- 1School of Environmental Sciences, University of Guelph, Guelph, ON, Canada
- 2Swift Current Research and Development Centre, Agriculture and Agri-Food Canada, Swift Current, SK, Canada
- 3Department of Geography, Environment and Geomatics, University of Guelph, Guelph, ON, Canada
- 4Department of Biology, University of Western Ontario, London, ON, Canada
Leaching of nitrate (NO3−)—a reactive nitrogen form with impacts on ecosystem health—increases during the non-growing season (NGS) of agricultural soils under cold climates. Cover crops are effective at reducing NGS NO3− leaching, but this benefit may be altered with less snow cover inducing more soil freezing under warmer winters. Our objective was to quantify the effect of winter warming on NO3− leaching from cover crops for a loamy sand (LS) and a silt loam (SIL) soil. This research was conducted over 2 years in Ontario, Canada, using 18 high-precision weighing lysimeters designed to study ecosystem services from agricultural soils. Infra-red heaters were used to simulate warming in lysimeters under a wheat-corn-soybean rotation planted with a cover crop mixture with (+H) and without heating (-H). Nitrate leaching determination used NO3− concentration at 90 cm (discrete sampling) and high temporal resolution drainage volume measurements. Data were analyzed for fall, overwinter, spring-thaw, post-planting, and total period (i.e., November 1 to June 30 of 2017/2018 and 2018/2019). Warming significantly affected soil temperature and soil water content—an effect that was similar for both years. As expected, experimental units under + H presented warmer soils at 5 and 10 cm, along with higher soil water content in liquid form than –H lysimeters, which translated into higher drainage values for + H than –H, especially during the overwinter period. NO3− concentrations at 90 cm were only affected by winter heating for the LS soil. The drainage and NO3− concentrations exhibited high spatial variation, which likely reduced the sensitivity to detect significant differences. Thus, although absolute differences in NO3− leaching between LS vs. SIL and +H (LS) vs. –H (LS) were large, only a trend occurred for higher leaching in LS in 2018/2019. Our research demonstrated that soil heating can influence overwinter drainage (for LS and SIL soils) and NO3− concentration at 90 cm in the LS soil—important NO3− leaching controlling factors. However, contrary to our initial hypothesis, the heating regime adopted in our study did not promote colder soils during the winter. We suggest different heating regimes such as intermittent heating to simulate extreme weather freeze/thaw events as a future research topic.
Introduction
Agricultural soils can experience high nitrate (NO3−) leaching losses over winter as a result of NO3− accumulation at a time when plant uptake is low and water surplus is high, coupled with high drainage during snowmelt (de Wit et al., 2008; Durán et al., 2013; Endo et al., 2018; Chantigny et al., 2019). Nitrate leaching can further intensify after soils are exposed to severe freezing, or after an increase in the frequency of soil freeze-thaw cycles, due to microbial cell or root lysis, or the disruption of soil aggregates and plant litter (Groffman et al., 2001; Schimel et al., 2007; Machado et al., 2020; Starkloff et al., 2017). Soil freezing can be mediated by snow cover insulation from the cold air temperatures that occur overnight or during cold spells (Qian et al., 2011; Yang et al., 2018; Boswell et al., 2020). Paradoxically, in northern temperate regions, the most intense soil freezing can thus occur in warm winters as a result of reduced snow cover (Sharratt, 1993; Groffman et al., 2011; Zhou et al., 2017). However, despite the potential for increased NO3− leaching in response to increased soil freezing, it remains unclear to what extent climate warming might alter NO3− leaching over winter in agricultural soils. Specifically, freeze-thaw cycles of mild to moderate intensity do not always affect soil NO3− concentrations, which indicates that increased NO3− leaching in response to increased soil freezing may only occur at threshold soil temperatures (Joseph and Henry, 2008). Moreover, soil warming over winter or a decrease in the length of winter can increase soil nitrogen (N) mineralization, and thus increase the pool of NO3− potentially susceptible to leaching prior to uptake by the summer crop (Dalias et al., 2002; Turner and Henry, 2009). Effects on the pool of NO3− coupled with changes in drainage due to warming could lead to enhanced non-growing season NO3− leaching.
An emerging strategy to reduce NO3− leaching is to plant a cover crop after harvesting the cash crop, to enable soil NO3− uptake during the non-growing season (NGS) (Drury et al., 2014; De Notaris et al., 2018; Abdalla et al., 2019; Langelier et al., 2021). These crops are not harvested and can be either winter killed or terminated by herbicides prior to the next growing season (Rosario-Lebron et al., 2019; Toom et al., 2019). Single species cover crop studies are plentiful and demonstrate that non-leguminous species decrease soil NO3−, which could decrease NO3− leaching (Shepard et al., 1993; Hooker et al., 2008; Kramberger et al., 2009; Carey et al., 2016). Leguminous cover crop species can increase N availability through biological N fixation (Kramberger et al., 2014, Machado et al., 2021a), but were shown to reduce soil NO3− compared to bare soil (Vogeler et al., 2019). Increasing crop diversity—e.g., by using cover crops—could potentially reduce the impacts of climate change as well as reduce NO3− leaching during the non-growing season under varying weather conditions (Constantin et al., 2011; Gaudin et al., 2015). For a four species cereal-crucifer-legume mixture planted after spring wheat, we recently demonstrated a dramatic decrease in NO3− leaching of 67% averaged across two soil types compared to no cover crop use, with most of the reduction occurring over winter and spring thaw (Lapierre et al., 2022). However, altered winter conditions through increased soil freezing caused by decreased snow cover could change cover crop decomposition dynamics and affect NO3− leaching (Alonso-Ayuso et al., 2014; Toom et al., 2019). These effects could differ among soil types, both because of variation in cation exchange capacity and drainage, and because the damage to soil caused by soil freezing can depend on soil texture (Song et al., 2017; Starkloff et al., 2017).
Efforts to quantify NO3− leaching in response to variation in winter conditions have been complicated by methodological limitations (Henry, 2007). First, while instantaneous measurements of NO3− concentrations in the plant rooting zone (e.g., via soil extraction) or more integrative measures (e.g. via resin bags/strips) can reveal the accumulation of soil NO3− resulting from freezing damage (or conversely increased microbial N mineralization in warm soil), these studies do not reveal how much of this NO3− is ultimately lost below the rooting zone via leaching (Bingham and Biondini, 2011; Kirschke et al., 2019). Likewise, while 15N tracer studies provide a powerful tool for quantifying the loss of a cohort of soil NO3− over a given sampling interval, the form and timing of NO3− losses remain unknown (Turner and Henry, 2009). Soil solution samplers can be inserted below the rooting zone, but it is difficult to place the resulting concentration data in the context of drainage (Pawlick et al., 2019). Experiments under controlled conditions, using intact soil mesocosms are an alternative to study soil N dynamics in response to variations in winter temperatures (Libby et al., 2020), but simulating natural conditions can be challenging, and freezing and drainage artifacts can occur (Joseph and Henry, 2008). Likewise, snow removal or exclusion field experiments can exaggerate soil freezing intensity by exposing the soil to cold air at a time when snow cover would typically be present (i.e., snow removal is most effective for simulating reduced precipitation, not warming) (Groffman et al., 2001). Alternatively, warming of field plots via overhead heaters is a good alternative to study winter warming (Kimball et al., 2008). Coupling the use of overhead heaters to newer generation weighing lysimeters where the lower boundary is closely controlled to mimic field conditions, drainage is directly measured and NO3− concentrations can be determined below the root zone through soil solution sampling (Pütz et al., 2016) has the potential to provide NO3− leaching estimates under cover crop management in response to variation in winter conditions.
The objective of this research was to quantify the effect of simulated winter warming on NO3− leaching from cover crops for both a loamy sand (LS) soil and a silt loam (SIL) soil. We hypothesized that winter warming would increase NO3− leaching based on the assumption that decreased snow cover would alter soil water and temperature conditions and increase the freezing intensity resulting in substrate release from cover crops, while also increasing over-winter drainage. In addition, we also hypothesized that the warming effect on NO3− leaching would vary by soil type.
Materials and methods
Research site and infrastructure
This experiment complements the results from research conducted at this same lysimeter facility, during the same period, to study NO3− leaching from two contrasting soils in response to use of a cover crop mixture in comparison to no cover crop use (Lapierre et al., 2022). Here, we investigated NO3− leaching losses for the cover crop treatment subjected to winter warming compared to a non-warmed control. The lysimeter facility was designed and installed by METER Group Inc., Munich, Germany in July 2016 at the Elora Research Station, Ontario, Canada (43o38’21.2″ N 80o22’56.2″ W) and is similar to sites described by Pütz et al. (2016). The facility is under a humid continental climate (Dfb) according to the Köppen—Geiger classification system (Peel et al., 2007), with a mean annual temperature of 6.6°C and average annual precipitation of 879 mm (ECCC, 2020). Detailed information on lysimeter installation and operation is presented in Lapierre et al. (2022), Zeitoun et al. (2021), and Brown et al. (2021). A brief description is provided here.
The experimental site has three blocks of 6 lysimeters (i.e., 18 in total), each with a 1 m2 surface area. Each block consists of 6 cylindrical concrete basins in which lysimeters were placed that are connected via underground conduits to one of three centralized service wells (Supplementary Figure S1). Each lysimeter consists of a 1.5 m deep stainless-steel cylinder containing an undisturbed soil monolith. Soil cores were extracted following the methodology of Hertel and von Unold (2014) and were transported to the lysimeter site. The lysimeter cores rest on balances with a resolution of 10 g (0.01 mm precipitation). Sensors (soil temperature, matric potential, and volumetric water content) were installed at 5, 10, 30, 60, and 90 cm depth in each lysimeter. Each lysimeter was also equipped with porous ceramic cups installed at the same depths that are connected to a vacuum pump and water sampling system. Each lysimeter is equipped with a boundary control system to ensure that each lysimeter’s matric potential at the lower boundary is similar to field conditions. The lower boundary of each lysimeter is monitored by one tensiometer installed at 140 cm. Field conditions are monitored using three tensiometers installed at 140 cm depth in the field adjacent to the lysimeter site. The boundary control system of each lysimeter consists of a bi-directional pump that is connected to ceramic cups installed in each lysimeter at 140 cm depth. Tubing connects the ceramic cups to the pump, which is then connected to a drainage tank (one for each lysimeter) placed in the service well and resting on a balance. The weight of each drainage tank is measured by a balance with a resolution of 1 g (0.001 mm precipitation). The pumps either draw water out of the lysimeter to the drainage tank or pump drainage tank water back into the lysimeter. A program in the datalogger determines the amount and direction of flow to match the water potential at 140 cm in the lysimeter core with the water potential measured in the surrounding field at the same depth.
Half of the experimental units contain grey-brown luvisol soil extracted at the Elora Research Station (43o38’20.2″ N 80o24’36.9″ W). This soil has a particle distribution in the 0-32 cm horizon of 38.0, 54.5, and 7.5%, sand, silt, and clay, respectively—a silt loam A horizon (SIL). The remaining 9 cores were extracted at a farm situated in Cambridge, Ontario, Canada (43o27’27.6″ N 80o20’47.5″ W), a coarser brunisolic grey-brown luvisol with a particle distribution in the 0-28 cm horizon of 79.2, 17.5, and 3.3%, sand, silt, and clay, respectively—a loamy sand A horizon (LS) (Zeitoun et al., 2021).
Experimental design and simulated winter warming
The lysimeters of each soil type were under a diverse crop rotation (i.e., soybean—spring wheat [+ cover crops]—corn [+ cover crops]). The following treatments were randomly assigned per soil type, each with three replicates: (i) diverse rotation with simulated winter warming (+H); (ii) diverse rotation without simulated winter warming (-H). An initial year of measurements before treatments were applied (i.e., with all lysimeters under the same crop management and no winter heating) showed minimal variability of water balance components between lysimeters indicating little disturbances from the soil extraction process (Brown et al., 2021). For this study, measurements were performed from November 2017 to June 2018 (2017/2018) and November 2018 to June 2019 (2018/2019). A longer period of monitoring would be ideal to capture a greater range of climatic conditions; however, this study was limited to 2 years due to cost and labour constraints. Most studies examining nitrate leaching from diversified cropping systems have been of 2-3 years duration (Tonitto et al., 2006; Abdalla et al., 2019). Measurements were stopped after June as drainage events did not occur after that time. The simulation of winter warming was achieved with infra-red heaters, equipped with 1000W 120v ceramic heating elements (Mor Electric ALEX Radiant Fixtures, Comstock, Michigan, United States). The methodology used to warm the lysimeters followed the methods of Kimball et al. (2008), but with only one heater per plot to account for the smaller area being warmed. Following installation, the heat distribution at the ground surface was assessed using thermal imaging, and the reflectors were adjusted to maximize even distribution (data not shown). Heaters were turned on once air temperature was consistently below 0°C and the soil surface started to freeze. In 2017/2018, heaters were turned on December 4 and removed May 1. In 2018/2019, heaters were turned on November 20 and removed May 2.
Crop management in 2017 (the growing season preceding this study) and 2018 were previously described in Lapierre et al. (2022). Briefly, diverse lysimeters were on a spring-wheat and corn phase in 2017 and 2018, respectively. In the wheat phase in 2017, 67, 33.5, and 33.5 kg ha−1 of nitrogen, phosphorus, and potassium fertilizer, respectively, were applied at planting on April 27. Wheat was harvested on August 1, 2017, and following the harvest, a cover crop mixture—selected based on ability to fix N, take up residual soil NO3−, and grow in Ontario (Kramberger et al., 2009; Carey et al., 2016; OMAFRA, 2017)—was planted on August 19 and received 50 kg N ha−1 as urea on August 25. The cover crop mixture used in 2017 contained daikon radish (Raphanus sativus var. Longipinnatus), crimson clover (Trifolium incarnatum), oats (Avena sativa), and cereal ryegrass (Secale cereale). The cover crops were terminated by herbicide on 18 May 2018, 1 week before corn was planted in 2018.
The corn phase of 2018 was planted on May 23, received 16.7 kg N ha−1 and 87 kg phosphorus ha−1 on May 10, followed by 74 kg N ha−1 applied as urea before planting on May 22, 77 kg N ha−1 applied as urea on June 27, and was harvested on October 24. The mixture of cover crops used in 2018 consisted of crimson clover and annual ryegrass and was under-seeded into the corn at the sixth leaf stage on June 26.
Nitrate leaching determination
Total leaching was calculated using net drainage measurements from the lysimeter system and discrete NO3− concentration in the drained water at 90 cm depth (a depth below the root zone and less influenced by the lower boundary controls of the lysimeter). Transport of NO3− is rapid through soils as it is highly water soluble (van Kessel et al., 2009). Transit times to the 90 cm depth based on the hydraulic conductivity measured for each horizon in Brown et al. (2021) were 5.6 h for LS and 51.3 h for SIL (Supplementary Table S1). Therefore, it is assumed that the NO3− leaching measured in each season was contemporaneous to that season. Net drainage (mm) was set as the daily sum of gains and losses of weight of the drainage tank. For the 2017/2018 season, soil solution samples at 90 cm depth were collected approximately weekly from November to June, with a maximum in-between sampling period of 14 days, a minimum of 2 days, and mean sampling occurring every 7.6 days. The soil became too dry by the end of June, determining the end of our sampling period, as that was an indication that NO3− leaching was unlikely to occur after this date. In 2018/2019, soil solution samples were collected approximately weekly from November to June, with a maximum sample period of 14 days, a minimum sample period of 3 days, and a mean sample period of 7.2 days. Supplementary Figure S2 Identifies the sampling times. This is an adequate sampling frequency as interpolations of NO3− concentration data from soil suction samplers between intervals of 9 to 21 days have been found to have a negligible effect on nitrate drainage calculations (Vogeler et al., 2020). The beginning and end of the study period were matched to 2017/2018. Soil solution collection consisted of setting up vacuum pumps approximately 18 h before the desired collection time and applying 300 hPa of suction to the soil solution samplers. There is no consensus as to the optimal degree of suction to avoid sampling water from mesopores (Weihermüller et al., 2007). We used a lower degree of vacuum than typical measurements from ceramic suction samplers (e.g., 600 to 700 hPa; Vogeler et al., 2020; Zotarelli et al., 2007) to reduce the potential of over-sampling soil pore water. This was followed by sub-sampling 30 ml of soil solution from the lysimeter water sampling system. The samples were transported in coolers and placed in a freezer within 24 h of turning on the vacuum pumps. Colorimetric analyses using a discrete autoanalyzer (SmartChem 140, Westco Scientific, Brookfield, Connecticut, United States) were used to determine the concentration of NO3− in the soil solution samples, as described in Elliott and Henry (2009). Readers are referred to Lapierre et al. (2022) and Brown et al. (2021) for a detailed description of the soil solution sampling system of the lysimeter, drainage measurements, and nitrate leaching calculations.
Statistical analysis
Mean comparisons were performed with a two-way analysis of variance (ANOVA) designed for a randomized block trial with two soil warming levels (+H and -H) and two soil types (SIL and LS). The three blocks (lysimeter nests) entered the statistical model as a random factor. The statistical analysis targeted the following periods: (i) from November 1 until December 14 (fall); (ii) from December 15 until March 22 (over-winter); (iii) from March 23 until May 18 (spring-thaw); (iv) from May 19 until June 30 (post-planting); and (v) total from November 1 to June 30. The same sampling periods were considered in both the 2017/2018 and 2018/2019 seasons. The assumptions of normality and homogeneity of variances were tested with a Shapiro-Wilk and Levene’s test, respectively, prior to the ANOVA test. For all statistical tests, significance was determined when p ≤ 0.05. All statistical calculations were performed on SPSS ver. 27 (IBM® SPSS® Statistics).
Results
Weather and cover crop growth
Climatic conditions for this research were described in detail in the complementary study by Lapierre et al. (2022) and are further presented in Supplementary Table S2. Briefly, 2017/2018 was subjected to slightly colder (1.9 vs. 2.4°C air temperature averages) and wetter (640 vs. 586 mm of total precipitation from November to June) climate than the 1988–2018 long-term average for this site (ECCC, 2020). The fall (day of year [DOY] 305–348), overwinter (DOY 349–81), spring-thaw (DOY 82–138), and post-planting (DOY 139-181) periods of 2017/2018 presented air temperature averages of −0.4, −6.2, 5.3, and 17.8°C and total precipitation of 114, 239, 216, and 71 mm, respectively (Lapierre et al., 2022). The weather in 2018/2019 was also colder (1.3 vs. 2.4°C) and wetter (798 vs. 586 mm) than normal, and higher total precipitation occurred compared to 2017/2018 (798 vs. 640 mm). For 2018/2019, air temperature averages of −1.4, −6.1, 5.3, and 15.5°C, and total precipitation of 127, 311, 227, and 133 mm occurred for the fall, overwinter, spring-thaw, and post-planting periods, respectively. Cover crop performance was distinct between 2017/2018 and 2018/2019. Whereas the mixture of cover crops seeded after wheat performed well in 2017/2018, the same did not occur for the mixture of cover crops under seeded into corn in 2018/2019, which presented poor establishment and performance (Lapierre et al., 2022).
Soil temperature and volumetric water content
Soil temperature and water dynamics were significantly affected by the artificial heating in 2017/2018 and 2018/2019 (Figure 1; Supplementary Table S2). Soil temperature averages at 5 and 10 cm from +H were consistently higher than -H, influenced by the continuous operation of the infrared heaters between late fall/early winter and mid-spring (Figure 1). Soil temperature at 5 and 10 cm were also affected by soil type (Figure 1; Supplementary Table S2). Overall, LS presented higher soil temperatures than SIL during the PP and ST periods of both years. Soil heating affected water dynamics in soil in a similar pattern for 2017/2018 and 2018/2019 (Figure 1; Supplementary Table S2). The sensors measuring volumetric water content (VWC) detect only liquid water content and as such periods of sub-freezing soil temperatures corresponded to decreases in VWC. During the W and ST periods of 2017/2018, +H was 1.4- and 1.1-times wetter (i.e., had higher VWC in liquid form) than -H at 5 cm, respectively. At 10 cm, +H was 1.3-times wetter than -H during the winter. During the winter of 2018/2019 the lysimeters also experienced statistically significant differences due to soil heating and +H was wetter than -H at 5 and 10 cm. The soil type effect on VWC was clear, with SIL wetter than LS in all periods of 2017/2018 and 2018/2019, and for both the 5 and 10 cm soil depths (Figure 1; Supplementary Table S2)
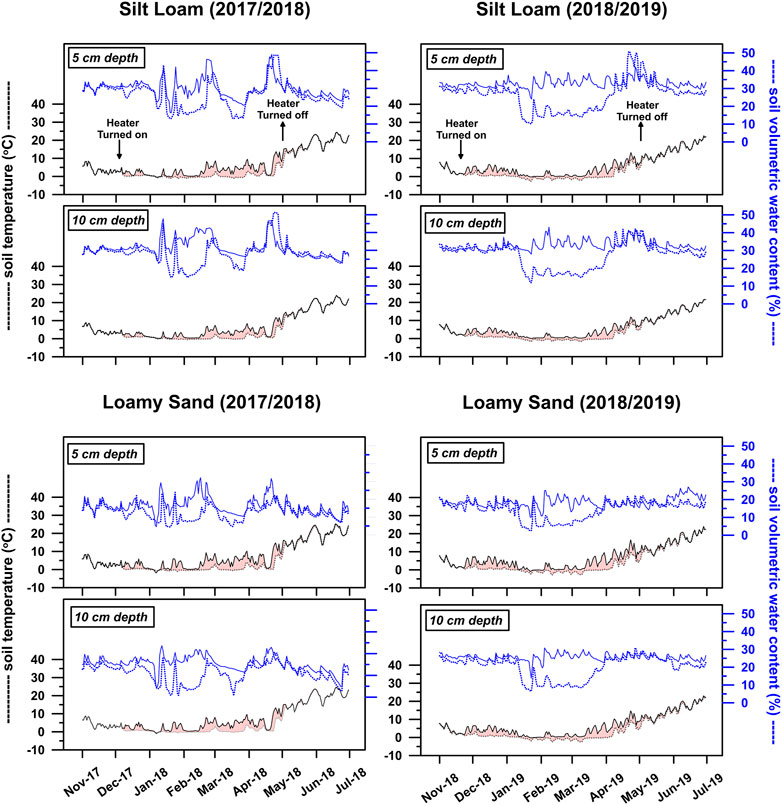
FIGURE 1. Soil temperature and volumetric water content daily averages at 5 and 10 cm depths of the silt loam and loamy sand soils. Solid lines, heated lysimeter; dashed lines, non-heated lysimeter. The red shade illustrates differences in soil temperature between heated and non-heated lysimeters.
Drainage responses to soil type and heating
Drainage was affected by treatments in similar ways in 2017/2018 and 2018/2019 (Table 1; Supplementary Figure S2). The significantly higher temperature and soil VWC induced by the infrared heaters (Figure 1), translated into 2.1- and 3.2-times higher drainage in +H than -H in the overwinter period of 2017/2018 and 2018/2019, respectively (Table 1). The higher overwinter drainage in +H influenced the total drainage, which was also the highest for + H in both years for SIL and LS. Soil type affected drainage during the fall and post-planting period of both years (Table 1). In the fall of 2017/2018, both soils were drier than the control -H (data not shown), but LS gained 1.6-times more water from the lower boundary control than SIL. For the fall of 2018/2019, while SIL drained 4.5 mm (averaged across heating regimes), LS was drier than the reference soil and as such gained water from the lower boundary control. The soil effect on drainage during the post planting period was similar in both years, with higher values for LS than SIL (65 vs. 12 and 68 vs. 34 mm for 2017/2018 and 2018/2019, respectively; averaged across soil heating regimes) (Table 1).
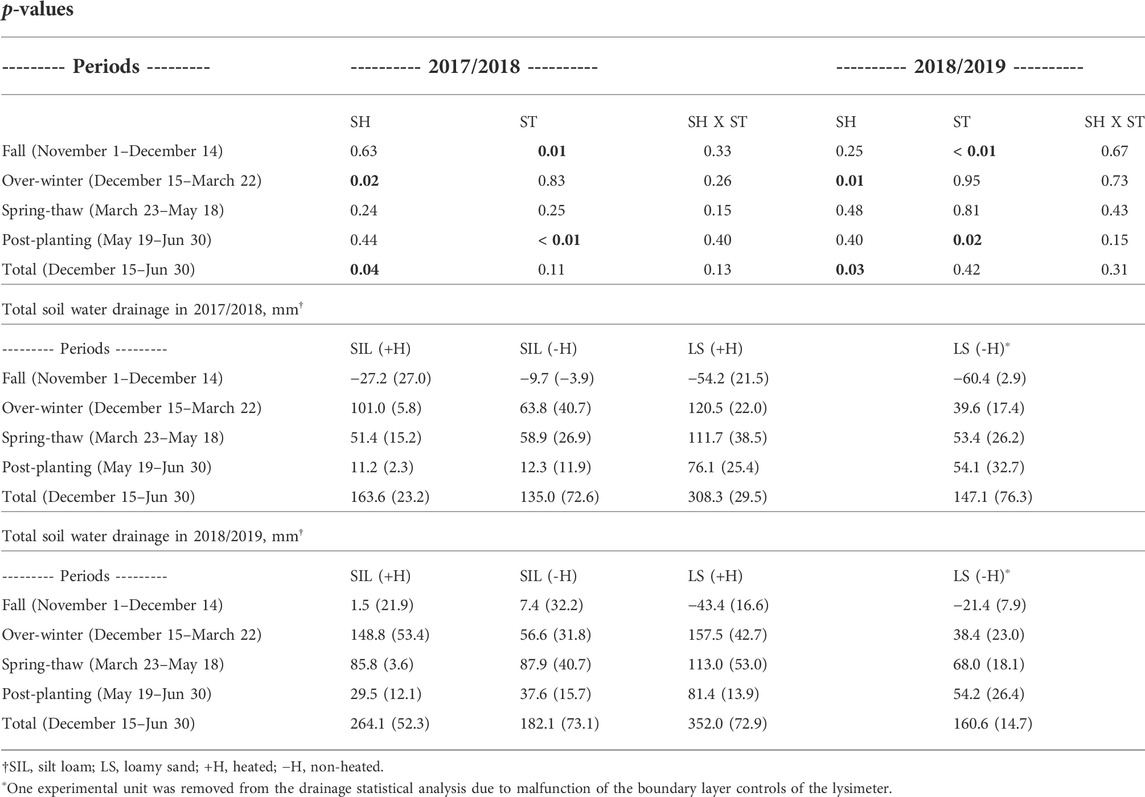
TABLE 1. p-values of the two-way ANOVA used to test main effects (SH, soil heating; ST, soil type) and the interaction SH × ST on the total drainage during periods of the 2017/2018 and 2018/2019 seasons, and respective totals (standard deviation is shown in parenthesis). Bolded values indicate p ≤ 0.05.
Nitrate concentration in drainage water
Treatment effects on nitrate concentration at 90 cm were not as clear as treatment effects on drainage, soil temperature and VWC. Soil heating did not have an effect for any period studied over the 2 years (Table 2). Soil type had an effect for fall in both years and for the fall, overwinter, and post planting periods in 2018/2019. For 2017/2018, there was an interaction between heating and soil type for the fall period, and a trend in SH x ST occurred overwinter (p >0.1 and <0.2), but not for the overall period (Table 2). The interaction effect meant + H LS had higher NO3− concentrations than + H SIL for the fall period in 2017/2018 and a similar trend was seen for the overwinter period (marginally significant). However, both soils presented similar concentrations for -H and there were no differences between +H and -H within a soil type (Table 2). In general, the 2018/2019 season presented higher NO3− concentrations than 2017/2018, and LS showed higher (or a trend for higher) NO3− concentration than SIL during the fall, overwinter, and post planting periods, translating in 2.6-times higher values for LS over the whole period, averaged across treatments (Table 2).
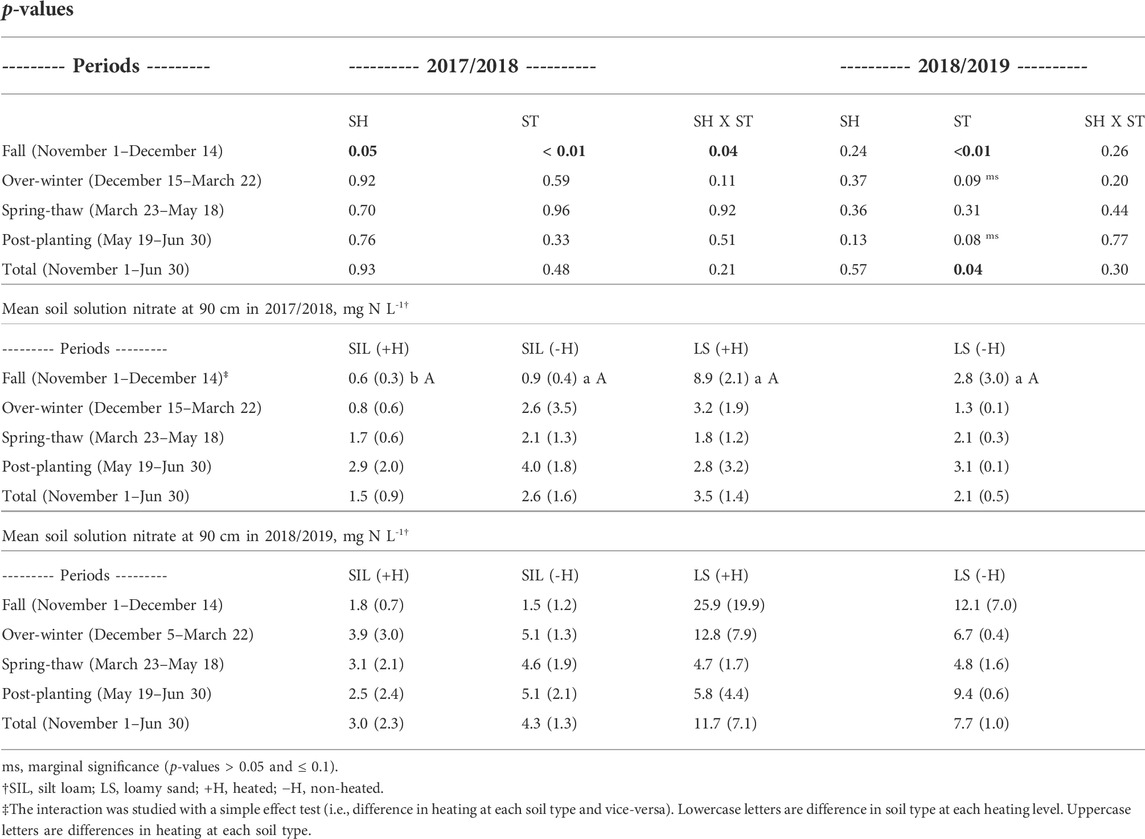
TABLE 2. p-values of the two-way ANOVA used to test main effects (SH, soil heating; ST, soil type) and the interaction SH × ST on the nitrate concentration at 90 cm, for periods of the 2017/2018 and 2018/2019 seasons, and respective averages (standard deviation is shown in parenthesis). Bolded values indicate p ≤ 0.05.
Nitrate leaching
Interannual variation in NO3− leaching occurred and whereas 2017/2018—the year cover crops performed well– presented lower losses (4.1 kg N ha−1 averaged across all treatments)- high losses occurred in 2018/2019 (14.5 kg N ha−1) (Figure 2; Table 3). Soil type only had a marginally significant effect in 2017/2018 for the post-planting period when LS had ∼2.9-times higher NO3− leaching than SIL, but that did not translate into differences over the whole study period (Table 3). In 2018/2019, ST affected nitrate leaching during fall, overwinter, and post-planting periods, with LS presenting higher losses than SIL during the overwinter and post-planting periods (Table 3). Soil heating affected NO3− leaching only overwinter in 2018/2019, a year with higher precipitation than 2017/2018 and poor cover crop performance, with +H presenting 2.6-times higher losses than -H. However, there was large spatial variation, with only a trend (p > 0.10 and ≤ 0.20) for SH, ST, and SH x ST for the NO3− leaching average of the total 2018/2019 period (Table 3).
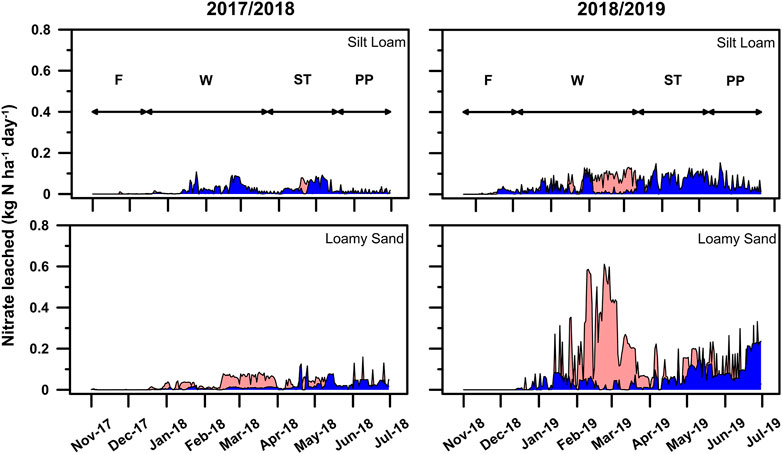
FIGURE 2. Daily nitrate leaching values averaged per heating regime (with/without heating) and soil type (silt loam and loamy sand) for the 2017/2018 and 2018/2019 seasons. Lysimeters without heating are represented by a solid black line and blue shade, and artificially heated lysimeters by a solid line and light red shade. Black horizontal lines delineate the analysis periods: F, fall; W, winter; ST, spring-thaw and PP, post-planting periods. Cover crops established and performed poorly in 2018/2019.
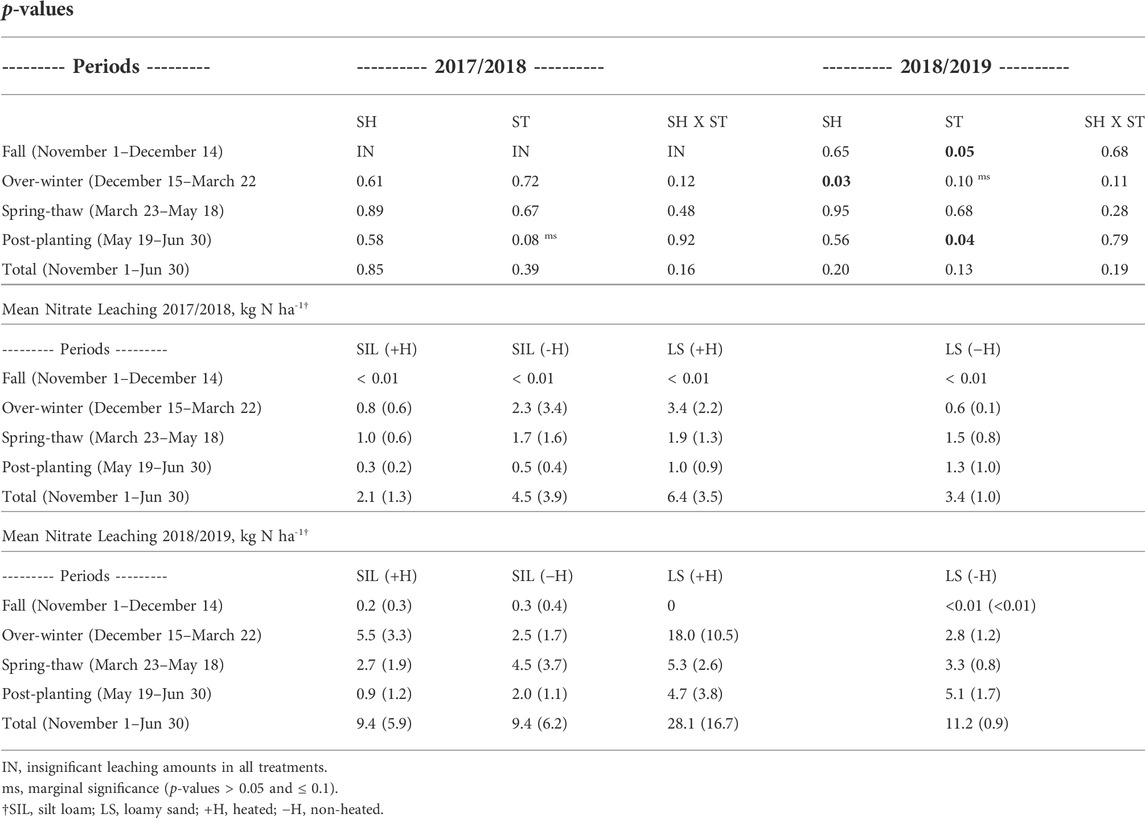
TABLE 3. p-values of the two-way ANOVA used to test main effects (SH, soil heating; ST, soil type) and the interaction SH × ST on the nitrate leaching, for periods of the 2017/2018 and 2018/2019 seasons, and respective averages (standard deviation is shown in parenthesis). Bolded values indicate p ≤ 0.05.
Discussion
Winter warming affected drainage, soil temperature, and soil water content
The infrared ceramic heaters were effective at simulating soil warming under field conditions, aligning with previous research that used such infrastructure (Kimball et al., 2008; Meromy et al., 2015). In our study, the heaters remained active continuously from late fall until late-spring and, as such, simulated a constant regime of increased air temperatures. The heating effect on soil conditions was consistent across years. Not surprisingly, soils under an active ceramic heater presented significantly higher soil temperature than soils under natural conditions. This translated into higher VWC and drainage for +H than for –H, particularly during the over-winter period. The difference in soil VWC between +H and –H was in part affected by differences in dieletric permittivity between liquid and solid water, which affects time domain reflectometry VWC measurements (Jones et al., 2002; He et al., 2021), and indicated higher liquid water content for +H. Contrary to our initial hypothesis, the heating regime adopted in our study did not promote colder soils during the winter, a phenomenon previously described in the literature and associated with the insulation properties of the snow pack (Brown and DeGaetano, 2011; Zhou et al., 2017; Halim and Thomas, 2018). Future research on this topic should evaluate the effect of other heating regimes on soil properties during the winter, such as intermittent heating, which could promote snow melting, followed by subsequent freezing which would penetrate deeper into the soil due to lack of snow cover. This could result in colder soils during the winter (Brown and DeGaetano, 2011; Zhou et al., 2017) and more frequent freeze-thaw cycles. Colder soils with more frequent freeze-thaw cycles could promote higher mineralization of organic N (Christensen and Christensen, 1991; Herrmann and Witter, 2002), microbial cytoplasmatic release (Schimel et al., 2007), and disruption of soil aggregates previously protected by soil organic matter (Oztas and Fayetorbay, 2003)—all factors with potential to affect soil nitrate leaching.
Treatment effects on nitrate concentrations were inconsistent across years
The soil type and warming effect on soil nitrate concentration was not as consistent as the drainage, soil temperature, and soil VWC results. For 2017/2018, the year cover crops established and performed well, there was a significant interaction between soil type and the heating regime for the fall, and a trend effect for the overwinter period (Table 2). Within warmed lysimeters, LS soils presented higher NO3− concentrations at 90 cm than SIL. For our study, the significant effect in the fall and the overwinter trend did not translate to different averages over the whole period. However, differences over winter can impact year-round NO3− leaching totals, as this period has been identified as a potential ‘hot moment’ for NO3− leaching losses (Bowles et al., 2018)). No difference in NO3− concentrations at 90 cm occurred between +H and –H within SIL lysimeters in any of the periods of 2017/2018, evidence that the artificial heating mostly affected the coarser soil. A soil warming effect on soil NO3−concentrations has been reported in the literature (Groffman et al., 2001; Joseph and Henry, 2008; Patil et al., 2010; Sahoo, 2022). This, combined with the greater likelihood of N adsorption by finer-textured soils due to the chemical properties of clays and their greater surface area for ion exchange (Lazaratou et al., 2020; Nouri et al., 2022), likely lead to the higher NO3− concentrations for LS. In 2018/2019, the year with poor cover crop performance, only soil type affected NO3− concentrations at 90 cm and LS presented averages over the whole period that were 2.6- times higher than SIL across heating regimes (Table 2). Interestingly, NO3− concentrations at 90 cm in 2018/2019 were 3.5- and 1.9- times higher than the averages reported for 2017/2018 for LS and SIL averaged across heating regimes, respectively. Previous research has highlighted the potential of catch crops to promote lower post-harvest soil nitrate concentrations (Tonitto et al., 2006; De Notaris et al., 2018; Vogeler et al., 2019; Zhao et al., 2020). Thus, the difference in cover crops performance between 2017/2018 and 2018/2019 likely explains the much higher NO3− concentration averages that occurred in 2018/2019. Comparatively, the LS soil without cover crops presented an average concentration of 13.6 mg N L−1 for 2017/2018 (Lapierre et al., 2022), which was much higher than the 3.5 and 2.1 mg L−1 averages reported here for heated and non-heated LS lysimeters with cover cropping, respectively. We speculate that a heated LS soil without cover crops could have experienced even higher soil nitrate concentrations, information that needs to be confirmed in future research on this topic.
There is the potential that the inconsistent results were an artifact of uncertainties associated with the soil water sampling using porous ceramic cups. Uncertainty exists for all methods of soil solution sampling (Weihermüller et al., 2007; Fares et al., 2009; Wey et al., 2021). Ceramic soil solution samplers have the potential to over- or underestimate soil nitrate concentrations by either missing macropore flow or by drawing excess soil water from the mesopores (Allaire et al., 2009; Singh et al., 2018). The solution samplers were installed in the Ck layer, which had fairly homogeneous porosity for both soil types (Brown et al., 2021), and thus likely had proper hydraulic contact with the soil for a representative sampling. Suction soil solution samplers make point samples, which is often a source of uncertainty in field studies due to spatial heterogeneity (Lilburne et al., 2012). This uncertainty is likely reduced in lysimeters, due to the constrained and relatively smaller volume of soil sampled compared to studies in areas > 1 m2. It is also unlikely that the lack of a clear relationship between soil heating and NO3− concentrations were due to any lag in transport from the surface to the 90 cm sampling depth since transit times through the soil columns were short (Supplementary Table S1).
Cover crops can reduce adverse nitrate leaching effects from winter warming
The design adopted in this research, with continuous warming promoted by the infrared heaters, enabled identification of two potential adverse consequences from winter warming to soil NO3− leaching: (i) increased over-winter drainage, leading to an overall increased drainage over the whole season; and (ii) increased over-winter NO3− concentration at 90 cm in the LS soil. These two factors are key controllers of soil NO3− leaching. The drainage and NO3− concentration at 90 cm in our study exhibited high spatial variation—common in studies evaluating soil nitrogen dynamics (Kurunc et al., 2011; Chadwick et al., 2014; Machado et al., 2021b). Variability among subjects is a key factor influencing statistical power (Vandenbygaart and Allen, 2011; Kravchenko and Robertson, 2015), and might have reduced the sensitivity of the statistical test to detect significant differences (i.e., increased risks of type II statistical error). Thus, although the absolute difference between LS and SIL was large (i.e., 10.3 kg N ha−1 averaged across heating regimes), only a trend (p >0.10 and <0.20) occurred for the soil type effect on NO3− leaching in 2018/2019. Similarly, a large difference (i.e., 16.9 kg N ha−1; Table 3) occurred between +H and –H in LS lysimeters in 2018/2019, which translated into a trend effect for the interaction between soil heating and soil type on NO3− leaching. However, other observed large differences in drainage for SIL and 2017/2018 did not translate into differences in NO3− leaching because NO3− concentrations trends added variability or trended in opposite direction (i.e., +H<-H).
Previous research at this site showed that cover crops can significantly reduce post-harvest soil NO3− leaching (Lapierre et al., 2022). The current study complements these findings by showing a trend effect (p >0.1 and <0.2) of the heating by soil type interaction on NO3− leaching, with +H trending higher than –H for LS soil. For example, consider the rates from 2017/2018, 6.4 and 3.4 kg N ha−1 leached from heated and non-heated LS lysimeters with cover crops, respectively, versus 12.3 kg N ha−1 from non-heated LS lysimeter without cover crops (Lapierre et al., 2022). A heated LS lysimeter without cover crops could have experienced even higher nitrate leaching than the 12.3 kg N ha−1 reported by Lapierre et al. (2022) for non-heated LS soil—information that needs to be evaluated in additional research on this topic. Besides, further research should simulate other potential consequence of global warming to soil NO3− leaching (e.g., extreme weather events). This could be achieved with ceramic infrared heaters under different operation regimes (e.g., intermittent heating).
Conclusion
In this study, ceramic heaters and weighing lysimeters were used to further our knowledge on NO3− leaching of agricultural rotations that include cover crops in response to winter warming and soil types. Results on the impact of simulated winter warming on NO3− leaching varied depending on soil type, cover crop establishment, and season. Top-down heating demonstrated potential to influence NO3− leaching by increasing overwinter drainage of SIL and LS soils. However, NO3− concentrations at 90 cm were only affected by winter heating for the LS soil. Overall, heating showed only a trend effect on NO3− leaching for the coarser soil type, despite consistently higher drainage associated with heating. This indicates effects on NO3− concentration either cancelled out the increased drainage effect or added variability to results making the detection of significant differences more difficult. Contrary to our initial hypothesis, the heating regime adopted in our study did not promote colder soils during the winter. This result provides new insight for winter warming experiments as well as perspectives and directions for future research on this topic. We suggest different heating regimes to simulate extreme weather freeze/thaw events need to be simulated under similar settings, to provide a comprehensive understanding of the potential consequences of winter warming on NO3− leaching from agriculture.
Data availability statement
The datasets presented in this study can be found in online repository. The names of the repository/repositories and accession number(s) can be found below: https://borealisdata.ca/dataset.xhtml?persistentId=doi:10.5683/SP/XUK5UJ.
Author contributions
JL, HH, and CW-R conceptualized the study. JL conducted field work, sample analysis, and calculations. PM expanded the data analysis. JL and PM wrote the manuscript. ZD and SB provided technical assistance for data analysis. SJ oversaw all field work related to lysimeter operation and functioning. AaB and AsB contributed technical expertise. CW-R acquired funding and led the research. All authors contributed to writing.
Funding
Funding for installation of the soil lysimeter facility was provided by the Canada Foundation for Innovation and the Ontario Ministry of Research and Innovation (project 33143). Research funds were provided by Canada’s Natural Sciences and Engineering Research Council Strategic Grants Program (project STPGP 494224–16), the Ontario Ministry of Agriculture, Food and Rural Affairs through its Ontario Agri-Food Innovation Alliance program (project UofG 2015-2253) and Grain Farmers of Ontario (project C2016AG01).
Conflict of interest
The authors declare that the research was conducted in the absence of any commercial or financial relationships that could be construed as a potential conflict of interest.
Publisher’s note
All claims expressed in this article are solely those of the authors and do not necessarily represent those of their affiliated organizations, or those of the publisher, the editors and the reviewers. Any product that may be evaluated in this article, or claim that may be made by its manufacturer, is not guaranteed or endorsed by the publisher.
Supplementary material
The Supplementary Material for this article can be found online at: https://www.frontiersin.org/articles/10.3389/fenvs.2022.897221/full#supplementary-material
References
Abdalla, M., Hastings, A., Cheng, K., Yue, Q., Chadwick, D., Espenberg, M., et al. (2019). A critical review of the impacts of cover crops on nitrogen leaching, net greenhouse gas balance and crop productivity. Glob. Change Biol. 25 (8), 2530–2543. doi:10.1111/gcb.14644
Allaire, S. E., Roulier, S., and Cessna, A. J. (2009). Quantifying preferential flow in soils: A review of different techniques. J. Hydrol. 378 (1), 179–204. doi:10.1016/j.jhydrol.2009.08.013
Alonso-Ayuso, M., Gabriel, J. L., and Quemada, M. (2014). The kill date as a management tool for cover cropping success. PLoS ONE 9 (10), e109587. doi:10.1371/journal.pone.0109587
Bingham, M. A., and Biondini, M. (2011). Nitrate leaching as a function of plant community richness and composition, and the scaling of soil nutrients, in a restored temperate grassland. Plant Ecol. 212 (3), 413–422. doi:10.1007/s11258-010-9832-8
Boswell, E. P., Balster, N. J., Bajcz, A. W., and Thompson, A. M. (2020). Soil aggregation returns to a set point despite seasonal response to snow manipulation. Geoderma 357, 113954. doi:10.1016/j.geoderma.2019.113954
Bowles, T. M., Atallah, S. S., Campbell, E. E., Gaudin, A. C. M., Wieder, W. R., and Grandy, A. S. (2018). Addressing agricultural nitrogen losses in a changing climate. Nat. Sustain. 1 (8), 399–408. doi:10.1038/s41893-018-0106-0
Brown, P. J., and DeGaetano, A. T. (2011). A paradox of cooling winter soil surface temperatures in a warming northeastern United States. Agric. For. Meteorol. 151 (7), 947–956. doi:10.1016/j.agrformet.2011.02.014
Brown, S., Wagner-Riddle, C., Debruyn, Z., Jordan, S., Berg, A., Ambadan, J. T., et al. (2021). Assessing variability of soil water balance components measured at a new lysimeter facility dedicated to the study of soil ecosystem services. J. Hydrol. 603, 127037. doi:10.1016/j.jhydrol.2021.127037
Carey, P. L., Cameron, K. C., Di, H. J., Edwards, G. R., and Chapman, D. F. (2016). Sowing a winter catch crop can reduce nitrate leaching losses from winter-applied urine under simulated forage grazing: A lysimeter study. Soil Use Manag. 32 (3), 329–337. doi:10.1111/sum.12276
Chadwick, D. R., Cardenas, L., Misselbrook, T. H., Smith, K. A., Rees, R. M., Watson, C. J., et al. (2014). Optimizing chamber methods for measuring nitrous oxide emissions from plot‐based agricultural experiments. Eur. J. Soil Sci. 65 (2), 295–307. doi:10.1111/ejss.12117
Chantigny, M. H., Bittman, S., Larney, F. J., Lapen, D., Hunt, D. E., Goyer, C., et al. (2019). A multi-region study reveals high overwinter loss of fall-applied reactive nitrogen in cold and frozen soils. Can. J. Soil Sci. 99 (2), 126–135. doi:10.1139/cjss-2018-0151
Christensen, S., and Christensen, B. T. (1991). Organic matter available for denitrification in different soil fractions: effect of freeze/thaw cycles and straw disposal. Eur. J. Soil Sci. 42 (4), 637–647. doi:10.1111/j.1365-2389.1991.tb00110.x
Constantin, J., Beaudoin, N., Laurent, F., Cohan, J. P., Duyme, F., and Mary, B. (2011). Cumulative effects of catch crops on nitrogen uptake, leaching and net mineralization. Plant Soil 341 (1-2), 137–154. doi:10.1007/s11104-010-0630-9
Dalias, P., Anderson, J. M., Bottner, P., and Coûteaux, M. M. (2002). Temperature responses of net nitrogen mineralization and nitrification in conifer forest soils incubated under standard laboratory conditions. Soil Biol. Biochem. 34 (5), 691–701. doi:10.1016/S0038-0717(01)00234-6
De Notaris, C., Rasmussen, J., Sørensen, P., and Olesen, J. E. (2018). Nitrogen leaching: A crop rotation perspective on the effect of N surplus, field management and use of catch crops. Agric. Ecosyst. Environ. 255, 1–11. doi:10.1016/j.agee.2017.12.009
de Wit, H. A., Hindar, A., and Hole, L. (2008). Winter climate affects long-term trends in stream water nitrate in acid-sensitive catchments in southern Norway. Hydrol. Earth Syst. Sci. 12, 393–403. doi:10.5194/hess-12-393-2008
Drury, C. F., Tan, C. S., Welacky, T. W., Reynolds, W. D., Zhang, T. Q., Oloya, T. O., et al. (2014). Reducing nitrate loss in tile drainage water with cover crops and water-table management systems. J. Environ. Qual. 43, 587–598. doi:10.2134/jeq2012.0495
Durán, J., Rodriguez, A., Morse, J. L., and Groffman, P. M. (2013). Winter climate change effects on soil C and N cycles in urban grasslands. Glob. Chang. Biol. 19, 2826–2837. doi:10.1111/gcb.12238
(ECCC) Environment and Climate Change Canada (2020). Historical climate data. Available at: weather.gc.ca(accessed June, 2020). (
Elliott, A. C., and Henry, H. A. (2009). Freeze–thaw cycle amplitude and freezing rate effects on extractable nitrogen in a temperate old field soil. Biol. Fertil. Soils 45 (5), 469–476. doi:10.1007/s00374-009-0356-0
Endo, A., Kato, K., and Sarker, B. C. (2018). Leaching characteristics of nitrate nitrogen in an apple orchard andosol under significant snow accumulation. Geoderma 319, 24–33. doi:10.1016/j.geoderma.2018.01.002
Fares, A., Deb, S. K., and Fares, S. (2009). Review of vadose zone soil solution sampling techniques. Environ. Rev. 17, 215–234. doi:10.1139/A09-010
Gaudin, A. C. M., Tolhurst, T. N., Ker, A. P., Janovicek, K., Tortora, C., Martin, R. C., et al. (2015). Increasing crop diversity mitigates weather variations and improves yield stability. PLoS ONE 10 (2), e0113261–20. doi:10.1371/journal.pone.0113261
Groffman, P. M., Driscoll, C. T., Fahey, T. J., Hardy, J. P., Fitzhugh, R. D., and Tierney, G. L. (2001). Colder soils in a warmer world: A snow manipulation study in a northern hardwood forest ecosystem. Biogeochemistry 56, 135–150. doi:10.1023/A:1013039830323
Groffman, P. M., Hardy, J. P., Fashu-Kanu, S., Driscoll, C. T., Cleavitt, N. L., Fahey, T. J., et al. (2011). Snow depth, soil freezing and nitrogen cycling in a northern hardwood forest landscape. Biogeochemistry 102 (1), 223–238. doi:10.1007/s10533-010-9436-3
Halim, M. A., and Thomas, S. C. (2018). A proxy-year analysis shows reduced soil temperatures with climate warming in boreal forest. Sci. Rep. 8 (1), 16859–9. doi:10.1038/s41598-018-35213-w
Hansen, E. M., Nielsen, S., Labouriau, R., Cichota, R., Olesen, J. E., Thomsen, I. K., et al. (2020). Nitrate leaching from suction cup data: Influence of method of drainage calculation and concentration interpolation. J. Environ. Qual. 49 (2), 440–449. doi:10.1002/jeq2.20020
He, H., Aogu, K., Li, M., Xu, J., Sheng, W., Jones, S. B., et al. (2021). A review of time domain reflectometry (TDR) applications in porous media. Adv. Agron. 168, 83–155. doi:10.1016/bs.agron.2021.02.003
Henry, H. A. L. (2007). Soil freeze-thaw cycle experiments: Trends, methodological weaknesses and suggested improvements. Soil Biol. Biochem. 39, 977–986. doi:10.1016/j.soilbio.2006.11.017
Herrmann, A., and Witter, E. (2002). Sources of C and N contributing to the flush in mineralization upon freeze–thaw cycles in soils. Soil Biol. Biochem. 34 (10), 1495–1505. doi:10.1016/S0038-0717(02)00121-9
Hertel, C., and von Unold, G. (2014). “Third-generation lysimeters: Scientific engineered monitoring systems,” in Environmental science and engineering (subseries: Environmental science). doi:10.1007/978-3-319-01017-5_9
Hooker, K. V., Coxon, C. E., Hackett, R., Kirwan, L. E., O’Keeffe, E., and Richards, K. G. (2008). Evaluation of cover crop and reduced cultivation for reducing nitrate leaching in Ireland. J. Environ. Qual. 37, 138–145. doi:10.2134/jeq2006.0547
Jones, S. B., Wraith, J. M., and Or, D. (2002). Time domain reflectometry measurement principles and applications. Hydrol. Process. 16 (1), 141–153. doi:10.1002/hyp.513
Joseph, G., and Henry, H. A. L. (2008). Soil nitrogen leaching losses in response to freeze-thaw cycles and pulsed warming in a temperate old field. Soil Biol. Biochem. 40, 1947–1953. doi:10.1016/j.soilbio.2008.04.007
Kimball, B. A., Conley, M. M., Wang, S., Lin, X., Lou, C., Morgan, J., et al. (2008). Infrared heater arrays for warming ecosystem field plots. Glob. Chang. Biol. 14, 309–320. doi:10.1111/j.1365-2486.2007.01486.x
Kirschke, T., Spott, O., and Vetterlein, D. (2019). Impact of urease and nitrification inhibitor on NH4+ and NO3− dynamic in soil after urea spring application under field conditions evaluated by soil extraction and soil solution sampling. J. Plant Nutr. Soil Sci. 182 (3), 441–450. doi:10.1002/jpln.201800513
Kramberger, B., Gselman, A., Janzekovic, M., Kaligaric, M., and Bracko, B. (2009). Effects of cover crops on soil mineral nitrogen and on the yield and nitrogen content of maize. Eur. J. Agron. 31, 103–109. doi:10.1016/j.eja.2009.05.006
Kramberger, B., Gselman, A., Kristl, J., Lešnik, M., Šuštar, V., Muršec, M., et al. (2014). Winter cover crop: The effects of grass-clover mixture proportion and biomass management on maize and the apparent residual N in the soil. Eur. J. Agron. 55, 63–71. doi:10.1016/j.eja.2014.01.001
Kravchenko, A. N., and Robertson, G. P. (2015). Statistical challenges in analyses of chamber‐based soil CO2 and N2O emissions data. Soil Sci. Soc. Am. J. 79 (1), 200–211. doi:10.2136/sssaj2014.08.0325
Kurunc, A., Ersahin, S., Uz, B. Y., Sonmez, N. K., Uz, I., Kaman, H., et al. (2011). Identification of nitrate leaching hot spots in a large area with contrasting soil texture and management. Agric. Water Manag. 98 (6), 1013–1019. doi:10.1016/j.agwat.2011.01.010
Langelier, M., Chantigny, M. H., Pageau, D., and Vanasse, A. (2021). Nitrogen-15 labelling and tracing techniques reveal cover crops transfer more fertilizer N to the soil reserve than to the subsequent crop. Agric. Ecosyst. Environ. 313, 107359. doi:10.1016/j.agee.2021.107359
Lapierre, J., Machado, P. V. F., Debruyn, Z., Brown, S. E., Jordan, S., Berg, A., et al. (2022). Cover crop mixtures: A powerful strategy to reduce post-harvest surplus of soil nitrate and leaching. Agric. Ecosyst. Environ. 325, 107750. doi:10.1016/j.agee.2021.107750
Lazaratou, C., Vayenas, D., and Papoulis, D. (2020). The role of clays, clay minerals and clay-based materials for nitrate removal from water systems: A review. Appl. Clay Sci. 185, 105377. doi:10.1016/j.clay.2019.105377
Libby, M. D., VanderZaag, A. C., Gregorich, E. G., and Wagner-Riddle, C. (2020). An improved laboratory method shows that freezing intensity increases N2O emissions. Can. J. Soil Sci. 100 (2), 1–14. doi:10.1139/cjss-2019-0073
Lilburne, L., Carrick, S., Webb, T., and Moir, J. (2012). Computer-based evaluation of methods to sample nitrate leached from grazed pasture. Soil Use Manag. 28 (1), 19–26. doi:10.1111/j.1475-2743.2011.00378.x
Machado, P. V. F., Farrell, R. E., Bell, G., Taveira, C. J., Congreves, K. A., Voroney, R. P., et al. (2020). Crop residues contribute minimally to spring-thaw nitrous oxide emissions under contrasting tillage and crop rotations. Soil Biol. Biochem. 152, 108057. doi:10.1016/j.soilbio.2020.108057
Machado, P. V. F., Farrell, R., Deen, W., Voroney, P., Congreves, K., and Wagner-Riddle, C. (2021a). Contribution of crop residue, soil, and fertilizer nitrogen to nitrous oxide emissions varies with long-term crop rotation and tillage. Sci. Total Environ. 767, 145107. doi:10.1016/j.scitotenv.2021.145107
Machado, P. V. F., Farrell, R. E., and Wagner-Riddle, C. (2021b). Spatial variation of nitrous oxide fluxes during growing and non-growing seasons at a location subjected to seasonally frozen soils. Can. J. Soil Sci. 101 (3), 555–564. doi:10.1139/cjss-2021-0003
Meromy, L., Molotch, N. P., Williams, M. W., Musselman, K. N., and Kueppers, L. M. (2015). Snowpack-climate manipulation using infrared heaters in subalpine forests of the Southern Rocky Mountains, USA. Agric. For. Meteorol. 203, 142–157. doi:10.1016/j.agrformet.2014.12.015
Nouri, A., Lukas, S., Singh, S., Singh, S., and Machado, S. (2022). When do cover crops reduce nitrate leaching? A global meta-analysis. Glob. Change Biol. 28 (15), 4736–4749. doi:10.1111/gcb.16269
OMAFRA (2017). 2016 field crop Statistics. Available at: http://www.omafra.gov.on.ca/english/stats/crops/. (Accessed October 31, 2016).
Oztas, T., and Fayetorbay, F. (2003). Effect of freezing and thawing processes on soil aggregate stability. Catena 52 (1), 1–8. doi:10.1016/S0341-8162(02)00177-7
Patil, R. H., Laegdsmand, M., Olesen, J. E., and Porter, J. R. (2010). Effect of soil warming and rainfall patterns on soil N cycling in Northern Europe. Agric. Ecosyst. Environ. 139 (1-2), 195–205. doi:10.1016/j.agee.2010.08.002
Pawlick, A. A., Wagner-Riddle, C., Parkin, G. W., and Berg, A. A. (2019). Assessment of nitrification and urease inhibitors on nitrate leaching in corn (Zea mays L.). Can. J. Soil Sci. 99 (1), 80–91. doi:10.1139/cjss-2018-0110
Peel, M. C., Finlayson, B. L., and McMahon, T. A. (2007). Updated world map of the Köppen-Geiger climate classification. Hydrol. Earth Syst. Sci. 11 (5), 1633–1644. doi:10.5194/hess-11-1633-2007
Pütz, T., Kiese, R., Wohlschlaeger, U., Groh, J., Rupp, H., Zacharias, S., et al. (2016). TERENO-SOILCan: A lysimeter-network in Germany observing soil processes and plant diversity influenced by climate change. Environ. Earth Sci. 75, 1242–1256. doi:10.1007/s12665-016-6031-5
Qian, B., Gregorich, E. G., Gameda, S., Hopkins, D. W., and Wang, X. L. (2011). Observed soil temperature trends associated with climate change in Canada. J. Geophys. Res. 116 (D2), D02106. doi:10.1029/2010JD015012
Rosario-Lebron, A., Leslie, A. W., Yurchak, V. L., Chen, G., and Hooks, C. R. R. (2019). Can winter cover crop termination practices impact weed suppression, soil moisture, and yield in no-till soybean [Glycine max (L.) Merr.]? Crop Prot. 116, 132–141. doi:10.1016/j.cropro.2018.10.020
Sahoo, M. (2022). Winter soil temperature and its effect on soil nitrate status: A support vector regression-based approach on the projected impacts. Catena 211, 105958. doi:10.1016/j.catena.2021.105958
Schimel, J., Balser, T. C., and Wallenstein, M. (2007). Microbial stress‐response physiology and its implications for ecosystem function. Ecology 88 (6), 1386–1394. doi:10.1890/06-0219
Sharratt, B. S. (1993). Freeze-thaw and winter temperature of agricultural soils in interior Alaska. Cold Regions Sci. Technol. 22, 105–111. doi:10.1016/0165-232X(93)90049-E
Shepard, M. A., Davies, D. B., and Johnson, P. A. (1993). Minimizing nitrate losses from arable soils. Soil Use Manag. 9 (3), 94–98. doi:10.1111/j.1475-2743.1993.tb00936.x
Singh, G., Kaur, G., Williard, K., Schoonover, J., and Kang, J. (2018). Monitoring of water and solute transport in the vadose zone: A review. Vadose Zone J. 17 (1), 160058. doi:10.2136/vzj2016.07.0058
Song, Y., Zou, Y., Wang, G., and Yu, X. (2017). Altered soil carbon and nitrogen cycles due to the freeze-thaw effect: A meta-analysis. Soil Biol. Biochem. 109, 35–49. doi:10.1016/j.soilbio.2017.01.020
Starkloff, T., Larsbo, M., Stolte, J., Hessel, R., and Ritsema, C. (2017). Quantifying the impact of a succession of freezing-thawing cycles on the pore network of a silty clay loam and a loamy sand topsoil using X-ray tomography. Catena 156, 365–374. doi:10.1016/j.catena.2017.04.026
Tonitto, C., David, M. B., and Drinkwater, L. E. (2006). Replacing bare fallows with cover crops in fertilizer-intensive cropping systems: A meta-analysis of crop yield and N dynamics. Agric. Ecosyst. Environ., 112(1), 58–72. doi:10.1016/j.agee.2005.07.003
Toom, M., Talgre, L., Mäe, A., Tamm, S., Narits, L., Edesi, L., et al. (2019). Selecting winter cover crop species for northern climatic conditions. Biol. Agric. Hortic. 35 (4), 263–274. doi:10.1080/01448765.2019.1627908
Turner, M. M., and Henry, H. A. L. (2009). Interactive effects of warming and increased nitrogen deposition on 15N tracer retention in a temperate old field: seasonal trends. Glob. Chang. Biol. 15 (12), 2885–2893. doi:10.1111/j.1365-2486.2009.01881.x
van Kessel, C., Clough, T., and van Groenigen, J. W. (2009). Dissolved organic nitrogen: An overlooked pathway of nitrogen loss from agricultural systems? J. Environ. Qual. 38 (2), 393–401. doi:10.2134/jeq2008.0277
Vandenbygaart, A. J., and Allen, O. B. (2011). Experiment design to achieve desired statistical power. Can. J. Soil Sci. 91 (2), 309–310. doi:10.4141/cjss2010-068
Vogeler, I., Hansen, E. M., Thomsen, I. K., and Østergaard, H. S. (2019). Legumes in catch crop mixtures: Effects on nitrogen retention and availability, and leaching losses. J. Environ. Manage. 239, 324–332. doi:10.1016/j.jenvman.2019.03.077
Weihermüller, L., Siemens, J., Deurer, M., Knoblauch, S., Rupp, H., Göttlein, A., et al. (2007). In situ soil water extraction: A review. J. Environ. Qual. 36 (6), 1735–1748. doi:10.2134/jeq2007.0218
Wey, H., Hunkeler, D., Bischoff, W.-A., and Bünemann, E. K. (2021). Field-scale monitoring of nitrate leaching in agriculture: assessment of three methods. Environ. Monit. Assess. 194 (1), 4. doi:10.1007/s10661-021-09605-x
Yang, X. M., Drury, C. F., and Reeb, M. R. (2018). No-tillage had warmer over-winter soil temperatures than conventional tillage in a brookston clay loam soils in southwestern Ontario. Soil Sci. Soc. Am. J. 82 (2), 307–314. doi:10.2136/sssaj2017.05.0167
Zeitoun, R., Vandergeest, M., Vasava, H. B., Machado, P. V. F., Jordan, S., Parkin, G., et al. (2021). In-situ estimation of soil water retention curve in silt loam and loamy sand soils at different soil depths. Sensors 21 (2), 447. doi:10.3390/s21020447
Zhao, J., De Notaris, C., and Olesen, J. E. (2020). Autumn-based vegetation indices for estimating nitrate leaching during autumn and winter in arable cropping systems. Agric. Ecosyst. Environ. 290, 106786. doi:10.1016/j.agee.2019.106786
Zhou, Y., Berruti, F., Greenhalf, C., and Henry, H. A. (2017). Combined effects of biochar amendment, leguminous cover crop addition and snow removal on nitrogen leaching losses and nitrogen retention over winter and subsequent yield of a test crop (Eruca sativa L.). Soil Biol. Biochem. 114, 220–228. doi:10.1016/j.soilbio.2017.07.023
Keywords: water pollution, global warming, catch crops, N losses, snow insulation, soil water, NO3, ceramic heaters
Citation: Lapierre J, Machado PVF, Debruyn Z, Brown SE, Jordan S, Berg A, Biswas A, Henry HAL and Wagner-Riddle C (2022) Winter warming effects on soil nitrate leaching under cover crops: A field study using high-frequency weighing lysimeters. Front. Environ. Sci. 10:897221. doi: 10.3389/fenvs.2022.897221
Received: 15 March 2022; Accepted: 02 August 2022;
Published: 31 August 2022.
Edited by:
Alexandra Contosta, University of New Hampshire, United StatesReviewed by:
Haojie Liu, University of Rostock, GermanyHolger Rupp, Helmholtz Association of German Research Centres (HZ), Germany
Copyright © 2022 Lapierre, Machado, Debruyn, Brown, Jordan, Berg, Biswas, Henry and Wagner-Riddle. This is an open-access article distributed under the terms of the Creative Commons Attribution License (CC BY). The use, distribution or reproduction in other forums is permitted, provided the original author(s) and the copyright owner(s) are credited and that the original publication in this journal is cited, in accordance with accepted academic practice. No use, distribution or reproduction is permitted which does not comply with these terms.
*Correspondence: Claudia Wagner-Riddle, Y3dhZ25lcnJAdW9ndWVscGguY2E=