- 1Unité d'Enseignement et de Recherche en Hydrobiologie Appliquée (UERHA), Département de Biologie-Chimie, Institut Supérieur Pédagogique de Bukavu (ISP/Bukavu), Bukavu, Congo
- 2Centre de Recherche en Environnement et Géo-Ressources, Université Catholique de Bukavu (UCB), Bukavu, Congo
- 3Rwanda Wildlife, Kigali, Rwanda
- 4Department of Animal Ecology and Systematics, Justus Liebig University Giessen, Giessen, Germany
- 5Centre de Recherche Universitaire du Kivu, Institut Supérieur Pédagogique de Bukavu, Bukavu, Congo
- 6Department of Hydrobiology and Aquaculture, University of Kisangani, Kisangani, Congo
- 7Department of Biology, Mbarara University of Science and Technology, Mbarara, Uganda
- 8Institute of Physical Geography, Goethe University Frankfurt, Frankfurt, Germany
- 9Swiss Federal Institute of Technology Lausanne (EPFL), Limnology Center, Lausanne, Switzerland
- 10Eawag (Swiss Federal Institute of Aquatic Science and Technology), Surface Waters–Research and Management, Kastanienbaum, Switzerland
Hydroelectric power (HP) represents the main source of electricity in Africa, including the Democratic Republic of Congo. The demand for new dam construction is high, and major projects are currently progressing through planning and implementation stages. New HP dams should comply with both past and emerging environmental requirements. River systems need water to maintain hydraulic and ecological functions. Flow regime disturbance can prevent rivers from providing their ecosystem services and disrupt riparian communities. Most dammed rivers in Africa are understudied, however, in terms of their environmental flow requirements. This study analysed the hydrological regime and water quality of the Ruzizi River. The research investigated conditions of minimum water flow and hydropeaking at the Ruzizi I HP dam in terms of land management constraints and ecological impacts. According to Gumbel’s hydrological model, a discharge of ∼130 m3/s showed the longest return period (12 years) among the most recurrent flows. By contrast, the maximum recorded discharge of 143 m3/s showed a return time of 76 years. Any discharge between 46 and 120 m3/s could occur at any time within three years. The discharge–hydropower production relationship for the power plant provided a possible minimum environmental flow of 28 m3/s (i.e., 25%). Drinking water quality was assessed according to WHO water quality index (WQI) standards. Turbidity (i.e., total suspended solids) upstream and downstream of dams correlated strongly with rainfall (r = 0.8; n = 12) and land use. WQI values observed in excess of WHO drinking water standards indicate that the Ruzizi River is currently unsuitable for drinking water purposes.
Introduction
Human communities will experience increasing demand for freshwater and energy in the coming decades (Holland et al., 2015). Freshwater represents only ∼3% of global water reservoirs (Du Plessis, 2017) with much less of this accessible for human use. Dams provide irrigation, urban water supplies, navigation, hydropower, and flood control services (WCD, 2000). In 2020, hydroelectric power (HP) capacity accounted for ∼16% of the global electricity supply (Mekonnen and Hoekstra, 2011). This included a worldwide power production capacity of 1,330 Gigawatts and a total of 4,370 Terawatt-hours annual HP energy production (IHA, 2019). Global estimates indicate the number of large dams (over 15 m high or with a volume larger than three million m3) to be around ∼45,000 and the number of small dams and reservoirs to be in millions (Moore et al., 2010). In the Democratic Republic of Congo (DRC), 85% of domestic energy use derives from fuel wood (Seyler et al., 2010) and only ∼6% of the population has access to electricity (Kahindo, 2012). Government initiatives seek to use large rivers for HP production. The country currently hosts 51 HP dams, which are operational or under construction, and plans to build 13 more (Winemiller et al., 2016). Unfortunately, almost all of these dams have been built without environmental impact assessments, which could reconcile environmental imperatives with electricity production (Wang et al., 2012). Dombrowsky et al. (2014) describe the limited address of social and environmental impacts before and during the construction of the Ruzizi II HP dam. In their cost assessment of 245 dam projects in 65 countries between 1934 and 2007, Ansar et al. (2014) found that many of these dams did not meet expected economic returns. Poor planning and management thus may cause significant economic and environmental losses. Economic and political instability in developing countries often prevents effective HP planning and implementation (Jadoon et al., 2020; Kiriqi et al., 2021).
Research on environmental impacts of HP projects has occurred in an unequally distributed manner leaving African countries understudied. Truffer et al. (2003) considered impacts of water abstraction/diversion on water quality and biodiversity (fishes, macroinvertebrates, macrophytes, etc.). In Switzerland, national-level HP plant regulations require operators to reduce the negative ecological impacts of hydropeaking (Bruder et al., 2016; Tonolla et al., 2017). Hydropeaking usually entails upstream (reservoir) inundation and intensive, unnatural downstream discharges that can drastically impact the channel and sediment transport regimes over short timescales (Bruder et al., 2016). Jones (2014) describes river hydropeaking as creating two rivers, one of low flow and one of peak flow.
The concept of environmental flows (E-Flows) appeared in Europe and the United States in the mid-20th century as a response to rapid water resource infrastructure development and the impact of severe flow regulation and diversion of natural waters on biodiversity (Matthews et al., 2014; Winemiller et al., 2016). Before, little or no consideration had been given to ecosystem services or the water needs of river ecosystems. Since the 1980s, E-Flow research assessing ecological impacts of HP plants has proliferated exponentially in the scientific records (Kuriqi et al., 2021). E-Flow regime implementation has become a crucial framework for conserving fluvial ecosystems and mitigating ecological impacts of HP plants. The E-Flow for a river ecosystem includes estimates of not only the amount of water to be released downstream of diversions and abstractions but also the frequency, duration, timing, and rate of discharge changes (Matthews et al., 2014; Kuriqi et al., 2021). E-Flows must guarantee permanent connectivity of the river continuum for fish and other aquatic organisms, as well as habitat integrity (e.g., water quality, substrate, riverbank, and bed morphology) upstream and downstream of the diversions. In less well-studied river ecosystems, hydraulics-based methods using tables or other basic data to estimate E-Flows include the Tennant (1976) and Tessman (1980) methods. These specifically use percentages of the mean annual flow of the duration curve (% MAF; Tennant, 1976; Tessmann, 1980; Smakhtin et al., 2004; 2006). Other methods include hydraulic and habitat modelling, holistic methods (King and Louw, 1998; Hughes et al., 2001), and recently developed “dynamic approaches” (Kuriqi et al., 2021). Although criticized for not addressing ecological factors, hydrology-based methods require fewer resources and have provided effective results in several cases (King and Louw, 1998; Hughes 2001; Smakhtin et al., 2006; Pastor et al., 2014).
Tennant (1976) recommends a minimum instantaneous flow representing 10% of the average flow of a river necessary to sustain habitat and survival for most aquatic life on short timescales. The study also recommended a flow average of 30% to sustain the biological integrity of the river ecosystem as a whole. Tessmann (1980) described monthly time steps for determining flow thresholds and suggested that monthly mean flows (MMFs) may act as E-Flows if they equal ∼40% of the river’s mean annual flows (MAFs). According to Pastor et al. (2014), 60% of the MMF can be allocated during the low-flow season, 45% during the intermediate-flow season, and 30% during the high-flow season. Low-, intermediate-, and high-flow seasons are respectively defined as periods with MMF <40% of MAF, MMF between 40 and 80% of MAF, and MMF >80% of MAF. Smakhtin et al. (2004) used annual river discharge to define E-Flow requirements for global applications by determining pristine, good, fair, and degrading hydrological conditions for river ecosystems. This method used Q50, Q75, and Q90 percentiles to respectively designate good, moderate, and fair or degrading ecological status for the river. Q90 was then used as a base flow for the E-Flow requirements.
This study describes the Ruzizi River, which forms the border between DRC and the Republic of Rwanda to the north, and DRC and the Republic of Burundi to the south. The HP dams Ruzizi I and Ruzizi II (28 MW and 44 MW installed capacity, respectively; Table 1) have operated along the river since 1959 and 1989. Ruzizi I rests 3 km downstream of Lake Kivu outflow (Mururu site), while Ruzizi II rests 16 km downstream (Mumosho site; TRACTIONEL and RRI, 1980; Fichtner, 2008; ONEC-BAD, 2015). The Ruzizi River links Lake Kivu with Lake Tanganyika, which receives 30% of its total riverine inputs from the Ruzizi (Vandelannoote et al., 1999). No EIAs were conducted prior to the construction of either dam. According to ONEC-BAD (2015), two more dams are planned, including Ruzizi III (147 MW; Dombrowsky et al., 2014) and Ruzizi IV (also known as “Sisi V”; 287 MW). These or other forthcoming projects demonstrate the urgent need for hydrological and ecological analysis of the system (SHER and ARTELIA, 2017).
Ruzizi I and Ruzizi II have been subject to technical problems and poor management leading to dam malfunction and major flow disturbances. Most of the time, these dams operate in a way that causes habitat fragmentation for migrating fish. Field campaigns from 2015 to 2018 revealed that the dam operators were not aware of E-Flow requirements. Water levels were not maintained in fish ladders installed during dam construction (1959, 1989) for migrating fish including cyprinids.
Geochemical studies of river basins have helped constrain exogenic cycles of elements in the continent–river–lake–ocean system (Giridharan et al., 2010; Khan et al., 2022). The hydrogeochemical properties of water are important factors determining its suitability for domestic use, irrigation and industrial purposes. Interactions of water with lithologic units control water chemistry and quality (Subramani et al., 2009; Khan et al., 2022). Khan et al. (2022) reported that discharge and human activities within the basin were among the major factors responsible for temporal and spatial variation in sediment discharge of the Ramganga River (India). Several approaches have been used to assess the chemical status of water quality in rivers (Tsegaye et al., 2006; Möller et al., 2007). Ramakrishnaiah et al. (2009) evaluated the water quality of the Tumkur and Karnataka (India) and Aksu (SW Turkey) rivers using Turkish water quality indexes. Yidana and Yidana (2010) used conventional graphical methods with multivariate statistical methods and GIS to interpret controlling hydrochemical factors at different locations in a river system. These researchers also used water quality index (WQI) methods to assess the suitability of groundwater for human consumption. Kannel et al. (2007) used WQIs to evaluate spatial and seasonal changes in the water quality of the Bagmati River Basin. Debels et al. (2005) reported WQI estimates based on nine physicochemical parameters periodically measured from 18 sites to characterize spatial and temporal variability of surface water quality in the Chill’an River basin.
The present study differs from previous studies in that it uses empirical data to estimate E-Flow for power production and ecological integrity in an existing river basin. Compliance with E-Flow standards can render energy produced by Ruzizi dams safer and more sustainable. This study also analysed environmental challenges related to HP production along the Ruzizi River. The research specifically sought to 1) analyse the hydrological regime, 2) evaluate the sustainability of HP operations, 3) quantify the minimum hydrological flow required to cope simultaneously with electricity production and river ecosystem functioning, and 4) evaluate the current water quality status and sediment transport within the river.
Study Site
The Ruzizi River formed during the Quaternary period in the eastern part of the Congo basin’s Albertine Rift Region (Figure 1A). Beginning with its outflow from Lake Kivu, the Ruzizi drains a 5,800-km2 catchment (Eisenberg, 2018). The drainage density map shows five Strahler’s orders (Figure 1B; Stahler, 1952). The Upper Ruzizi (where Ruzizi I and Ruzizi II are located) follows a V-shaped valley in a mountainous and high altitude region (1,460 m a.s.l. at Bukavu–Cyangugu; Figure 1A&B). The Lower Ruzizi (below Ruzizi III) meanders across a wide floodplain before reaching Lake Tanganyika after 120 km of total river length (770 m a.s.l. at Uvira–Bujumbura; Asselberghs, 1939). Eruptions of the Virunga volcanoes reversed the drainage of Lake Kivu from the Nile into the Congo basin (Célérier, 1931). The Upper Ruzizi catchment experiences a humid climate with a bimodal annual precipitation regime in the Lake Kivu basin of ∼1,200 mm/yr and evapotranspiration of ∼1,100 mm/yr (Muvundja et al., 2014). The Lower Ruzizi catchment experiences lower precipitation (∼800–900 mm/yr, Hyghes and Hyghes, 1992) and higher temperature differences (mean monthly minima: 14.5–17°C; mean monthly maxima: 30.5–32°C) relative to the Upper Ruzizi. The rainy season spans from September to May and the dry season from June to August.
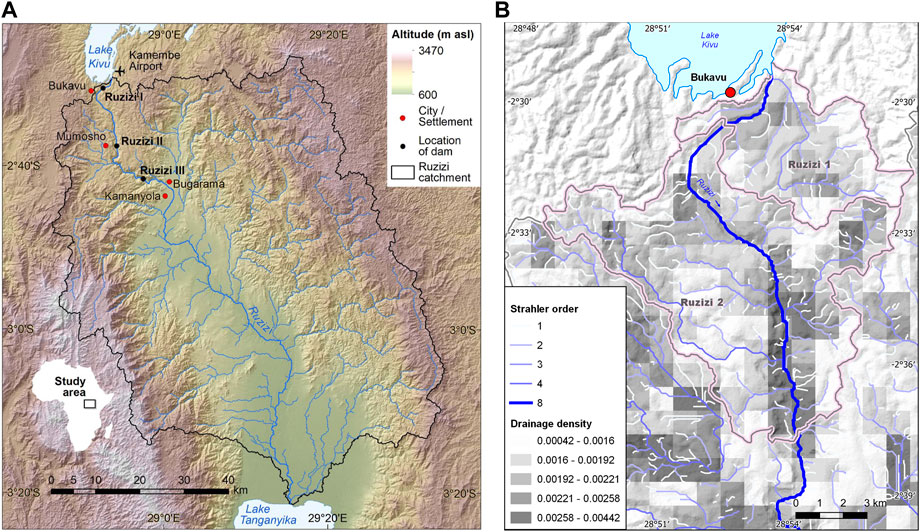
FIGURE 1. (A) Map of the Ruzizi catchment showing hydropower dam locations modified after Eisenberg (2018) (B). Drainage density and the Strahler order map for the Ruzizi River catchment according to Strahler (1952).
The Ruzizi River basin also hosts a diverse range of endangered and legally protected aquatic fauna including mammals such as hippopotami and otters, reptiles (crocodiles, snakes, and turtles), fish (lungfish, endemic cichlids, cichlids, carps, and perch), birds (both resident and migratory), amphibians, and freshwater crustaceans and other invertebrates (Hyghes and Hyghes, 1992). The wetland vegetation around the basin is dominated by Phragmites sp., Cyperus sp., Love reed (Typha sp.), and Panicum sp. (Hyghes and Hyghes, 1992). The ecological uniqueness and biodiversity found in the Ruzizi River basin arise from its relief, varied land types, and climate (Lambinon and Reekmans, 1980).
Dam construction for electricity generation began on the Ruzizi River in the mid-20th century. Table 1 lists information on existing and planned dam projects. This study addressed Ruzizi I and II dams and the downstream Ruzizi III project (Figure 1). Dams were designed assuming a theoretical usable discharge of 92–100 m3/s (TRACTIONEL and RRI, 1980). Reservoir volumes of 1.46 × 106 and 1.75 × 106 m3 (Table 1) give water residence times of ∼4.7 and ∼4.9 h, respectively. Land use in the Ruzizi I catchment consists mostly of urban activity. The Ruzizi II catchment is a mosaic of urban, peri-urban, and rural land use types. The reservoirs of both dams are polluted by urban wastewater.
The Ruzizi waters exhibit higher salinities than those of its tributaries. The salinity of the topmost Upper Ruzizi is the same as that of Lake Kivu surface waters (∼1.1 g/L or 1,200 μS/cm of electrical conductivity (EC) at 20°C; Schmid et al., 2005). The Lower Ruzizi exhibits a salinity of ∼0.5 g/L (∼650 μS/cm) at Kiliba station (Kubaburhanzi, 2015). According to Kubaburhanzi (2015), most tributaries exhibit less than 2 m3/s discharge during low water periods but become torrential during heavy rainfall with discharge up to 20 or 30 m3/s. Values of pH range between 7.5 and 8.5 in the main channel. Tributary pH ranges between 7.2 and 7.5, and EC rarely exceeds 330 μS/cm (Kubaburhanzi, 2015; Cizungu, 2016; Hange, 2016; Kikuni, 2016; Muhindo, 2016).
Materials and Methods
Hydrometeorology and Hydrologic Data Analysis
Monthly rainfall data obtained for a 2003–2010 study period from a local meteorological station located at Kamembe Airport (Rwanda, Figure 1) were compared with data covering the same period from three grid cells of the global satellite precipitation data (rainfall data by the U.S. National Oceanic and Atmospheric Administration). These span most of the Upper Ruzizi River catchment. The two data sets showed a strong linear correlation (
Minimum Flow Analysis
This study used time series data from 1941 to 2015 and hydrological methods (look-up tables) to evaluate E-Flows at Ruzizi I (almost identical at Ruzizi II). Several hydrological indexes were calculated to evaluate minimum flow requirements. In addition to MAF, the indexes termed Q25, Q50, Q75, and Q90 follow a format, where Qi represents flows equal or exceeding the ith percentile of the recording period (Tennant 1976; Tessmann 1980; Pastor et al., 2014). Given its adequate performance for large rivers, this research used the Tessmann method to estimate minimum flow requirements (Karakoyun et al., 2018). The mean minimum flow was calculated as the difference between the long-term average river discharge and the long-term usable turbine discharge. The latter was derived from a regression model relating generated electric power to the turbine discharges for the period between 1989 and 2003 (Figure 2). The lack of ecological data precluded testing of other E-Flow methods.
Turbidity and Total Suspended Solids
Daily water turbidity measurements were performed upstream and downstream of the Ruzizi II dam from 10 September 2016 to 30 October 2017 using an HACH 2100Q turbidimeter. Several measurements were also made at Ruzizi I. Standard chemical solutions made for stabilized formazine turbidity values of 20, 100, and 800 NTU were used to calibrate the turbidimeter prior to field measurements (HACH 2009). Samples registering a turbidity value of more than 800 NTU were diluted with distilled water. Turbidity measurements are reported as mean values of triplicate analyses (coefficient of variation <6%). A calibration curve between turbidity of the standard solutions and total suspended solids concentration (TSS) was developed by preparing liquid samples from red clay Ferralsols (Muvundja et al., 2009), which typically occur in the catchment. In this procedure, soil samples were collected from the upper 10 cm of soil horizons and dried overnight in an oven at 110°C. Samples were thereafter stored in a desiccator until grinding and sieving at 1-µm mesh. Powdered samples were weighed and suspended (Minella et al., 2008; Perkins et al., 2017) in rainwater for calibration measurements.
Water Quality
Physicochemical parameters such as pH, dissolved oxygen (DO), water temperature, and EC were measured in situ at around 08:00 h on a monthly basis over the entire sampling period. In situ pH, water temperature, DO, and EC were measured using a Hydrolab probe (Data sonde ®4a USA). Water transparency (m) was measured with a 20-cm-diameter Secchi disk, with quadrants painted in black and white. Secchi depth was calculated as the average depth of disappearance and reappearance of the disk in water. Water samples were analysed for ammonium, soluble reactive phosphorus (SRP), nitrites, and silica. Biological oxygen demand (BOD) and chemical oxygen demand (COD) samples were collected using 4-L bottles downstream of Ruzizi I (twice per month) and Ruzizi II (once per month) over a 12-month period from January 2016 to February 2017. At each same location, equal sample volumes were collected from surface and middle depths before being mixed into a composite sample. Samples were preserved on ice during transport from the field to the laboratory and kept frozen until analysis. Standard methods were used for nutrient analysis. These included ammonia (NH4+) by the dichloroisocyanurate–salicylate method, SRP by the ascorbic acid method, nitrite (NO2−) by the colorimetric method, and soluble reactive silica by the molybdate complex method (APHA, 2005). Concentrations of these nutrients were estimated from UV/Vis spectrophotometry (Spectronic ®20 Genesys, USA). Total alkalinity concentration was estimated by titrimetric methods. Chloride concentrations in water samples were analysed based on titration with silver nitrate (Bartram and Ballance 1996; APHA, 2005; Rodier, 2009). BOD was determined using Oxitop bottles, while the analysis of COD was performed on K2Cr2O7 oxidation-treated samples using spectrophotometric methods (Barttram and Ballance, 1996; Rodier, 2009; Lina, 2016). All analyses were performed at the Unité d’Enseignement et de Recherche en Hydrobiologie Appliquée (UERHA), Institut Supérieur Pédagogique de Bukavu. The electric charge-balance error of the water samples fell within <5% of measured values. The data were analysed with the help of the Statgraphics software. The water quality of the samples was assessed by calculating WQI values based on two WHO (2008) and TSI-266 (2005) standards.
Calculation of Water Quality Indexes
The Water Quality Index (WQI) is defined as a rating reflecting the composite influence of different water parameters (Sahu and Sikdar, 2008). In this study, the water quality of the Ruzizi River was evaluated for drinking and other purposes using the WQI method based on pH, HCO3−, Cl−, COD, BOD, organic matter, NH4+, NO2−, PO43-, SiO2, turbidity, and total alkalinity. Interpretations used World Health Organization (WHO, 2008) limits for reference. To calculate WQI values at each sampling station, weightings (wi) were assigned to each parameter according to their relative importance in the overall water quality expression for drinking purposes. The highest weight of five was assigned to nitrate (NO3−) and nitrite (NO2−) parameters due to their strong effects on drinking water (Şener et al., 2017). Consumption of water with high nitrate or nitrite concentrations can cause methemoglobinaemia disease (blue baby syndrome) in infants, gastric carcinomas, abnormal pain, central nervous system birth defects, and diabetes (Vasanthavigar et al., 2010; Varol and Davraz, 2015). The parameters pH, COD, BOD, SiO2, and turbidity were assigned a weight of 4, while HCO3− and Cl− received a value of 3. The minimum weight of 1 was assigned to total alkalinity. Relative weights (Wi) were then calculated for each parameter using Eq. 1. The WQI and overall WQI values for all samples were calculated using Eqs 2–6 to establish water quality designations for each sampling point.
The relative weight (Wi) is calculated as follows:
where wi is the weight of each parameter i (1 to n). A quality rating (qi) for each parameter was calculated by dividing the concentration
To calculate WQI, the
Calculated WQI values were then parsed into five categories (Sahu and Sikdar, 2008; Yidana and Yidana, 2010; Şener et al., 2017) according to the WQI intervals:
WQI <50: excellent water quality.
50 < WQI <100: good water quality.
100 < WQI <200: poor water quality.
200 < WQI <300: very poor water quality.
WQI >300: unsuitable for drinking water.
In addition, the effective weight (EWi) for each water quality parameter i was defined by dividing its subindex parameter value SIi by the overall WQI value (Eq. 4) and multiplied by 100:
The relative weights (Wi) reflect the significance of each parameter in relation to the other parameters used in WQI calculations. WQI is usually calculated in terms of a specific and intended use of water. In this study, the WQI was calculated relative to drinking water standards set as:
Results
Flood Frequency Analysis of Flow at Ruzizi I
Discharge records (1941–2015) suggest a long-term mean flow of 112 m3/s for the Upper Ruzizi dam (Figure 2). Results of the flood magnitude–frequency analysis following Gumbel (1941) and Dalrymple (1960) indicate an exponential relationship between the 75 years of discharge data (1941–2012) and the recurrence time interval (Figure 3A; R2 = 0.98). The high coefficient of determination indicates the suitability of Gumbel’s distribution (also called extreme value distribution) in predicting river flow. The curve exhibits linear behaviour for smaller recurrence interval values. Discharges between 46 and 120 m3/s can occur at any time within a 3-year time interval (Figure 3A). Longer recurrence interval values (t > 12.6 years) associate with discharge values of ∼130 m3/s (Figure 3A). The maximum flood event recorded occurred in 1998 with a peak flow of 143 m3/s and recurs over a 76-year interval according to the flood frequency curve (Supplementary Table S1). Excessive flows like these likely occur or may be exceeded in a given year with a probability of ∼1.3% (Supplementary Table S1 and Figure 3B). The lowest flow of 46 m3/s occurred in 1944 and represents a minimum recurrence value that will be exceeded with a probability of 100% (Supplementary material, Table 1 and Figure 3B). The discharge–exceedance probability curve for natural Ruzizi flows gives values of 126, 96, 79, and 62 m3/s for Q25, Q50, Q75, and Q90, respectively (Figure 4).
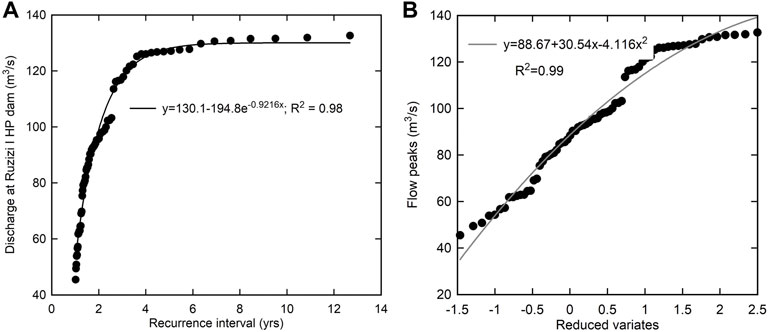
FIGURE 3. (A) Magnitude–frequency curve for flows (Gumbel’s curve) at the Ruzizi I HP dam (data from SNEL, 1941–2015). (B) Relationship between flood flows and reduced variates (same data set as in A).
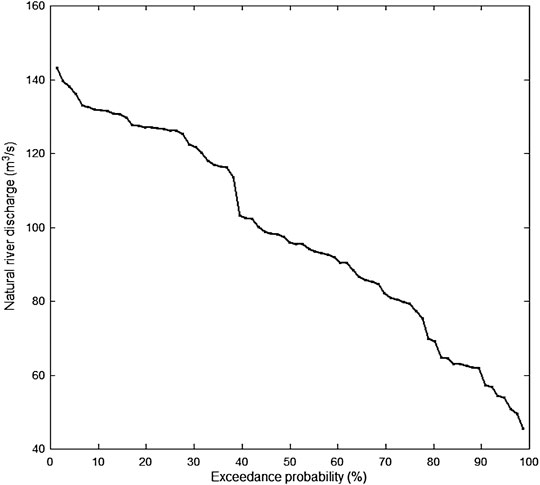
FIGURE 4. River flows at Lake Kivu outflow vs. exceedance probability (same data set as in Figure 3A).
Minimum Hydrological Flow
The relationship between generated electric power and turbine discharges over a period of 14 years (1989–2003; Figure 5) yields 15.7 MW for a river discharge of 83.7 m3/s. This is the long-term average power production at the corresponding long-term average flow of 112 m3/s. The difference is 28 m3/s (= 112−84), a value that should be implemented as the volume of permanently and freely flowing water in the bypass and fish ladders.
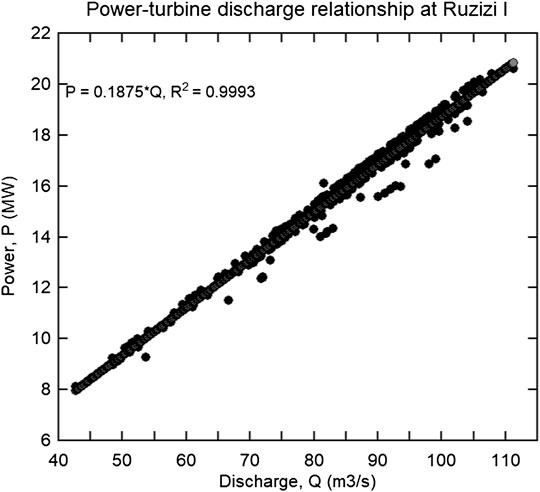
FIGURE 5. Power–turbine discharge relationship at the Ruzizi I HP dam (SNEL, 1989–2009). Data sets represent monthly power production and monthly discharge averages of the turbines.
Surface Sediment Transport and Water Quality
We monitored turbidity and total suspended solids both upstream and downstream of Ruzizi II (Figure 1) over an annual cycle. Few data were available for Ruzizi I during the rainy season. The comparison between mean monthly turbidity at Ruzizi II and mean monthly rainfall (Figure 6) showed that turbidity and precipitation followed the same seasonal trend. The two parameters show moderate correlation (R2 = 0.62 for upstream and 0.58 for downstream). The highest peaks of turbidity appeared during rainy seasons (September to June) with mean values exceeding 20 NTU. Maximum values were recorded in October (∼30 NTU) and February (∼36 NTU). The river gave lower turbidity values during the dry season. Values ranging from ∼9 to ∼13 NTU appeared from April to August with the lowest values in August (Figure 5). During the rainy season, the mean annual turbidity at the surface was 6.5 (upstream) and 5.1 NTU (downstream) of Ruzizi I (Supplementary material, Table 2). The annual mean was 24.5 upstream and 22.9 NTU downstream of Ruzizi II during rainy seasons, whereas dry season turbidity fell to 10.8 and 9.4 NTU upstream and downstream, respectively. In the Ruzizi I reservoir, turbidity measurements indicated 8.5, 7.6, 7.7, 8.3, 8.1, 5.8, and 6.9 NTU for 1, 2, 3, 4, 5, 6, and 7 m depth, respectively. For the Ruzizi II reservoir, the measurements yielded 11.3 NTU at 1 m, 13.1 NTU at 2 m, and 22.0 NTU at 3 m depths.
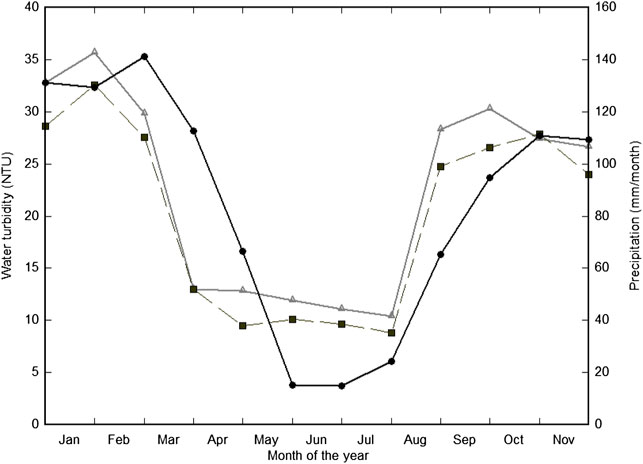
FIGURE 6. Seasonal variation of precipitation and water turbidity upstream and downstream of the Ruzizi II dam. Data sets represent monthly averages of turbidity and catchment rainfall from September 2016 to October 2017.
Waters generally appeared more turbid upstream than downstream of the dams. The difference was not significant for Ruzizi I (p-value = 0.6818 at p < 0.05, N = 22, two-tailed Mann–Whitney U test). However, the Ruzizi II site gave higher turbidity values by a factor of two than the Ruzizi I site. Upstream and downstream values showed statistically significant differences (p-value = 0.008 at p < 0.01, N = 308, two-tailed Mann–Whitney U test). The Ruzizi II reservoir exhibited higher turbidity (by ∼2x) than the Ruzizi I reservoir. Daily turbidity data indicated differences of about 10% between the reservoirs, 10% above and below the Ruzizi II dam, and 22% above and below the Ruzizi I dam. This indicates a higher sedimentation rate at Ruzizi I relative to Ruzizi II.
Figure 7 shows a model used to convert turbidity measurements to total suspended solids (TSS). The TSS concentrations at Ruzizi I averaged 10 mg/L (both at upstream and at downstream; applicable to the rainy season only). For Ruzizi II, the average turbidity values found during the rainy season reached 60 and 50 mg/L upstream and downstream, respectively, compared to 20 mg/L upstream and downstream during the dry season. Annual mean TSS at Ruzizi II was 50 mg/L. A mean discharge of 98 m3/s corresponds to an annual particle transport of 155 kt/yr and 1 kt/km2/yr over the entire Ruzizi II subcatchment.
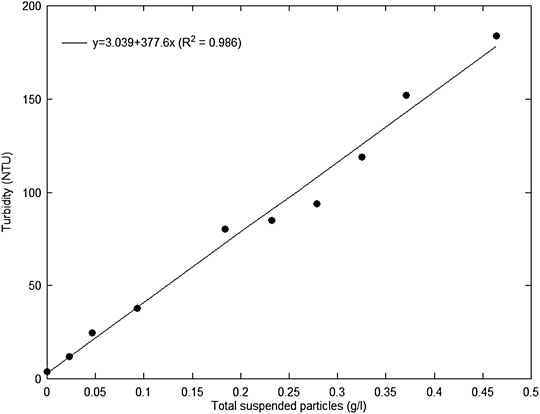
FIGURE 7. Calibration curve between turbidity and catchment soil particle concentrations (No. of samples = 12). Soil samples (Ferralsols) were collected at ISP Bukavu in the town of Bukavu (Figure 1A).
During the sampling period, the pH values varied from 6.1 to 10.0 with a mean value of ∼9. Water temperature ranged from 23 to 26°C, and EC varied over 1,021 to 1,225 μS/cm (Supplementary Table S3). In situ measured DO values of water samples ranged from ∼0 to 9 mg/L, with an average value of ∼5–6 mg/L depending on the site (Supplementary Table S3). Low DO values were measured in all reservoirs but not consistently. The DO saturation varied between 3 and 122% with averages between 60 and 86% depending on the site (Supplementary Table S3).
The mean COD ranged from 527 to 783 mg/L, whereas BOD varied from 8 to 26 mg/L, with an average of 19 mg/L (Supplementary Table S3). The concentration of total alkalinity ranged from ∼0 to 2 mg/L (Supplementary Table S4). Both minimum and maximum values were measured upstream and downstream of Ruzizi I, respectively.
Ammonium (NH4+) contents of the Ruzizi River ranged from 0.01 to 1.60 mg NH4+/L. The higher value was measured upstream of Ruzizi I. Nitrite (NO2−) contents ranged from ∼0 to 9.80 mgNO2-/L during the sampling period. Elevated values may reflect leaching of waste disposal, sanitary landfills, overdosage of inorganic nitrate fertilizer, or improper manure practices (Chapman, 1996). Phosphate concentrations ranged from 0.01 to 0.73 mgPO43-/L. The highest phosphate values occurred upstream of the dam. The silica contents of the water samples ranged from ∼0 to 31 mgSiO2/L.
The WQI-relevant parameters included COD (with mean effective weights between 55 and 73% depending on the sites), BOD (3–11% mean effective weight), and finally nutrients and pH (Supplementary Table S4). The results obtained from this study (Table 2) gave WQI values for the Ruzizi River ranging from 605 to 792 over all sampling stations. These significantly exceeded drinking water limits (WQI <100).
Discussion
Minimum Hydrological Flow Under Current Ruzizi HP Dam Operations
The Ruzizi I and II HP plants never reach their installed capacity (28 and 44 MW, respectively; SHER and ARTELIA, 2017) due to recurrent technical problems caused by ageing or outdated technology, and poor management and maintenance. Ruzizi I operates at an average power of ∼16 MW (Figure 5), whereas Ruzizi II averages at 25 MW (EGL 2015). This means that if efficiently managed, they would require only ∼85 m3/s of the long-term discharge of ∼112 m3/s.
The recorded base flow is ∼65 m3/s, while the maximum flow is ∼140 m3/s (Figure 2). The base flow is almost the same as the Q90 value (62 m3/s; Figure 4) according to the percentile of discharge modelling by Smakhtin et al. (2004). This E-Flow requirement represents fair hydrological conditions below which river ecological integrity will start to decline (Smakhtin et al., 2004). Excess flow beyond the optimal power generation discharge (85 m3/s) is 28 m3/s, which should flow freely and permanently outside the turbines. This means that turbines can operate efficiently on average without closing the bypasses and fish ladders. This water amount can likely sustain short-term aquatic life when hydropeaking is required for dam operations and turbine maintenance. This water flow is equivalent to ∼25% of the average river discharge and can be considered as an ideal potential E-Flow. Minimum flow represents more than the 10% required to sustain short-term preservation of habitat for most aquatic life forms, and approaches the 30% flow value recommended for sustaining the biological integrity of riverine ecosystems (Tenant, 1976). The ∼25% value also approaches the 30% of MAF E-Flow suggested by Pastor et al. (2014) for high-flow seasons. The calculated minimum hydrological flow represents 61% of the lowest natural flow (i.e., 46 m3/s) ever observed from the Ruzizi River (Supplementary Table S1; Supplementary Table S4). This minimum flow fits with the 60% MMF value recommended for E-Flow during low-flow seasons (Pastor et al., 2014). For Ruzizi dams, respective Q50, Q75, and Q90 that equal 96, 79, and 62 m3/s represent good, moderate, and fair ecological conditions (Figure 4).
Environmental Impacts
According to Lidec and Quintero (2003), large dams can vary considerably in terms of their adverse environmental impacts and can thus be classified as “good” or “bad” dams. Given their site selection features, Ruzizi dams were categorized as “good.” These features include the following: 1) minor evaporation loss from a small reservoir surface area, 2) minor losses of natural habitat and wildlife, 3) a relatively small river with limited aquatic biodiversity at risk, 4) a deep reservoir with low sedimentation rate, 5) many downstream tributaries, 6) little or no flooding of forests, 7) no tropical diseases, and 8) no floating weed problems. The typical “bad” dam features around Ruzizi dams include extensive soil erosion (Eisenberg, 2018; Eisenberg and Muvundja, 2020) and solid waste pollution from urbanized areas of the catchment. The solid waste in urban and peri-urban areas accumulates in the reservoirs, particularly from Bukavu, and disturbs electricity production. This waste consists primarily of plastics and plant debris. Soil erosion of the surrounding hillsides and deeply incised tributaries, where poor soil protection prevails, also contributes sediment to the reservoirs (Eisenberg and Muvundja, 2020). Water run-off from impermeable urban surface triggers significant landslides and gullies, which contribute to soil erosion and reservoir siltation.
Hydropeaking Effects
Despite environmental obligations of the dam operating companies, the fish ladders operate only occasionally. When facing technical problems, such as solid waste blockage, typical management practices consist of changing river flows. According to the HP management at Ruzizi II, downstream flow is emptied every Wednesday and Sunday for almost two hours for purposes of turbine maintenance and solid waste evacuation leading to hydropeaking in the riverine ecosystem (Liévin Cizungu, Pers. Comm.). Figure 6 shows operating discharges at Ruzizi I ranging between 60 and 100 m3/s for ∼86% of the time (Figure 6). Extreme values occur only ∼14% of the time. From 1989 to 2003, Ruzizi I operated within its expected range of electricity production (Figure 5) with enough water in the bypass (35 m3/s, i.e., ∼30% of the average river flow; Figure 6). According to Figures 5, 6, all incoming water was used from 2004 to 2009 without any perceptible increase in power production (Figures 5, 6). This reveals that technical problems including maintenance and ageing equipment have increased the environmental impact of these dams.
Flood frequency analysis of the Lake Kivu outflow shows that the natural Ruzizi discharge remained stable and did not experience any important interannual or seasonal perturbations. The highest flood flow over the last 5 decades occurred in 1998 at a peak of 143 m3/s with a recurrence interval of 76 years (Supplementary Table S1). A similar peak flow (140 m3/s) occurred once in 1963 simultaneous with the so-called “Centennial” rising of the Rivers Congo and Nile (Bergonzini et al., 2002; Muvundja et al., 2014) but associated with a recurrence interval of 38 years (Supplementary Table S1). In terms of power production, neither Ruzizi I (Figure 5) nor Ruzizi II ever reached their installed capacity (SHER and ARTELIA, 2017). At Ruzizi I, turbine discharges ranged between 43 and 112 m3/s from 1989 to 2009 (Figure 5), a period during which lake water levels allowed full utilization capacity, except during the 2004-to-2006 dry spell (Muvundja et al., 2014). By contrast, electricity production trends during this period (Figure 5) indicate that power production fluctuations depend on lake outflow and dam operation. HP plants work only at ∼60% of their capacity regardless of available flow. The 16 and 25 MW (Table 1) production thresholds for Ruzizi I and Ruzizi II can each be reached without emptying the bypasses and/or closing the fish ladders. This allows reserving enough E-Flow to bypass the dams. Our calculations yielded a flow of 42 m³/s as representing 40% of MAF, a value widely accepted by E-Flow experts (Tennant, 1976; Acreman and Dunbar, 2004; Linnansaari et al., 2013; Pastor et al., 2014). This volume of water supports ecosystems by maintaining aquatic habitats for native biota (plankton, macroinvertebrates, macrophytes, fish, and mammals; Annear et al., 2004).
The ecosystem disturbance caused by dams depends on both the quantity of water and its flushing rates. Hydropeaking floods and scours the downstream riverbed for up to two hours twice per week, flushing out plankton, macroinvertebrates, fish, food sources, eggs, and larvae (Bruder et al., 2016; Tonolla et al., 2017). During these events, mobile or nektonic organisms can take refuge in isolated waters. At these extreme flow rates, fragile organisms washed downstream suffer low survival rates. Even accidental drowning events (especially affecting children) have occurred several times downstream of Ruzizi II. Hydropeaking often results in poor macroinvertebrate diversity in dammed rivers (Bruder et al., 2016). Habitat changes caused by upstream floods resulted in declines for 1) fish species in the Ruzizi River (e.g., haplochromines and cyprinids; Munini et al., 2011), 2) zooplankton such as Alona sp. and Ascormopha ecaudis (Kisekelwa and Isumbisho, 2009), and 3) macroinvertebrate species (Hyangya et al., 2011).
Management practices impact the distribution of biota downstream of dams (Mantel et al., 2010). Declines in lake fish stocks reported by fishermen result from disruption due to hydropeaking during critical developmental stages. Overfishing may have also exacerbated the situation. Fishing communities argue that some species have become extinct and no longer appear either upstream or downstream (Bahimba Janda Morgan, Mumosho, pers. comm.). Despite their adaption to unstable hydrology, even cyprinid fish (Barbus spp.) appear impacted. These fish can swim through rapids and temporarily migrate into small tributaries for spawning and refugia (Masilya et al., 2020). Following E-flow protocols and other practices can mitigate these impacts (Tennant, 1976; Pastor et al., 2014). Specifically, Leclerc and Quintero (2003) suggest the following for hydroelectric projects:
(1) Always maintain between 28 m3/s (40% MAF) and 62 m3/s (Q90) of water flow in the bypasses and fish ladders to sustain permanent outlets and allow upward and downward fish migration at any time. Dam operators should always keep fish ladders operational to help migratory fishes move up and down the river bypass. The E-Flow will also help rehabilitate the cultural and religious site of Butagarura Falls near the Ruzizi II dam.
(2) Improve the management of water releases to reduce the impacts of hydropeaking on biota. Water can be released incrementally allowing motile organisms in and below the reservoir to survive such events (Ledec and Quintero, 2003).
(3) Fish hatcheries should be implemented to support populations of native fish species such as tilapia and African catfish in the reservoirs. Fishing regulation should be enforced to maintain viable populations of commercial fish species.
(4) The area needs a long-term and integrated waste management plan for towns and facilities within the catchment and adjacent to the reservoir.
Forthcoming dam construction projects should install adequate reservoir volumes for flow requirements. Project planning should specifically include a minimum flow of ∼28 m3/s (outside the turbines) to avoid unrealistic estimates of power production. A 10% of MAF E-Flow estimate suggested for Ruzizi III (ONEC-BAD 2015) will not suffice and should be revised prior to project implementation.
Surface Sediment Transport
Sedimentation reduces live storage and power generation over time and thus compromises the HP and environmental services provided by the dam (Ledec and Quintero, 2003). Greater turbidity and longer stagnation in the reservoir lead to higher sedimentation rates.
Ruzizi River turbidity varies from levels appropriate for natural waters (e.g., T < 25 NTU; domestic use, fisheries, and recreation) to high suspended particle concentrations of T > 25 NTU (MPCA, 2008). According to MPCA (2008), turbidity levels of 25 NTU in rivers and streams equal a suspended particle concentration of ∼58 mg/L. This value can vary significantly for individual streams and rivers even within the same large-scale catchment (MPCA, 2008). For the Ruzizi River basin, 25 NTU was surprisingly consistent with the empirical value of 58 mg/L.
Population density (>400 inhabitants/km2), topographic relief in the Kivu region, intense pluviosity, and soil degradation cause considerable erosion and frequent landslides in the Ruzizi catchment (Muvundja et al., 2009; Eisenberg and Muvundja, 2020). This contributes to high levels of turbidity in the Ruzizi River. A high correlation (r = 0.79; N = 12) between precipitation and turbidity (Figure 6) confirms this interpretation. Turbidity recorded during the dry season (∼10 NTU) represents a baseline more or less corresponding to Lake Kivu seston, which matches surface water turbidity (10–20 NTU; Pasche et al., 2013).
Soil erosion represents a major environmental issue in this region given the impacts of reservoir siltation. A related study reported an erosion rate of ∼577 kt/yr for the entire catchment (Eisenberg, 2018). A corresponding area-normalized value of ∼5 kt/km2/yr falls within the range of an 8.4 kt/km2/yr estimate reported in Nambajimana et al. (2020) for soil losses in the same river catchment of the Rwandan District of Rusizi. The present study estimated surface sediment transport of ∼1 kt/km2/yr (see above). This represents only a quarter of the loss estimated by Eisenberg (2018). The discrepancy may reflect the large quantity of soil particles deposited as sediment within the catchment along tributaries and within the reservoirs. Comparing the Ruzizi catchment with neighbouring subcatchment of Lake Kivu having similar soil types, land use, and geological and climatic conditions can help constrain the understanding of soil loss risk as it applies to the Ruzizi catchment. The area-specific TSS load transported by the Upper Ruzizi reaches values three to four times higher than those reported for Kawa, Mugaba, and Murhundu Rivers and 14 times higher than those reported for the whole Lake Kivu catchment average (Muvundja et al., 2009).
Reservoir siltation implies nutrient removal from waters downstream of dams. Data given in Muvundja et al. (2009) exhibited a high correlation (
The Ruzizi II reservoir suffers from greater soil erosion, siltation, and flooding. The bottom waters of the reservoir were 62% more turbid than surface waters. This indicates that incoming particulate material and nutrients predominantly sink to bottom water areas but remain susceptible to transport. Based on their work on Lake Brienz (Switzerland), Finger et al. (2006) reported that HP dams drastically diminish particle fluxes and filter solid particulates according to size and density. Coarser particles settle in upstream areas of the reservoir, while fine-grained particles remain suspended and pass downstream. These researchers found that only ∼3% of the fine-grained materials were deposited as sediment. We therefore assert that the suggested minimum flow would help maintain sediment transport downstream of dams even though some siltation would still occur.
Measures to mitigate reservoir sedimentation require effective catchment management. Management can include the implementation of conservation practices on agricultural land such as terracing and planning of road construction, mining projects, and other land use changes using sustainable best practices (Ledec and Quintero, 2003). A protected area established in the Ngomo mountainous region, which abuts the Ruzizi IV (Sisi V; Figure 1), would reduce sediment flows into reservoirs of planned future dams (SHER and ARTELIA, 2017).
Water Quality
Low DO concentrations likely arise from waste discharges along the course of the river, which stimulate increased microbial oxidation of organic matter (Patnaik, 2005). While the average measured DO falls within ‘good’ water quality standards (Riziki, 2016), water samples gave a mean COD of 623 mg/L (Supplementary Table S4). Values indicate voluminous quantities of oxidizable organic and inorganic pollutants (Otukune and Biukwu, 2005). The total alkalinity contents of water samples fell within TSI-266 (2005) and WHO (2008) limits.
Nutrients, such as bioavailable forms of phosphorus and nitrogen (ammonia, nitrate, and nitrite), can strongly impact lake water quality (Şener et al., 2013) due to their role in eutrophication (Soulsby et al., 2001). Orthophosphates can rapidly be absorbed by plants and generally exert a greater influence on eutrophication than nitrogen (Sharpley et al., 2001). The pH- and temperature-dependent, un-ionised forms of ammonia, however, pose high toxicity risk to fish even at low concentrations (Debels et al., 2005). Most of NH4+ concentration measurements fell within acceptable standard limits (0.5 mg/L, WHO, 2008; Rodier, 2009). The NO2 contents of water samples collected upstream of Ruzizi I exceeded permissible TSI-266 (2005) and WHO (2008) limits. Most sample sites yielded phosphate values higher than eutrophication-limiting values (0.1 mg PO43-/L; Bartram and Balance, 1996; Rodier, 2009; Şener et al., 2017). All silica values fell within permissible WHO (2008) limits. The highest silica concentration value occurred upstream of Ruzizi I likely reflecting the Lake Kivu origin of Ruzizi waters.
Calculated WQI values ranged between 600 and 800 (Table 2). The computed WQI average for all monitored stations was 271. This value exceeds WHO standard values (Table 2) and falls within the category of poor quality for drinking water (Sahu and Sikdar, 2008; Yidana and Yidana, 2010). The high WQI likely arises from municipal waste and organic loads generated by agricultural activity. While solid waste was not investigated by this study, solid waste accumulation is a significant problem. The Ruzizi I and II reservoirs respectively receive 1,200 and 1700 m3/yr consisting of 60% domestic organic waste and 40% industrial solid waste (EGL, 2015). This material limits power generation at the two dams (SNEL and SINELAC, Pers. Comm.).
Sustainable Management of Ruzizi and Other African HP Dams
Sustainable management of HP resources requires a minimum flow to maintain (as best as possible) the “natural river course.” This limits sediment accumulation in reservoirs. Local government and dam managers need to implement agricultural practices that reduce land degradation and soil/nutrient loss. This will improve the river water quality and expand the ecosystem services provided by both the land and rivers. Soil conservation and habitat expansion can support riparian communities. Dam maintenance requiring flow cessation (repairs and cleaning) should use incremental changes in flow to avoid scouring of the channel or catastrophic downstream transport of aquatic organisms (juveniles and even adult fishes, macroinvertebrates, and zooplankton). According to Welcome et al. (2006), poor flow control can devastate species that produce semi-pelagic eggs. Consistent, natural flows disperse eggs and larvae to new habitats representing appropriate juvenile nursery grounds on floodplains or in backwaters.
The plastics and other solid pollutants in Lake Kivu and the Ruzizi River require a comprehensive and integrated waste management plan. Such an initiative could also support Lake Tanganyika, a body fed by the Ruzizi River and a hot spot of freshwater biodiversity. Dam management could fund waste management by allocating resources equivalent to what they lose in electricity production caused by waste evacuation towards recycling or waste capture/reduction programmes. Current initiatives of converting plastic waste to cobblestones and building blocks offer examples of creative and economically lucrative waste diversion. The river and reservoir projects face the dual problem of poverty and human encroachment along the riparian corridor and reservoir areas. Initiatives wherein HP companies work with local communities to reduce poverty, conduct restoration and protect buffer zones between the river and human settlements or cultivated land along the HP dam cascade could reduce reservoir siltation and pollution. Specialized agricultural methods such as terracing, afforestation, and pasture improvement for steeply sloping areas can reduce landslides, gully formation, and erosion. Each of these compromises the environmental integrity of tributaries and the Ruzizi dam operations. Finally, government agencies should enforce EIA policies and regulations regarding forthcoming HP dams.
Data Availability Statement
The original contributions presented in the study are included in the article/Supplementary Material; further inquiries can be directed to the corresponding author.
Author Contributions
FM, AK, CA, JE, and AW actively contributed to the research elaboration and methodological approach design. Except AK, CA, and AW, all other authors contributed to fieldwork and laboratory work. All authors substantially contributed to analysis and interpretation, which led to consensus and agreement on publication.
Funding
This study was funded by the Volkswagen Foundation under the initiative “Knowledge for Tomorrow—Cooperative Research Projects in Sub-Saharan Africa” (Grant No. 89358).
Conflict of Interest
The authors declare that the research was conducted in the absence of any commercial or financial relationships that could be construed as a potential conflict of interest.
Publisher’s Note
All claims expressed in this article are solely those of the authors and do not necessarily represent those of their affiliated organizations, or those of the publisher, the editors, and the reviewers. Any product that may be evaluated in this article, or claim that may be made by its manufacturer, is not guaranteed or endorsed by the publisher.
Acknowledgments
We would like to express our gratitude to Mirimba Pierrot (Mumosho) for turbidity data collection. We thank our laboratory members Hyangya Beni and Mazambi Jacques (both in Bukavu) for their assistance in the field. We also thank the Société Nationale d’Electricité (SNEL, Bukavu) and Société Internationale d’Electricité des Pays des Grands Lacs (SINELAC, Bukavu-Mumosho) for access to hydrological data from the Ruzizi I and II HP dams and their collaboration during the field campaigns.
Supplementary Material
The Supplementary Material for this article can be found online at: https://www.frontiersin.org/articles/10.3389/fenvs.2022.892591/full#supplementary-material
Abbreviations
APHA, American Public Health Association; BOD, biological oxygen demand [mg/L]; COD, chemical oxygen demand [mg/L]; DO, dissolved oxygen [mg/L]; DRC, Democratic Republic of Congo; EC, electrical conductivity of water [μS/cm]; E-Flow, environmental flow [m3/s]; EGL, Energie des Grands Lacs; EWi, effective weight of ith species; HP, hydropower; IHA, International Hydropower Association; MAF, monthly annual flow [m3/s]; MMF, monthly mean flow [m3/s]; MPCA, Minnesota Pollution Control Agency; NTU, nephelometric turbidity unit; ONEC-BAD, Energy, Environment and Climate Change Department, African Development Bank; Q25, Q50, Q75, and Q90, percentile exceedances of flow duration curve [m3/s]; RRI, Rhein Ruhr International; SINELAC, Société Internationale d’Electricité des Pays des Grands Lacs; SNEL, Société Nationale d’Electricité; SRP, soluble reactive phosphorus [mg/L]; TSI-266, Turkish Standards Institution; TSS, total suspended solids [mg/L]; VMF, variable monthly flow [mg/L]; WCD, World Commission on Dams; WHO, World Health Organization; and WQI, water quality index.
References
Acreman, M. C., and Dunbar, M. J. (2004). Defining Environmental River Flow Requirements - a Review. Hydrol. Earth Syst. Sci. 8 (5), 861–876. doi:10.5194/hess-8-861-2004
Annear, T., Chisholm, I., Beecher, H., Locke, A., Aarrestad, P., Coomer, C., et al. (2004). Instream Flows for Riverine Resource Stewardship. revised edition. Cheyenne, WY: Instream flow council, 268.
Ansar, A., Flyvbjerg, B., Budzier, A., and Lunn, D. (2014). Should We Build More Large Dams? the Actual Costs of Hydropower Megaproject Development. Energy Policy 69, 43–56. doi:10.1016/j.enpol.2013.10.069
APHA (American Public Health Association) (2005). in Standard Methods for the Examination of Water and Waste Water. Editors A. Eaton21st edition (Centennial edition) (Washington DC, USA: APHA, AWWA and WEF).
Asselberghs, E. (1939). Notice explicative de la carte géologique de la région du Kivu au 1/500000. Mémoire: Planche V.
Bartram, J., and Ballance, R. (1996). Water Quality Monitoring - A Practical Guide to the Design and Implementation of Freshwater Quality Studies and Monitoring Programmes. Geneva, Switzerland: CRC Press.
Bergonzini, L., Richard, Y., and Camberlin, P. (2002). Variation interannuelle du bilan hydrique du lac Tanganyika (1932-1995): changement dans la relation précipitation-excédent lacustre/Interannual variation of the water budget of Lake Tanganyika (1932-1995): changes in the precipitation-lake water excess relationship. Hydrological Sci. J. 47 (5), 781–796. doi:10.1080/02626660209492980
Bruder, A., Tonolla, D., Schweizer, S. P., Vollenweider, S., Langhans, S. D., and Wüest, A. (2016). A Conceptual Framework for Hydropeaking Mitigation. Sci. Total Environ. 568, 1204–1212. doi:10.1016/j.scitotenv.2016.05.032
Célérier, J. (1931). Le Kivu et le fossé des Grands Lacs Africains. Ann. Géogr. Trans. 40225, 331–333.
Cizungu, N. (2016). Hydrologie et qualité Physico-Chimique des eaux de la rivière Nshengezimu à Ibambiro/Nyangezi. Mémoire de Licence, inédit, Département de Chimie-physique, ISP Bukavu. Bukavu, DRC.
Dalrymple, T. (1960). Flood-frequency Analyses. Manual of Hydrology. Washington, DC: Flood Flow-Techniques. Part 3, Available at: https://doi.org/Geological Survey Water-supply paper 1543-A.
Debels, P., Figueroa, R., Urrutia, R., Barra, R., and Niell, X. (2005). Evaluation of Water Quality in the Chillán River (Central Chile) Using Physicochemical Parameters and a Modified Water Quality Index. Environ. Monit. Assess. 110, 301–322. doi:10.1007/s10661-005-8064-1
Dombrowsky, I., Bastian, J., DäschleHeisig, D. S., Heisig, S., Peters, J., and Vosseler, C. (2014). International and Local Benefit Sharing in Hydropower Projects on Shared Rivers: the Ruzizi III and Rusumo Falls Cases. Water Policy 16 (6), 1087–1103. doi:10.2166/wp.2014.104
Du Plessis, A. (2017). Global Context of Freshwater Resources. Freshwater Challenges of South Africa and its Upper Vaal River. Springer Series. 3–11. doi:10.1007/978-3-319-49502-6_1
EGL (Energie des Grands Lacs) (2015). Rapports d’étude de faisabilité pour la réhabilitation de Ruzizi I et II. Volume I. Burundi: Bujumbura.
Eisenberg, J. (2018). “Multi-temporal Quantification of Erosion in Selected Catchment Areas of the Ruzizi River (DRC) Using the (R)USLE Model,” in ArcGIS ModelBuilder (10.5) (Mainz, Germany: Master thesis, University of Applied Sciences Mainz).
Eisenberg, J., and Muvundja, F. A. (2020). Quantification of Erosion in Selected Catchment Areas of the Ruzizi River (DRC) Using the (R)USLE Model. Land 9 (4), 125. doi:10.3390/land9040125
Fichtner (2008). Etudes préalables pour l’aménagement d’un site hydroélectrique communautaire Ruzizi III sur la rivière Ruzizi et le développement du volet énergie des activités de la Communauté Economique des Pays des Grands Lacs (CEPGL) (Fichtner, Stuttgart, Germany: Energie des Grands Lacs (EGL)-Union Eur. (UE). Lot 1 Lot 2 Etude de Faisabilité.
Finger, D., Schmid, M., and Wüest, A. (2006). Effects of Upstream Hydropower Operation on Riverine Particle Transport and Turbidity in Downstream Lakes. Water Resour. Res. 42, W08429. doi:10.1029/2005WR004751
Giridharan, L., Venugopal, T., and Jayaprakash, M. (2010). Identification and Evaluation of Hydrogeochemical Processes on River Cooum, South India. Environ. Monit. Assess. 162, 277–289. doi:10.1007/s10661-009-0795-y
Gumbel, E. J. (1941). The Return Period of Flood Flows. Ann. Math. Stat. 12 (2), 163–190. doi:10.1214/aoms/1177731747
Hange, M. S. (2016). Hydrologie et qualité physico-chimique des eaux des rivières Mugera et Mahyu/Nyangezi. Mémoire de Licence, inédit, Département de Chimie-physique, ISP Bukavu, Bukavu, DRC.
Hecky, R. E., Spigel, R. H., and Coulter, G. W. (1991). The Nutrient Regime. Editors G. W. Coulter, and L. Tanganyika, and Its. Life (London, UK: Natural History Museum Publications, Oxford University Press).
Holland, R. A., Scott, K. A., Flörke, M., Brown, G., Ewers, R. M., Farmer, E., et al. (2015). Global Impacts of Energy Demand on the Freshwater Resources of Nations. Proc. Natl. Acad. Sci. U.S.A. 112 (48), E6707–E6716. doi:10.1073/pnas.1507701112
Hughes, D. A. (2001). Providing Hydrological Information and Data Analysis Tools for the Determination of Ecological Instream Flow Requirements for South African Rivers. J. Hydrology 241, 14015–14151. doi:10.1016/S0022-1694(00)00378-4
Hyangya, L., Munini, M., Mushagalusa, M., Kisekelwa, T., Masilya, M. P., Isumbisho, M. P., et al. (2011). Inventaire systématique des macroinvertébrés du lac de barrage de Mumosho sur La rivière Ruzizi (Bukavu, RDC). Bukavu, DRC: Cahiers Du CERUKI/Nouvelle Série Numéro spécial Professeur Alphonse Byamungu N.
IHA (2019). Hydropower Status Report: Sector Trends and Insights. London, England: Report of the International Hydropower Association Limited.
Jadoon, T. R., Ali, M. K., Hussain, S., Wasim, A., and Jahanzaib, M. (2020). Sustaining Power Production in Hydropower Stations of Developing Countries. Sustain. Energy Technol. Assessments 37, 100637. doi:10.1016/j.seta.2020.100637
Jones, N. E. (2014). The Dual Nature of Hydropeaking Rivers: Is Ecopeaking Possible? River Res. applic. 30, 521–526. doi:10.1002/rra.2653
Kahindo, L. B. (2012). “Projets régionaux d’énergie pour aider l’industrialisation du secteur Minier dans l’Est. Cas Du Projet Ruzizi III,” in Conférences IPAD, Kinshasa, 2012. Kinshasa: Ministère de Ressources hydrauliques et Electricité (Kinshasa, RDC.
Kannel, P. R., Lee, S., Lee, Y.-S., Kanel, S. R., and Khan, S. P. (2007). Application of Water Quality Indices and Dissolved Oxygen as Indicators for River Water Classification and Urban Impact Assessment. Environ. Monit. Assess. 132, 93–110. doi:10.1007/s10661-006-9505-1
Karakoyun, Y., Dönmez, A. H., and Yumurtacı, Z. (2018). Comparison of Environmental Flow Assessment Methods with a Case Study on a Runoff River-type Hydropower Plant Using Hydrological Methods. Environ. Monit. Assess. 190, 722. doi:10.1007/s10661-018-7107-3
Khan, M. Y. A., Panwar, S., and Wen, J. (2022). Geochemistry of the Dissolved Load of the Ramganga River, Ganga Basin, India: Anthropogenic Impacts and Chemical Weathering. Front. Environ. Sci. 10, 823385. doi:10.3389/fenvs.2022.823385
Kikuni, B. C. (2016). Hydrologie et qualité physico-chimique des eaux de la rivière Lurhandala en République Démocratique Du Congo. Mémoire de Licence inédit, Département de Chimie-Physique, ISP Bukavu, Bukavu, DRC
King, J., and Louw, D. (1998). Instream Flow Assessments for Regulated Rivers in South Africa Using the Building Block Methodology. Aq. Ecosys. Health Manage. 1, 109–124. doi:10.1080/14634989808656909
Kisekelwa, T., and Isumbisho, M. (2009). Composition quantitative du zooplancton en amont et aval du barrage de Mururu. Cah. Du. CERUKI/Nouv. Sér. 40, 28–33.
Kubaburhanzi, K. C. (2015). Caractérisation du gradient spatial de la salinité des eaux de La rivière Ruzizi et ses implications au sujet de l’hydrologie de son bassin versant. Bukavu, DRC: Mémoire de Licence, inédit, Département de Chimie-physique, ISP Bukavu.
Kuriqi, A., Pinheiro, A. N., Sordo-Ward, A., Bejarano, M. D., and Garrote, L. (2021). Ecological Impacts of Run-Of-River Hydropower Plants-Current Status and Future Prospects on the Brink of Energy Transition. Renew. Sustain. Energy Rev. 142 (2021), 110833. doi:10.1016/j.rser.2021.110833
Lambinon, J., and Reekmans, M. (1980). Le Burundi et les régions voisines : Carrefour de la végétation d’Afrique tropicale et centre potentiel de tourisme culturel dans l’Est de l’Afrique Centrale. Iris Rev. Belg. Echanges Cult. Bujumb. 9, 15–20.
Lidec, G., and Quintero, J. D. (2003). Good and Bad Dams: Environmental Criteria for Site Selection of Hydroelectric Projects. Latin America and Carribean Region. Sustainable Development Working Paper No. 16. Washington, DC: The World Bank.
Linnansaari, T., Monk, W. A, Baird, D. J., and Curry, R. A. (2013). Review of Approaches and Methods to Assess Environmental Flows across Canada and Internationally. Can. Sci. Advis. Secr. VII (039), 75. doi:10.1016/j.ijpara.2007.04.003
Mantel, S. K., Muller, N. W., and Hughes, D. H. (2010). Ecological Impacts of Small Dams on South African Rivers Part 2: Biotic Response – Abundance and Composition of Macroinvertebrate Communities. Water SA. 36 (3), 361–370. ISSN 1816-7950.
Masilya, M. P., Muvundja, F. A., Isumbisho, M., Hyangya, L., Kisekelwa, T., and Kaningini, M. B. (2020). Food and Feeding Habits of Raïamas Moorei Boulenger 1900, Enteromius Pellegrini (Poll, 1939), and Enteromius Kerstenii (Peters, 1868), Three Cyprinid Species of Lake Kivu (East Africa). Environ. Biol. Fish. 103, 635–645. doi:10.1007/s10641-020-00965-w
Matthews, J. H., Forslund, A., McClain, M. E., and Tharme, R. E. (2014). More Than the Fish: Environmental Flows for Good Policy and Governance, Poverty Alleviation and Climate AdaptationWorld Water Week, September 1-6, 2013, Stockholm, Sweden. Aquat. Procedia 2, 16–23. doi:10.1016/j.aqpro.2014.07.004
Mekonnen, M. M., and Hoekstra, A. Y. (2011). “The Water Footprint of Electricity from Hydropower,” in Value of Water Research Report Series 51 (Delft, Netherlands: UNESCO-IHE).
Minella, J. P. G., Merten, G. H., Reichert, J. M., and Clarke, R. T. (2008). Estimating Suspended Sediment Concentrations from Turbidity Measurements and the Calibration Problem. Hydrol. Process. 22, 1819–1830. doi:10.1002/hyp.6763
Möller, P., Rosenthal, E., Geyer, S., Guttman, J., Dulski, P., Rybakov, M., et al. (2007). Hydrochemical Processes in the Lower Jordan Valley and in the Dead Sea Area. Chem. Geol. 239, 27–49. doi:10.1016/j.chemgeo.2006.12.004
Moore, D., Dore, J., and Dipak, G. (2010). The World Commission on Dams + 10: Revisiting the Large Dam Controversy. Water Altern. 3 (2), 3–13.
MPCA (2008). Turbidity: Description, Impact on Water Quality, Sources, and Measures. Minnesota, USA: Minnesota Pollution Control Agency. Available at: https://www.wrc.org.za/wp-content/uploads/mdocs/TT-207-03.pdf.
Muhindo, K. W. (2016). Hydrologie et qualité physico-chimique des eaux de la rivière Cihanda à Nyangezi-Centre, En République Démocratique Du Congo. Bukavu, DRC: Mémoire de Licence, inédit, Département de Chimie-physique, ISP Bukavu.
Munini, M., Hyangya, L., Kulimushi, M., Kisekelwa, T., Masilya, M., Isumbisho, M., et al. (2011). Observations préliminaires sur le régime alimentaire des poissons du lac de barrage de Mumosho sur la rivière Ruzizi (R.D. Congo). Cah. Du. CERUKI/Nouvelle Ser. Numéro spécial Alphonse Byamungu.
Muvundja, F. A., Pasche, N., Bugenyi, F. W. B., Isumbisho, M., Müller, B., Namugize, J.-N., et al. (2009). Balancing Nutrient Inputs to Lake Kivu. J. Gt. Lakes. Res. 35 (3), 406–418. doi:10.1016/j.jglr.2009.06.002
Muvundja, F. A., Wüest, A., Isumbisho, M., Kaningini, M. B., Pasche, N., Rinta, P., et al. (2014). Modelling Lake Kivu Water Level Variations over the Last Seven Decades. Limnologica 47, 21–33. doi:10.1016/j.limno.2014.02.003
Nambajimana, J. d. D., He, X., Zhou, J., Justine, M. F., Li, J., Khurram, D., et al. (2020). Land Use Change Impacts on Water Erosion in Rwanda. Sustainability 12 (1), 50. doi:10.3390/su12010050
ONEC-BAD (2015). Groupe de la Banque Africaine de Dévelopement Projet: centrale hydroélectrique de Ruzizi III (147 MW) Pays: Multinational (Burundi-Rd Congo-Rwanda), Abidjan, Côte d’Ivoire: Banque Africaine de Développement. ONEC-2/ONE. Available at: https://www.afdb.org/sites/default/files/documents/environmental-and-social-assessments/multinational-burundi-rwanda-rdc-projet_hydroelectrique_de_ruzizi_iii-resume_par_-_fr_-_08_2015.pdf.
Otokunefor, T. V., and Biukwu, C. O. (2005). Impact of Refinery Effluent on the Physicochemical Properties of a Water Body in the Niger Delta. Appl. Ecol. Env. Res. 3, 61–72. doi:10.15666/aeer/0301_061072
Pasche, N., Rugema, E., Mugisha, A., Uwasempabuka, A., Tuyisenge, J., and Muzana, A. (2013). Lake Kivu Monitoring Program: Lake-wide Monitoring. Report May 2012 to April 2013, Gisenyi, Rwanda: EWSA, Kigali, 15 August 2013
Pastor, A. V., Ludwig, F., Biemans, H., Hoff, H., and Kabat, P. (2014). Accounting for Environmental Flow Requirements in Global Water Assessments. Hydrol. Earth Syst. Sci. 18 (12), 5041–5059. doi:10.5194/hess-18-5041-2014
Patnaik, K. N. (2005). Studies on Environmental Pollution of Major Industries in Paradip Area. Utkal, India: Ph.D Thesis, Utkal University.
Perkins, R., Wilson, B. N., Gulliver, J. S., and Hanson, B. (2017). Relationship between Suspended Sediment Concentration and Turbidity at Construction Sites. J. Soil Water Conservation 72 (1), 26–35. doi:10.2489/jswc.72.1.26
Ramakrishnaiah, C. R., Sadashivaiah, C., and Ranganna, G. (2009). Assessment of Water Quality Index for the Groundwater in Tumkur Taluk, Karnataka State, India. E-Journal Chem. 6 (2), 523–530. doi:10.1155/2009/757424
Riziki, W. J. (2016). Suivi hydrologique et chimique des eaux de la riviere Ruzizi en amont et en aval des barrages Ruzizi I et II à l'Est de la RD. Congo, mémoire de master, inédit, Département d'Aménagement et Gestion de l'Environnement, UAC. Cotonou, Bénin.
Sahu, P., and Sikdar, P. K. (2008). Hydrochemical Framework of the Aquifer in and Around East Kolkata Wetlands, West Bengal, India. Environ. Geol. 55, 823–835. doi:10.1007/s00254-007-1034-x
Schmid, M., Halbwachs, M., Wehrli, B., and Wüest, A. (2005). Weak Mixing in Lake Kivu: New Insights Indicate Increasing Risk of Uncontrolled Gas Eruption. Geochem. Geophys. Geosyst. 6 (7), 1–11. doi:10.1029/2004GC000892
Şener, Ş., Şener, E., and Davraz, A. (2017). Evaluation of Water Quality Using Water Quality Index (WQI) Method and GIS in Aksu River (SW-Turkey). Sci. Total Environ. 584-585, 131–144. doi:10.1016/j.scitotenv.2017.01.102
Şener, Ş., Davraz, A., and Karagüzel, R. (2013). Evaluating the Anthropogenic and Geologic Impacts on Water Quality of the Eğirdir Lake, Turkey. Environ. Earth Sci. 70, 2527–2544. doi:10.1007/s12665-013-2296-0
Seyler, J., Thomas, D., Mwanza, N., and Mpoyi, A. (2010). Democratic Republic of Congo: Biodiversity and Tropical Forestry Assessment (118/119) No. Final) USAID/Democratic Republic of the Congo.
Sharpley, A. N., McDowell, R. W., and Kleinman, P. J. A. (2001). Phosphorus Loss from Land to Water: Integrating Agricultural and Environmental Management. Plant Soil 237, 287–307. doi:10.1023/A:1013335814593
SHER and ARTELIA (2017). Inventaire des ressources en eau et des usages dans les bassins du Lac Kivu et de La Rivière Rusizi. Kigali, Rwanda: RAPPORT Final.
Smakhtin, V., Revenga, C., and Döll, P. (2004). A Pilot Global Assessment of Environmental Water Requirements and Scarcity. Water Int. 29, 307–317. doi:10.1080/02508060408691785
Smakhtin, V. U., Shilpakar, R. L., and Hughes, D. A. (2006). Hydrology-based Assessment of Environmental Flows: an Example from Nepal. Hydrological Sci. J. 51, 207–222. doi:10.1623/hysj.51.2.207
Soulsby, C., Langan, S. J., and Neal, C. (2001). Environmental Change, Land Use and Water Quality in Scotland: Current Issues and Future Prospects. Sci. Total Environ. 265 (1-3), 387–394. doi:10.1016/S0048-9697(00)00678-1
Strahler, A. N. (1952). Hypsometric (Area-Altitude) Analysis of Erosional Topography. Geol. Soc. Am. Bull. 63, 1117–1142. doi:10.1130/0016-7606(1952)63[1117:haaoet]2.0.co;2
Subramani, T., Rajmohan, N., and Elango, L. (2009). Groundwater Geochemistry and Identification of Hydrogeochemical Processes in a Hard Rock Region, Southern India. Environ. Monit. Assess. 162 (1-4), 123–137. doi:10.1007/s10661-009-0781-4
Tennant, D. L. (1976). Instream Flow Regimens for Fish, Wildlife, Recreation and Related Environmental Resources. Fisheries 1 (4), 37–41. doi:10.1577/1548-8446(1976)001<0006
Tessmann, S. (1980). Environmental Assessment, Technical Appendix E in Environmental Use Sector Reconnaissance Elements of the Western Dakotas Region of South Dakota Study Brookings. South Dakota: Water Resources Institute, South Dakota State University.
Tonolla, D., Bruder, A., and Schweizer, S. (2017). Evaluation of Mitigation Measures to Reduce Hydropeaking Impacts on River Ecosystems - a Case Study from the Swiss Alps. Sci. Total Environ. 574, 594–604. doi:10.1016/j.scitotenv.2016.09.101
TRACTIONELRRI (1980). Projet définitif d’une deuxième centrale hydroélectrique sur la Ruzizi, Hydrologie. Bruxelles- Dortmund.
Truffer, B., Bratrich, C., Markard, J., Peter, A., Wüest, A., and Wehrli, B. (2003). Green Hydropower: the Contribution of Aquatic Science Research to the Promotion of Sustainable Electricity. Aquat. Sci. 65 (2), 99–110. doi:10.1007/s00027-003-0643-z
Tsegaye, T., Sheppard, D., Islam, K. R., Tadesse, W., Atalay, A., Marzen, L., et al. (2006). Development of Chemical Index as a Measure of In-Stream Water Quality in Response to Land-Use and Land Cover Changes. Water Air Soil Pollut. 174, 161–179. doi:10.1007/s11270-006-9090-5
Vandelannoote, A., Deelstra, H., and Ollevier, F. (1999). “The Inflow of the Rusizi River to Lake Tanganyika,” in From Limnology to Fisheries: Lake Tanganyika and Other Large Lakes. Hydrobiologia, Editors O. V. Lindqvist, H. Mölsä, K. Salonen, and J. Sarvala, 407, 65–73. doi:10.1007/978-94-017-1622-2_6
Wang, Q. G., Du, Y. H., Su, Y., and Chen, K. Q. (2012). Environmental Impact Post-assessment of Dam and Reservoir Projects: A Review. Procedia Environ. Sci. 13, 1439–1443. doi:10.1016/j.proenv.2012.01.135
WCD (World Commission on Dams) (2000). Dams and Development: A New Framework for Decision-Making. London: Earth scan publications Ltd.
Welcome, R. L., Bene, C., Brown, C. A., Arthington, A., Dugan, P., King, J. M., et al. (2006). “Predicting the Water Requirements of River Fisheries,” in Wetlands and Natural Resource Management. Ecological Studies (Analysis and Synthesis). Editors J. T. A. Verhoeven, B. Beltman, R. Bobbink, and D. F. Whigham (Berlin, Heidelberg: Springer), Vol 190.
Winemiller, K. O., McIntyre, P. B., Castello, L., Fluet-ChouinardGiarrizzo, E. T., GiarrizzoBaird, T. I. G., Nam, S., et al. (2016). Balancing Hydropower and Biodiversity in the Amazon, Congo, and Mekong. Science 351 (6269), 128–129. doi:10.1126/science.aac7082
Keywords: hydropeaking, reservoir siltation, reservoir pollution, water quality index, minimum hydrological flow
Citation: Muvundja FA, Walumona JR, Dusabe M-C, Alunga GL, Kankonda AB, Albrecht C, Eisenberg J and Wüest A (2022) The Land–Water–Energy Nexus of Ruzizi River Dams (Lake Kivu Outflow, African Great Lakes Region): Status, Challenges, and Perspectives. Front. Environ. Sci. 10:892591. doi: 10.3389/fenvs.2022.892591
Received: 09 March 2022; Accepted: 21 April 2022;
Published: 12 July 2022.
Edited by:
Tatenda Dalu, University of Mpumalanga, South AfricaReviewed by:
Alban Kuriqi, Universidade de Lisboa, PortugalMohd Yawar Ali Khan, King Abdulaziz University, Saudi Arabia
Copyright © 2022 Muvundja, Walumona, Dusabe, Alunga, Kankonda, Albrecht, Eisenberg and Wüest. This is an open-access article distributed under the terms of the Creative Commons Attribution License (CC BY). The use, distribution or reproduction in other forums is permitted, provided the original author(s) and the copyright owner(s) are credited and that the original publication in this journal is cited, in accordance with accepted academic practice. No use, distribution or reproduction is permitted which does not comply with these terms.
*Correspondence: Fabrice Amisi Muvundja, YW1pc2ltdXZAeWFob28uZnI=