- College of Desert Control Science and Engineering, Inner Mongolia Agricultural University, Hohhot, China
Aims: In order to explore the adaptation mechanisms of Elaeagnus angustifolia to the arid environment in desert areas under the treatment of artificial plant retardants, we used to investigate artificial regulation by using retardants of paclobutrazol (PP333), paclobutrazol+adhesive (NPP333), and chlormequat (CCC) based on multiple factors and multiple levels.
Methods: Orthogonal experimental design of L9 (34) was used to design the experimental treatment combinations. Leaf morphological and structural characteristics determined by the paraffin section method were used to explain the effect of different treatments and their combinations.
Results: The leaves of Elaeagnus angustifolia were iso-petalous with obvious stellate epidermal fuzzy borders on the upper epidermis. The palisade tissue was well developed and tightly arranged. The T1-T9 treatment significantly increased leaf thickness. Conversely, leaf length and width showed a delayed growth effect, while leaf growth developed as an elongated type after application. After plant retardant control, the upper epidermal, palisade tissue, and spongy tissue thickness of the leaves showed a significant trend to increase, at the same time, the number of xylem rows increased and the number of cells per row increased and were arranged closely. Meanwhile, there was a synergistic evolution phenomenon among the indexes. The best treatment combination of plant retardants to regulate the leaf configuration of plants was selecting the concentration of 600 mg/L of PP333, using the root application + leaf application method for two applications.
Conclusions: The study showed that plant retardants improved the ability of plants to resist external environmental stress by reducing leaf area, increasing leaf and epidermal thickness, and promoting the development of mesophyll and vein structures in order to improve water retention capacity and prevent transitional transpiration.
1 Introduction
Under the macro-climate of global warming, land desertification is an ecological challenge facing arid and semi-arid regions (Huang et al., 2020). The ecological environment in arid and semi-arid zones is relatively fragile and human activities have put ecological pressure on the land. Desertification threatens regional human life and economic and ecological security (D’Odorico et al., 2019). Therefore, if regional ecological restoration is to be carried out, the use of economic and ecological plant measures applied to desertification control is the primary choice, as sandy plants will be able to withstand certain wind and sand hazards and resist external influences (Su et al., 2007). However, understanding the drought-resistant mechanisms of plants is of some practical importance in combating land desertification.
At present, there have been many studies on the physiological changes of plants under drought stress and the mechanism of drought tolerance in arid and semi-arid regions (Ramachandra Reddy et al., 2004). When the growing environment changes, plants change their morphological and physiological characteristics to adapt the growing environment (Baquedano and Castillo, 2006). As a consequence, plants growing in different conditions show significant differences in photosynthesis, anatomical characteristics, and physiological characteristics (Dolatabadian et al., 2011; Khandaker et al., 2017; Leon-Sanchez et al., 2020). In arid and semi-arid regions, water deficit and high light exposure are important factors limiting plant growth (Prasad et al., 2008). At the same time, as one of the most important stresses in the environment, drought can cause a series of physiological and biochemical responses in plants.
Identification of plant morphological forms and functions is the first step in improving the resistance of vegetation to harsh growing conditions (Toscano et al., 2018). It is well known that leaves are the main organ for the transpiration and photosynthesis of plants. They have been exposed to the growing environment for a long time, with the largest area of contact with the outside environment. Compared with the branching structure aboveground, leaves are highly sensitive to environments (Xin et al., 2012; Yang et al., 2014). The microscopic anatomical tissue structure of plants, such as epidermal thickness and palisade tissue, affects the intercellular CO2 concentration and diffusion of gases, with the results of long-term adaptation to harsh environments (He et al., 2008; Galle et al., 2010).
Elaeagnus angustifolia is distributed in arid, semi-arid, and saline areas in China with precipitation less than 150 mm (multi-year average). It is resistant to wind erosion and protects the land surface from erosion, which is usually used to build oasis protection forests in China (Huang et al., 2005; Liu et al., 2014; Yunus et al., 2005). Plant growth retardants delay longitudinal growth, increase aboveground branching structure, and reduce transpiration by reducing leaf area (Karimi et al., 2019). At present, most scholars have studied plant growth retardants to regulate leaf growth characteristics, photosynthesis, and physiological characteristics (Kasele, 1992; Zhou et al., 2019), while fewer scholars have studied the effect on leaf tissue structure. In general, changes in the cellular organization can efficiently raise mesophyll which is mainly due to the thicker leaf caused by well-developed palisade tissue and spongy tissue. Cells have an excellent capacity to store water and adaptation to drought conditions is enhanced (Chartzoulakis et al., 2002; Gratani and Bombelli, 2000; Hameed et al., 2002). Plant retardants have low toxicity and high efficiency, which are widely used to regulate plant growth and development (Sponsel and Hedden, 2010). They mainly inhibit the division and expansion of the apical meristem of the stem, thereby regulating the distribution of nutrient growth materials to affect the water content of the leaves, the chlorophyll content of the leaves, and the growth of the plant, leaf area, and leaf anatomy (Maienfisch et al., 2001; Zhu and Stafne, 2019). Such plant retardants can alter plant height and leaf size, however, it is not clear whether they can alter the intrinsic anatomy of the leaf to make it drought-tolerant in terms of tissue structure.
This study aimed to select application methods that would modify the morphological characteristics of leaves to enable them to survive successfully in arid and semi-arid environments. In addition, we assessed the relevance of the anatomical and morphological characteristics of leaves after application. We chose Elaeagnus angustifolia, a model plant, to evaluate the effects of plant growth retardants regulating leaf characteristics and anatomy. The results will provide a theoretical basis for the subsequent use of Elaeagnus angustifolia in desert areas for ecological vegetation restoration and construction of wind and sand-fixing forests.
2 Materials and Methods
2.1 Growth Conditions and Plant Material Preparation
The experimental area is located at the southwest edge of Ulanbuhe Desert, in Jilantai, Inner Mongolia Autonomous Region, China (39°46′59″N,105°37′31″E) which is located in the transition from a semi-arid to an arid region. The climate is a typical temperate continental climate. The mean average precipitation is 109.9 mm, while the average annual evaporation is 2,944 mm. The soil pH is 8.49–9.19, the top soil (0–30 cm) organic matter (SOM) content is 7.15–7.59 g kg−1, the top soil available phosphorus (AP) content is 2.95–4.20 mg kg−1, and the top soil available potassium (AK) content is 571–618 mg kg−1.
Two-year-old Elaeagnus angustifolia (from Jilantai local woodland) were used and transplanted in March 2019 and were spaced 1 m × 1 m in the field. Ten plants of each treatment were used as experimental plants and irrigated every 15 days to maintain plant growth. From April to May, watering took place every 10 days to ensure the survival of young plants.
2.2 Experimental Design
The Elaeagnus angustifolia were planted in the town of Jilantai. The experiment used an L9 (34) orthogonal test design with four factors including application method (A), application frequency (B), application type (C), and application concentration (D) with three levels of each factor, as shown in Table 1. The experiment was designed with nine treatment combinations as shown in Table 2 and each treatment was replicated 10 times with a total of 100 plants.
2.3 Method for the Determination of Morphological Characteristics
At the end of plant growth in September 2019, five plants from different treatment methods (T1-T9) were selected from three branches according to East, West, South and North directions. The sampling method was adopted for the random determination of morphological indicators. Ten fresh leaves were collected in each direction and the blade length and width were measured using a cursor caliper (0.01 mm).
2.4 Leaf Anatomical Structure Material Collection
Leaf anatomical measurements were obtained at the end of the experiment in September 2019. The branches selected for the test material were consistent with morphological indexes of mature leaves in the middle of the branches, and two leaves were sampled in each direction. A total of 40 leaves were sampled in each treatment, totaling 360 leaves.
2.5 Paraffin Section Production Method
Mature leaves were taken in different directions (the orthogonal test design plan T1-T9) and properly marked, in 20 ml vials containing FAA fixative (70% alcohol: formaldehyde: glacial acetic acid = 90:5:5). The vials were pumped (inoculated) using a medical syringe during fixation, and then sealed, sorted, and transported back to the laboratory for leaf dissection tests. The samples were made by the conventional paraffin sectioning method, stained with saffron-solid green, and sealed with neutral resin glue (Galle et al., 2010). A LEICA DM2000 microscope was used to observe leaf anatomy, and LAS V4.12 was used to capture pictures of samples and determine leaf thickness, upper cuticle thickness, upper epidermal thickness, lower epidermal thickness, and fenestrated tissue thickness. The palisade and spongy ratio, tightness of leaf palisade tissue, and tightness of leaf spongy tissue were determined by the formulas below (Karimi et al., 2019).
2.6 Data Analysis
Data were analyzed by Excel 2010 and SAS software without considering interactions. Effect of changes in the anatomical properties of plant leaves under different treatments (Table 4) was determined by one-way analysis of variance (ANOVA). Tukey’s HSD (honestly significant difference) test was used to analyze the differences between morphological characteristics. The data presented in the table are the mean value of three replications (mean ± standard deviation).
The data in this study were analyzed using the relative growth of each plant indicator for comparison.
Note: Mi is the relative growth amount of the i indicator in the growth period; MXi is the measured value of the i indicator at the end of growth in September 2019 after artificially regulated treatment; MYi is the basal value of the i indicator in May 2019 before application; Ki is the growth amount of the i indicator after application relative to CK; CKi is the control group of the i indicator with the application of clear water.
3 Results
3.1 Morphological Structure of Elaeagnus Angustifolia Leaves
The leaf morphological characteristics revealed significant differences among different treatments from T1-T9 (Table 3). The morphological structure of Elaeagnus angustifolia leaves changed considerably under the effect of artificial control. Different control methods (T1-T9) had a growth-promoting effect. Plant leaf thickness significantly increased (p < 0.05) with maximum growth under T8 treatment (13.03 μm) and the minimum under T3 treatment (2.39 μm). Leaf length growth ranged between 2.11 and 13.67 μm which was smaller compared with control, and leaf width growth ranged between 1.96 and 12.34 μm which was also smaller than control. Relative growth ratio between the leaf length and width was greater than 1, which ranged from 1.01 to 3.06 μm while the changes were not significant.
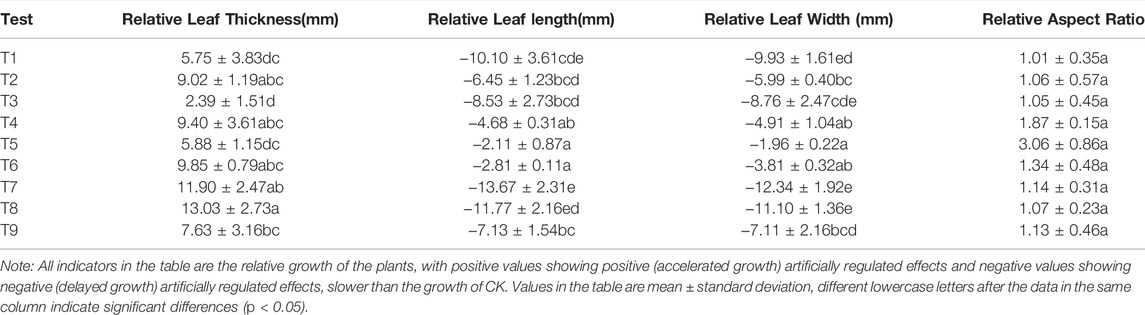
TABLE 3. Variability analysis of the growth of morphological characteristic indexes of Elaeagnus angustifolia leaves under different regulation methods.
3.2 Analysis of Anatomical Structure of Elaeagnus Angustifolia Leaves
3.2.1 Epidermis
Elaeagnus angustifolia leaves are isobilateral which indicates that the main veins of the leaf blade are raised on the back of the leaf blade in an irregular semi-circular shape. The surface of the leaf blade showed stellate glandular hairs (Figure 1A), which reduced the damage of strong light and control transpiration of the plant. Both the upper and lower epidermis of the Elaeagnus angustifolia leaves consisted of one layer of cells which were small and closely arranged. The upper epidermal cells consisted of a nearly rectangular arrangement of cells while the lower epidermal cells were nearly circular (Figures 1B,C). The upper cells were significantly larger than the lower cells. The upper epidermis showed an accelerated growth trend after each treatment and the maximum growth under T8 treatment reached 1.52 μm in thickness compared with control. The lower epidermis showed a delayed growth trend and the growth of the lower epidermal cells were lower than control (Table 4). The smallest growth was under T8 treatment.
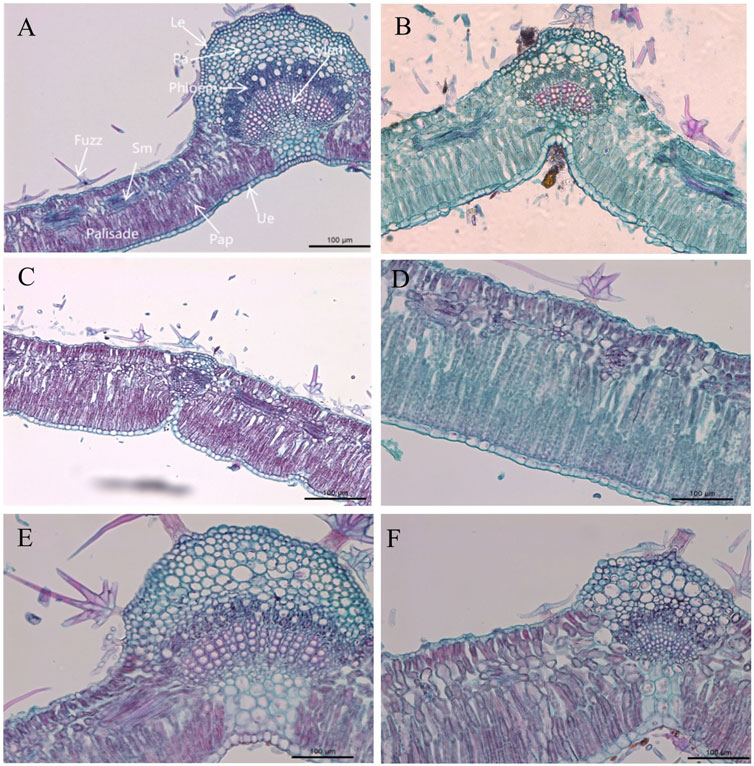
FIGURE 1. Transverse section of a date palm leaf blade. (A–C) Leaf cross-sections of midrib 10x; (D). Cross-sections of mesophyll 20x; (E,F). Cross-sections of leaf veins 20x; (A) CK, (B) T1 treatment, (C) T5 treatment, (D) T6 treatment showing spongy tissue with obvious fenestration, (E) T8 treatment showing thin-walled tissue development, (F) T9 treatment showing vascular bundle development. Ue. upper epidermis; Le. lower epidermis; Pap. palisade tissue; Sm. spongy tissue.
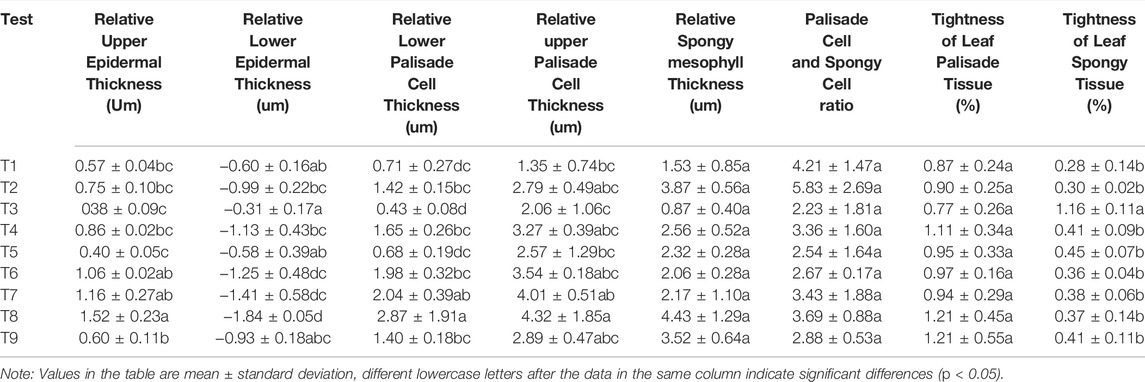
TABLE 4. Analysis of variability in the growth of anatomical structural indicators of Elaeagnus angustifolia leaves under different regulation methods.
3.2.2 Mesophyll
The leaf mesophyll of Elaeagnus angustifolia consists of two parts as fenestrations and spongy tissues. The fenestrations had a double-fenestrated structure and were well developed. The fenestrations were arranged on the inner side of the upper epidermis (Figure 1D). According to the cross-section of the leaf, the fenestrations of Elaeagnus angustifolia showed a significant increase in thickness under the nine different treatments used in this experiment (P<0.05).
Table 4 shows that the upper fenestrations consisted of long cylindrical cells arranged closely together and their thickness increased from 1.35 to 4.32 μm. The thickness of the lower fenestrations increased from 0.43 to 2.48 μm indicating that the upper fenestrations were thicker than the lower fenestrations. The spongy tissues also showed a trend of thickening ranging from 0.87 to 4.43 μm while the difference was not significant (p > 0.05). The ratio of spongy tissue growth to fenestrated tissue decreased. The spongy tissues gradually fenestrated under T6, T8, and T9 treatment where their relative fenestrated ratio showed an increasing trend. The relative tissue firmness and laxity of the leaf showed an increasing trend and the relative firmness of the leaf tissue structure varied from 77 to 121% with no significant difference among the treatments (p > 0.05). Relative laxity of leaf tissue structure varied from 28 to 116% under T3 treatment which was significantly higher than other treatment groups (p < 0.05). The relative thickness of the upper and lower fenestrations ranked as T8 > T7 > T6 > T4 > T2 > T9 > T1 > T3 > T5. The relative thickness of the spongy tissues ranked as T2 > T8 > T9 > T4 > T5 > T6 > T7 > T1 > T3 which was slightly different from the fenestrations. This showed that drought resistance of Elaeagnus angustifolia was improved by plant retardant regulation in terms of leaf mesophyll tissue structure.
3.2.3 Veins
The main veins of leaves were well developed with rounded and prominent structures which consisted of vascular bundles and mechanical tissue. The xylem was composed of 2-8 layers and 9–25 rows of the vessel and the caliber of which increased radially from the upper epidermis to the lower epidermis. The thick-horned tissue and thin-walled cells were well developed under each mode of regulation and showed a good sparing and supporting effect (Figure 1E and Figure 1F).
The k values (Table 5) indicated the strength of the influence under each treatment at different levels on the traits and the best combination was determined according to k1, k2, and k3. The extreme difference characterized the primary and secondary relationship between the influence of the factors on the measured traits, and the larger the extreme difference R, the greater the influence of the factor on the traits. As can be seen from Table 5, the effects of four treatments on the upper epidermal thickness, lower fenestrated tissue thickness, spongy tissue thickness, and leaf thickness of Elaeagnus angustifolia ranked as A > D > C > B, therefore, application method > application concentration > application type > application frequency. In conclusion, all the anatomical structures of Elaeagnus angustifolia leaves were positively regulated after artificial control and the optimal combination for the regulation of Elaeagnus angustifolia leaf was A3B2C1D2, (A root application + leaf application B application of two times C PP333 D 600 mg/L).
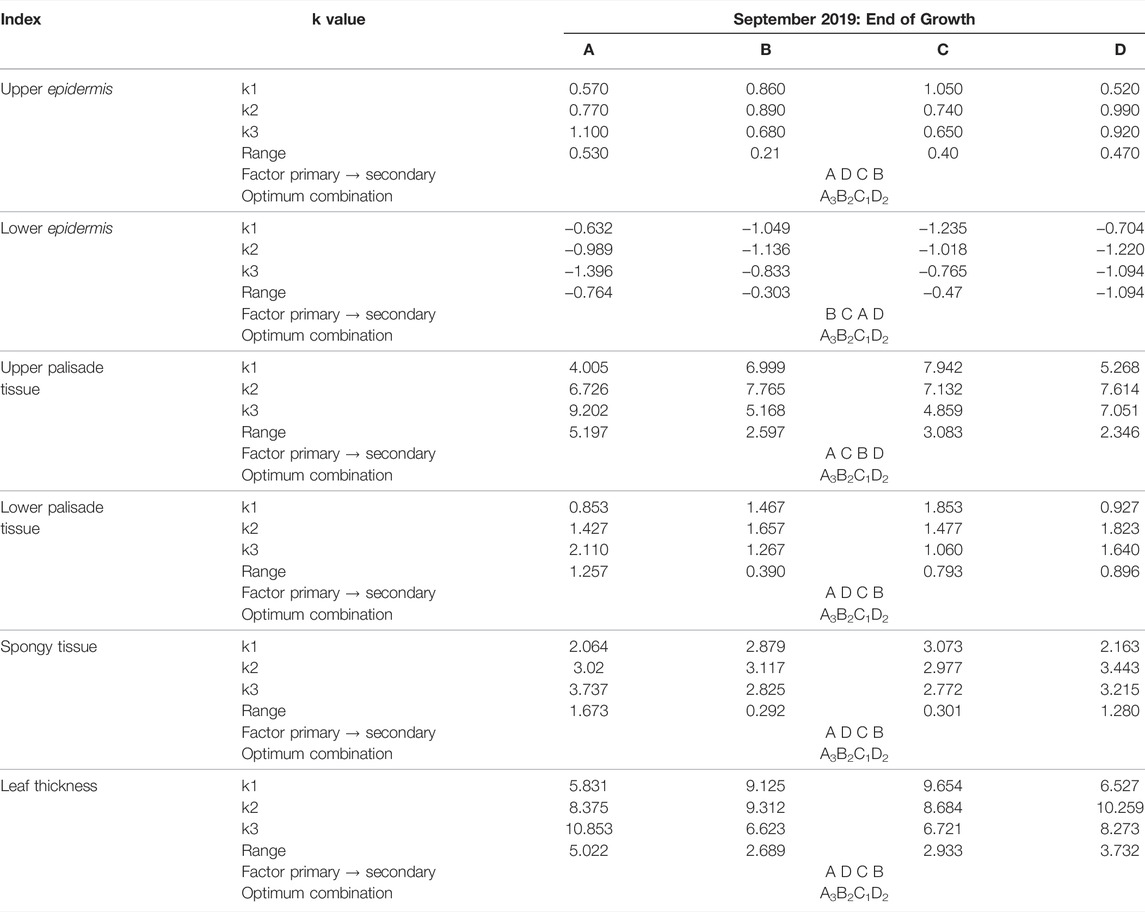
TABLE 5. Orthogonal test analysis of artificially regulated growth of leaf conformation indicators of Elaeagnus angustifolia.
4 Discussion
As shown in Figure 2, the average temperature in the trial area was high during the plant growth period (March to October). Rainfall was extremely low, but evaporation was high. This makes it difficult for the plants to maintain normal growth. So, applying plant retardants by slowing down the growth rate of the leaves and increasing their transpiration rate is an effective way to increase the growth rate of plants in drought conditions. The leaf is an important organ for plant respiration, photosynthesis, absorption, and secretion, and its morphological characteristics can give feedback on the effect of the ability to adapt to a complex environment (Singh et al., 2005). Photosynthesis is the basis for the synthesis of organic matter and energy storage in plants. It is a decisive factor in the productivity of plants. The leaf is the main organ of photosynthesis and the anatomical structure of the leaf is closely related to the photosynthetic efficiency of the plant. Some studies have shown that photosynthetic rate and leaf epidermal cell thickness, and leaf thickness are positively correlated. On the other hand, the thicker the leaf palisade tissue, the more beneficial the light energy capture. At the same time, it can effectively increase the photosynthetic rate of the leaves (Yang et al., 2017). For example, the thickness of Nitraria tangutorum thinned significantly with increasing drought, epidermal and palisade tissue became smaller and more closely packed, and abundant areas of spongy tissue turned into palisade tissue. The leaf size of Dryas octopetala var. asiatica (Nakai) Nakai is increased by air temperature (Zhou et al., 2019). Furthermore, the anatomical structure of the plant leaf is extremely sensitive to changes in external environmental factors and adapts to the growing environment by adjusting its tissue structure to increase photosynthetic rate, reduce transpiration rate, and enhance water storage capacity. Leaf thickness affects the absorption of light by plants, and leaf thickness increase the light energy utilization and water storage capacity of leaves while preventing excessive transpiration and facilitating photosynthesis. The result showed that leaf thickness in desert areas can characterize water use efficiency and the thicker the leaf thickness, the stronger the water use efficiency. The results of this study showed that the application of plant retardant had a thickening effect on plant leaf thickness (compared with the control) which may be caused by other factors, such as our research area located in a high-temperature and low-rainfall area. All these factors may have an effect on the relatively large number of epidermal cells on the leaf surface and further reduce water transpiration to improve plant water storage performance. The results of the present study also demonstrated that the relative leaf length growth and relative leaf width growth of leaves showed inhibition under different regulation methods. The relative leaf length to width ratio increased which indicated that the shape of the plant leaf was long and narrow after the regulation.
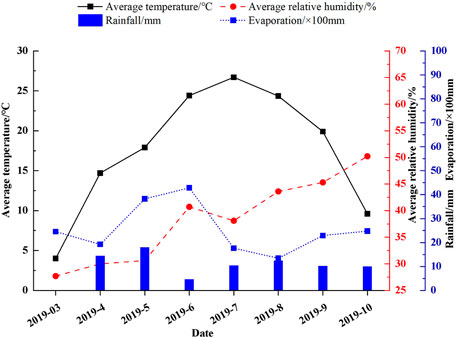
FIGURE 2. Characteristics of changes in meteorological factors in the pilot area between March and October 2019.
Epidermal cells have good water retention and storage properties which help to enhance plant water regulation and prevent excessive water transpiration, thereby resisting external environmental stress (Wyka et al., 2019). The study in this paper showed that the increase in thickness of the upper epidermal cells of the leaves was growth-promoting (positive growth compared to the control) while the increase in the thickness of the lower epidermal cells delayed growth (negative growth compared to the control). Palisade tissues affect the absorption and use of light energy by plants, and well-developed, closely arranged palisade tissues can effectively alleviate the damage to plant leaves from stresses such as strong light and water deficit. The relatively reduced spongy tissue and palisade tissue ratio improves the efficiency of CO2 conduction from the lower stomatal chamber to the photosynthesis site, while alleviating the phenomenon of reduced CO2 conduction rate due to changes in leaf flesh tissues (Azoz, S. N. et al., 2020), enabling plants to achieve a high conduction rate. The improvement of photosynthesis can make plants adapt to arid environment. The well-developed thick-horn tissue and duct in the main vein can store water and also have protective function. At the same time, developed guard cells can improve the transpiration rate of plants and promote the transport of water by plants (Zaky, I. F. et al., 2019). In this study, several characteristics of the transverse anatomical structure of Elaeagnus angustifolia leaves (e.g., thickness of palisade tissue, the thickness of spongy tissue, compactness, laxity, etc.) increased under the treatments of nine plant retardants. The cell volume of palisade tissue was reduced and the number of layers increased while vascular tissue was well developed.
As can be seen from Table 6, photosynthesis in Elaeagnus angustifolia leaves was significantly improved by the application of plant retardants. This is consistent with the researchers’ conclusion that by altering leaf size, leaf anatomy can improve the photosynthetic capacity of plants.
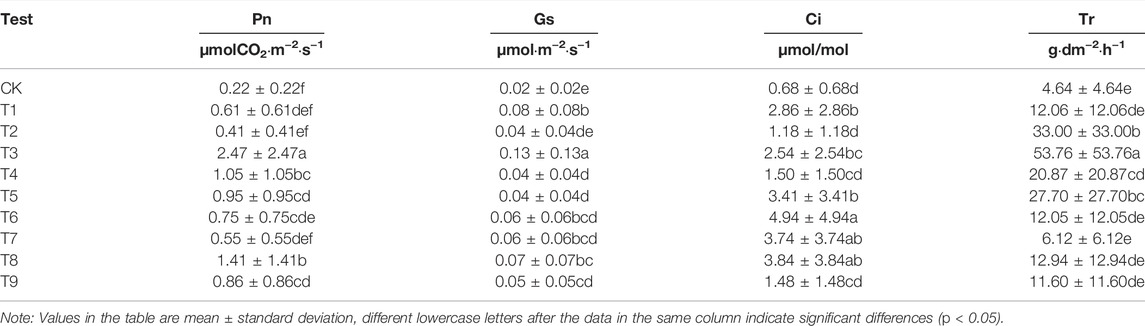
TABLE 6. Changes in the photosynthetic characteristics of Elaeagnus angustifolia Under different regulation methods.
5 Conclusion
In summary, plant retardant treatments T1 to T9 at the early stage of growth affected the morphological structure of plant leaves. These changes reduced transpiration and improved photosynthesis through reducing leaf area and increasing leaf thickness, among which T7 treatment (A root application + leaf application B one application C PP333 + adhesive D 600 mg/L) had the best effect. At the same time, it can changed the surface of the leaf blade, in which T8 treatment (A root application + B leaf application with two applications of C PP333 + 600 mg/L) had the best effect. The optimal combination of leaf regulation was A3B2C1D2 (600 mg/L of PP333, using the root application + leaf application method twice).
Data Availability Statement
The original contributions presented in the study are included in the article/Supplementary Material, further inquiries can be directed to the corresponding author.
Author Contributions
YG, conceptualization: WL and CZ, methodology: ZX and XT, software: CZ, formal analysis and data curation: WL, CZ, and ZX, writing, reviewing, and editing: WL and CZ. All authors have read and agreed to the published version of the manuscript.
Funding
This study was funded by the Inner Mongolia Autonomous Region Science and Technology Program (2021GG0073).
Conflict of Interest
The authors declare that the research was conducted in the absence of any commercial or financial relationships that could be construed as a potential conflict of interest.
Publisher’s Note
All claims expressed in this article are solely those of the authors and do not necessarily represent those of their affiliated organizations, or those of the publisher, the editors and the reviewers. Any product that may be evaluated in this article, or claim that may be made by its manufacturer, is not guaranteed or endorsed by the publisher.
Acknowledgments
We would like to thank Wiley S. Kollar and Yingmei Ma for revising this manuscript.
References
Baquedano, F. J., and Castillo, F. J. (2006). Comparative Ecophysiological Effects of Drought on Seedlings of the Mediterranean Water-Saver Pinus Halepensis and Water-Spenders Quercus Coccifera and Quercus ilex. Trees 20 (6), 689–700. doi:10.1007/s00468-006-0084-0
Chartzoulakis, K., Patakas, A., Kofidis, G., Bosabalidis, A., and Nastou, A. (2002). Water Stress Affects Leaf Anatomy, Gas Exchange, Water Relations and Growth of Two Avocado Cultivars. Sci. Hortic. 95 (1-2), 39–50. doi:10.1016/s0304-4238(02)00016-x
D’Odorico, P., Rosa, L., Bhattachan, A., and Okin, G. S. (2019). “Desertification and Land Degradation,” in Dryland Ecohydrology. Editors P. D'Odorico, A. Porporato, and C. Wilkinson Runyan (Cham: Springer International Publishing), 573–602.
Dolatabadian, A., Sanavy, S. A. M., and Ghanati, F. (2011). Effect of Salinity on Growth, Xylem Structure and Anatomical Characteristics of Soybean. Not. Sci. Biol. 3 (1), 41–45. doi:10.15835/nsb315627
Galle, A., Esper, J., Feller, U., Ribas-Carbo, M., and Fonti, P. (2010). Responses of Wood Anatomy and Carbon Isotope Composition of Quercus Pubescens Saplings Subjected to Two Consecutive Years of Summer Drought. Ann. For. Sci. 67 (8), 1–8. doi:10.1051/forest/2010045
Gratani, L., and Bombelli, A. (2000). Leaf Anatomy, Inclination, and Gas Exchange Relationships in Evergreen Sclerophqldous and Drought Semideciduous Shrub Species. Photosynthetica 37 (4), 573–585. doi:10.1023/A:1007171525298
Hameed, M., Mansoor, U., Ashraf, M., and Rao, A.-U.-R. (2002). Variation in Leaf Anatomy in Wheat Germplasm from Varying Drought-Hit Habitats. Int. J. Agric. Biol. 4, 12–16.
He, C.-X., Li, J.-Y., Zhou, P., Guo, M., and Zheng, Q.-S. (2008). Changes of Leaf Morphological, Anatomical Structure and Carbon Isotope Ratio with the Height of the Wangtian Tree (Parashorea Chinensis) in Xishuangbanna, China. J. Integr. Plant Biol. 50 (2), 168–173. doi:10.1111/j.1744-7909.2007.00620.x
Huang, J., MaimaitijiangYang, C., and Wang, C. (2005). Present Situation and Prospect about the Study of Elaeagnus Angustifolia L. Chin. Wild Plant Resour. 2005, 26−28+33
Huang, J., Zhang, G., Zhang, Y., Guan, X., Wei, Y., and Guo, R. (2020). Global Desertification Vulnerability to Climate Change and Human Activities. Land Degrad. Dev. 31 (11), 1380–1391. doi:10.1002/ldr.3556
Karimi, M., Ahmadi, A., Hashemi, J., Abbasi, A., Tavarini, S., Pompeiano, A., et al. (2019). Plant Growth Retardants (PGRs) Affect Growth and Secondary Metabolite Biosynthesis in Stevia rebaudiana Bertoni under Drought Stress. South Afr. J. Bot. 121, 394–401. doi:10.1016/j.sajb.2018.11.028
Kasele, I. N. (1992). Growth Retardants Promote Drought Resistance in Corn (Zea mays L.). Colorado: Colorado State University.
Khandaker, M., Awang, I., and Ismail, S. Z. (2017). Effects of Naphthalene Acetic Acid and Gibberellic Acid on Plant Physiological Characteristics of Wax Apple (Var. Jambu Madu). Bulg. J. Agric. Sci. 23, 396–404.
Leon-Sanchez, L., Nicolas, E., Prieto, I., Nortes, P., Maestre, F. T., and Ignacio Querejeta, J. (2020). Altered Leaf Elemental Composition with Climate Change Is Linked to Reductions in Photosynthesis, Growth and Survival in a Semi-arid Shrubland. J. Ecol. 108 (1), 47–60. doi:10.1111/1365-2745.13259
Liu, Z., Zhang, H., Sheng, Y., Yang, X., and Di, W. (2014). Effects of NaCl Stress on Growth and Photosynthetic Characteristics of Elaeagnus Angustifolia Seedlings. Sci. Silvae Sin. 50 (1), 32–40. doi:10.11707/j.1001-7488.20140106
Maienfisch, P., Huerlimann, H., Rindlisbacher, A., Gsell, L., Dettwiler, H., Haettenschwiler, J., et al. (2001). The Discovery of Thiamethoxam: a Second-Generation Neonicotinoid. Pest. Manag. Sci. 57 (2), 165–176. doi:10.1002/1526-4998(200102)57:2<165:aid-ps289>3.0.co;2-g
Prasad, P. V. V., Staggenborg, S. A., and Ristic, Z. (2008). Impacts of Drought And/or Heat Stress on Physiological, Developmental, Growth, and Yield Processes of Crop Plants. Response of Crops to Limited Water, 301–355.
Reddy, A. R., Chaitanya, K. V., and Vivekanandan, M. (2004). Drought-induced Responses of Photosynthesis and Antioxidant Metabolism in Higher Plants. J. Plant Physiology 161 (11), 1189–1202. doi:10.1016/j.jplph.2004.01.013
Singh, S. P., Bargali, K., Joshi, A., and Chaudhry, S. (2005). Nitrogen Resorption in Leaves of Tree and Shrub Seedlings in Response to Increasing Soil Fertility. Curr. Sci. 89 (2), 389–396. doi:10.1073/pnas.0505226102
Sponsel, V. M., and Hedden, P. (2010). Gibberellin Biosynthesis and inactivation∥Davies P J. Plant Hormones. Dordrecht: Springer, 63–94. doi:10.1007/978-1-4020-2686-7_4
Su, Y. Z., Zhao, W. Z., Su, P. X., Zhang, Z. H., Wang, T., and Ram, R. (2007). Ecological Effects of Desertification Control and Desertified Land Reclamation in an Oasis-Desert Ecotone in an Arid Region: A Case Study in Hexi Corridor, Northwest China. Ecol. Eng. 29 (2), 117–124. doi:10.1016/j.ecoleng.2005.10.015
Toscano, S., Ferrante, A., Tribulato, A., and Romano, D. (2018). Leaf Physiological and Anatomical Responses of Lantana and Ligustrum Species under Different Water Availability. Plant Physiology Biochem. 127, 380–392. doi:10.1016/j.plaphy.2018.04.008
Wyka, T. P., Bagniewska-Zadworna, A., Kuczynska, A., Mikolajczak, K., Ogrodowicz, P., Zytkowiak, M., et al. (2019). Drought-induced Anatomical Modifications of Barley (Hordeum Vulgare L.) Leaves: An Allometric Perspective. Environ. Exp. Bot. 166, 103798. doi:10.1016/j.envexpbot.2019.103798
Xin, C., Zhang, H., and Zhang, Z. (2012). Morphological,Anatomical,Photosynthetic and Physiological Responses of Sorbus Amabilis Seedlings under Different Shade Environments. J. Northeast For. Univ. 40, 24−27+33. doi:10.13759/j.cnki.dlxb.2012.10.025
Yang, S.-J., Sun, M., Zhang, Y.-J., Cochard, H., and Cao, K.-F. (2014). Strong Leaf Morphological, Anatomical, and Physiological Responses of a Subtropical Woody Bamboo (Sinarundinaria Nitida) to Contrasting Light Environments. Plant Ecol. 215 (1), 97–109. doi:10.1007/s11258-013-0281-z
Yang, W. L., Zhou, W. Q., and Sun, J. F. (2017). Effects of PP333 and Exogenous ABA on Leaf Tissue Structure of Armeniaca vulgari‘Luntaibaixing ’[J]. Agric. Sci. Technol. 18 (12), 2241–2245. doi:10.16175/j.cnki.1009-4229.2017.12.011
Yunus, Q., Xiu-Xia, L. I., Yang, L. I., and Jiuguang, G. (2005). Effects of Salt Stress on Membrane Lipid Peroxidation and Protective Enzymes in Leaves of Elaeagnus Angustifolia L. Arid Zone Res. 2005 (4), 87–91. doi:10.13866/j.azr.2005.04.016
Zhou, Y., Deng, J., Tai, Z., Jiang, L., Han, J., Meng, G., et al. (2019). Leaf Anatomy, Morphology and Photosynthesis of Three Tundra Shrubs after 7-Year Experimental Warming on Changbai Mountain. Plants (Basel) 8 (8), 271. doi:10.3390/plants8080271
Keywords: plant retardant, Elaeagnus Angustifolia, leaf anatomy, environmental adaptability, arid and semi-arid area
Citation: Zhang C, Li W, Gao Y, Xu Z and Tian X (2022) Artificial Regulation Effect of Plant Retardants on Leaf Anatomical Characteristics of Elaeagnus Angustifolia. Front. Environ. Sci. 10:900960. doi: 10.3389/fenvs.2022.900960
Received: 21 March 2022; Accepted: 19 April 2022;
Published: 06 June 2022.
Edited by:
Jifeng Deng, Shenyang Agricultural University, ChinaReviewed by:
Zhi Dong, Shandong Agricultural University, ChinaMetin Turan, National Eye Institute (NIH), United States
Copyright © 2022 Zhang, Li, Gao, Xu and Tian. This is an open-access article distributed under the terms of the Creative Commons Attribution License (CC BY). The use, distribution or reproduction in other forums is permitted, provided the original author(s) and the copyright owner(s) are credited and that the original publication in this journal is cited, in accordance with accepted academic practice. No use, distribution or reproduction is permitted which does not comply with these terms.
*Correspondence: Yong Gao, MTM5NDg4MTU3MDlAMTYzLmNvbQ==
†The author have contributed equally to this work and share first authorship