts to enter the largest pores, thereby causing a decrease in moisture with increasing suction; the slope of the desaturation curve (n); the residual water content (θw_res) and the suction at which this residual water content is reached, i.e. the water entry value (ψWEV) (Morel-Seytoux, 1993). Certain soils may present a hysteretic behaviour, as shown in Figure 1. The curve to be adopted in a conservative MMOS design is the one that gives the highest water content for the same level of suction (for the selected soil), thereby forcing the designer to work with the worst-case scenario of occlusion of the pores by water. Accordingly, the drying curve, which is also the easiest to obtain experimentally, is to be selected.
FIGURE 1. Water retention curves of simulated laboratory experiments on two materials forming a capillary barrier: sand (the moisture retaining layer; or MRL) and gravel (the capillary break layer; or CBL). The wetting path is a schematic representation, i.e. not derived from actual experiments.
Due to the direct connection between pore radius and matric potential (or suction; Young-Laplace equation), the WRC also yields the pore size distribution of a soil. The sum of all pores with a diameter of >50 μm, corresponding to a matric suction of <6 kPa, are too large to exert the capillary forces required to hold water against gravity and are hence available to gas transport. This share is referred to as air capacity (AC), the remaining share of pores is referred to as field capacity (FC), corresponding to the maximum volume of water a soil can hold against gravity (FAO, 1985; Blume et al., 2016; Table 3). In Figure 1, air capacity and field capacity amount to 32 vol% and 10 vol%, respectively. Air filled porosity and distribution of this porosity over different pore sizes determine a material’s gas transport properties (effective diffusivity, conductivity, see Gas transport). To derive the effective diffusivity required to warrant the influx of atmospheric O2 that suffices to oxidise a given CH4 load, conservative MMOS design should also determine these gas transport properties for an air-filled porosity corresponding to the water content at field capacity, given for different soils at different bulk densities in Supplementary Material S2. Under field conditions dryer than field capacity, a larger volume of pores than corresponding to air capacity are available for gas transport.
In MMOS design, the WRC of the MOL and filter materials are ideally determined experimentally using one of a variety of methods presented in the literature (e.g. Klute, 1986; Fredlund et al., 2002; Vanapalli et al., 2008; Orlando et al., 2014). If it is not possible to determine it directly, pedotransfer functions, such as proposed by Arya and Paris (1981) can be used to approximate a material’s WRC. With a set of experimental data (or data from a pedotransfer function), such as the simulated laboratory results shown in Figure 1, one can fit the WRC curve by applying one of several simplified method that use shape-regression equations, such as the empirical models proposed by van Genuchten (1980), and Fredlund and Xing (1994). Leong and Rahardjo (1997) and Simms and Yanful (2002) also present several equations to model the WRC. The parameters describing the WRC (such as the van Genuchten parameters) are key input data in numerical modelling (see step 3 in the section Design Steps Based on Hydraulic Considerations).
The hydraulic permeability function (k-fct, Figure 2) is the relationship between the hydraulic permeability of the soil and soil suction. Its main features are the saturated permeability, ksat (i.e. the value of k when suction is equal to zero), the slope of the desaturation curve, and the air entry value (ψAEV). The value of the ψAEV is the same as the one obtained from the WRC. The k-fct, a fundamental tool in MMOS design, is one of the main input data in numerical modelling of unsaturated flow of water. Modelling allows determination of the point along the interface between MOL and the filter layer (part of the drainage system) where air becomes occluded. This is explained in detail in Design Steps Based on Hydraulic Considerations.
FIGURE 2. Hydraulic permeability function (k-fct) of the same soil in Figure 1.
The k-fct can be determined experimentally Abu-Hejleh et al. (1993), Wildenschild et al. (1997), McCartney and Zornberg (2010a), but this is rather difficult. Consequently, the most common method is to derive the k-fct from the WRC. The Mualem-van Genuchten model (van Genuchten, 1980; Schaap and Leij, 2000) is often adopted for this purpose. Pedotransfer functions also have been incorporated into some mainstream computer codes, such as Hydrus (Simunek and Van Genuchten, 2012). As explained in detail in step 3 (Design Steps Based on Hydraulic Considerations), several runs of a model should be performed to test all candidate materials.
The contrasting textures between soils and, therefore, the resulting contrast in unsaturated hydraulic conductivity, may lead to accumulation of moisture in the zone just above their interface (finer material on top of coarser material), and may impede gas transport across this interface. This phenomenon is known as the capillary barrier effect. The extent of moisture accumulation depends on material characteristics, on climate and on physical characteristics of the cover, such as slope angle and length. Capillary barriers can be purposely engineered to reduce infiltration into the waste body by diverting the water downslope within the finer material (capillary layer) just above the interface to the coarser material (capillary block layer) (e.g. Melchior et al., 1990; Kaempf and Montenegro, 1997; Parent and Cabral, 2005, 2006; Abdolahzadeh et al., 2011a, b; Williams et al., 2011).
In MMOS constructed with the general layering sequence described in Typical Layouts of Methane Oxidation Systems, the contrast between hydraulic characteristics of the materials naturally leads to the formation of capillary barriers. Due to downslope movement of water, when moisture levels become high enough right above the MOL-GDL (or MOL-filter) interface, the pores in the soil may become occluded with water, thereby creating obstruction to gas flow. Accordingly, one of the main challenges in MMOS design is related to the potentially conflicting water and gas transport processes.
When pores are occluded (blocked) by water, permeability to gas becomes lower downslope than upslope (see Advective Gas Transport: The Gas Conductivity or Gas Permeability Function (kG-fct)), leading to gas migration upward within the GDL, therefore reaching the base of the MOL in areas where pores are not blocked. Such zones with lower moisture content are located near the top of the sloping MMOS, as observed by Berger et al. (2005) for an experimental laboratory capillary barrier system. In an experimental field biocover system, Geck et al. (2016a, b) concluded that preferential upward migration caused higher upslope CH4 concentrations within the soil and higher surface emissions. For this same field biocover, Röwer et al. (2016b) assessed the difference in gas diffusivity (Deff, see Diffusive Gas Transport) and gas conductivity (kGas, see Advective Gas Transport: The Gas Conductivity or Gas Permeability Function (kG-fct) between downslope and upslope areas based measurements of volumetric water content measured in 80 cm depth, i.e. near the GDL-MOL interface. The authors demonstrated the variability of this difference over a period of 16 months and inferred the corresponding shifts in the balance between diffusive and advective landfill gas flow. Cabral et al. (2010a) observed in a field experiment the same behaviour as Geck et al. (2016a), particularly during wet periods. Ahoughalandari and Cabral (2017a) assesed the response of several configurations using numerical modelling, by focusing on the location of the occlusion point along the interface. The section Design of Microbial Methane Oxidation Systems gives suggestions as how to minimize this phenomenon in the design process.
The soil’s or material’s gas transport properties determine the flow of CH4 and O2 through the MOL and thereby govern system performance. Consideration of gas transport properties and their impacting parameters is therefore highly relevant in the design of methane oxidation systems. Gas transport in methane oxidation systems can be both diffusive and advective: concentration gradients inducing diffusive transport drive the flow of CH4 from the landfill or from the gas supply pipe to the atmosphere and the inversely oriented flow of oxygen from the atmosphere into the methane oxidation system. The pressure gradient between the landfill or between the outlet of the gas supply pipe and the atmosphere induces advective transport of landfill gas through the methane oxidation system. Additional advective flow of atmospheric air or landfill gas through the methane oxidation system can be driven by wind or changes in barometric pressure, just like through any other part of the landfill. Further, the methane oxidation process also induces negative pressures, resulting from the consumption of 3 mol of gas (1 mol CH4, 2 mol O2), while only 1 mol of gas (CO2) is produced, which induces advective transport of both landfill gas and atmospheric oxygen (Huber-Humer, 2005).
Gas transport rates are governed by the interconnected volume and the geometry (distribution of pore radii, tortuosity) of the water-free pore space. This pore space is also referred to as air-filled porosity (εa), or volumetric air content (θa). Air-filled porosity equals total porosity (n) minus the volumetric water content (θw; θa = n-θw), and, if connected, represents the share of total porosity that is available for gas transport (Tuli et al., 2005; Gebert et al., 2011b; Martínez et al., 2016). Total porosity and geometric properties are determined by the soil’s particle size distribution and compaction, and define the soil’s permeability (unit: m2), which is an intrinsic property that is independent of soil moisture or the through-flowing medium. In the case of diffusive gas transport, considering soil moisture and the viscosity of the migrating gas leads to the property of effective gas diffusivity (unit: m2/s), often simply termed diffusivity. With advective gas transport, consideration of moisture gives the soil’s effective gas permeability (unit: m2). If also the kinematic viscosity of the gas is considered, there is spoken of gas conductivity, expressed by the coefficient of gas conductivity (kgas, unit: m/s). This coefficient expresses the resistance of the medium to the movement of the gas in question when it is submitted to a pressure gradient. This is the typical situation in MMOS, where landfill gas is either actively fed to the GDL or enters it passively following the pressure gradient between the waste body and the atmosphere. In the geotechnical field, and also in this paper, the term gas permeability is often used synonymously with the term gas conductivity.
MMOS design (including choice of soils) and dimensioning should also consider the possible effect of processes reducing the air-filled porosity and hence diffusivity and gas conductivity. These include changes in soil moisture due to precipitation and evapotranspiration and further settlement, caused, for instance, by self-weight consolidation or compaction during construction. Additional long-term post-construction processes that change pore architecture include physical (aggregation/segregation) and biological (e.g. burrowing fauna) soil structure forming processes. These processes should be considered in the design process and monitored during MMOS operation.
The gas conductivity function relates the air-filled porosity (θa) with the coefficient of gas conductivity (or coefficient of gas permeability), kGas. The kG-fct can be obtained in the laboratory following any of a variety of procedures published in the technical literature (e.g. Iversen et al., 2001; Samingan et al., 2003; Marinho and Teixeira 2013; Ahoughalandari et al., 2018). In short, the soil’s volumetric air content θa is progressively reduced (either by increasing soil moisture or soil compaction) and the corresponding change in gas conductivity (coefficient kGas) is measured. In the field, at the soil’s given bulk density, the value of θa decreases if moisture is added (e.g. infiltration of water) and increases following evapotranspiration. The best practise is to define what is needed to obtain in terms of pore interconnection (effective porosity) and specify compaction accordingly. This is described in Design Steps Based on Hydraulic Considerations. General requirements on gas permeability/conductivity are formulated in Materials for the Methane Oxidation Layer (MOL).
Figure 3 presents two types of behaviours one can expect to find when determining the kG-fct by varying soil moisture. In the field this would correspond to periods of increased precipitation and/or lowered evapotranspiration. On the left (fine sand), there is a single sharp drop in kGas when the interconnected pores most relevant to advective gas transport become blocked by water, causing discontinuity of the air-filled pore space. The associated value of volumetric air content is denominated θa_occ. In the other case (compost with sand admixture, Figure 3, right), two drops in kGas were identified upon addition of water, pointing at a bimodal distribution of large-diameter pores. Usually these pores retain much less water by capillary forces; as a result, water is drained out, therefore causing a recovery of gas conductivity.
FIGURE 3. Gas permeability function with volumetric air content (or air-filled porosity εa) adjusted by soil moisture: single drop (fine sand, left) and dual-drop (compost-sand mixture, right). Fluid = air. Adapted from Ahoughalandari et al. (2018).
As suggested by Ahoughalandari et al. (2018), a conservative approach with MOL materials presenting the behaviour shown on the right of Figure 3 is to consider occlusion when the first drop is obtained. This point was termed conservative volumetric air content, or θa_Cons_occ. Figure 3 shows that, for the materials tested, density (corresponding to the following degrees of compaction: 100, 94 and 89%) did not significantly affect the value of θa_occ (or θa_Cons_occ), while it had a minor effect on the kG_fct.
For MMOS design, the relevant parameter is the volumetric air content at occlusion, θa_occ (or θa_Cons_occ) (Design Steps Based on Hydraulic Considerations). Ahoughalandari et al. (2018) presented the various steps to obtain the volumetric air content at occlusion (θa_occ) using the air (gas) permeability function (ka-function) of the MOL material. They also presented a more expeditious method to estimate θa_occ in the absence of the ka-function; in this case, the Standard Proctor curve of the MOL candidate material can be used. In certain types of soils, such as sandy soils, θa_occ correspond approximately to the soil’s air capacity, i.e. the share of air-filled pores at a matric potential of −6 kPa.
The gas permeability function is controlled by changes in soil moisture and the value of θa_occ depends on the soil’s particle size distribution and the degree of compaction (or relative density), which, combined determine the distribution of pore radii in the soil. The addition of water to dry soil usually leads to soil pores being filled in the order of decreasing capillary suction (lower matric potential), i.e. finer pores are saturated first and coarser pores later. As far as gas transport through soils is concerned, larger diameter pores can provide unrestricted flow paths as long as their number and interconnectivity are sufficient. Consequently, in more coarsely textured substrates the susceptibility to moisture content variation can even be lower than for the fine sand shown in Figure 3. This can be observed in Figure 4, which shows how kGas for four sandy MOL materials compacted to the same relative density (properties presented in Gebert et al., 2019) evolved with varying air-filled porosity. Addition of water hardly affected the value of the coefficient of gas conductivity, meaning that these soils maintained a high share of well-drained interconnected pores. For soil 1, even an increase in kGas with increasing moisture could be observed, which was associated with structure forming processes that increased the share of large diameter pores (Van Verseveld and Gebert, 2020). In this experiment, only for soil two the critical threshold of θa_occ was reached by the levels of moisture tested.
FIGURE 4. Gas permeability function (fluid = air) with air-filled porosity (εa) adjusted by soil moisture in different sandy candidate MOL soils. Adapted from Gebert et al. (2019). θa_occ not reached for soils 1, 4 and 5.
Soil compaction first reduces the large-diameter pores. With increasing compaction energy smaller pore diameters are also affected (Møldrup et al., 2001; Richard et al., 2001; Gebhardt et al., 2009; Gebert et al., 2011a; Wickramarachchi et al., 2011; van Verseveld and Gebert, 2020). Since the pore radius affects advective flow to the power of four (Hagen-Poiseuille’s law), the gas conductivity (coefficient kGas) is strongly impacted by a decrease in large diameter pores due to compaction, for example during MMOS construction. Poulsen and Blendstrup (2008) obtained a log-linear decrease of gas conductivity with increasing compaction effort, which was also found by Gebert et al. (2019) for seven different candidate MOL soils (Figure 5). The latter was also observed that the change in gas conductivity with changing air-filled porosity varied significantly for the different soils, presumably due to the differing share of large-diameter pores.
FIGURE 5. Gas permeability function (fluid = air) with air-filled porosity (εa) adjusted by degree of soil compaction in different sandy candidate MOL soils. Note logarithmic scale on y-axis. From Gebert et al. (2019).
Due to the importance of kGas for the spatial distribution of the landfill gas in the GDL underneath the MOL, the degree of MOL compaction has to be considered in system design (see Materials for the methane oxidation layer (MOL), Pairing of GDL and MOL materials regarding spatial homogenization of CH4 load). Empirical data of air capacity (air-filled porosity at maximum water retention, corresponding to a suction of −6 kPa) for different soil textures and bulk densities for mineral soil substrates are given in Supplementary Material S2.
In contrast to gas conductivity, the absolute share of water-free pores is relevant for soil gas diffusivity, irrespective of how this share is distributed over the various pore radii. This assumes that Knudsen diffusion, which is governed by collisions between gas molecules and pore walls dominating over intermolecular collisions (Warrick, 2002), is irrelevant for the coarsely textured soils used in MMOS. Knudsen diffusion only becomes dominant for pore diameters below 0.1 μm (De Visscher and Van Cleemput, 2003a) which are fine pores mainly associated with clay and filled with water.
In line with what was argued above it has been observed that gas diffusivity declines almost linearly with decreasing air-filled porosity (θa), as also described by Penman (1940), and is strongly affected by the level of soil moisture in the five investigated sandy soils (Figure 6, left). All five soils fit a similar near-linear relationship, only with values of θa below 10–15% diffusivity levels off slightly. Seasonal changes in soil moisture, diminishing θa, therefore greatly affect the diffusive ingress of atmospheric oxygen and hence the CH4 oxidation capacity. Because in the investigated soils diffusivity was insensitive to the pore diameter and hence Knudsen diffusion was not relevant, the effect of diminishing θa by increasing soil moisture was within the same range as when air-filled porosity was reduced by increasing compaction levels (Figure 6, right).
FIGURE 6. Effective gas diffusivity (Deff) in relation to air-filled porosity (εa), adjusted by soil moisture (left) and by degree of compaction (right) in different sandy candidate MOL soils. Fluid = artificial landfill gas mixture. Adapted from Gebert et al. (2019).
Various authors have observed a non-linear decline of diffusivity with decreasing air-filled porosity (Richter et al., 1991; Møldrup et al., 2000; Allaire et al., 2008; Gebert et al., 2011a), meaning a less than proportional diffusivity at lower shares of air-filled porosity (θa). Examples of models describing this behaviour include those by Buckingham (1904), Millington and Quirk (1961), Troeh et al. (1982) and Møldrup et al. (2005), see also a review by Werner et al. (2004). The effect can be explained by increased tortuosity and reduced connectivity of the remaining air-filled pores at high moisture contents or high levels of compaction and is more likely to be relevant in soils with a relevant share of fine grains and therefore smaller average pore radii. In coarse-grained, sandy soils such as the ones depicted in Figure 6, pore connectivity and tortuosity appear to limit diffusivity to a lesser extent at low values of θa.
In contrast to gas conductivity (Figure 5), effective diffusivity declined (only) linearly with the compaction-induced decrease in air-filled porosity (Figure 6, right). This indicates that conductivity and, hence, advective gas transport is affected more significantly by compaction-related loss of large diameter pores than diffusive gas transport. Also, the slope of Deff over air filled porosity εa (specific diffusivity, Dspec = Deff/εa), a measure of the efficiency of the pore system to transport the gas, was much more similar for the different soils than the changes in specific conductivity (kspec = kGas/εa) with changing air-filled porosity. This indicates that the pore size distribution, specific for each individual soil, plays a greater role for advective than for diffusive transport.
The requirements on the soil’s diffusivity in relation to the design CH4 load are described in Materials for the Methane Oxidation Layer (MOL). For mineral soils, generic relationships between particle size distribution, compaction (bulk density) and air-filled porosity at maximum water retention can be derived from Supplementary Material S4 and used to infer the soil’s effective diffusion coefficient. For composts, they have to be determined case-specifically.
The following steps, presented in chronological order, are suggested for an optimized design, construction and operation of a methane oxidation system.
The targets for implementing a methane oxidation system to treat residual emissions can be many-fold. In most cases they also depend on specific local aspects like the proximity of nearby housing, the intended post-closure after-use of the landfill, whether the site will be accessible to the public or not, or the cost-effectiveness of the measure. Examples of possible targets are the reduction of climate-forcing emissions and/or warranting safe on-site conditions by minimising the risk of explosive CH4 concentrations at the landfill surface. Sometimes the target is set to just improve the current situation with no further specific requirement on system performance. These general targets can then be differentiated based on, for example, acceptable emissions and surface concentrations, spatial targets (e.g. certain parts of the landfill must be emission-free), oxidation targets (e.g. an average reduction or a certain percentile of the load must be abated), and temporal targets (e.g. oxidation target always met, including stress states such as low temperatures or not).
As the motivation for control of residual emissions and the specific situation of each landfill is highly diverse, the definition of abatement targets is subject to consideration by the relevant stakeholders (e.g., competent authorities, municipalities, operators). The abatement target for any site incurs different requirements on the design process. In different countries different general targets are pursued, and, in parts, target values are proposed for emission levels, oxidation rates, and oxidation efficiencies (Ritzkowski, 2018; Supplementary Material S1).
Depending on the boundary conditions, the following options are conceived to estimate CH4 fluxes:
(1) If a gas collection system is present and the methane oxidation system shall be connected to it, gas composition and flow rate (i.e. CH4 load) are easily determined at the gas collection system (gas well, outlet pipe leading to methane oxidation system etc.). The CH4 load can either be determined under passive conditions (gas flow following pressure difference between landfill and atmosphere only), or by a gas pumping test. The latter allows loading estimation within the sphere of influence of the specific well.
(2) If the methane oxidation system serves to remediate a hotspot, a baseline study should be performed to assess the greatest CH4 emission possible from the hotspot under a worst-case scenario. Accordingly, measurements should be carried out when, for example, soil moisture values are high, temperatures are low, and atmospheric pressure is decreasing. This would ensure minimal (to none) CH4 oxidation within the cover material during measurement. The convergence of load and emission can be ascertained by measuring the ratio of CH4 and CO2 in the emitted gas. If this ratio equals the one measured in the landfill gas, it can be assumed that there is no oxidation (carbon balance approach, Christophersen et al., 2001; Gebert et al., 2011c; Geck et al., 2016a).
(3) If the MMOS is a biocover to be implemented over the entire landfill or landfill cell, and if no recent or reliable data on gas generation of the underlying waste body are available, gas generation can be estimated using gas prognosis models (an overview is given by Scharff and Jacobs, 2006; Andreottola et al., 2019; Sun et al., 2019; Lagos et al., 2017). The reliability of gas production modelling depends highly on the available information on the waste in place and its time of deposition; the resulting uncertainty needs to be considered.
This section describes the requirements on materials for use in MMOS layers, including the respective rationale. The parameters that should be analysed to assess the materials’ suitability and the respective analytical methods are given in Supplementary Material S4.
GDL material should exhibit long-term stability and be inert to any biogeochemical reactions that would otherwise change its pore structure. It should also have very low (to nil) organic and inorganic carbon content. The increased partial pressure in carbon dioxide can result in precipitation of carbonates and hence in the reduction of pore volume available for gas transport (Humer and Lechner, 2001b).
Since the GDL is in a zone of changing redox conditions, the material should be free of dissolvable iron to avoid mobilisation and precipitation of iron oxides/hydroxides clogging the pore space. Corresponding recommendations have been formulated in the German technical guidance document on methane oxidation covers (LAGA Ad hoc AG Deponietechnik, 2020). Carbonate-poor or carbonate-free gravels, crushed glass and coarse quartz sands represent suitable materials. Recycled coarse materials (e.g. from Construction-Demolition-Renovation industries), if carbonate-free, or tire-shreds, could also be considered, if the risk of pollution or generation of H2S (as is the case with materials containing gypsum) and other odorous gases or CH4 are not a concern.
To warrant maximum conductivity for gas and water, GDL material should be characterised by particle diameters in the range of coarse sands or greater. Such a choice will result in a material with pores large enough to always drain freely and, thus, minimize water retention capacity, which is desirable.
Drainage layers made with geo-nets sandwiched between nonwoven geotextiles (McCartney and Zornberg 2010b) are only suitable as GDL if the load by the overlying MOL does not compress them to an extent that their conductivity to gas and water become too low. Precipitation of solutes, growth of biofilm and capillary effects at the soil-geotextile interface may also be a concern (blocking of pores). Further research would be required to assess the efficiency of geosynthetics (such as geocomposite drainage layers) in MMOS applications.
Finally, as far as thickness of coarse-grained materials is concerned, a few centimetres would suffice. However, the thicknesses of the GDL is dictated by constructability with available construction equipment and is typically recommended to be >0.1–0.3 m (for example, DepV, 2009; Nordic Council of Ministers, 2009; Sarsby, 2000). When applying loading using fishbone-like piping, it is advisable to cover the piping with the GDL material before laying down a filter layer if necessary.
As is the case of the gas distribution layer, the filter layer (if applied) is in a zone of changing redox conditions. Accordingly, one should choose materials free of dissolvable iron. When designing with granular materials, use of known conservative filter criteria (e.g. Messerklinger, 2013; U.S. Corps of Engineers, 1986) should be sufficient to prevent erosion of the MOL material, while maintaining high-enough permeability/diffusivity of water and gas. Filter layer thickness also depends on constructability.
If geosynthetic materials are used, a conservative filter criterion must be adopted (e.g. Giroud, 2010). Using geosynthetics increases the risk of interface effects such as clogging by fine particles and/or iron oxides/hydroxides or by biofilm growth.
The requirements on material properties suggested in the following concern both mineral and organic materials, with the obvious exception of requirements on organic matter content.
Soil physical properties, including hydraulic properties, are the main controlling factors of gas exchange between the soil and the atmosphere (Richter et al., 1991; Poulsen and Blendstrup, 2008; Gebhardt et al., 2009; Smith et al., 2018), and between the different layers within the MMOS (Ahoughalandari et al., 2017a; Ahoughalandari et al. 2017b; Ahoughalandari et al. 2018). Therefore, they play a prominent role in the design and performance of MMOS (Gebert et al., 2011a; Rachor et al., 2011; Van Verseveld and Gebert, 2020). Detailed design steps using hydraulic properties are presented in Design Steps Based on Hydraulic Considerations.
Compaction of the MOL material must be kept to a minimum. The use of compactors or too many passes thereof are to be avoided. The MOL material must be placed at a density that ensures its physical stability (such as slope stability in the case of biocovers) and reduces further compaction due to water infiltration or self-weight consolidation. For organic materials, microbial degradation will increase the degree of consolidation after construction., depending on the share of degradable organic matter still present. Accordingly, the pore-size distribution will be affected, thereby affecting water retention and gas transport properties of the MOL. This type of preoccupation must be incorporated in the design phase.
Compaction of the MOL may be necessary to optimize the difference in gas conductivity between MOL and GDL, which is needed to ensure spatial spread of the CH4 load at the base of the MOL, i.e. its uniform distribution. In proper design, these requirements must be balanced against the incurred reduction in MOL diffusivity.
MOL material must meet the following requirements:
(1) Long-term stability: To foster nutrient availability, soil structure, and water retention, organic matter is necessary. However, to avoid biological degradation and, hence, sustain gas permeability and MMOS functionality, designers must carefully consider using materials with minimal degradable organic matter contents (for organic materials, see Use of MOL Materials Rich in Organic Matter). In view of long-term stability, natural humus as usually present in mineral topsoils is favoured. Most topsoils can support microbial activity and sustain a vegetated cover.
(2) The contrast in hydraulic properties will inevitably create one or more capillary barriers within the MMOS. One major design concern is, therefore, avoiding widespread and permanent water clogging at the interfaces (mainly MOL/filter or MOL/GDL), which would hinder gas transport. Some level of clogging during wetter periods can be incorporated into the design. The focus point is on the volumetric air content at occlusion, θa_occ, and the associated volumetric water content at occlusion, θw_occ (see Water retention and transport, Gas Transport, Effect of Layering of Different Materials). The design steps associated with this concern are addressed in Design Steps Based on Hydraulic Considerations.
(3) The gas conductivity coefficient or coefficient of gas permeability (kGas), of the MOL, where landfill gas migrates vertically, must be lower than the gas conductivity of the GDL, where gas migrates horizontally, i.e. kGas_MOL << kGas_GDL. This ensures that landfill gas does not enter the MOL right above the gas feed lines or through cracks; on the contrary, the contrast in gas conductivity coefficients forces pressure loss to be homogenized over all path lengths, near or far to the gas inlet point (see Pairing of GDL and MOL materials regarding spatial homogenization of CH4 load). The magnitude of the difference between kGas_MOL and kGas_GDL needs to be assessed during the design phase.
(4) High air capacity: Gas diffusivity, and hence the O2 ingress required for CH4 oxidation are strongly linked to the share of freely draining pores available for gas transport, i.e. pores with equivalent diameter greater than 50 µm (Richter and Grossgebauer, 1978; Richter et al., 1991; Møldrup et al., 2000; Allaire et al., 2008; Gebert et al., 2011a). This share is referred to as “air capacity.” A conservative design must consider the state of maximum water retention (“field capacity” condition) incurred, for example, after an abundant precipitation. The air capacity comprises the share of pores that are easily drained, even under a condition of maximum water retention. As such, they remain open for gas transport. A minimum “through gate” of interconnected water-free pores, i.e. air-filled porosity above the point of occlusion (θa occ), through which oxygen can diffuse into the MOL, must be maintained. Empirical data of air capacity for different soil textures and bulk densities for mineral soil substrates are given in Supplementary Material S2. The required air capacity depends on the CH4 flux to be treated (Estimation of the Methane Flux to be Treated) and the expected CH4 oxidation capacity of the MOL material (Estimation of methane oxidation capacity).
(5) Available field capacity: If the methane oxidation system is part of a recultivation layer or a water balance layer, requirements on plant-available field capacity should be met, balancing the water demand by vegetation with the potential evapotranspiration under the given climatic conditions. For instance, in Germany (temperate European climate) the requirements listed in attachment 1 section 2.3.1 of the landfill order (DepV, 2009) must be observed: the recultivation layer should be at least 1 m thick and have an available field capacity of 140 mm. A dedicated water balance layer must be at least 1.5 m thick at an available field capacity of 200 mm. Empirical data on available field capacity for different textures and different bulk densities are given in Supplementary Material S2.
(6) Susceptibility to compaction: Compaction should in general be kept to a minimum, preventing a decrease in air capacity, and ensuring continuity of the air-filled pores. The sensitivity of air-filled porosity to compaction (increasing bulk density) for differently textured soils can be derived from Table 1 in Supplementary Material S2. Well graded soils are generally more susceptible to compaction than soils with a narrow range of particle diameter. Some compaction of MOL material is usually needed to create a contrast in kGas between the MOL and GDL, or to prevent unwanted post-construction settlement. In the planning process, an end value of bulk density for a given MOL candidate material should be defined, and all relevant properties should be determined for the associated relative compaction (Proctor density).
(7) Susceptibility towards crack formation: Secondary macropores, such as cracks and clefts resulting from soil aggregation and/or settlement or desiccation, create preferential pathways for gas migration and are a frequent cause of high local emissions. Fine-grained loamy textures, which are prone to aggregation due to their clay content, are hence not suitable for methane oxidation layers. In addition, such fine-grained soils hold too much water, which can obstruct gas diffusion.
If mineral soils are to be used as MOL material, a texture dominated by the sand fraction or artificial mineral substrates such as porous clay (Gebert et al., 2003; Gebert et al., 2009) or perlite (Pratt et al., 2012) are excellent candidates to be employed in methane oxidation systems, given the above criteria. Supplementary Material S2 provides values for air capacity, field capacity, and available field capacity for a large range of particle size distributions (textures) of mineral soils, useful to deduce gas conductivity and diffusivity (air capacity, in connection with Gas transport), water retention (field capacity) and its plant available share (available field capacity).
To offer a geochemical environment favourable for methanotrophic activity and to provide adequate nutrient supply, MOL materials should have the following chemical properties:
(1) pH value: Between 5.5 and 8.5 (Scheutz et al., 2009a), which is the optimum pH for methanotrophs reported in the literature.
(2) Organic matter content between 2 and 8% with respect to dry weight for mineral soils forming the topsoil, (TOC = 1–4%) less than 1% for the subsoil; to enhance nutrient supply and plant-available field capacity. Organic rich materials like composts are an exception, see Use of MOL materials rich in organic matter.
(3) Electric conductivity: lower than 4 mS/cm. At higher values, methanotrophic activity declines significantly due to osmotic stress (Gebert et al., 2003; Zhang et al., 2011). Thermally treated materials (such as porous clay, construction and demolition residues) are prone to elevated salt concentrations.
(4) Ammonium: Ammonium (NH4+) competes with CH4 enzymatic binding sites and has been reported to inhibit CH4 oxidation (e.g. De Visscher and Van Cleemput, 2003b). Materials with high NH4+ concentrations as part of the mineral N fraction should therefore be avoided.
Organic materials such as compost are frequently used solely or as an amendment to mineral covers (e.g., Humer and Lechner, 2001a, b; Barlaz et al., 2004; Huber-Humer et al., 2011) or as filter beds (mixed or not with soils or other structuring materials) for biofilters (Du Plessis et al., 2003; Dever et al., 2011) and biowindows (Pedersen et al., 2011; Roncato and Cabral, 2012; Capanema et al., 2013; Ndanga et al., 2013; Cassini et al., 2017). Composts usually offer high nutrient supply, high surface area for microbial colonisation; but also, high water retention capacity. Due to the presence of coarse pores, gas diffusivity as well as gas and water conductivity are generally high. Thermal conductivity in porous compost substrates is generally low, resulting in greater insulation within the cover that would be beneficial to CH4 oxidation rates under cooler climatic conditions, since self-heating due to the exothermic methane oxidation process can positively impact the overall MMOS (Huber-Humer, 2004a). However, there may be a further undesired increase in temperature within the MOL due to the decomposition process of the organic material, if too immature compost materials are applied. This could have the opposite effect especially in warmer seasons; however, this effect has not yet been documented in the scientific literature.
High CH4 oxidation rates up to 24 g m−2 h−1 have been observed for composts (Felske 2003; Scheutz et al., 2003, 2009b; Huber-Humer, 2004a; Barlaz et al., 2004; Wilshusen et al., 2004; Abichou et al., 2006, 2009; Haubrichs and Widmann, 2006; Mor et al., 2006; Stern et al., 2007; Tanthachoon et al., 2008; Philopoulos et al., 2009; Huber-Humer et al., 2011; Pawlowska et al., 2011; Mei et al., 2015) and up to 33 g m−2 h−1 for compost mixed with sand (Roncato and Cabral, 2012). Another advantage of compost is that it is usually easily available at a low cost.
The main disadvantage of organic materials lies in their microbial degradability, eventually leading to settlement and a subsequent reduction of gas permeability/conductivity and diffusivity. Mixing compost with rather inert structuring materials, such as large wood chips or mineral soils, reduces the problems related to diminished permeability/diffusivity. Further, the aerobic degradation of organic matter competes with the high oxygen requirements of the CH4 oxidation process (Scheutz et al., 2011b). Therefore, the organic substance must be “stable,” meaning not easily degradable and available for microorganisms. The Austrian guideline on methane oxidation layers (ÖVA, 2008), for example, therefore stipulates a maximum respiratory activity of <8 mg O2 per g dry substance measured over 7 days. Under moist conditions, high content of organic matter that is not sufficiently humified and hence stabilised, enhances the formation of anaerobic niches and can lead to the formation of CH4 from the methane oxidation system or the landfill cover itself (Barlaz et al., 2004; Scheutz et al., 2009b; Bogner et al., 2011). Mei et al. (2015) observed CH4 generation from aged green waste (not treated by composting) that met the respiration (maturity) requirement of <8 mg O2 gDS 7d−1 and that had been classified as “very mature” according to the California Compost Quality Council, 2001). However, in this study, inhibition of microbial respiration may have occurred during respiration tests due to low pH-values, which can lead to longer lag-phases and thus suppressed respiration values in the 7-day testing process.
More recently, the potential of biochar to enhance CH4 oxidation has been investigated. Biochar is a porous carbon-rich product made from pyrolysis of biomass produced in air-free conditions at 350–750°C. Literature suggests that amending soils with biochar can positively impact soil health by changing physicochemical and biological properties of soils (Vijay et al., 2021). Biochars have large surface areas, increase soil porosity, water retention, reduce soil bulk density, and change soil texture (Yi et al., 2020). These characteristics make biochar a suitable amendment for MOS. Biochars have been used as a soil amendment to enhance CH4 oxidation in landfill covers, where CH4 removal rates were enhanced with biochar when incubated over 500 days (Yargicoglu and Reddy 2017a). Biochar application rate of 2% (w/dw) at 5–6% gravimetric moisture content was correlated with enhanced methane oxidation rates (Yargicoglu and Reddy, 2017a), and the highest methane oxidation rates were observed on landfill cover soil amended with 10% (w/dw) biochar (Yargicoglu and Reddy, 2018). The results from these experiments suggested that biochar surface pores enabled higher water sorption than the control soils, thereby enhancing the population of methanotrophs (Zornberg et al., 2010; Yargicoglu and Reddy, 2017a; Yargicoglu and Reddy, 2017b; Yargicoglu and Reddy, 2018).
Overall, the use of degradable organic materials such as composts requires enhanced quality control (chemical and particularly biological stability parameters) and monitoring effort, especially with respect to settlement, moisture accumulation and competition for available O2. As mentioned previously, mixing compost with structuring materials, such as large wood chips or mineral soils may reduce occurrence of blockage due to excess water accumulation or diminished diffusivity. Loosening the MOL, in case of compaction, or excavation and exchange of MOL material can be part of the design strategy that would render mature compost a viable option.
Uniform spatial CH4 load distribution reaching the MOL prevents overloading and hotspot formation. This can be accomplished by considering the following concerns: The first relates to the extent of pore blockage due to water seepage and accumulation along the MOL/GDL (or FL) interface. One of the design goals must be to maximize the length of unrestricted gas migration (LUGM, explained in detail in Design Steps Based on Hydraulic Considerations) along the sloped interface. In other words, the design must ensure that the area where biogas can enter the MOL is maximized.
The second relates to minimization of the difference in pressure loss between the longest path and the shortest path (the latter being typically straight up from the gas inlet point to the MOL) followed by the landfill gas before reaching the base of the MOL. This is achieved when the kGas of the GDL exceeds kGas of the MOL. The magnitude of the difference between kGas_MOL and kGas_GDL needs to be assessed during the design phase. The design goal should be to minimize differences in pressure loss within the GDL over its base area. The necessary difference in conductivity depends on the distance between gas inlet points in the GDL and on the acceptable differences in pressure loss. For example, if the pressure loss difference between the closest (higher) and the farthest (lower) point is 5%, then the closest point would receive a correspondingly higher CH4 load. This would require a higher CH4 oxidation capacity at the closest point in order not to have any emissions. Depending on the robustness of the design, the methanotrophic population can easily adapt to a slightly higher load. Alternatively, small differences in efficiency across the site could also be accepted.
Typical GDL materials, such as coarse sand or gravel, have a high share in large diameter pores and retain very little water, warranting a high gas conductivity. The difference in gas conductivity between GDL and MOL is, therefore, mostly driven by the properties of the MOL material. Which soil texture, degree of compaction and, hence, construction practice is adequate, depends on the magnitude of the bottom landfill gas flux and the area over which the gas loading must be distributed. In case that a gas supply system is available, perforated tubing could also be used to enhance spatial distribution of gas within the GDL.
The CH4 oxidation capacity of a material under specific environmental conditions can be determined in laboratory tests such as batch or column test sets (see an overview in Tables 2, 3 of Scheutz et al., 2009a). An estimate of the CH4 oxidation capacity under expected field conditions can be obtained using different models. The Methane Oxidation Tool (MOT, Gebert et al., 2011d), for example, considers the impact of the three most relevant influential factors on the CH4 oxidation process: soil temperature, water potential (or matric suction) and water-free (air-filled) pore volume and calculates an oxidation potential based on empirical relationships between these parameters and observed CH4 oxidation and O2 diffusion rates. As soil temperature and matric suction are dependent on the local climate, the most relevant factor that can be designed for is the air-filled porosity, governing diffusive ingress of O2 (Figure 6) and hence the soil’s CH4 oxidation capacity. Design is made by choice of a soil with appropriate particle size distribution and its level of compaction (Supplementary Material S2). If the available area is limited, it is also possible to follow an inverse approach: the MOT can be used to calculate requirements on water-free porosity, and therefore, on particle size distribution and density required to reach the CH4 oxidation capacity that meets the desired design levels, for a given area. In a further iteration, the plausibility of the calculated required capacity then needs to be assessed using the available literature.
A more refined approach has been introduced by Spokas et al. (2011), presenting a process-based inventory model for landfill CH4 emissions considering seasonal variability of soil microclimate and CH4 oxidation activity (California Landfill Methane Inventory Model, CALMIM). However, CALMIM considers diffusion as the sole gas transport mechanism, neglecting advective processes. Abichou et al. (2009) developed a numerical model to simulate CH4 transport and oxidation through a biocover. This model includes governing equations to simulate advective and diffusive transport, and CH4 oxidation. The role of vegetation can also be simulated by the model (Abichou et al., 2011; Abichou et al., 2015). Molins and Mayer, 2007, Molins et al., 2008) and De Visscher and Van Cleemput (2003a) provide a substrate-concentration dependent kinetic CH4 oxidation model that is coupled to gas transport models.
As system design must cover the long-term perspective, it is important to consider the possible reduction in air capacity or the large diameter pores due to expected self-consolidation after placement of the methane oxidation layer (see Supplementary Material S3, settlement). Also, clogging of the pore system due to biofilm growth can reduce permeability, particularly under high loads, and has so far mainly been reported for compost-based MMOS (Hilger et al., 1999; Wilshusen et al., 2004). The consequences from reduced diffusivity and conductivity on system performance – therefore on emissions calculations and subsequent reporting – can be calculated using settlement, water content, and suction data. With such data, one can reassess the saturated hydraulic permeability (Abdolahzadeh et al., 2011b), redraw the water retention curve and the kfct, and use the MOT, CALMIM, or other appropriate models to continuously assess the oxidation rate over large areas with greater precision.
In MMOS design, the estimated CH4 oxidation capacity is valid for the MOL area that is loaded with landfill methane, hence the area corresponding to the length of unrestricted gas migration (LUGM) at the MOL-GDL interface (Design Steps Based on Hydraulic Considerations).
To obtain a reliable estimate of both CH4 load and CH4 oxidation rate, a baseline data pool providing a statistically sound basis to design the MMOS must be available or must be generated. This baseline study should cover measured or modelled data on the possible temporal variability, including states of stress induced by, for example, very high or very low temperatures and very high and very low moisture contents.
By example, the dimensioning approach described in the Austrian guideline on methane oxidation covers (ÖVA, 2008) relies on the maximal CH4 oxidation capacity (= oxidation potential) of the selected material for the MOL (determined under suitable and controlled conditions in lab pre-tests at 20°C) and the maximal expected (calculated/measured) CH4 load per m2 in the field should - with respect to safety issues - not exceed 50% of the determined oxidation capacity in the lab.
Examples of studies and factors driving variability of emissions and CH4 oxidation in the field are:
(1) Atmospheric pressure: Czepiel et al. (2003); Gebert and Groengroeft (2006b); Nwachukwu and Anonye (2013); Poulsen et al. (2013); Rachor et al. (2013); Xu et al. (2014).
(2) Wind: Poulsen and Møldrup (2006).
(3) Soil temperature and moisture: Borjesson et al. (2004); Ishigaki et al. (2005); Gebert and Groengroeft (2006a); Jugnia et al. (2008); Tecle et al. (2008); Rachor et al. (2013); Zhang et al. (2011), Geck et al. (2016a).
(4) CH4 concentration and load: Dever et al. (2005); Röwer et al. (2011); Geck et al. (2016b).
In case that the gas load to the MMOS is not routed through a gas supply pipe but migrates freely from the waste body into the GDL, the spatial variability of the CH4 load from the waste to the MMOS-to-be-built should be assessed during the baseline study.
Microbial methane oxidation systems are often built on slopes, as the surface of many landfills is sloped. If built on a plateau, the interface of MOL and GDL should be sloped to facilitate drainage of percolating water. Specific challenges are posed to system design due to the potentially conflicting water and gas transport processes, as discussed in Gas transport. The contrasting textures between coarse GDL and finer MOL material, and therefore, their contrasting hydraulic properties create a capillary break, which leads to accumulation of moisture in the zone just above their interface. This phenomenon of moisture accumulation, the capillary barrier effect, depends on material characteristics, on climate and, further, on construction design (amongst others slope angle and diversion length). Upslope migration of landfill gas may lead to areas that become overloaded with CH4, which result in higher surface emissions, or hotspots (Wawra and Holfelder, 2003; Berger et al., 2005; Geck et al., 2016b; Röwer et al., 2016b). Further, lateral migration within the GDL redirects gas flow to the side walls of the MMOS, a zone sensitive to the development of preferential pathways. One relevant goal in MMOS design is therefore the maximization of the area or path length free of obstruction to gas migration.
Ahoughalandari et al. (2018) introduced a design criterion that translates the concern about pore occlusion at interfaces: The Length of Unrestricted Gas Migration (LUGM). As schematically presented in Figure 7, LUGM is the length along the MOL-GDL interface where upward gas migration is unrestricted (if a filter layer is needed, LUGM should be obtained along the MOL-FL interface). LUGM is highly dependent on unsaturated hydraulic conductivity and hence on material properties and characteristics, and on climate as well as on slope inclination and diversion length. It will vary seasonally, being shorter in seasons of high precipitation and low temperature (reduced evapotranspiration), and longer or extending to the full length of the interface in drier and warmer seasons. The influence of several key parameters on the magnitude of LUGM was assessed by Ahoughalandari and Cabral (2017b).
Key to high performance of sloped biofilters, biowindows or biocovers is to design the system in a way that the value of LUGM is maximized so that the CH4 loading can migrate freely to a larger area, where it is oxidized, reducing CH4 emissions to the desired levels. A conservative value of LUGM must be determined during the design phase. Generally, the location of the point of occlusion is not a singularity. Rather, pore occlusion occurs within a certain zone. The conservative way is to adopt as occlusion volumetric air content (θa-occ) the highest value obtained from air permeability tests (θa-pocc in Figure 3, right).
Step 1. Determine LUGM. This requires a sequence of steps that starts with laboratory testing to determine the volumetric air content at occlusion, θa_occ (see Water Retention and Transport, Gas Transport) for the chosen substrate at the chosen degree of compaction.A simplified approach is proposed by Ahoughalandari et al. (2018), who found that the degree of water saturation, Sr, associated with the line of optima for a sand-compost mixture used as MOL coincided with the Sr values when occlusion of air pores was detected during the determination of the ka-function for this soil at different compaction energies. This seems to indicate that θa_occ could potentially be inferred from the optimum water content determined from the Standard Proctor test (ASTM D6–8 - 12e2). However, for another substrate (fine sand) also successfully employed as MOL (Ndanga et al., 2015), Ahoughalandari et al. (2018) found that the Sr value associated with occlusion was greater than that given by the line of optima. As such, the simplification proposed, i.e. use the optimum moisture content as an indicator of occlusion must be used with care. Further studies will eventually offer more precise guidelines about this simplification for other types of soils. For the time being, it is still recommended to obtain θa_occ from the ka-function.In mineral soils, certainly in sand-dominated soils, θa_occ and the soil’s air capacity (the share of pores >50 µm available for gas transport at maximum water retention, see The water Retention Curve (WRC) and Pore Size Distribution) are closely related. Accordingly, an alternative to assessing θa_occ is to obtain the air capacity as air filled porosity at a suction (pore pressure) of −6 kPa. Alternatively, air capacity can be estimated from the particle size distribution and bulk density (Supplementary Material S2).Based on the linear relationship between air filled porosity and soil effective diffusivity (Diffusive Gas Transport) it can be assessed whether the effective diffusivity of the soil allows for a diffusive influx of oxygen that suffices to oxidise the accruing CH4 load. It is suggested to assume the top 40 cm as active CH4 oxidation layer.
Step 2. Once θa_occ has been determined, its associated volumetric water content at occlusion, θw_occ, can be calculated as follows: θw_occ = n-θa_occ, where n is the porosity of the soil, and ρd (M.L−3) is the dry bulk density to which the material will be compacted. At the design phase, it is recommended to assess θa_occ for several values of ρd.
Step 3. In this next step, numerical modelling is used to assess where θw_occ is reached along the MOL-GDL (or MOL-FL) interface. It is recommended to test different pre-selected MOL candidates at various slopes. Several runs of the model are performed for the various materials, densities and θw_occ associated with these densities.Several commercial programs can be used to perform this design step (e.g. Vadose/W, Hydrus-2D, Unsat-H). Input parameters include the WRC (or the parameters describing it; The water retention curve (WRC) and pore size distribution) and the hydraulic permeability function (kfct; The hydraulic permeability function (k-fct)) of the MOL, climatic data and boundary conditions. The most problematic boundary condition is found at the interface between the MOL and the filter or the GDL. The reason for this is simple: the slope of the kfct of granular materials is often steep, which poses serious convergence problems (small changes in suction lead to significant changes in the value of the hydraulic permeability). There is no standard practice to avoid this; one solution is to not include the FL (or GDL) altogether and consider this interface as a seepage face. In most programs (perhaps using different nomenclature), when the water entry value (ψWEV; Figure 2) of the FL (or GDL) layer is reached at this boundary, water flows out of the system and is computed as a boundary flux. Usually, the bottom boundary is considered as a unit-gradient interface. The framework must be big enough to avoid interference from lateral boundaries.
Step 4. Revisit to step 1 until the value of LUGM is high enough for the corresponding area to be sufficiently large to cope with the design CH4 loading (Estimation of the Methane Flux to be Treated), given the estimated CH4 oxidation capacity (Estimation of methane oxidation capacity).Further considerations concerning designing focusing on hydraulic aspectsConcepts, such as the jagged interface proposed by Cassini et al. (2017) may help reduce the negative impact of the capillary barrier effect on the response of the system. The jagged interface may leave a length of unrestricted gas migration near each of the crests. In configurations with such jagged interface, there is a compromise to be reached between keeping LUGM as high as possible, and a large enough difference in moisture content values between the top and the bottom of each segment. This is possible by an appropriate choice of geometrical features and materials whose drainage capacity may lead to less moisture accumulation. Ahoughalandari and Cabral (2017a) assessed LUGM for variants of this scheme for a specific climate and concluded that, in certain cases, LUGM can be practically nil (i.e. landfill gas would be forced to migrate to the top of the MMOS, drastically reducing its CH4 oxidation efficiency), depending on the materials (and stack thereof) selected, and on the length of each segment. One potential drawback of this concept are the high construction costs.An alternative option proposed by Röwer et al. (2016b) is to increase the diversion length for downslope water transport beyond the dimensions of the MMOS, so that the occluded part of the GDL-MOL interface remains downslope of the area intended to accomplish the CH4 oxidation process. Thereby, LUGM may be maximised along the spatial extension of the methane oxidation system. In any case, dimensioning of sloped MMOS should be iterative. Following determination of the required area (MMOS surface area and thickness), one should assess how much this area is compromised by a temporary reduction of LUGM due to water seepage following a precipitation event, and whether the increased CH4 load in upslope direction does or does not exceed the CH4 oxidation capacity of the upslope area. If it does exceed, the lateral extension of the MMOS should be enlarged accordingly.
To determine the base area of the methane oxidation system onto which the gas load must be distributed in order to warrant complete oxidation, the emission (in case of a hotspot) or the known CH4 load is divided by the oxidation capacity, i.e.: Area = Emission/OC, where Area is the base area of the MMOS (m2); Emission is the CH4 load (for example given in g CH4 h−1), and OC is the CH4 oxidation capacity (for example, given in g CH4 m−2 h−1). An example for the determination of the needed area based on pre-lab-test of the MOL-material is given in ÖVA (2008), where calculations included a safety-factor for field applications.
It is relevant to note that the area is directly determined by the magnitude of the previously calculated LUGM. For example, if LUGM corresponds to 70% of the entire length of the MMOS, then the area needs to be reduced accordingly. Several iterations may be needed during the design phase to evaluate different thicknesses (which affects OC and then the area), lengths, slopes, and materials. Determination of the oxidation capacity is time-consuming and requires capacity in a laboratory. Alternatively, one can resort, for example, to the Methane Oxidation Tool (Estimation of Methane Oxidation Capacity) for an approximation.
In many national regulations, the minimum thickness of a landfill cover is stipulated to be 1 m and requirements, for example, on the amount of water that can be stored or is available to the cover vegetation, are based on this thickness. The Methane Oxidation Tool assumes that the oxidation process occurs in the top 40 cm of the MOL. However, the final thickness of the MMOS needs to be greater, because the top part of the soil is most prone to desiccation and very high temperatures in the dry and/or warm period, and to colder temperatures during the rest of the year. Moreover, if the MMOS is to be vegetated, requirements on water storage also need to be met. Altogether, these aspects ask for redundancy in thickness. In the German technical recommendation on biocovers (LAGA Ad hoc AG Deponietechnik, 2020), thickness is therefore aligned with the requirement on thickness for landfill covers in general, i.e. 1 m. However, this does not preclude design.
Vegetating open MMOS prevents wind or water-induced erosion or crusting of the MOL through the above ground biomass and the proliferation of roots, which warrants a well-structured MOL matrix. It also visually integrates the landfill cover to the surrounding environment. A well rooted MOL matrix can enhance diffusive ingress of atmospheric air and thereby improve the efficiency of the MMOS (Bohn et al., 2011; Reichenauer et al., 2011). Ndanga et al. (2015), on the other hand, did not find relevant gains in oxidation efficiency when selected soils were vegetated with different plant mixes as compared to non-vegetated ones.
It is highly recommended to include top soil with organic matter (humus) to support the main rooting zone if mineral materials are used for the MOL sub-soil. This increases water and nutrient supply not only to the vegetation but also to the methanotrophic and other microorganisms. Natural, stable humus contents in top-soils of temperate climates are sufficient. Despite the preceding, in a study involving laboratory and field experiments, Ndanga et al. (2013) alluded that plant roots can also become preferential pathways, thereby decreasing the CH4 oxidation efficiency. An even grass cover with a spatially even proliferation of roots is preferred over species with fewer, or single, but deeper roots, that create pathways for preferential gas flow. The choice of vegetation should, therefore, maximize spatial homogeneity of MOL structural properties to prevent the formation of preferential pathways. In addition, the evapotranspiration by vegetation reduces the moisture content of the MOL near the surface, thereby reducing blockage of pores in the MOL and reducing the volume of percolate, which, in MMOS with a hydraulic connection to the waste body, may contribute to landfill leachate production.
MMOS can be applied on diverse types of sanitary landfills and non-sanitary old deposits or waste dumps to reduce residual emissions. Some prominent examples are summarized below:
Worldwide, uncovered and mostly uncontrolled dumps are still a prominent problem. Old landfills that were constructed before sanitary landfill regulation came into force often fall under soil and water/groundwater protection legislation, not under waste disposal regulations. Frequently they have a cover made of any material that was available to the operator at the time of construction. As the largest part of the emissions typically occurs via spatially confined hotspots, a remediation of the relevant spots through methane oxidation windows would be a rational compromise. It is possible that, in many cases, a well-designed passive MMOS (biocover or biowindow) made of locally available materials could be implemented as part or instead of the existing cover. Such low-maintenance systems would be a much better alternative to no action, particularly in countries lacking legal requirements and viable modern and expensive technical solutions.
Typical sites in this category are sealed with natural clayey materials or geosynthetic clay liners, into which, in some cases, windows (vents) are inserted for the mere release or for the treatment of landfill gas. Often, inadequate window filling materials which have ceased to function due to settlement and consolidation are encountered with a concurrent loss of gas diffusivity and conductivity. If leaking, these windows can be remediated by exchanging the filling material, preferably with long-term stable materials.
Additionally, the clay top liner is prone to cracking due to - for example - differential settlement. Landfill gas leaks through this cracks and remediation, insofar as gas emissions are concerned, calls for installation of a MMOS.
Sanitary landfills with adequate top covers and gas collection systems and landfills for mechanically and biologically pre-treated wastes
For sites in this category, a MMOS is applicable as a complementary measure during active gas extraction and technical treatment, when the top liner is not yet in place, or as a walk-away solution, after technical gas treatment has been terminated. Existing gas wells can be used to actively or passively feed biofilters or biowindows. These can also be integrated into the cover in retrospect. If the biological gas treatment is to be carried out using the entire area of the landfill cover (biocovers) and a top liner already exists, attention needs to be given to sufficient spatial distribution of the load above the existing liner using a gas piping system within/beneath the gas distribution layer. If a top liner is already in place, this needs to be punctured/penetrated to direct the landfill gas to a gas distribution layer below a recultivation layer optimized for CH4 oxidation (biocover). For mechanically-biologically pre-treated waste landfills, i.e. with inherently low gas production, passively vented biowindows (integrated in surface liners) or biocovers are suitable.
For older sanitary landfills approaching landfill closure, but still containing relevant amounts of biodegradable organic waste (MSW), permeable temporary top covers could be a meaningful measure in certain countries. These covers shall be designed to allow water infiltration into the waste body to enhance degradation processes of the waste material. For example, permeable temporary covers for (maximal) 20 years are required in the Austrian landfill directive (DVO, 2008) for the closing phase of former landfills made of untreated municipal solid waste. However, temporary covers must concurrently mitigate CH4 emissions, i.e. act as a biocover. The Austrian landfill directive (DVO, 2008) sets limit values for gaseous CH4 emission from temporary covers, with 5 kg CH4 m−2 a−1 being the average value for the entire landfill (single hotspots shall not exceed 10 kg CH4 m−2 a−1). Landfill operators are obliged to submit a project to the local authorities regarding final covering including an impermeable liner of the landfill surface after the 20-year temporary period.
Landfills in this category usually have a low gas generation potential, making them suitable sites for passive gas collection and subsequent gas treatment by biofilters. Carefully spaced biowindows inserted in the existing top cover would also be a viable alternative. Alternatively, biocovers are a suitable option if, as above, the landfill gas can be routed to a gas distribution layer through penetrations in the existing liner if necessary.
Old municipal solid waste landfills, that still contain biodegradable waste material and on which an in-situ aeration plant is operated to accelerate the mineralisation and stabilisation processes, can be capped with a biocover to abate the remaining methane emissions (Ritzkowski and Stegmann, 2012; Laux, 2015). On in-situ aerated landfills that operate a gas collection system, the collected air-gas mixture can be actively (or passively) routed to an external biofilter. Challenges for effective operation of these filters include high gas fluxes and low CH4 concentrations. Any residual gas emissions remaining after completion of the in-situ aeration measure can be abated by a properly designed MMOS.
Figure 8 presents a decision tree that supports the decision on which MMOS would best fit the needs at a specific site. It addresses most conceivable aspects relevant for decision-making, such as recoverable CH4 flow, availability of gas extraction wells, landfill geometry, etc. The user is guided through the decision tree by following technical questions, which can be answered by yes or no. Potential after-use is an important aspect in the final decision. Questions such as whether the surface of a MMOS must be sealed for further use as, e.g., parking lot or industrial use are not addressed in this decision tree.
FIGURE 8. Exemplary decision tree on use of MMOS. Developed from the decision tree presented in ÖVA (2008).
The starting point (and most significant aspect) is to determine if there is still relevant gas formation and/or gas emission potential present on the landfill site that would justify implementing MMOS to abate CH4 emissions. Based on recommendations by Heyer et al., 2013) on criteria for release of landfills from aftercare, engineered MMOS are recommended to abate residual emissions when the approximate CH4 load to the cover layer is still greater than 0.5 L CH4 m−2 h−1 (0.36 g CH4 m−2 h−1 under conditions of standard temperature and pressure). This threshold was also recommended by ÖWAV (2008) for considerations regarding the completion of intensive aftercare measures at MSW landfills. It is assumed that lower CH4 loads can be oxidised by any cover soil or layer, also those not optimised for microbial methane oxidation. The exact magnitude of residual flux that merits the changeover from active gas extraction and technical treatment (flare or energetic use) to treatment of CH4 in MMOS is site-specific. It depends on the size of the landfill and therefore the total CH4 flux, the efficiency of active gas extraction and technical treatment that is in place, but also on the intended after-use of the site.
Further key-aspects requested in the decision tree concern technical equipment of the landfill (gas wells/gas extraction, existing cover layer, bottom liner/leachate collection), landfill geometry and protection of groundwater. Considerations and questions regarding subsequent and/or supplementary technical adaptations of the site are further aspects of the decision process.
Design of methane oxidation systems is challenging because of the necessity to consider the coupled processes of gas and water transport, in addition to microbial methane oxidation, which depends on choice of materials. Gas and water transport are impacted by temporally changing environmental conditions (e.g., temperature, precipitation), and, certainly on larger and sloped areas are always subject to some spatial variability. Overarching goals of MMOS design, beyond the choice of material that can sustain methanotrophic activity, are 1) to ensure that gas fluxes through the MMOS are evenly spaced to avoid high methane loads in certain areas, which would compromise the overall efficiency; and 2) to achieve an optimal CH4 load that can be oxidized by the MMOS. The latter includes consideration of adverse conditions such as high degrees of (water) saturation that reduce the air-filled porosity, the formation of capillary barriers provoking spatially non-uniform flow of gas, and the changes in temperature that may affect methanotrophic activity. The aspects mentioned above must be dealt with during the design phase, mainly through choice of an adequate combination of materials for use in the gas distribution and the methane oxidation layers; one that offers a delicate balance between sufficient air-filled porosity and water retention, while creating the necessary difference in permeability to avoid uncontrolled upward migration of landfill gas.
While detailed knowledge on microbial CH4 oxidation has been generated over time, a comprehensive proposal for design steps to successful MMOS was still missing. This paper aims to fill this gap and combines the scientific background with the most important aspects of system layout, design and monitoring. In doing so, it proposes guidance to the different stakeholders involved in designing, operating, and regulating methane oxidation systems for the abatement of methane emissions from waste disposal sites. Future technical guidance documents for practitioners can build on the information provided. The paper focuses exclusively on the technical fundamentals and suggests guiding principles of system choice and a step-by-step approach for implementation but does not include considerations of cost or cost-effectiveness of MMOS construction and operation. However, the choice of gas treatment system (be it thermal (flare or energy recovery) or an MMOS) and the abatement targets will always depend on multiple aspects and site-specific conditions and constraints, including the situation and land use around the site (e.g. housing), the intended post-closure after-use and economic considerations.
This review is truly a shared piece of work. All authors contributed to, reviewed and amended all sections. Focus of JG and ARC: Typical layouts, Fundamentals and Design. Focus of MH-H: Intro, Typical Layouts and Recommendations.
The Canadian author received support from the National Science and Engineering Research Council of Canada (NSERC) and Waste Management Inc. (CRD 379885-08), and from the Consortium de recherche et innovations en bioprocédés industriels du Québec (CRIBIQ).
The authors declare that the research was conducted in the absence of any commercial or financial relationships that could be construed as a potential conflict of interest.
All claims expressed in this article are solely those of the authors and do not necessarily represent those of their affiliated organizations, or those of the publisher, the editors and the reviewers. Any product that may be evaluated in this article, or claim that may be made by its manufacturer, is not guaranteed or endorsed by the publisher.
The authors would like to thank all members of the IWWG task group CLEAR, but also all other colleagues from the broader field, for the constructive meetings and fruitful discussions concerning landfill methane generation, oxidation and emission over the past years. Several agencies around the world have supported the studies cited in this paper. Their importance is also acknowledged. We also wish to thank Susan Yi and Heijo Scharff for pre-reviewing and proofreading the manuscript.
The Supplementary Material for this article can be found online at: https://www.frontiersin.org/articles/10.3389/fenvs.2022.907562/full#supplementary-material
Abdolahzadeh, A. M., Lacroix Vachon, B., and Cabral, A. R. (2011b). Assessment of the Design of an Experimental Cover with Capillary Barrier Effect Using 4 Years of Field Data. Geotech. Geol. Eng. 29 (5), 783–802. doi:10.1007/s10706-011-9417-x
Abdolahzadeh, A. M., Lacroix Vachon, B., and Cabral, A. R. (2011a). Evaluation of the Effectiveness of a Cover with Capillary Barrier Effect to Control Percolation into a Waste Disposal Facility. Can. Geotech. J. 48, 996–1009. doi:10.1139/t11-017
Åberg, B. (1992). Void Ratio of Noncohesive Soils and Similar Materials. J. Geotech. Engrg. 118 (9), 1315–1334. doi:10.1061/(asce)0733-9410(1992)118:9(1315)
Aberg, B. (1996). Void Sizes in Granular Soils. J. Geotechnical Eng. 122 (3), 236–239. doi:10.1061/(asce)0733-9410(1996)122:3(236)
Abichou, T., Chanton, J., Powelson, D., Fleiger, J., Escoriaza, S., Lei, Y., et al. (2006). Methane Flux and Oxidation at Two Types of Intermediate Landfill Covers. Waste Manag. 26, 1305–1312. doi:10.1016/j.wasman.2005.11.016
Abichou, T., Clark, J., and Chanton, J. (2011). Reporting Central Tendencies of Chamber Measured Surface Emission and Oxidation. Waste Manag. 31 (5), 1002–1008. doi:10.1016/j.wasman.2010.09.014
Abichou, T., Kormi, T., Yuan, L., Johnson, T., and Francisco, E. (2015). Modeling the Effects of Vegetation on Methane Oxidation and Emissions through Soil Landfill Final Covers across Different Climates. Waste Manag. 36, 230–240. doi:10.1016/j.wasman.2014.11.002
Abichou, T., Mahieu, K., Yuan, L., Chanton, J., and Hater, G. (2009). Effects of Compost Biocovers on Gas Flow and Methane Oxidation in a Landfill Cover. Waste Manag. 29, 1595–1601. doi:10.1016/j.wasman.2008.11.007
Abu-Hejleh, A. N., Znidarcic, D., and Illangasekare, T. H. (1993). “Permeability Determination for Unsaturated Soils,” in Proceedings of the 1993 ASCE National Convention and Exposition, October 24, 1993 - October 28. Editors S. L. Huston, and W. K. Wray (Reston (Virginia): ASCE), 163–174.
Aghdam, E. F., Scheutz, C., and Kjeldsen, P. (2019). Impact of Meteorological Parameters on Extracted Landfill Gas Composition and Flow. Waste Manag. 87, 905–914. doi:10.1016/j.wasman.2018.01.045
Ahoughalandari, B., and Cabral, A. R. (2017b). Influence of Capillary Barrier Effect on Biogas Distribution at the Base of Passive Methane Oxidation Biosystems: Parametric Study. Waste Manag. 63, 172–187. doi:10.1016/j.wasman.2016.11.026
Ahoughalandari, B., and Cabral, A. R. (2017a). Landfill Gas Distribution at the Base of Passive Methane Oxidation Biosystems: Transient State Analysis of Several Configurations. Waste Manag. 69, 298–314. doi:10.1016/j.wasman.2017.08.027
Ahoughalandari, B., Cabral, A. R., and Leroueil, S. (2018). Elements of Design of Passive Methane Oxidation Biosystems: Fundamental and Practical Considerations about Compaction and Hydraulic Characteristics on Biogas Migration. Geotech. Geol. Eng. 36 (4), 2593–2609. doi:10.1007/s10706-018-0485-z
Ait-Benichou, S., Jugnia, L.-B., Greer, C. W., and Cabral, A. R. (2009). Methanotrophs and Methanotrophic Activity in Engineered Landfill Biocovers. Waste Manag. 29 (9), 2509–2517. doi:10.1016/j.wasman.2009.05.005
Allaire, S. E., Lafond, J. A., Cabral, A. R., and Lange, S. F. (2008). Measurement of Gas Diffusion through Soils: Comparison of Laboratory Methods. J. Environ. Monit. 10, 1326–1336. doi:10.1039/B809461F
Andreottola, G., Cossu, R., and Ritzkowski, M. (2019). “Landfill Gas Generation Modelling,” in Solid Waste Landfilling - Concepts, Processes, Technologies. Editors R. Cosu, and R. Stegmann (Amsterdam: Elsevier), 419–437. doi:10.1016/C2012-0-02435-0
Arya, L. M., Leij, F. J., van Genuchten, M. T., and Shouse, P. J. (1999). Scaling Parameter to Predict the Soil Water Characteristic from Particle-Size Distribution Data. Soil Sci. Soc. Am. J. 63 (3), 510–511519. doi:10.2136/sssaj1999.03615995006300030013x
Arya, L. M., and Paris, J. F. (1981). A Physicoempirical Model to Predict the Soil Moisture Characteristic from Particle-Size Distribution and Bulk Density Data. Soil Sci. Soc. Am. J. 45, 1023–1030. doi:10.2136/sssaj1981.03615995004500060004x
Barbour, S. L. (1998). Nineteenth Canadian Geotechnical Colloquium: The Soil-Water Characteristic Curve: a Historical Perspective. Can. Geotech. J. 35 (5), 873–894. doi:10.1139/t98-040
Barlaz, M. A., Green, R. B., Chanton, J. P., Goldsmith, C. D., and Hater, G. R. (2004). Evaluation of a Biologically Active Cover for Mitigation of Landfill Gas Emissions. Environ. Sci. Technol. 38, 4891–4899. doi:10.1021/es049605b
Berger, J., Fornés, L. V., Ott, C., Jager, J., Wawra, B., and Zanke, U. (2005). Methane Oxidation in a Landfill Cover with Capillary Barrier. Waste Manag. 25 (4), 369–373. doi:10.1016/j.wasman.2005.02.005
Blume, H.-P., Brümmer, G. W., Fleige, H., Horn, R., Kandeler, E., Kögel-Knabner, I., et al. (2016). Scheffer/Schachtschabel – Soil Science. Springer, 618. doi:10.1007/978-3-642-30942-7
Bogner, J., Abdelrafie Ahmed, M., Diaz, C., Faaij, A., Gao, Q., Hashimoto, S., et al. (2007). “Waste Management,” in Climate Change: Mitigation. Contribution of Working Group III to the 4th Assess. Report of the Intergovernmental Panel on Climate Change (Cambridge, New York: Cambridge University Press), 586–618.
Bogner, J. E., Chanton, J. P., Blake, D., Abichou, T., and Powelson, D. (2010). Effectiveness of a Florida Landfill Biocover for Reduction of CH4 and NMHC Emissions. Environ. Sci. Technol. 44, 1197–1203. doi:10.1021/es901796k
Bogner, J. E., Spokas, K. A., and Chanton, J. P. (2011). Seasonal Greenhouse Gas Emissions (Methane, Carbon Dioxide, Nitrous Oxide) from Engineered Landfills: Daily, Intermediate, and Final California Cover Soils. J. Environ. Qual. 40, 1010–1020. doi:10.2134/jeq2010.0407
Bohn, S., Brunke, P., Gebert, J., and Jager, J. (2011). Improving the Aeration of Critical Fine-Grained Landfill Top Cover Material by Vegetation to Increase the Microbial Methane Oxidation Efficiency. Waste Manag. 31, 854–863. doi:10.1016/j.wasman.2010.11.009
Börjesson, G., Sundh, I., and Svensson, B. H. (2004). Microbial Oxidation of CH4 at Different Temperatures in Landfill Cover Soils. FEMS Microbiol. Ecol. 48, 305–312.
Bour, O., Zdanevitch, I., Huyard, A., Akerman, A., Lacour, G., and Chassagnac, T. (2015). “Passive Biomitigation of Diffuse Landfill Gas Emissions on 12 French Landfills: Return of Experience on Design Criteria Used,” in Proceedings of Sardinia 2015 - 15th International Waste Management and Landfill Symposium (Italy: CISA).
Cabral, A. R., Capanema, M. A., Gebert, J., Moreira, J. F., and Jugnia, L. B. (2010a). Quantifying Microbial Methane Oxidation Efficiencies in Two Experimental Landfill Biocovers Using Stable Isotopes. Water Air Soil Pollut. 209 (1-4), 157–172. doi:10.1007/s11270-009-0188-4
Cabral, A. R., Moreira, J. F. V., and Jugnia, L.-B. (2010b). Biocover Performance of Landfill Methane Oxidation: Experimental Results. J. Environ. Eng. 136 (8), 785–793. doi:10.1061/(asce)ee.1943-7870.0000182
Capanema, M. A., and Cabral, A. R. (2012). Evaluating Methane Oxidation Efficiencies in Experimental Landfill Biocovers by Mass Balance and Carbon Stable Isotopes. Water Air Soil Pollut. 223 (9), 5623–5635. doi:10.1007/s11270-012-1302-6
Capanema, M. A., Ndanga, É. M., Lakhouit, A., and Cabral, A. R. (2013). “Methane Oxidation Efficiencies of a 6-Year-Old Experimental Landfill Biocover,” in Proceedings of the Fourteenth International Waste Management and Landfill Symposium (Sardinia 2013). S. Margherita di Pula, Cagliari, Italy, 30 Sept-4 Oct. 2013. Editor R. Cossu (Padova: CISA Publisher), 209.
Cassini, F., Scheutz, C., Skov, B. H., Mou, Z., and Kjeldsen, P. (2017). Mitigation of Methane Emissions in a Pilot-Scale Biocover System at the AV Miljø Landfill, Denmark: 1. System Design and Gas Distribution. Waste Manag. 63, 213–225. doi:10.1016/j.wasman.2017.01.013
Christophersen, M., Kjeldsen, P., Holst, H., and Chanton, J. (2001). Lateral Gas Transport in Soil Adjacent to an Old Landfill: Factors Governing Emissions and Methane Oxidation. Waste Manag. Res. 19, 595–612. doi:10.1177/0734242x0101900616
Czepiel, P. M., Shorter, J. H., Mosher, B., Allwine, E., McManus, J. B., Harriss, R. C., et al. (2003). The Influence of Atmospheric Pressure on Landfill Methane Emissions. Waste Manag. 23, 593–598. doi:10.1016/s0956-053x(03)00103-x
De Visscher, A., and Cleemput, O. V. (2003b). Induction of Enhanced CH4 Oxidation in Soils: NH4+ Inhibition Patterns. Soil Biol. Biochem. 35, 907–913. doi:10.1016/s0038-0717(03)00122-6
De Visscher, A., and van Cleemput, O. (2003a). Simulation Model for Gas Diffusion and Methane Oxidation in Landfill Cover Soils. Waste Manag. 23 (7), 581–591. doi:10.1016/s0956-053x(03)00096-5
DepV – Deponieverordnung (2009). Verordnung über Deponien und Langzeitlager, vom 27.04.2009 (BGBl. I, Nr. 22, S. 900), zuletzt geändert durch Artikel 2 der Verordnung vom 30. Juni 2020 (BGBl. I Nr. 32), 1533.
Dever, S. A., Swarbrick, G. E., Annett, L., and Stuetz, R. M. (2005). “The Effect of Landfill Gas Loading on the Performance of a Passive Biofiltration System Operating under Australian Conditions,” in Tenth International Waste Management and Landfill Symposium (Padova: CISA).
Dever, S. A., Swarbrick, G. E., and Stuetz, R. M. (2011). Passive Drainage and Biofiltration of Landfill Gas: Results of Australian Field Trial. Waste Manag. 31, 1029–1048. doi:10.1016/j.wasman.2010.10.026
DVO – Deponieverordnung (2008). Verordnung des Bundesministers für Land- und Forstwirtschaft, Umwelt und Wasserwirtschaft über Deponien. BGBl. II Nr. 39/2008, geändert durch BGBl. II Nr. 185/2009 und BGBl. II Nr. 178/2010, zuletzt geändert durch die Verordnung BGBl. II Nr. 455/2011 German. Austria.
Einola, J., Sormunen, K., Lensu, A., Leiskallio, A., Ettala, M., and Rintala, J. (2009). Methane Oxidation at a Surface-Sealed Boreal Landfill. Waste Manag. 29, 2105–2120. doi:10.1016/j.wasman.2009.01.007
FAO (1985). Irrigation Water Management: Training Manual No. 1 - Introduction to Irrigation. Available at: http://www.fao.org/3/r4082e/r4082e00.htm#Contents.
Fischedick, F., Cabana, H., Ndanga, É. M., and Cabral, A. R. (2018). Biofiltration of Methane from Cow Barns: Effects of Climatic Conditions and Packing Bed Media Acclimatization. Waste Manag. 78, 669–676. doi:10.1016/j.wasman.2018.06.038
Felske, C. (2003). Minimierung von Restgasemissionen aus Siedlungsabfalldeponien durch Methanoxidation in Deponieabdeckschichten, Doctoral thesis at the University Duisburg-Essen, Fachbereich Bauwesen, Schriftenreihe Forum Siedlungswasserwirtschaft und Abfallwirtschaft. Essen: Universität Essen, 20.
Fredlund, D. G., and Xing, A. (1994). Equations for the Soil-Water Characteristic Curve. Can. Geotech. J. 31, 521–532. doi:10.1139/t94-061
Fredlund, M. D., Wilson, G. W., and Fredlund, D. G. (2002). Use of the Grain-Size Distribution for Estimation of the Soil-Water Characteristic Curve. Can. Geotech. J. 39 (5), 1103–1117. doi:10.1139/t02-049
Gebert, J., GrÃngrÃft, A., Schloter, M., and Gattinger, A. (2004). Community Structure in a Methanotroph Biofilter as Revealed by Phospholipid Fatty Acid Analysis. FEMS Microbiol. Lett. 240, 61–68. doi:10.1016/j.femsle.2004.09.013
Gebert, J., Groengroeft, A., and Miehlich, G. (2003). Kinetics of Microbial Landfill Methane Oxidation in Biofilters. Waste Manag. 23, 609–619. doi:10.1016/s0956-053x(03)00105-3
Gebert, J., and Groengroeft, A. (2006b). Passive Landfill Gas Emission - Influence of Atmospheric Pressure and Implications for the Operation of Methane-Oxidising Biofilters. Waste Manag. 26, 245–251. doi:10.1016/j.wasman.2005.01.022
Gebert, J., Groengroeft, A., and Pfeiffer, E.-M. (2011a). Relevance of Soil Physical Properties for the Microbial Oxidation of Methane in Landfill Covers. Soil Biol. Biochem. 43, 1759–1767. doi:10.1016/j.soilbio.2010.07.004
Gebert, J., and Gröngröft, A. (2006a). Performance of a Passively Vented Field-Scale Biofilter for the Microbial Oxidation of Landfill Methane. Waste Manag. 26, 399–407. doi:10.1016/j.wasman.2005.11.007
Gebert, J., Gröngröft, A., Rachor, I., Geck, C., Streese-Kleeberg, J., Back, S., et al. (2017). Systeme zur Methanoxidation auf Deponien, Leitfaden II des Forschungsverbundes MiMethox. Hambg. Bodenkd. Arb. 81. doi:10.2136/sssaj2016.11.0387
Gebert, J., Harms, C., and Steinert, B. (2015). “Full-scale Implementation of Methane Oxidation Windows on a Mono-Landfill Technical Design and Monitoring Concept,” in Proceedings Sardinia 2015, Fifteenth International Waste Management and Landfill Symposium (Italy: CISA).
Gebert, J., Huber-Humer, J., Oonk, H., and Scharff, H. (2011d). Methane Oxidation Tool – an Approach to Estimate Methane Oxidation on Landfills. Available at: https://www.afvalzorg.com/content/uploads/2020/05/Methane-Oxidation-Tool-explanatory-note.pdf (Accessed May 11th, 2020).
Gebert, J., Rachor, I.-M., Streese-Kleeberg, J., and Pfeiffer, E.-M. (2016). Methane Oxidation in a Landfill Cover Soil under Conditions of Diffusive and Advective Flux, Assessed by In-Situ and Ex-Situ Methods. Cee 3 (2), 144–160. doi:10.2174/2212717803666160628093746
Gebert, J., Rachor, I., Gröngröft, A., and Pfeiffer, E.-M. (2011b). Temporal Variability of Soil Gas Composition in Landfill Covers. Waste Manag. 31, 935–945. doi:10.1016/j.wasman.2010.10.007
Gebert, J., Röwer, I. U., Scharff, H., Roncato, C. D. L., and Cabral, A. R. (2011c). Can Soil Gas Profiles Be Used to Assess Microbial CH4 Oxidation in Landfill Covers? Waste Manag. 31, 987–994. doi:10.1016/j.wasman.2010.10.008
Gebert, J., Singh, B. K., Pan, Y., and Bodrossy, L. (2009). Activity and Structure of Methanotrophic Communities in Landfill Cover Soils. Environ. Microbiol. Rep. 1, 414–423. doi:10.1111/j.1758-2229.2009.00061.x
Gebert, J., van Verseveld, C. J. W., Blom, H. P. W., and Heimovaara, T. J. (2019). “Effect of Compaction and Moisture on Gas Diffusivity and Conductivity of Soils for Use in Methane Oxidation Systems,” in Proceedings Sardinia 2019, 17th International Waste Management and Landfill Symposium (Italy: CISA).
Gebhardt, S., Fleige, H., and Horn, R. (2009). Effect of Compaction on Pore Functions of Soils in a Saalean Moraine Landscape in North Germany. Z. Pflanzenernähr. Bodenk. 172 (5), 688–695. doi:10.1002/jpln.200800073
Geck, C., Roewer, I. U., Kleinschmidt, C., and Gebert, J. (2016a). Design, Validation and Implementation of a Novel Accumulation Chamber System for the Quantification of CH4 and CO2 Emissions from Landfills. Technical report. Available at: https://www.afvalzorg.nl/content/uploads/2017/09/Novel-large-emission-measurement-chamber.pdf.
Geck, C., Scharff, H., Pfeiffer, E.-M., and Gebert, J. (2016b). Validation of a Simple Model to Predict the Performance of Methane Oxidation Systems, Using Field Data from a Large Scale Biocover Test Field. Waste Manag. 56, 280–289. doi:10.1016/j.wasman.2016.06.006
Giroud, J. P. (2010). “Development of Criteria for Geotextile and Granular Filters,” in 9th International Conference on Geosynthetics - Geosynthetics: Advanced Solutions for a Challenging World. Editors E. M. Palmeira, D. M. Vidal, A. S. J. F. Sayao, and M. Ehrlich (Guarujá, Brazil: ICG), 45–64. May 23, 2010 - May 27, 2010.
Haubrichs, R., and Widmann, R. (2006). Evaluation of Aerated Biofilter Systems for Microbial Methane Oxidation of Poor Landfill Gas. Waste Manag. 26, 408–416. doi:10.1016/j.wasman.2005.11.008
Héroux, M., Guy, C., and Millette, D. (2010). A Statistical Model for Landfill Surface Emissions. J. Air Waste Manag. Assoc. 60 (2), 219–228.
Heyer, K.-U., Hupe, K., and Stegmann, R. (2013). Methane Emissions from MBT Landfills. Waste Manag. 33, 1853–1860. doi:10.1016/j.wasman.2013.05.012
Hilger, H. A., Cranford, D. F., and Barlaz, M. A. (1999). Methane Oxidation and Microbial Exopolymer Production in Landfill Cover Soils. Soil Biol. Biochem. 32, 457–467.
Huber-Humer, M. (2004). Abatement of Landfill Methane Emissions by Microbial Oxidation in Biocovers Made of Compost. Vienna: Universität für Bodenkultur Wien, Institut für Abfallwirtschaft. [Dissertation].
Huber-Humer, M. (2005). “Extended Effects of Methane Oxidation and Their Relevance for Biocover Application in the Field,” in Proceedings of SARDINIA 2005 – International Waste Management and Landfill Symposium (Italy: CISA).
Huber-Humer, M., Gebert, J., and Hilger, H. (2008b). Biotic Systems to Mitigate Landfill Methane Emissions. Waste Manag. Res. 26, 33–46. doi:10.1177/0734242x07087977
Huber-Humer, M., Hrad, M., Schloffer, K., and Kammerer, G. (2017). “Implementation of Biowindows for Degasification of an Older Municipal Solid Waste Landfill after Removal of the Active Gas Extraction System,” in Proceedings of ‘SARDINIA 2017 – International Waste Management and Landfill Symposium’ (Italy: CISA).
Huber-Humer, M. (2004b). International Research into Landfill Gas Emissions and Mitigation Strategies - IWWG Working Group “CLEAR”. Waste Manag. 24, 425–427. doi:10.1016/j.wasman.2004.02.005
Huber-Humer, M., Lechner, P., and Görtler, N. (2008a). “Impact of Different Biocover Designs on Methane Mitigation,” in Management of Pollutant Emission from Landfills and Sludge. International Workshop on Management of Pollutant Emission from Landfills and Sludge, Kazimierz Dolny, Poland, 16 - 19 September 2006. Editors M. Pawlowska, and L. Pawlowski (London, UK: Taylor & Francis Group).
Huber-Humer, M., Röder, S., and Lechner, P. (2009). Approaches to Assess Biocover Performance on Landfills. Waste Manag. 29 (7), 2092–2104. doi:10.1016/j.wasman.2009.02.001
Huber-Humer, M., Tintner, J., Böhm, K., and Lechner, P. (2011). Scrutinizing Compost Properties and Their Impact on Methane Oxidation Efficiency. Waste Manag. 31, 871–883. doi:10.1016/j.wasman.2010.09.023
Humer, M., and Lechner, P. (2001a). “Design of a Landfill Cover Layer to Enhance Methane Oxidation, Results From a Two-Year Field Investigation,” in ‘‘SARDINIA 2001 – Eighth International Waste Management and Landfill Symposium” (Italy: CISA).
Humer, M., and Lechner, P. (2001b). Technischer Aufbau eines Methanoxidationssystems für Deponien. KA – Wasserwirtsch. Abwasser Abfall 48, 510–513.
Ishigaki, T., Yamada, M., Nagamori, M., Ono, Y., and Inoue, Y. (2005). Estimation of Methane Emission from Whole Waste Landfill Site Using Correlation between Flux and Ground Temperature. Environ. Geol. 48, 845–853. doi:10.1007/s00254-005-0008-0
Iversen, B. V., Schjønning, P., Poulsen, T. G., and Moldrup, P. (2001). In Situ, On-Site and Laboratory Measurements of Soil Air Permeability: Boundary Conditions and Measurement Scale. Soil Sci. 166 (2), 97–106. doi:10.1097/00010694-200102000-00003
Jugnia, L.-B., Cabral, A. R., and Greer, C. W. (2008). Biotic Methane Oxidation within an Instrumented Experimental Landfill Cover. Ecol. Eng. 33 (2), 102–109. doi:10.1016/j.ecoleng.2008.02.003
Kämpf, M., and Montenegro, H. (1997). On the Performance of Capillary Barriers as Landfill Cover. Hydrol. Earth Syst. Sci. 1, 925–930. doi:10.5194/hess-1-925-1997
King, F. H. (1905). Contributions to Our Knowledge of the Aeration of Soils. Science 22 (564), 495–499. doi:10.1126/science.22.564.495
Kjeldsen, P., and Scheutz, C. (2016). Etablering Og Monitering Af Biocoversystemer På Affaldsdeponerings-Anlæg [Establishment and Monitoring of Biocover Systems on Landfills] in Danish. Miljøprojekt Nr. 1817, 2016 Miljøstyrelsen. København: Danish Environmental Protection Agency.
Kjeldsen, P., and Scheutz, C. (2018). “Landfill Gas Management by Methane Oxidation,” in Solid Waste Landfilling - Concepts, Processes, Technology. Editors R. Cossu, and R. Stegmann (Elsevier), 477–497. doi:10.1016/b978-0-12-407721-8.00024-3
Klute, A. (1986). “Water Retention: Laboratory Methods,” in Methods of Soil Analysis, Part 1. Physical and Mineralogical Methods. Editor A. Klute (Madison, WI: American Society of Agronomy - Soil Science Society of America), 635–662.
LAGA Ad hoc AG Deponietechnik (2016a). Bundeseinheitlicher Qualitätsstandard 7-1. Rekultivierungsschichten in Deponieoberflächenabdichtungssystemen. Vom 04.02. Available at: https://www.laga-online.de/documents/bqs-7-1-reku-13-04-16_1507195527.pdf (Accessed May 11th, 2020).
LAGA Ad hoc AG Deponietechnik (2016b). Bundeseinheitlicher Qualitätsstandard 7-2. Wasserhaushaltsschichten in Deponieoberflächenabdichtungssystemen. Vom 20.10. Available at: https://www.laga-online.de/documents/bqs-7-2-whs-13-04-16__1507195533.pdf (Accessed May 11th, 2020).
LAGA Ad hoc AG Deponietechnik (2020). Bundeseinheitlicher Qualitätsstandard 7-3. Methanoxidationsschichten in Deponieoberflächenabdichtungssystemen. Vom 02.12. Available at: https://www.laga-online.de/documents/bqs-7-3-mos-20-12-02_1615544765.pdf (Accessed January 8, 2022).
Lagos, D. A., Héroux, M., Gosselin, R., and Cabral, A. R. (2017). Optimization of a Landfill Gas Collection Shutdown Based on an Adapted First-Order Decay Model. Waste Manag. 63, 238–245. doi:10.1016/j.wasman.2016.08.012
Laux, D. (2015). In situ Aerobisierung von Deponien zur Verkürzung der Nachsorgezeit am Beispiel der Deponie Konstanz-Dorfweiher (In situ aeration of landfills to shorten aftercare periods – case study landfill Konstanz-Dorfweiher), Doctoral thesis, Forschungs- und Entwicklungsinstitut für Industrie- und Siedlungswasserwirtschaft sowie Abfallwirtschaft e.V., Stuttgart, Stuttgarter Berichte zur Abfallwirtschaft, Band 121. München: DIV Deutscher Industrieverlag GmbH.
Leong, E. C., and Rahardjo, H. (1997). Review of Soil-Water Characteristic Curve Equations. J. Geotechnical Geoenvironmental Eng. 123 (12), 1106–1117. doi:10.1061/(asce)1090-0241(1997)123:12(1106)
Marinho, F. A. M., and Teixeira, P. F. (2013). “Air Permeability of Cover Soil from the Bandeirantes Landfill in São Paulo, Brazil,” in Advances in Unsaturated Soils. Editors C. M. Bernardo Caicedo, L. Hoyos, J. E. Colmenares, and I. R. Berdugo (Taylor & Francis Group), 253–258.
Martínez, I., Chervet, A., Weisskopf, P., Sturny, W., Rek, J., and Keller, T. (2016). Two Decades of No-Till in the Oberacker Long-Term Field Experiment: Part II, Soil Porosity and Gas Transport Parameters. Soil Tillage Res. 163, 130–140.
McCartney, J. S., and Zornberg, J. G. (2010b). Centrifuge Permeameter for Unsaturated Soils. II: Measurement of the Hydraulic Characteristics of an Unsaturated Clay. J. Geotech. Geoenviron. Eng. 136 (8), 1064–1076. doi:10.1061/(asce)gt.1943-5606.0000320
McCartney, J. S., and Zornberg, J. G. (2010a). Effects of Infiltration and Evaporation on Geosynthetic Capillary Barrier Performance. Can. Geotech. J. 47 (11), 1201–1213. doi:10.1139/T10-024
Mei, C., Yazdani, R., Han, B., Mostafid, M. E., Chanton, J., VanderGheynst, J., et al. (2015). Performance of Green Waste Biocovers for Enhancing Methane Oxidation. Waste Manag. 39, 205–215. doi:10.1016/j.wasman.2015.01.042
Melchior, S., Braun, G., and Miehlich, G. (1990). “The Use of Capillary Barriers in Landfill Covers,” in Contaminated Soil ’90: Third International KfK/TNO Conference on Contaminated Soil, 10–14 December 1990, Karlsruhe, Federal Republic of Germany. Editors F. Arendt, M. Hinsenveld, and W. J. Van Den Brink (Dordrecht: Springer Netherlands), 1211–1212. doi:10.1007/978-94-011-3270-1_278
Melse, R. W., and van der Werf, A. W. (2005). Biofiltration for Mitigation of Methane Emission from Animal Husbandry. Environ. Sci. Technol. 39, 5460–5468. doi:10.1021/es048048q
Messerklinger, S. (2013). “The Design of Filter Materials and Their Importance in Geotechnical Engineering,” in 18th International Conference on Soil Mechanics and Geotechnical Engineering (Paris: ISSMGE), 3313–3316.
Meza, N., Lammen, H., Cruz, C., Heimovaara, T. J., and Gebert, J. (2021). “Spatial Variability of Gas Composition in Landfills under In-Situ Aeration,” in Proceedings Sardinia 2021, 18th International Waste Management and Landfill Symposium.
Millington, R. J., and Quirk, J. P. (1961). Permeability of Porous Solids. Trans. Faraday Soc. 57, 1200. doi:10.1039/TF9615701200
Møldrup, P., Olesen, T., Komatsu, T., Schjønning, P., and Rolston, D. E. (2001). Tortuosity, Diffusivity, and Permeability in the Soil Liquid and Gaseous Phases. Soil Sci. Soc. Am. J. 65 (3), 613–623.
Møldrup, P., Olesen, T., Schjønning, P., Yamaguchi, T., and Rolston, D. E. (2000). Predicting the Gas Diffusion Coefficient in Undisturbed Soil from Soil Water Characteristics. Soil Sci. Soc. Am. J. 64, 1588–1594.
Moldrup, P., Olesen, T., Yoshikawa, S., Komatsu, T., and Rolston, D. E. (2005). Predictive-descriptive Models for Gas and Solute Diffusion Coefficients in Variably Saturated Porous Media Coupled to Pore-Size Distribution. Soil Sci. 170 (11), 854–866. doi:10.1097/01.ss.0000196768.44165.1f
Molins, S., and Mayer, K. U. (2007). Coupling between Geochemical Reactions and Multicomponent Gas and Solute Transport in Unsaturated Media: A Reactive Transport Modelling Study. Water Resour. Res. 43, W05435. doi:10.1029/2006wr005206
Molins, S., Mayer, K. U., Scheutz, C., and Kjeldsen, P. (2008). Transport and Reaction Processes Affecting the Attenuation of Landfill Gas in Cover Soils. J. Environ. Qual. 37, 459–468. doi:10.2134/jeq2007.0250
Mor, S., De Visscher, A., Ravindra, K., Dahiya, R. P., Chandra, A., and Van Cleemput, O. (2006). Induction of Enhanced Methane Oxidation in Compost: Temperature and Moisture Response. Waste Manag. 26, 381–388. doi:10.1016/j.wasman.2005.11.005
Morel-Seytoux, H. J. (1993). “Dynamic Perspective on the Capillary Barrier Effect at the Interface of an Upper Fine Layer with a Lower Coarse Layer,” in Engineering Hydrology, San Francisco, July 25-30 1993. Editor C. Y. Kuo (Reston (Virginia): ASCE), 467–472.
Ndanga, É. M., Bradley, R. L., and Cabral, A. R. (2015). Does Vegetation Affect the Methane Oxidation Efficiency of Passive Biosystems? Waste Manag. 38 (1), 240–249. doi:10.1016/j.wasman.2015.01.031
Ndanga, É. M., Cabral, A. R., Bradley, R., and Johnson, T. R. (2013). “Potential Effect of Vegetation on Methane Oxidation Efficiency of Biocovers: Laboratory and Field Experiment,” in Proceedings of the Fourteenth International Waste Management and Landfill Symposium. S. Margherita di Pula, Cagliari, Italy, 30 Sept-4 Oct 2013. Editor R. Cossu (Padova: CISA Publisher), 214.
Ndanga, É. M., Lopera, C. B., Bradley, R. L., and Cabral, A. R. (2016). Effects of Preconditioning the Rhizosphere of Different Plant Species on Biotic Methane Oxidation Kinetics. Waste Manag. 55, 313–320. doi:10.1016/j.wasman.2016.04.035
Nelson, B., Zytner, R. G., Kanmacher, Z., Yochim, A., Vaillancourt, R., Boss, B., et al. (2021). “Mitigating Fugitive Methane Emissions from Closed Landfills: A Pilot-Scale Field Study,” in Proceedings Sardinia 2021, 18th International Waste Management and Landfill Symposium.
Nordic Council of Ministers, (2009). Materials for Construction of Top Cover in Landfills. 978-92-893-1886-0.
NSW (2019). Handbook for the Design, Construction, Operation, Monitoring and Maintenance of a Passive Landfill Gas Drainage and Biofiltration System. Sydney: Department of Environment, Climate Change and Water NSW.
Nwachukwu, A. N., and Anonye, D. (2013). The Effect of Atmospheric Pressure on CH4 and CO2 Emission from a Closed Landfill Site in Manchester, UK. Environ. Monit. Assess. 185, 5729–5735. doi:10.1007/s10661-012-2979-0
Orlando, P., Mendes, R., and Marinho, F. (2014). “Soil Water Retention Curves of the Unsaturated Residual Soil from the Brazilian Coast,” in Geomechanics from Micro to Macro. Editors K. Soga, K. Kumar, G. Biscontin, and M. Kuo (Cambridge, UK, 1431–1436. doi:10.1201/b17395-259
ÖVA (Österreichischer Verein für Altlastenmanagement) (2008). Leitfaden Methanoxidationsschichten. Available at: http://www.altlastenmanagement.at/home/documents/ak_tl/Leitfaden_Methanox%20.docx.pdf (Accessed April 17, 2020).
ÖWAV (2008). ÖWAV-Positionspapier „Konzeptionelle Überlegungen zur Entlassung aus der Deponienachsorge”. Vienna: Unterausschuss Deponienachsorge of the Austrian Water and Waste Management Association.
Parent, S.-É., and Cabral, A. (2006). Design of Inclined Covers with Capillary Barrier Effect. Geotech. Geol. Eng. 24 (3), 689–710. doi:10.1007/s10706-005-3229-9
Parent, S.-É., and Cabral, A. R. (2005). Material Selection for the Design of Inclined Covers with Capillary Barrier Effect. Geofrontiers, 24–26. doi:10.1061/40789(168)25
Parker, T., Childs, A., Pointer, P., Baker, D., Johnson, T., and Wilson, W. (2013). “Lessons Learned from First Full Size Methane Oxidation Biofilter in the UK,” in Proceedings Sardinia 2013, 14th International Waste Management and Landfill Symposium S. Margherita di Pula, Cagliari, Italy, 30 September - 4 October 2013 (Italy: CISA).
Pawłowska, M., Rożej, A., and Stępniewski, W. (2011). The Effect of Bed Properties on Methane Removal in an Aerated Biofilter-Mmodel Studies. Waste Manag. 31, 903–913. doi:10.1016/j.wasman.2010.10.005
Pecorini, I., Bacchi, D., Frassinetti, L., and Olivieri, F. (2013). “Long Term Behaviour of Pilot Biocovers for Microbial Methane Oxidation and Odours Mitigation,” in Proceedings Sardinia 2013, 14th International Waste Management and Landfill Symposium S. Margherita di Pula, Cagliari, Italy, 30 September - 4 October 2013 (Italy: CISA).
Pedersen, G. B., Scheutz, C., and Kjeldsen, P. (2011). Availability and Properties of Materials for the Fakse Landfill Biocover. Waste Manag. 31, 884–894. doi:10.1016/j.wasman.2010.11.020
Penman, H. L. (1940). Gas and Vapour Movements in the Soil: I. The Diffusion of Vapours through Porous Solids. J. Agric. Sci. 30 (3), 437–462. doi:10.1017/s0021859600048164
Philopoulos, A., Felske, C., and McCartney, D. (2008). Field-scale Treatment of Landfill Gas with a Passive Methane Oxidizing Biofilter. J. Environ. Eng. Sci. 7 (5), 531–542. doi:10.1139/s08-021
Philopoulos, A., Ruck, J., McCartney, D., and Felske, C. (2009). A Laboratory-Scale Comparison of Compost and Sand-Compost-Perlite as Methane-Oxidizing Biofilter Media. Waste Manag. Res. 27, 138–146. doi:10.1177/0734242x08091555
Plessis, C., Strauss, J. M., Sebapalo, E. M. T., and Riedel, K.-H. J. (2003). Empirical Model for Methane Oxidation Using a Composted Pine Bark Biofilter. Fuel 82, 1359–1365. doi:10.1016/s0016-2361(03)00040-1
Poulsen, T. G., Christophersen, M., Moldrup, P., and Kjeldsen, P. (2013). Relating Landfill Gas Emissions to Atmospheric Pressure Using Numerical Modelling and State-Space Analysis. Waste Manag. Res. 21, 356–366. doi:10.1177/0734242X0302100408
Poulsen, T. G., and Blendstrup, H. (2008). Predicting Air Permeability in Porous Media with Variable Structure, Bulk Density, and Water Content. Vadose Zone J. 7 (4), 1269–1275. doi:10.2136/vzj2008.0035
Poulsen, T. G., and Møldrup, P. (2006). Evaluating Effects of Wind-Induced Pressure Fluctuations on Soil-Atmosphere Gas Exchange at a Landfill Using Stochastic Modelling. Waste Manag. Res. 24 (5), 473–481. doi:10.1177/0734242X06066363
Powelson, D. K., Chanton, J., Abichou, T., and Morales, J. (2006). Methane Oxidation in Water-Spreading and Compost Biofilters. Waste Manag. Res. 24, 528–536. doi:10.1177/0734242x06065704
Pratt, C., Walcroft, A. S., Tate, K. R., Ross, D. J., Roy, R., Reid, M. H., et al. (2012). Biofiltration of Methane Emissions from a Dairy Farm Effluent Pond. Agric. Ecosyst. Environ. 152, 33–39. doi:10.1016/j.agee.2012.02.011
Rachor, I., Gebert, J., Gröngröft, A., and Pfeiffer, E.-M. (2011). Assessment of the Methane Oxidation Capacity of Compacted Soils Intended for Use as Landfill Cover Materials. Waste Manag. 31, 833–842. doi:10.1016/j.wasman.2010.10.006
Rachor, I. M., Gebert, J., Gröngröft, A., and Pfeiffer, E.-M. (2013). Variability of Methane Emissions from an Old Landfill over Different Time-Scales. Eur. J. Soil Sci. 64, 16–26. doi:10.1111/ejss.12004
Reichenauer, T. G., Watzinger, A., Riesing, J., and Gerzabek, M. H. (2011). Impact of Different Plants on the Gas Profile of a Landfill Cover. Waste Manag. 31, 843–853. doi:10.1016/j.wasman.2010.08.027
Richard, G., Cousin, I., Sillon, J. F., Bruand, A., and Guérif, J. (2001). Effect of Compaction on the Porosity of a Silty Soil: Influence on Unsaturated Hydraulic Properties. Eur. J. Soil Sci. 52 (1), 49–58. doi:10.1046/j.1365-2389.2001.00357.x
Richter, J., and Grossgebauer, A. (1978). Untersuchungen zum Bodenlufthaushalt in einem Bodenbearbeitungsversuch. 2. Gasdiffusionskoeffizienten als Strukturmaße für Böden. Z. Pflanzenernaehr. Bodenk. 141, 181–202. doi:10.1002/jpln.19781410206
Richter, J., Kersebaum, K. C., and Willenbockel, I. (1991). Gaseous Diffusion Reflecting Soil Structure. Z. Pflanzenernaehr. Bodenk. 154, 13–19. doi:10.1002/jpln.19911540105
Ritzkowski, M., and Stegmann, R. (2012). Landfill Aeration Worldwide: Concepts, Indications and Findings. Waste Manag. 32, 1411–1419. doi:10.1016/j.wasman.2012.02.020
Roncato, C. D. L., and Cabral, A. R. (2012). Evaluation of Methane Oxidation Efficiency of Two Biocovers: Field and Laboratory Results. J. Environ. Eng. 138 (2), 164–173. doi:10.1061/(asce)ee.1943-7870.0000475
Röwer, I. U., Geck, C., Gebert, J., and Pfeiffer, E. M. (2011b). Spatial Variability of Soil Gas Concentration and Methane Oxidation Capacity in Landfill Covers. Waste Manag. 31, 926–934. doi:10.1016/j.wasman.2010.09.013
Röwer, I. U. (2014). Reduction of Methane Emissions from Landfills: Processes, Measures and Monitoring Strategies. Hambg. Bodenkd. Arb. 75.
Röwer, I. U., Scharff, H., Pfeiffer, E.-M., and Gebert, J. (2016a). Optimized Landfill Biocover for CH4 Oxidation I: Experimental Design and Oxidation Performance. Curr. Environ. Eng. 3 (2), 80–93.
Röwer, I. U., Streese-Kleeberg, J., Gröngröft, A., Melchior, S., Steinert, B., and Pfeiffer, E.-M. (2011a). “Design, Implementation and Operation of Soil-Based Methane Oxidation Windows for the Remediation of Gas Emission Hotspots in Landfill Cover Soils,” in Proceedings Sardinia 2011, Thirteenth International Waste Management and Landfill Symposium (Italy: CISA).
Röwer, I. U., Streese-Kleeberg, J., Scharff, H., Pfeiffer, E.-M., and Gebert, J. (2016b). Optimized Landfill Biocover for CH4 Oxidation II: Implications of Spatially Heterogeneous Fluxes for Monitoring and Emission Prediction. Curr. Environ. Eng. 3 (2), 94–106.
Samingan, A. S., Leong, E.-C., and Rahardjo, H. (2003). A Flexible Wall Permeameter for Measurements of Water and Air Coefficients of Permeability of Residual Soils. Can. Geotech. J. 40, 559–574. doi:10.1139/t03-015
Schaap, M. G., and Leij, F. J. (2000). Improved Prediction of Unsaturated Hydraulic Conductivity with the Mualem‐van Genuchten Model. Soil Sci. Soc. Am. J. 64 (3), 843–851. doi:10.2136/sssaj2000.643843x
Scharff, H., and Jacobs, J. (2006). Applying Guidance for Methane Emission Estimation for Landfills. Waste Manag. 26, 417–429. doi:10.1016/j.wasman.2005.11.015
Scheutz, C., Pedicone, A., Pedersen, G. B., and Kjeldsen, P. (2011c). Evaluation of Respiration in Compost Landfill Biocovers Intended for Methane Oxidation. Waste Manag. 31, 895–902. doi:10.1016/j.wasman.2010.11.019
Scheutz, C., Cassini, F., De Schoenmaeker, J., and Kjeldsen, P. (2017). Mitigation of Methane Emissions in a Pilot-Scale Biocover System at the AV Miljø Landfill, Denmark: 2. Methane Oxidation. Waste Manag. 63, 203–212. doi:10.1016/j.wasman.2017.01.012
Scheutz, C., Fredenslund, A. M., Chanton, J., Pedersen, G. B., and Kjeldsen, P. (2011b). Mitigation of Methane Emission from Fakse Landfill Using a Biowindow System. Waste Manag. 31, 1018–1028. doi:10.1016/j.wasman.2011.01.024
Scheutz, C., Kjeldsen, P., Bogner, J. E., De Visscher, A., Gebert, J., Hilger, H. A., et al. (2009a). Microbial Methane Oxidation Processes and Technologies for Mitigation of Landfill Gas Emissions. Waste Manag. Res. 27, 409–455. doi:10.1177/0734242x09339325
Scheutz, C., Pedersen, G. B., Costa, G., and Kjeldsen, P. (2009b). Biodegradation of Methane and Halocarbons in Simulated Landfill Biocover Systems Containing Compost Materials. J. Environ. Qual. 38, 1363–1371. doi:10.2134/jeq2008.0170
Scheutz, C., Pedersen, R. B., Petersen, P. H., Jørgensen, J. H. B., Ucendo, I. M. B., Mønster, J. G., et al. (2014). Mitigation of Methane Emission from an Old Unlined Landfill in Klintholm, Denmark Using a Passive Biocover System. Waste Manag. 34, 1179–1190. doi:10.1016/j.wasman.2014.03.015
Scheutz, C., Samuelsson, J., Fredenslund, A. M., and Kjeldsen, P. (2011a). Quantification of Multiple Methane Emission Sources at Landfills Using a Double Tracer Technique. Waste Manag. 31, 1009–1017. doi:10.1016/j.wasman.2011.01.015
Scheutz, C., Bogner, J., Chanton, J., Blake, D., Morcet, M., and Kjeldsen, P. (2003). Comparative Oxidation and Net Emissions of Methane and Selected Non-methane Organic Compounds in Landfill Cover Soils. Environ. Sci. Technol. 37, 5150–5158. doi:10.1021/es034016b
Simms, P. H., and Yanful, E. K. (2002). Predicting Soil-Water Characteristic Curves of Compacted Plastic Soils from Measured Pore-Size Distributions. Géotechnique 52 (4), 269–278. doi:10.1680/geot.2002.52.4.269
Simunek, J., and Van Genuchten, M. T. (2012). Hydrus: Model Use, Calibration. Trans. Am. Soc. Agric. Biol. Eng. 5 (4), 1261–1274.
Smith, K. A., Ball, T., Conen, F., Dobbie, K. E., and Rey, A. (2018). Exchange of Greenhouse Gases between Soil and Atmosphere: Interactions of Soil Physical Factors and Biological Processes. Eur. J. Soil Sci. 54, 779–791. doi:10.1111/ejss.12539
Spokas, K. A., and Bogner, J. E. (2011). Limits and Dynamics of Methane Oxidation in Landfill Cover Soils. Waste Manag. 31, 823–832. doi:10.1016/j.wasman.2009.12.018
Spokas, K., Bogner, J., and Chanton, J. (2011). A Process-Based Inventory Model for Landfill CH4 emissions Inclusive of Seasonal Soil Microclimate and CH4 Oxidation. J. Geophys. Res. 116, G04017. doi:10.1029/2011JG001741
Stegmann, R., Heyer, K.-U., Hupe, K., Siederer, H., and Willand, A. (2006). Deponienachsorge – Handlungsoptionen, Dauer, Kosten und quantitative Kriterien für die Entlassung aus der Nachsorge. Umweltforschungsplan des Bundesministeriums für Umwelt, Naturschutz und Reaktorsicherheit. Abfallwirtschaft, Förderkennzeichen (UFOPLAN) 204 34 327, im Auftrag des Umweltbundesamtes.
Stern, J. C., Chanton, J., Abichou, T., Powelson, D., Yuan, L., Escoriza, S., et al. (2007). Use of a Biologically Active Cover to Reduce Landfill Methane Emissions and Enhance Methane Oxidation. Waste Manag. 27, 1248–1258. doi:10.1016/j.wasman.2006.07.018
Streese, J., and Stegmann, R. (2003). Microbial Oxidation of Methane from Old Landfills in Biofilters. Waste Manag. 23 (7), 573–580. doi:10.1016/s0956-053x(03)00097-7
Sun, W., Wang, X., DeCarolis, J. F., and Barlaz, M. A. (2019). Evaluation of Optimal Model Parameters for Prediction of Methane Generation from Selected U.S. Landfills. Waste Manag. 91, 120–127. doi:10.1016/j.wasman.2019.05.004
Tanthachoon, N., Chiemchaisri, C., Chiemchaisri, W., Tudsri, S., and Kumar, S. (2008). Methane Oxidation in Compost-Based Landfill Cover with Vegetation during Wet and Dry Conditions in the Tropics. J. Air & Waste Manag. Assoc. 58, 603–612. doi:10.3155/1047-3289.58.5.603
Tecle, D., Lee, J., and Hasan, S. (2008). Quantitative Analysis of Physical and Geotechnical Factors Affecting Methane Emission in Municipal Solid Waste Landfill. Environ. Geol. 56 (6), 1135–1143. doi:10.1007/s00254-008-1214-3
Troeh, F. R., Jabro, J. D., and Kirkham, D. (1982). Gaseous Diffusion Equations for Porous Materials. Geoderma 27 (3), 239–253. doi:10.1016/0016-7061(82)90033-7
Tuli, A., Hopmans, J. W., Rolston, D. E., and Moldrup, P. (2005). Comparison of Air and Water Permeability between Disturbed and Undisturbed Soils. Soil Sci. Soc. Am. J. 69, 1361–1371. doi:10.2136/sssaj2004.0332
U.S._Corps_of_Engineers (1986). Seepage Analysis and Control for Dams. Washington: U.S. Corps of Engineers.
van Genuchten, M. T. (1980). A Closed-form Equation for Predicting the Hydraulic Conductivity of Unsaturated Soils. Soil Sci. Soc. Am. J. 44, 892–898. doi:10.2136/sssaj1980.03615995004400050002x
van Verseveld, C. J. W., and Gebert, J. (2020). Effect of Compaction and Soil Moisture on the Effective Permeability of Sands for Use in Methane Oxidation Systems. Waste Manag. 107, 44–53. doi:10.1016/j.wasman.2020.03.038
Vanapalli, S. K., Nicotera, M. V., and Sharma, R. S. (2008). Axis Translation and Negative Water Column Techniques for Suction Control. Geotech. Geol. Eng. 26, 645–660. doi:10.1007/s10706-008-9206-3
Vijay, V., Shreedhar, S., Adlak, K., Payyanad, S., Sreedharan, V., Gopi, G., et al. (2021). Review of Large-Scale Biochar Field-Trials for Soil Amendment and the Observed Influences on Crop Yield Variations. Front. Energy Res. 9, 710766. doi:10.3389/fenrg.2021.710766
von Der Hude, N., Melchior, S., and Möckel, S. (1999). “Construction of a Capillary Barrier in the Cover of the Breinermoor Landfill,” in Seventh International Waste Management and Landfill Symposium, Sta Margarita di Pula. Editors T. H. Christenesen, R. Cossu, and R. Stegmann (Italy: CISA), 393–402.
Wawra, B., and Holfelder, T. (2003). “Development of a Landfill Cover with Capillary Barrier for Methane Oxidation - the Capillary Barrier as Gas Distribution Layer,” in Proceedings Sardinia 2003. Ninth International Waste Management and Landfill Symposium (Italy: CISA).
Werner, D., Grathwohl, P., and Hohener, P. (2004). Review of Field Methods for the Determination of the Tortuosity and Effective Gas-phase Diffusivity in the Vadose Zone. Vadose Zone J. 3, 1240–1248. doi:10.2113/3.4.1240
Wickramarachchi, P., Kawamoto, K., Hamamoto, S., Nagamori, M., Moldrup, P., and Komatsu, T. (2011). Effects of Dry Bulk Density and Particle Size Fraction on Gas Transport Parameters in Variably Saturated Landfill Cover Soil. Waste Manag. 31 (12), 2464–2472. doi:10.1016/j.wasman.2011.07.008
Wildenschild, D., Jensen, K. H., Hollenbeck, K. J., Sonnenborg, T., Butts, M. B., Illangasekare, T. H., et al. (1997). A Two-Stage Procedure for Determining Unsaturated Hydraulic Characteristics Using a Syringe Pump and Outflow Observations. Soil Sci. Soc. Am. J. 61 (2), 347–359. doi:10.2136/sssaj1997.03615995006100020002x
Williams, L., Hoyt, D., Dwyer, S., Hargreaves, G., and Zornberg, J. G. (2011). “Design Criteria and Construction of a Capillary Barrier Cover System: The Rocky Mountain Arsenal Experience,” in Proceedings of the GeoFrontiers 2011 Conference, Dallas, Texas, March 13-16 (Reston (Virginia): Geo-Institute of ASCE), 996–1005. doi:10.1061/41165(397)102
Wilshusen, J. H., Hettiaratchi, J. P. A., and Stein, V. B. (2004). Long-term Behavior of Passively Aerated Compost Methanotrophic Biofilter Columns. Waste Manag. 24, 643–653. doi:10.1016/j.wasman.2003.12.006
Xu, L., Lin, X., Amen, J., Welding, K., and McDermitt, D. (2014). Impact of Changes in Barometric Pressure on Landfill Methane Emission. Glob. Biogeochem. Cycles 28, 679–695. doi:10.1002/2013GB004571
Yargicoglu, E. N., and Reddy, K. R. (2018). Biochar-amended Soil Cover for Microbial Methane Oxidation: Effect of Biochar Amendment Ratio and Cover Profile. J. Geotech. Geoenviron. Eng. 144 (3), 04017123. doi:10.1061/(ASCE)GT.1943-5606.0001845
Yargicoglu, E. N., and Reddy, K. R. (2017a). Effects of Biochar and Wood Pellets Amendments Added to Landfill Cover Soil on Microbial Methane Oxidation: A Laboratory Column Study. J. Environ. Manag. 193, 19–31. doi:10.1016/j.jenvman.2017.01.068
Yargicoglu, E. N., and Reddy, K. R. (2017b). Microbial Abundance and Activity in Biochar-Amended Landfill Cover Soils: Evidence from Large-Scale Column and Field Experiments. J. Environ. Eng. 143 (9), 04017058. doi:10.1061/(ASCE)EE.1943-7870.0001254
Yi, S., Chang, N. Y., and Imhoff, P. T. (2020). Predicting Water Retention of Biochar-Amended Soil from Independent Measurements of Biochar and Soil Properties. Adv. Water Resour. 142, 103638. doi:10.1016/j.advwatres.2020.103638
Zeiss, C. A. (2006). Accelerated Methane Oxidation Cover System to Reduce Greenhouse Gas Emissions from MSW Landfills in Cold, Semi-arid Regions. Water Air Soil Pollut. 176, 285–306. doi:10.1007/s11270-006-9169-z
Zhang, J., Li, Z., Ning, T., and Gu, S. (2011). Methane Uptake in Salt-Affected Soils Shows Low Sensitivity to Salt Addition. Soil Biol. Biochem. 43, 1434–1439. doi:10.1016/j.soilbio.2011.03.010
Keywords: Methane oxidation, biofilter, biowindow, biocover, design, consruction, monitoring
Citation: Gebert J, Huber-Humer M and Cabral AR (2022) Design of Microbial Methane Oxidation Systems for Landfills. Front. Environ. Sci. 10:907562. doi: 10.3389/fenvs.2022.907562
Received: 29 March 2022; Accepted: 09 May 2022;
Published: 01 September 2022.
Edited by:
Keng Yuen Foo, University of Science Malaysia, MalaysiaReviewed by:
Kurt A. Spokas, United States Department of Agriculture, United StatesCopyright © 2022 Gebert, Huber-Humer and Cabral. This is an open-access article distributed under the terms of the Creative Commons Attribution License (CC BY). The use, distribution or reproduction in other forums is permitted, provided the original author(s) and the copyright owner(s) are credited and that the original publication in this journal is cited, in accordance with accepted academic practice. No use, distribution or reproduction is permitted which does not comply with these terms.
*Correspondence: Julia Gebert, ai5nZWJlcnRAdHVkZWxmdC5ubA==
Disclaimer: All claims expressed in this article are solely those of the authors and do not necessarily represent those of their affiliated organizations, or those of the publisher, the editors and the reviewers. Any product that may be evaluated in this article or claim that may be made by its manufacturer is not guaranteed or endorsed by the publisher.
Research integrity at Frontiers
Learn more about the work of our research integrity team to safeguard the quality of each article we publish.