- 1College of Ecology and Environment, Xinjiang University, Urumqi, China
- 2Key Laboratory of Oasis Ecology Ministry of Education, Xinjiang University, Urumqi, China
- 3Xinjiang Jinghe Observation and Research Station of Temperate Desert Ecosystem, Ministry of Education, Jinghe, Xinjiang, China
Salt crust is a soil layer formed by the cementation of soluble salt on the surface or inside of soil with soil particles. The salt crust can alter the soil’s ability to resist wind erosion. To explore the characteristics of different types of salt crust, six kinds of salt (CaCl2, KCl, Na2SO4, NaCl, Na2CO3, and NaHCO3) were selected and six salt contents (0.2, 0.5, 1, 2, 3, and 4%) were set up to conduct the experiment of a sandy soil crust. The experiment compares the characteristics of crust appearance, compressive strength, and toughness of six selected kinds of salt crust. The results showed that Na2SO4 formed the subflorescence, while CaCl2, KCl, NaCl, Na2CO3, and NaHCO3 formed the efflorescences. The compressive strength and toughness of Na2SO4 salt crust initially increased and then decreased as the salt content increased, and both the strength and toughness reached the maximum when the salt content was 3%. At first, the compressive strength of NaCl crusts increased and then stabilized as a result of the increase in salt content. The toughness of the NaCl salt crust experienced an initial increase and then a decrease, reaching the maximum when the salt content was 3%. The compressive strength and toughness of the salt crusts of CaCl2 and KCl increased as the salt content increased. There was a slight increase in the compressive strength of the crusts formed by Na2CO3 and NaHCO3, with hardly any noticeable change even as the salt content increased, and the maximum compressive strength was only 0.03 and 0.40 MPa, respectively. The crusts of Na2CO3 and NaHCO3 were susceptible to wind erosion, while the crusts of KCl, CaCl2, NaCl, and Na2SO4, having high compressive strength and crust toughness, were capable of resisting wind erosion.
Introduction
Soil wind erosion is an important component of soil desertification, and its control is important to sandy soil management (Yi et al., 2006). Some kinds of soil crusts can compact the soil structure and also improve the resistance of the soil to wind erosion (Li and Shen, 2006). There are two types of soil crust: biological crust and physical crust. A biological crust is a layer formed by mosses, algae, microorganisms, etc., and their secretions cement soil particles. Biocrust formation requires a long period, but it has high resistance to wind erosion. Physical crusts are formed when fine particles clog the soil surface pores during rainfall splashing or when fine particles adhere to large soil particles during soil water evaporation. Physical crusts are formed in a short time and are much less resistant to wind erosion than biological crusts (Langston and Neuman, 2005; Li and Shen, 2006). Salt crust is a special type of physical crust, which is a layer of soil crust formed when soluble salts in the soil cement with surface particles or internal particles (Li, 2018). The presence of salt crusts alters the structure of the soil and affects its resistance to wind erosion (Ji, 2001). In addition, salt crusts can increase the water content in the soil beneath the crusts (Zhang et al., 2010), thereby causing a further increase in the binding capacity of soil particles, which in turn improves the resistance of soil to wind erosion (Chepil, 1951; Yi et al., 2006). Where vegetation is scarce, the presence of salt crusts is of great importance to soil resistance to wind erosion.
The formation of salt crust is influenced by a variety of factors. When the brine solution evaporates in the porous medium, as evaporation proceeds, salt solubility increases and reaches the salt saturation concentration, resulting in precipitation of salt crystals. The initial salt concentration of the brine solution, temperature, and humidity affect the formation of salt crusts (Clercq et al., 2013; Rad et al., 2015; Menéndez and Petráňová, 2016; Shokri-kuehni et al., 2017a; Shokri-kuehni et al., 2017b). The higher the initial concentration of the brine solution, the higher the evaporation temperature of the evaporation surface, and the lower the humidity of air, the earlier the formation of salt crystals, and the easier it is to form salt crusts. The type of salt crust formed by different salts also varies. Na2SO4 forms the subflorescence at room temperature, with salt crystals crystallizing in the pores of the surface soil layer, while NaCl forms the efflorescences, with salt crystals aggregating on the soil surface (Benavente et al., 2015; Lindström et al., 2015; Desarnaud et al., 2016). The efflorescences of NaCl have two forms, “crusty” and “patchy”. When the particle size of the medium material is larger, when the medium has more water-repellent particles, or the medium particles have irregular angles, it tends to form a discrete crust, which is the “patchy” crust. When the particle size of the medium material is fine, it forms a continuity crust, that is, a “crusty” crust (Eloukabi et al., 2013; Rad and Shokri, 2014). In further studies on salt crust, it was found that continuity crusts had better resistance to wind erosion and reduced water evaporation from the soil (Nield et al., 2016a; Zhang et al., 2019).
To investigate the characteristics of different types of salt crust, six salts, CaCl2, KCl, Na2SO4, NaCl, Na2CO3, and NaHCO3, were selected in this study to compare the crust appearance, compressive strength, and crust toughness formed by the six salts. The aim was to compare the crust characteristics formed by the six salts to provide theoretical support in the practical application of salt crust.
Materials and methods
Materials
The sandy soil used in the experiment was obtained from the dunes at the Gurbantunggut Desert in Xinjiang, China. The distribution of the particle size of the natural sandy soils is shown in Table 1. To ensure the uniformity and representativeness of the sandy soil, we selected soil with a particle size range of 100–200 μm for the experiment. To remove the interference of soluble salts in the soil, the soil was cleaned with deionized water, and the cleaned sand was placed in an oven at 105°C for drying. The salts used for the experiment were CaCl2, KCl, Na2SO4, NaCl, Na2CO3, and NaHCO3 (purity ≥99.99%). Six gradients of salt content were set for each salt, 0.2, 0.5, 1, 2, 3, and 4% (the value of soluble salt mass as a percentage of the soil mass). The solvent was deionized water.
Crust experiment
For the experiment, the mass of the soil was fixed at 750 g, and the corresponding mass of salt at all salt content is shown in Table 2. 100 ml of deionized water was added, stirred well, and then poured into a mold (a cylindrical plastic cup of 3.5 cm height and 4 cm radius). The surface of the mold was then smoothed, and the bulk density is 1.43 g cm−3, after which the samples were placed in an oven at 75°C until all the water in the samples evaporated and the sample mass reached constant weight. Ten replicates were carried out for all salt content.
Data acquisition and processing
The dried samples were cooled to room temperature (the average temperature is 24.41°C and the average humidity is 20.85% indoor). Photographs were taken from above and on the side of the samples to observe the apparent morphological characteristics of the crusts. The process of taking pictures lasts about 2–3 min, and then the compressive strength was immediately measured. The crust compressive strength was tested with a digital electronic manometer (HANDPI, HP-1000N, accuracy: 0.1 N, range: 0–1000 N). The manometer was fixed on a holder, and the speed of the manometer probe was set to 1 mm s−1 and the diameter of the manometer probe is 10 mm. The stress-time curves were automatically recorded by the manometer connected to a computer. The compressive strength of a sample was the value of the maximum compressive strength on the stress-time curve of the sample. The average and standard deviation of the compressive strength of each salt content were calculated, and the average of the compressive strength was represented as the compressive strength of the samples at the same salt content. The energy absorbed by the crust per unit area is the toughness of the crust, that is, the ratio between the work done by the manometer probe and the area of the manometer probe when the compressive strength of the crust reaches its maximum.
Results
Characteristics of salt crust appearance
Figure 1 shows the appearance of the crusts of the six salts at different salt contents. As can be seen from Figure 1, there were differences in the appearance of crusts formed by the different salts. When the salt content was 0.2%, there were no macroscopic salt crystals on the surface of all six salt crusts, and the color of the crusts was brownish-red. There were a few cracks at the surface of Na2CO3 and NaHCO3 crusts, while cracks were not seen on other salt crusts. When the salt content was 1%, macroscopic salt crystals appeared on the surface of the salt crusts, except for Na2SO4. The salt crusts formed by Na2CO3 had the largest area of salt crystals on the surface and had some fine polygonal fractures. When the salt content was 4%, the salt crystals did not appear on the surface of Na2SO4 salt crusts still, and the salt crystals on the surface of the other five salt crusts increased noticeably. Polygonal fractures existed on the surface of both salt crusts of Na2CO3 and NaHCO3, and an obvious expansion could be seen in the crust formed by Na2CO3.
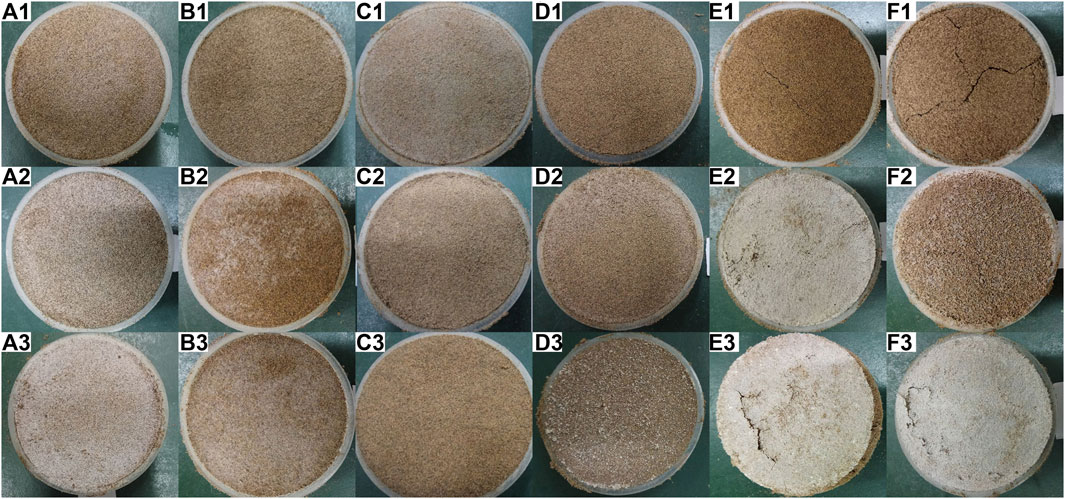
FIGURE 1. Apparent morphology of salt crusts (A, B, C, D, E, and F represent CaCl2, KCl, Na2SO4, NaCl, Na2CO3, and NaHCO3 six kinds of salt, respectively; 1, 2, and 3 indicate salt content of 0.2, 1, and 4% respectively).
The side view photographs of Na2CO3 and NaHCO3 crusts are shown in Figure 2. When the salt content was 0.2%, the surface of the crusts formed by Na2CO3 and NaHCO3 had cracked but did not exhibit swelling. When the salt content was 1%, obvious swelling could be seen in the Na2CO3 samples, and swelling was seen only in the surface edge area in the NaHCO3 samples. When the salt content was 4%, the swelling phenomenon inside both samples was further intensified, the crusts formed by Na2CO3 had separated from the soil thoroughly, and the internal loose sand under the crust could be seen. The crusts formed by NaHCO3 were lifted noticeably from the sample.
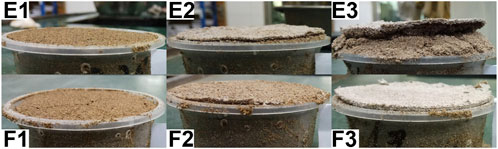
FIGURE 2. Side view photographs of Na2CO3 and NaHCO3 salt crusts (E, F represent Na2CO3 and NaHCO3 respectively; 1, 2, and 3 indicate salt content of 0.2, 1, and 4% respectively).
Compressive strength of salt crusts
Figure 3 showed the variation of compressive strength with salt content for the six salt crusts. The compressive strength of different salt crusts varied with the salt content variation. With the increase in the salt content, the compressive strength of the Na2SO4 crust increased first and then decreased, reaching a maximum of 5.12 MPa at a salt content of 3%. The compressive strength of the NaCl crust increased first and then remained stable, and the compressive strength of the crust of NaCl reached 3.59 MPa when the salt content was 3% and 3.76 MPa when the salt content was 4%, which was only an increase of 4.7%. The compressive strength of KCl and CaCl2 crusts increased almost exponentially with the increase of the salt content. The compressive strength of NaHCO3 crusts increased slightly but then decreases, reaching a maximum at 0.5% salt content, but its compressive strength was only 0.40 MPa. The compressive strength of Na2CO3 crusts was almost stable, and the maximum was only 0.03 MPa.
When the salt content was ≤3%, the compressive strength of the crust formed by Na2SO4 was significantly higher than that of the other five salt crusts (p < 0.05), and the compressive strength reached 1.15 MPa when the salt content was 0.2%. When the salt content was >3%, the compressive strength of the crusts formed by CaCl2 and KCl salts was higher than that of the crusts formed by Na2SO4. The compressive strength of CaCl2 and KCl salt crusts reached 5.36 and 4.98 MPa at 4% salt content, respectively, while the compressive strength of Na2SO4 and NaCl was slightly less, 4.53 and 3.76 MPa, respectively.
Stress-time curve and toughness of crusts
Figure 4 showed the stress-time curves of four salts, Na2SO4, NaCl, CaCl2, and KCl at different salt contents (the stress-time curve of Na2CO3 and NaHCO3 crusts was not considered because the compressive strength of their crusts was very small). The energy absorbed by the crust per unit area is the toughness of the crust, and Figure 5 is the graph of the crust toughness of four salts. From Figure 4 and Figure 5, it could be seen that the crust compressive strength and crust toughness did not always have a positive correlation, and there were differences in the toughness of different salt crusts. The toughness of the Na2SO4 crusts and the NaCl crusts all increased and then decreased. The crust toughness of KCl and CaCl2 increased gradually as the salt content increased, with the crust toughness of KCl being slightly higher than that of CaCl2. When the salt content is ≤3%, the crust toughness of Na2SO4 was higher than that of CaCl2, KCl, and NaCl, while in the range of the salt content between 2 and 3%, the difference between the crust toughness of Na2SO4 and NaCl was not obvious. When the salt content is 4%, the crust toughness of CaCl2 and KCl was greater than that of Na2SO4 and NaCl.
Discussion
Salt crust surface characteristics
Under these experimental conditions, Na2SO4 formed the subflorescence, with most of the salt crystals distributed inside the soil and fewer salt crystals distributed on the surface of the soil, which was the same as the results of the previous studies (Espinosa et al., 2007; Shahidzdeh-bonn et al., 2010; Gentilini et al., 2012; Li et al., 2021). NaCl, CaCl2, KCl, Na2CO3, and NaHCO3 formed the efflorescences. As the salt content increased, the crystals of salts gradually increased on the surface of a sample. The crusts formed by Na2CO3 and NaHCO3 produced the phenomena of cracks and swelling on the samples, which was related to the crystallization process of Na2CO3 and NaHCO3. Both solutions could produce hydrate crystals. The hydrate crystals were all bigger than the crystals of Na2CO3 and NaHCO3. The saturated Na2CO3 solution produced Na2CO3-10H2O crystals, and the Na2CO3-10H2O crystals would increase in volume, and the formation of hydrate crystals in the soil would produce stress to the surrounding sand grains, causing the soil to swell. The crystals of Na2CO3-10H2O would lose water at temperatures higher than 33°C to form anhydrous Na2CO3 crystals. The crystals of anhydrous Na2CO3 decreased in volume. Crystal expansion and shrinkage resulted in soil expansion and cracking. The Na2CO3-10H2O crystal layer crystallized on the surface of the soil lost water and then decreased in volume, resulting in the surface crust being cracked (Rijniers et al., 2005; Liu, 2016). The NaHCO3 solution had a similar phenomenon to the Na2CO3 solution, but the volume expansion of NaHCO3-10H2O was smaller than that of the Na2CO3-10H2O, so its cracks and expansion were smaller than those of Na2CO3. The swelling phenomenon of Na2CO3 and NaHCO3 salt crusts was related to salt content. The greater the salt content, the more obvious the salt swelling phenomenon, which could be verified from Figure 2.
Compressive strength
During the evaporation of soil water, the brine in the fine pores evaporates and the salt crystallizes as the salt solution reaches saturation solubility (Rad et al., 2013; Li et al., 2021). In this experiment, the crystals of Na2SO4 formed the subflorescence, and most of the salt crystals crystallized in the internal pores of the soil. When the Na2SO4 content was ≤3%, with the increase in salt content, the number of salt crystals crystallized in the pores gradually increased, the cementation between salt crystals and soil particles became strong, and the compressive strength of the salt crust increased. With a further increase in salt content (>3%), the crystals gradually filled the soil pores and generated salt swelling pressure, which damaged the soil structure, and the compressive strength of the salt crust formed by Na2SO4 decreased obviously. In these experiments, NaCl, KCl, and CaCl2 formed the efflorescences, with salt crystallization occurring mainly on the soil surface. As evaporation proceeded, the salts gradually accumulated on the soil surface, and the thickness of the salt crust on the surface increased; thus, the increase in salt content only increased the number of the crystals on the surface of the soil and did not affect the internal structure of the soil. Therefore, the compressive strength of these three salts gradually increased with the increase of salt content. The compressive strength of NaCl crusts remained stable at salt content >3%, which might be due to the detachment of the salt crust of NaCl from the soil surface, which hindered water evaporation and inhibited the movement of salt ions to the soil surface (Nachshon et al., 2018). The crystallization process of Na2CO3 and NaHCO3 salts resulted in severe salt swelling, which led to salt crust bulging. The compressive strength of the two salt crusts was extremely low, with the maximum compressive strength of the crusts of Na2CO3 being only 0.03 MPa and that of NaHCO3 being 0.40 MPa.
Implications for wind erosion resistance
The crust strength and crust toughness of the crusts affect the wind erosion resistance of the crusts. The greater the crust strength and crust toughness, the greater the potential resistance of the crust to wind erosion, and the more potentially erosive events the soil crust can withstand (Nickling and Ecclesstone, 1981; Nield et al., 2016b). This study compared the wind erosion resistance of six salt crusts by three indicators: crust appearance, crust compressive strength, and crust toughness. The surface of the crust formed by Na2CO3 and NaHCO3 salts had obvious swelling, exposing the loose soil beneath the crust (as shown in Figure 2), and the compressive strength of the crust was extremely low. The compressive strength of the crust formed by the two salts was far from the sand fixation strength of 1 MPa (Han et al., 2000), and the crusts formed by the two salts would be susceptible to wind erosion. The crusts formed by Na2SO4, NaCl, KCl, and CaCl2 were flat relatively, the salt expansion was not observed, and the compressive strength of the salt crust was stronger than that of other salt crusts. These salt crusts could be used for increasing soil resistance. The compressive strength and crust toughness of the Na2SO4 crust both increased and then decreased with the increase of the salt content. The compressive strength could reach 1.15 MPa at the salt content of 0.2%, and the compressive strength and crust toughness reached the maximum at the salt content of 3%, with the best resistance to wind erosion. There are two stable crystalline phases during Na2SO4 solution crystallization, decahydrate (Na2SO4-10H2O) and anhydrous state (Na2SO4); when the temperature is high, anhydrous Na2SO4 is generated, and as the humidity in the air increases, anhydrous Na2SO4 is transformed into Na2SO4-10H2O. The solubility of Na2SO4-10H2O is less than that of anhydrous Na2SO4, resulting in super-saturation of Na2SO4-10H2O, generating a crystallization pressure and causing damage to the medium material (Steiger and Asmussen, 2008; Saidov et al., 2015). Thus, the optimal salt content of the Na2SO4 crust for wind erosion resistance under field conditions still needs to be further investigated. The compressive strength of the NaCl crust increased first and then stayed stable with the increase of salt content, reaching the maximum of 3.76 MPa at the salt content of 4%. The toughness of NaCl crust increased first too and then decreased with the increase in salt content. The toughness reached its maximum at the salt content of 3%, and its compressive strength also reached 3.59 MPa, which was slightly lesser than that of 4% (about 0.16 MPa). Thus, for NaCl, the best potential resistance to wind erosion of the salt crust was the salt content of 3%. The compressive strength and crust toughness of KCl and CaCl2 crusts increased gradually with the increase in salt content, and the compressive strength already reached 1.94 and 1.60 MPa, respectively, at 2% salt content, both having wind erosion resistance. For the crusts formed by Na2SO4, NaCl, KCl, and CaCl2, the compressive strength and toughness of the crust formed by the Na2SO4 are higher than those of the remaining salts, and the wind erosion resistance is the best when the salt content is ≤3%; the crust formed by CaCl2 has the best wind erosion resistance when the salt content is 4%, its compressive strength is the greatest, and the crust toughness is also better.
Conclusion
In this article, Na2SO4, NaCl, CaCl2, KCl, Na2CO3, and NaHCO3 were selected for salt crusting experiments to investigate crust properties related to resistance to wind erosion, and the following was concluded.
1) Na2SO4 formed a subflorescence, and the appearance of the salt crust was not affected by the increase in the salt content. CaCl2, KCl, NaCl, NaHCO3, and Na2CO3 formed efflorescences. The area of crystals on the surface of the soil increased gradually with the increasing salt content. Soil expansion occurred with Na2CO3 and NaHCO3 crusts as salt content increased.
2) The compressive strength of Na2SO4 crusts increased first and then decreased with increasing salt content, reaching the maximum at 3% salt content. The crust compressive strength of CaCl2 and KCl increased continuously with increasing salt content. The compressive strength of the NaCl crust increased first and then stabilized at the salt content of 3% with increasing salt content. The crust compressive strength of Na2CO3 crusts was low, with a maximum of only 0.03 MPa. The compressive strength of NaHCO3 crusts was low too, and the maximum was only 0.40 MPa.
3) The crust toughness of both Na2SO4 and NaCl increased and then decreased with increasing salt content, and the crust toughness reached the maximum at 3% salt content. While the crust toughness of both KCl and CaCl2 gradually increased with increasing salt content.
The salt crust of Na2CO3 and NaHCO3 swelled, destroying the internal structure of the soil, and the compressive strength of the crusts was low, which is not suitable for protecting wind-sand soils. The salt crust formed by Na2SO4, NaCl, KCl, and CaCl2 had higher crust strength and better crust toughness, having the power to resist wind erosion. Under these experimental conditions, the optimum salt contents of the four salts were 3, 3, 4, and 4%, respectively. The crust formed by Na2SO4 has the best wind erosion resistance when the salt content is ≤3%, and the crust formed by CaCl2 has the best wind erosion resistance when the salt content is 4%.
Data availability statement
The original contributions presented in the study are included in the article/Supplementary Material; further inquiries can be directed to the corresponding author.
Author contributions
Conceptualization: CL; investigation, CL and ZL; resources, CL; data curation, BX; writing—original draft preparation, BX; writing—review and editing, BX; visualization, BX; supervision, CL; project administration, CL; funding acquisition, CL. All authors have read and agreed to the published version of the manuscript.
Funding
This research was funded by the National Natural Science Foundation of China (Nos. 41761005 and 41301286).
Conflict of interest
The authors declare that the research was conducted in the absence of any commercial or financial relationships that could be construed as a potential conflict of interest.
Publisher’s Note
All claims expressed in this article are solely those of the authors and do not necessarily represent those of their affiliated organizations, or those of the publisher, the editors, and the reviewers. Any product that may be evaluated in this article, or claim that may be made by its manufacturer, is not guaranteed or endorsed by the publisher.
References
Benavente, D., Brimblecombe, P., and Grossi, C. M. (2015). Thermodynamic calculations for the salt crystallisation damage in porous built heritage using PHREEQC. Environ. Earth Sci. 74 (3), 2297–2313. doi:10.1007/s12665-015-4221-1
Chepil, W. S. (1951). Properties of soil which influence wind erosion: IV. State of dry aggregate structure. Soil Sci. 72 (5), 387–402. doi:10.1097/00010694-195111000-00007
Clercq, H. D., Jovanović, M., Linnow, K., and Steiger, M. (2013). Performance of limestones laden with mixed salt solutions of Na2SO4–NaNO3 and Na2SO4–K2SO4. Environ. Earth Sci. 69 (5), 1751–1761. doi:10.1007/s12665-012-2017-0
Desarnaud, J., Bonn, D., and Shahidzadeh, N. (2016). The Pressure induced by salt crystallization in confinement. Sci. Rep. 6, 30856. doi:10.1038/srep30856
Eloukabi, H., Sghaier, N., Nasrallah, S. B., and Prat, M. (2013). Experimental study of the effect of sodium chloride on drying of porous media: The crusty–patchy efflorescence transition. Int. J. Heat. Mass Transf. 56 (1–2), 80–93. doi:10.1016/j.ijheatmasstransfer.2012.09.045
Espinosa, R., Franke, L., and Deckelmann, G. (2007). Predicting efflorescence and subflorescences of salts. MRS Proc. 1047, 105. doi:10.1557/PROC-1047-Y04-03
Gentilini, C., Franzoni, E., Bandini, S., and Nobile, L. (2012). Effect of salt crystallisation on the shear behaviour of masonry walls: An experimental study. Constr. Build. Mater. 37, 181–189. doi:10.1016/j.conbuildmat.2012.07.086
Han, Z. W., Hu, Y. D., Chen, G. T., Yao, Z. Y., and Shao, G. S. (2000). The suitability of chemical engineering stabilization in controlling aeolian hazard along the highway in tarim basin. Environ. Sci. 05, 86–88. doi:10.13227/j.hjkx.2000.05.021
Ji, F. (2001). Preliminary study on desert types and their anti-wind erosion characteristics in tarim basin. J. Soil Water Conservation 01, 16–18+53. doi:10.13870/j.cnki.stbcxb.2001.01.005
Langston, G., and Neuman, C. M. (2005). An experimental study on the susceptibility of crusted surfaces to wind erosion: A comparison of the strength properties of biotic and salt crusts. Geomorphology 72 (1-4), 40–53. doi:10.1016/j.geomorph.2005.05.003
Li, C. Z. (2018). A review: The formation, development and prospect of soil salt crust on the view of soil erosion. J. Xinjiang Univ. Sci. Ed. 35 (04), 402–408+415. doi:10.13568/j.cnki.651094.2018.04.003
Li, S. H., Li, C. Z., and Fu, X. L. (2021). Characteristics of soil salt crust formed by mixing calcium chloride with sodium sulfate and the possibility of inhibiting wind-sand flow. Sci. Rep. 11, 9746. doi:10.1038/s41598-021-89151-1
Li, X. L., and Shen, X. D. (2006). The analysis on the mechanism of anti - wind erosion of soil crust. J. Arid Land Resour. Environ. 20 (2), 203–207. doi:10.3969/j.issn.1003-7578.2006.02.039
Lindström, N., Heitmann, N., Linnow, K., and Steiger, M. (2015). Crystallization behavior of NaNO3–Na2SO4 salt mixtures in sandstone and comparison to single salt behavior. Appl. Geochem. 63, 116–132. doi:10.1016/j.apgeochem.2015.07.007
Liu, C. Y. N. (2016). Experimental study on the Pure Salt Expansion of silty clay and salt expansion characteristics analysis ([China (BJ)]: Beijing Jiaotong University). [master’s thesis].
Menéndez, B., and Petráňová, V. (2016). Effect of mixed vs single brine composition on salt weathering in porous carbonate building stones for different environmental conditions. Eng. Geol. 210, 124–139. doi:10.1016/j.enggeo.2016.06.011
Nachshon, U., Weisbrod, N., Katzir, R., and Nasser, A. (2018). NaCl crust architecture and its impact on evaporation: Three‐dimensional insights. Geophys. Res. Lett. 45 (12), 6100–6108. doi:10.1029/2018GL078363
Nickling, W. G., and Ecclesstone, M. (1981). The effects of soluble salts on the threshold shear velocity of fine sand. Sedimentology 28 (4), 505–510. doi:10.1111/j.1365-3091.1981.tb01698.x
Nield, J. M., Neuman, C. M., O’brien, P., Bryant, R. G., and Wiggs, G. F. (2016). Evaporative sodium salt crust development and its wind tunnel derived transport dynamics under variable climatic conditions. Aeolian Res. 23, 51–62. doi:10.1016/j.aeolia.2016.09.003
Nield, J. M., Wiggs, G. F., King, J., Bryant, R. G., Eckardt, F. D., Thomas, D. S., et al. (2016). Climate–surface–pore‐water interactions on a salt crusted playa: Implications for crust pattern and surface roughness development measured using terrestrial laser scanning. Earth Surf. Process. Landf. 41 (6), 738–753. doi:10.1002/esp.3860
Rad, M. N., and Shokri, N. (2014). Effects of grain angularity on NaCl precipitation in porous media during evaporation. Water Resour. Res. 50 (11), 9020–9030. doi:10.1002/2014WR016125
Rad, M. N., Shokri, N., Keshmiri, A., and Withers, P. J. (2015). Effects of grain and pore size on salt precipitation during evaporation from porous media. Transp. Porous Media 110 (2), 281–294. doi:10.1007/s11242-015-0515-8
Rad, M. N., Shokri, N., and Sahimi, M. (2013). Pore-scale dynamics of salt precipitation in drying porous media. Phys. Rev. E 88 (3), 032404. doi:10.1103/PhysRevE.88.032404
Rijniers, L. A., Pel, L., Huinink, H. P., and Kopinga, K. (2005). Salt crystallization as damage mechanism in porous building materials—A nuclear magnetic resonance study. Magn. Reson. Imaging 23 (2), 273–276. doi:10.1016/j.mri.2004.11.023
Saidov, T. A., Pel, L., and Van Der Heijden, G. H. A. (2015). Crystallization of sodium sulfate in porous media by drying at a constant temperature. Int. J. Heat. Mass Transf. 83, 621–628. doi:10.1016/j.ijheatmasstransfer.2014.12.043
Shahidzdeh-bonn, N., Desarnaud, J., Bertrand, F., Chateau, X., and Bonn, D. (2010). Damage in porous media due to salt crystallization. Phys. Rev. E 81 (6), 066110. doi:10.1103/PhysRevE.81.066110
Shokri-kuehni, S. M. S., Rad, M. N., Webb, C., and Shokri, N. (2017). Impact of type of salt and ambient conditions on saline water evaporation from porous media. Adv. Water Resour. 105 (7), 154–161. doi:10.1016/j.advwatres.2017.05.004
Shokri-kuehni, S. M. S., Vetter, T., Webb, C., and Shokri, N. (2017). New insights into saline water evaporation from porous media: Complex interaction between evaporation rates, precipitation, and surface temperature. Geophys. Res. Lett. 44 (11), 5504–5510. doi:10.1002/2017GL073337
Steiger, M., and Asmussen, S. (2008). Crystallization of sodium sulfate phases in porous materials: The phase diagram Na2SO4–H2O and the generation of stress. Geochimica Cosmochimica Acta 72 (17), 4291–4306. doi:10.1016/j.gca.2008.05.053
Yi, X. Y., Zhao, H. L., Zhao, X. Y., Li, Y. Q., Zuo, X. A., and Fu, Z. (2006). Erodibility of aeolian soils in moisture content. Acta Pedol. Sin. 43 (4), 684–687. doi:10.3321/j.issn:0564-3929.2006.04.023
Zhang, J. G., Li, H. W., Li, Y. F., Li, Y. N., Ma, Y., Tian, L. L., et al. (2019). Artificial cultivation of soil salt crust and effects of its damage rate on soil evaporation. Trans. Chin. Soc. Agric. Eng. 35 (13), 138–144. doi:10.11975/j.issn.1002-6819.2019.13.015
Keywords: salt crust, crust appearance, compressive strength, crust toughness, wind erosion resistance
Citation: Xu B, Li C and Li Z (2022) Comparison of the characteristics of six salt crusts. Front. Environ. Sci. 10:905410. doi: 10.3389/fenvs.2022.905410
Received: 27 March 2022; Accepted: 14 July 2022;
Published: 11 August 2022.
Edited by:
Brandon L. Edwards, New Mexico State University, United StatesReviewed by:
Prabir K. Kolay, Southern Illinois University Carbondale, United StatesSara Mola Ali Abasiyan, University of Maragheh, Iran
Copyright © 2022 Xu, Li and Li. This is an open-access article distributed under the terms of the Creative Commons Attribution License (CC BY). The use, distribution or reproduction in other forums is permitted, provided the original author(s) and the copyright owner(s) are credited and that the original publication in this journal is cited, in accordance with accepted academic practice. No use, distribution or reproduction is permitted which does not comply with these terms.
*Correspondence: Chengzhi Li, eGRsaWNoZW5nemhpQHhqdS5lZHUuY24=