- Department of Zoology, Guru Nanak Dev University, Amritsar, India
In addition to chemical pesticides and fertilizers, the use of vermicompost can help in the management of root-knot nematodes (RKN) while also augmenting plant growth. The present study is carried out to determine the role of neem-based vermicompost on plant growth during stress produced by Meloidogyne incognita. Vermicompost (Vcom) and soil were mixed in various proportions (0, 20, 40, 60, 80, and 100%) and used to treat tomato plants against nematode infestation. After 10 days of inoculation of second-stage juveniles of M. incognita, several morphological parameters such as root length, shoot length, root weight, shoot weight, number of galls, and number of leaves were evaluated to investigate the plant growth. Various photosynthetic pigments (chlorophyll a and b, total chlorophyll, and carotenoid content) and gaseous exchange parameters (photosynthesis rate, intercellular carbon dioxide intensity, stomatal conductance, and transpiration rate) were also investigated in order to better understand plant respiration and response to nematode stress. In biochemical studies, the protein content and unit activity of antioxidative enzymes such as catalase, superoxide dismutase, guaiacol peroxidase, glutathione-s-transferase, ascorbate peroxidase, and polyphenol oxidase were investigated. The analyses of malondialdehyde (MDA) and hydrogen peroxide (H2O2) contents were also performed to examine the stress caused by nematodes and the effect of vermicompost in overcoming that stress. Aside from that, the influence of vermicompost on several bioactive components of plants was investigated by quantifying non-antioxidative enzymes (ascorbic acid, glutathione, and tocopherol levels) and secondary metabolites (total phenolic, total flavonoid, and anthocyanin contents). The results of the foregoing experiments reveal a significant increase in all morphological, biochemical, and photosynthetic parameters except MDA and H2O2, which tend to decrease with increasing vermicompost concentration as compared to untreated and nematode-infected plants. The current study reveals that vermicompost has a high potential for lowering the nematode stress and enhancing plant growth and development through the augmentation of different bioactive components in plants.
1 Introduction
Plant tissues are continually targeted by pathogens present within the soil’s rhizosphere (Jacoby et al., 2017). Root-knot nematodes (RKNs) are the most deadly soil-borne parasites, infecting the root systems of a wide range of crops that are economically important (Bali et al., 2020). Nematode infestation affects 10% of the world’s vegetable production, with the Meloidogyne species (root-knot nematodes) accounting for half of these losses (Gautam et al., 2014). The Meloidogyne species are recognized as damaging agricultural pests globally (Mokrini et al., 2018). The plant’s intake of water and nutrients is significantly reduced due to a weakened root system, resulting in susceptibility to other pathogens and low yields (Williamson, 1998). RKNs change morphophysiological processes in hosts, causing stunted growth, increased discoloration, and lowered photosynthetic rates (Mukhtar, 2018). Generally, biotic stressors have a substantial impact on vegetable harvests, and tomato plants are particularly vulnerable to nematode infestation (Abd-Elgawad, 2016). Tomatoes are among the most widely grown crops worldwide. It is regarded as a preventive food owing to its nutritious value, since it includes critical elements such as beta-carotene, lycopene, vitamin C, flavonoids, and hydroxycinnamic acid derivatives (Kumari et al., 2020). Tomato has been identified as a host plant for RKN infection, which causes a complex series of changes in plant cell architecture. RKN feeding sites are recognized as large cells those serve as metabolic sources for RKN, reducing host plant growth and productivity (Bernard et al., 2017). RKN has caused approximately 32–40% of harm to tomato crops in India (Anwar and McKenry, 2012). Chemicals, nematicides, soil treatments, and biocontrol agents, as well as crop rotation, are used to control nematode infestation (Abrar et al., 2020). As the use of chemicals has a negative impact on the environment and can be hazardous to plants when used in high quantities, it is vital to develop innovative and eco-friendly techniques for nematode management (Mukhtar et al., 2014).
Vermicompost is produced by the non-thermophilic biodegradation of organic substances by the interaction of earthworms and microorganisms (Amiri et al., 2017). This organic composition is light, odorless, and free of weed seeds, and it requires less time to be produced (Hosseinzadeh and Ahmadpour, 2018). Plants grow faster with the addition of vermicompost as it includes plant growth hormones and humic acid (Lim et al., 2015; Singh and Singh, 2017). Humic acid promotes root hair development and mineral nutrient discharge in addition to being involved in oxidative phosphorylation, cellular metabolism, photosynthesis, protein synthesis, and numerous enzymatic processes (Singh et al., 2020). Researchers have found that mineral content in vermicompost is available in plant-accessible forms such as phosphates, nitrates, soluble calcium, and potassium (Jangra et al., 2019), in addition to growth factors and valuable microorganisms such as bacteria, fungi, and actinomycetes, making it more adequate and suitable for the overall growth and production of plants (Joshi et al., 2015). Vermicompost is a viable alternative source for higher production along with the management of root-knot nematodes (Ramakrishnan and Mahadevaswamy, 2012). It has been shown in studies to reduce the amount of plant-parasitic nematodes in the soil and their penetration into the roots (Kumar et al., 2011; Ramrao and Pathak, 2013). Furthermore, it is postulated that vermicompost can boost host plant defense against nematodes by increasing the expression of genes those encode for secondary metabolites or altering soil characteristics (Xiao et al., 2016). Depending on the kind of feedstock utilized, vermicompost contains chemicals with nematicidal action (Ntalli et al., 2020). Thus, keeping the preceding findings and requirements in mind, the purpose of this study is to determine the effectiveness of vermicompost on tomato plant development and biochemical reaction during nematode infestation.
2 Materials and Methodology
2.1 Plant Material and Setup of Experiments
Vermicompost was made by blending dried neem leaves with cow manure in 1:2 ratios and employing non-clitellated red wiggler earthworms (Eisenia fetida). Before inoculating the earthworms, pre-composting of the material was done by thoroughly mixing it for 15 days and spraying water. Earthworms were harvested after 60 days of inoculation, and the vermicompost was kept for air drying. Vermicompost extract was prepared by dissolving 5 g of air-dried vermicompost in 50 ml of deionized water and stirred on a magnetic stirrer. Tomato cv. Pusa Ruby seeds were presoaked in various dilutions (0, 20, 40, 60, 80, and 100%) of vermicompost extract before being planted in clay pots. Plants at the true leaf stage (on the 8th day of sowing) were transferred to separate pots having grading mixtures of vermicompost and soil. The transplanted plants were infected with freshly hatched J2s (@2J2/g soil) of M. incognita, and water was sprayed on a regular basis to keep the potting mixture wet. The plants were harvested after 10 days of nematode inoculation.
2.2 Morphological Studies
Several morphological characteristics were evaluated, including root and shoot length, fresh weights of roots and shoots, number of leaves, and number of galls on roots after harvesting.
2.3 Gaseous Exchange Parameters
The portable LI-COR 6400XT Infrared gas analyzer was used to evaluate gaseous exchange characteristics like photosynthesis rate, intercellular CO2 intensity, stomatal conductance, and transpiration rate. Gaseous exchange parameters were measured by calculating the difference between CO2 and H2O in the air passing through the reference cell against air passing across the chamber (sample). The analysis was carried out on a bright day between 11:00 a.m. and 12:00 p.m. The ideal conditions are as follows: relative humidity (70–90%), temperature (25–30°C), photon flux intensity (1,000 μmol m−2 g−1), and carbon dioxide concentration (400 μmol mol−1) (Khanna et al., 2019).
2.4 Biochemical Studies
2.4.1 Protein Content and Antioxidative Enzymes
Fresh Solanum lycopersicum plants were homogenized in a 0.1 M potassium phosphate buffer (pH 7.0) and centrifuged for 20 min at 13,000 rpm at 4°C. The Folin & Ciocalteu's phenol reagent and bovine serum albumin (BSA) were used to determine the protein level. A graph with a known amount of BSA vs. absorbance was created (Lowry et al., 1951).
Activities of different antioxidative enzymes like catalase (CAT) (Aebi, 1974), ascorbate peroxidase (APOX) (Nakano and Asada, 1981), superoxide dismutase (SOD) (Kono, 1978), polyphenol oxidase (PPO) (Kumar and Khan, 1982), guaiacol peroxidase (POD) (Pütter, 1974), and glutathione-S-transferase (GST) (Habig and Jakoby, 1981) of untreated and treated tomato plants were studied using standard protocols. The change in absorption per minute was taken on a UV-VIS spectrophotometer, and unit activity was calculated by using the given formula:
For the SOD enzyme, the enzyme activity was calculated as 50% inhibition of nitro blue tetrazolium (NBT) (dye):
2.4.2 Nonenzymatic Antioxidants
Ascorbic acid content: Plant samples were crushed in 2% metaphosphoric acid and centrifuged at 2,500 rpm for 10 min to prepare the homogenate. In test tubes, 100 µl of plant extract and 200 µl of 5% metaphosphoric acid were added and incubated for 30 min. Then, 500 µl n-amyl alcohol and 320 µl 2,6-dichlorophenolindophenol dye were injected into the mixture, which was then vortexed. The top layer’s absorbance was measured using a UV-VIS spectrophotometer at 546 nm (Bio-Spectrometer). A graph with a known amount of L-ascorbic acid vs. absorbance was formed (Chinoy, 1962).
Glutathione content: The homogenate was made by mashing the plant sample in 50 mM Tris buffer (pH 10), then centrifuging it at 12,000 g at 4°C for 15 min. The supernatant was then diluted with 5,5′-dithiobis(2-nitrobenzoic acid) (DTNB) and methanol and stored at room temperature for 15 min. After incubation, the material was centrifuged at 3,000 rpm for 15 min and the absorbance of the supernatant was taken at 412 nm. To examine the outcomes, a standard plot of known reduced glutathione content vs. absorbance was created (Sedlak, 1982).
Tocopherol content: The homogenate was made in the same manner as the total glutathione content. The supernatant was stirred after adding 100% ethanol and doubly distilled water. Now, xylene was administered, followed by 10 min of centrifugation at 3,000 g at 4°C. The top xylene layer was extracted and mixed with 2,4,6-tripyridyl-s-triazine before taking the absorbance at 600 nm. For assessment, a reference plot was drawn using the known concentration of tocopherol vs. absorbance (Martinek, 1964).
2.5 Stress Indices
Malondialdehyde (MDA) content: A homogenate was made by crushing fresh plant seedlings in 0.1% (w/v) trichloroacetic acid (TCA), then centrifuging it at 4°C at 5,000 rpm. The resulting supernatant was utilized for further investigation. Then, 1,000 µl of the supernatant was combined with 6 ml of 20% (w/v) TCA containing 0.5% (w/v) 2-thiobarbituric acid (TBA) and heated in a water bath at 95°C for 30 min before the reaction was stopped under ice cold surroundings. At 532 nm, the optical density of the supernatant was measured. By subtracting absorbance at 600 nm from the nonspecific absorbance, the nonspecific absorbance was adjusted (Fu and Huang, 2001).
Hydrogen peroxide (H2O2) content: For determining H2O2 content, 500 µl of plant extract, 500 µl of potassium iodide, and 1,000 µl of potassium phosphate buffer were added to a cuvette, and absorbance was measured at 390 nm using a UV-VIS spectrophotometer. A graph was created by employing known concentrations of H2O2 stock solution vs. absorbance (Velikova et al., 2000).
2.6 Photosynthetic Pigments
Chlorophyll and carotenoid contents were estimated by using acetone. Extraction of carotenoid and chlorophyll contents was done using 80% acetone and centrifuged at 4°C at 10,000 rpm. The absorbance of the supernatant was recorded at 480/510 nm and 645/663 nm, using a UV-VIS spectrophotometer (Arnon, 1949).
2.7 Osmolyte (Proline Content)
Proline content was determined by smashing plant sample in sulfosalicylic acid (3%) and filtering through Whatman no. 1 filter paper. In a test tube, equal volumes of plant extract, acid–ninhydrin, and glacial acetic acid were placed and maintained at 100°C for 1 h inside boiling water. Following that, the test tubes were placed in a cold bath to stop the reaction, which proceeded due to the introduction of toluene. The mixture was agitated forcefully on a vortex shaker for 10–15 s and then left undisturbed. On a UV-VIS spectrophotometer, the absorbance of the top layer was recorded at 520 nm. Proline content was determined in milligrams per gram tissue utilizing a solution containing L-proline (Bates et al., 1973).
2.8 Secondary Metabolites
Total Phenolic content: The homogenate was made by grinding the plant material in 80% methanol and centrifuging it for 20 min at 4°C and 10,000 rpm. Test tubes containing 0.1 ml plant extract, 2.9 ml distilled water, 0.5 ml of FC reagent (1:1 with water), and 2 ml of 20% sodium carbonate were swirled on a vortex shaker before being heated in boiling water for 1 min. The reaction was stopped in an ice bucket, and the absorbance at 760 nm was measured using a UV-VIS spectrophotometer. A standard plot was created using known catechol concentrations vs. absorbance (Malik and Singh, 1980).
Total flavonoid content: The above homogenate was also used for the estimation of flavonoid content. Then, 0.1 ml of the supernatant was placed in a test tube and a final volume of 3 ml was obtained by adding methanol. Serially, 0.1 ml of AlCl3⸱6H2O, 0.1 of 5% sodium potassium tartarate, and 0.5 ml of deionized water were introduced to this combination. After 30 min of incubation, the final solution was violently agitated, and the absorbance at 415 nm was measured. A standard plot was created using rutin concentrations that were known vs. absorbance (Lamaison and Carnet, 1990).
Anthocyanin content: The anthocyanin content was determined by grinding fresh S. lycopersicum plant sample in acidified methanol (methanol, distilled water, and hydrochloric acid in a 79:20:1 ratio). The extract was kept at 4°C overnight before being centrifuged at 10,000 g for 15–20 min at 4°C, and absorbance was measured at 530 and 657 nm (Mancinelli, 1990).
2.9 Statistical Analysis
The data were subjected to statistical analysis by using SPSS software version 16.0. Analysis of variance (ANOVA) and Tukey’s test were employed to the observations. Bar graphs were constructed by using GraphPad Prism version 7.00 software packages.
3 Results
3.1 Morphological Parameters
Plant growth was found suppressed in untreated and nematode-inoculated plants. The root length, shoot length, and root and shoot weights were significantly reduced in nematode-stressed plants and nematode galls were formed in the infected roots. Following nematode infection, the root length, shoot length, root weight, shoot weight, and number of leaves were found decreased by 38.57, 28.53, 23.08, 12.82, and 19.26%, respectively. Furthermore, the root and shoot length, as well as fresh weight and number of leaves had increased significantly in Vcom-treated plants (p ≤ 0.01). As nematode-infected tomato plants were treated with 100% Vcom, the root length, root weight, and number of leaves were found increased by 1.52, 2.32, and 1.19 times, whereas the shoot length and shoot weight had increased by 68.17 and 50.54%, respectively, when compared with untreated and nematode-stressed tomato plants (Figure 1) The number of galls significantly declined (61.59%) in Vcom-treated plants and decreased with an increase in Vcom concentration (Table 1).
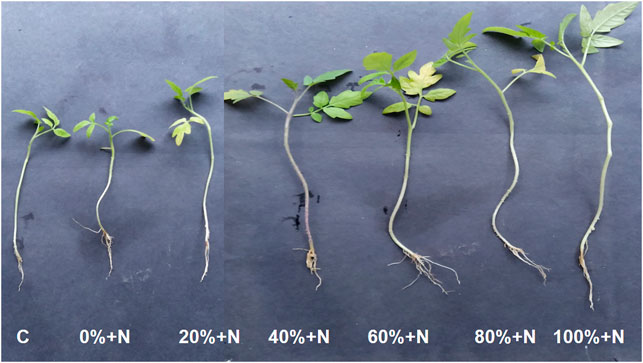
FIGURE 1. Effect of Vcom concentration (0–100%) on nematode-infected tomato plants after 10 days of inoculation. N denotes nematode-infected plants, whereas C denotes control (untreated and uninfected) plants.
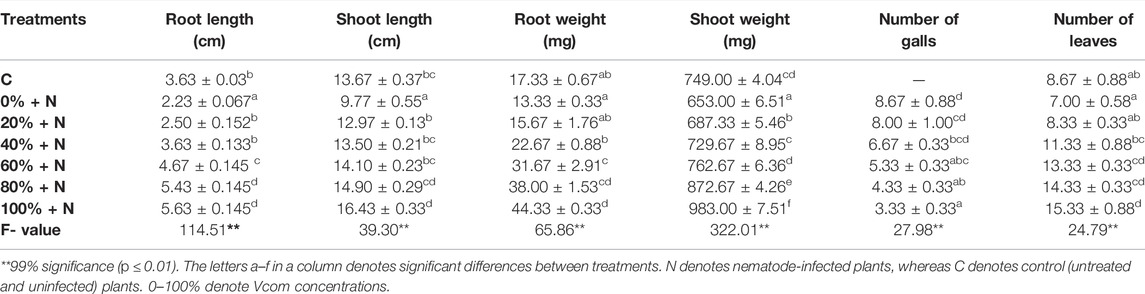
TABLE 1. Effect of vermicompost on morphological parameters of nematode-stressed tomato plants (mean ± SE).
3.2 Gaseous Exchange Parameters
The gaseous exchange parameters were found reduced after nematode infection in tomato plants. Approximately 3.45, 15.30, 27, and 7.63% reduction was observed, respectively, in the photosynthesis rate, intercellular CO2 intensity, stomatal conductance, and transpiration rate after nematode infection. However, all these parameters in nematode-infected and Vcom-treated tomato plants were significantly higher (p ≤ 0.01) than in infected and untreated plants. Approximately 84.52, 61.07, and 30.59% enhancement, respectively, was observed in the photosynthesis rate, intercellular CO2 intensity, and stomatal conductance, whereas 1.28 times enhancement was observed in case of the transpiration rate when nematode-stressed plants were treated with 100% Vcom (Table 2).
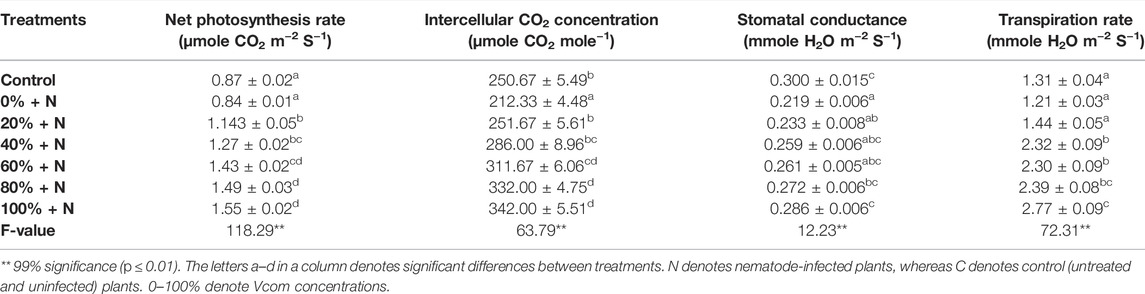
TABLE 2. Effect of vermicompost on gaseous exchange parameters of nematode-stressed tomato plants (mean ± SE).
3.3 Protein Content and Antioxidative Enzymes
The protein levels in Vcom-treated tomato plants were reported to be significantly (p ≤ 0.01) higher than in control plants (Table 3). The protein content of nematode-infected untreated tomato plants was reduced by around 17.13% as compared to the control. However, when 100% Vcom-treated plants were compared to infected and untreated plants, a two-fold elevation was seen.
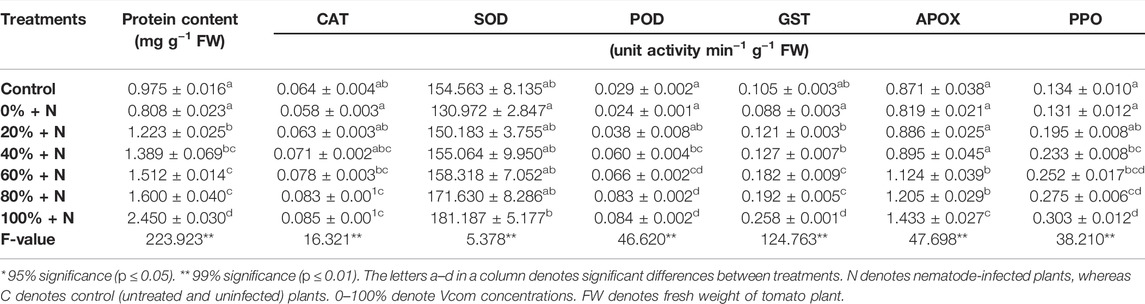
TABLE 3. Effect of vermicompost on protein content and antioxidative enzymes of nematode stressed tomato plants (mean ± SE).
The unit activity of antioxidant enzymes like CAT, SOD, POD, GST APOX, and PPO were found reduced after nematode infection. About 9.38, 15.26, 17.24, 16.19, 5.97, and 2.24% reduction was observed in unit activity of CAT, SOD, POD, GST APOX, and PPO enzymes, respectively, after nematode infection. However, the activities of these enzymes were significantly enhanced (p ≤ 0.01) after Vcom application as compared to infected and untreated plants. When plants were treated with 100% Vcom, then 46.54, 38.33, and 74.97% enhancement was observed in the activity of CAT, SOD, and APOX enzymes, respectively. Similarly, the enzyme activity of POD, GST, and PPO was enhanced by 2.5, 1.93, and 1.31 folds, respectively, in nematode-infected treated plants (Table 3).
3.4 Nonenzymatic Antioxidants
When plants were infected with nematodes, the levels of ascorbic acid, glutathione, and tocopherol were found to be decreased by 3.71, 8.20, and 8.28%, respectively, as compared to the control. Furthermore, when nematode-infested vermicompost-enriched tomato plants were compared to the control, the levels of these were significantly (p ≤ 0.01) higher. As nematode-infected tomato plants were treated with 100% Vcom, ascorbic acid content increased by 46.75%, whereas glutathione and tocopherol contents increased by 1.14 and 1.63 times, respectively, when compared with untreated and nematode-stressed tomato plants (Figure 2).
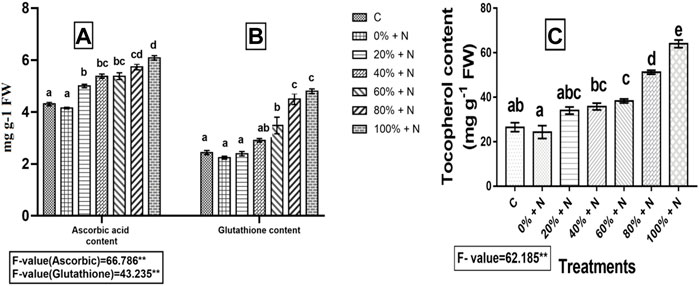
FIGURE 2. Effect of vermicompost on non-enzymatic antioxidants: (A) ascorbic acid content, (B) glutathione content, and (C) tocopherol content of tomato plants infected with nematodes. A bar with different letters (a–e) represents a significant difference (p ≤ 0.01), whereas one with the same letter represents a nonsignificant difference among the treatments. N represents plants with nematode infection, whereas C represents control (untreated and uninfected) plants. 0–100% denote Vcom concentrations. FW denotes fresh weight of tomato plant.
3.5 Stress Indices
The level of stress experienced by the plants is seen by estimating malondialdehyde (MDA) and H2O2 concentrations in the plants. When the plants were subjected to nematode stress, their MDA and H2O2 levels increased by 23.89 and 11.27%, respectively, when compared to the control. MDA and H2O2 contents in all treatments significantly (p ≤ 0.01) decreased after vermicompost treatment. When 100% Vcom-treated plants were compared to untreated and infected plants, a reduction of 39.59 and 39.05% was found for MDA and H2O2 contents, respectively (Figure 3).
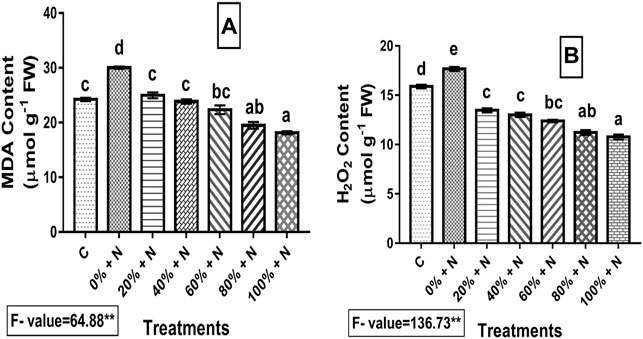
FIGURE 3. Effect of vermicompost on stress indices: (A) MDA and (B) H2O2 concentration of nematode-infected tomato plants. A bar with distinct letters (a–e) denotes a significant difference (p ≤ 0.01) among the treatments, whereas one with the same letter represents a nonsignificant difference. N denotes nematode-infected plants, whereas C denotes control (untreated and uninfected) plants. 0–100% denote Vcom concentrations. FW denotes fresh weight of tomato plant.
3.6 Photosynthetic Pigments
Chl a, Chl b, total Chl, and carotenoid content were decreased by 9.21, 32.86, 22.54 and 34.78%, respectively, in nematode-stressed and untreated plants as compared to the control. However, when plants were treated with Vcom, a significant increase (p ≤ 0.01) in all treated plants was detected. The 100% Vcom treatment showed 101, 85.90, 94.11, and 86.33% enhancement in Chl a, Chl b, total Chl, and carotenoid contents, respectively, as compared to nematode-stressed and untreated plants (Figure 4).
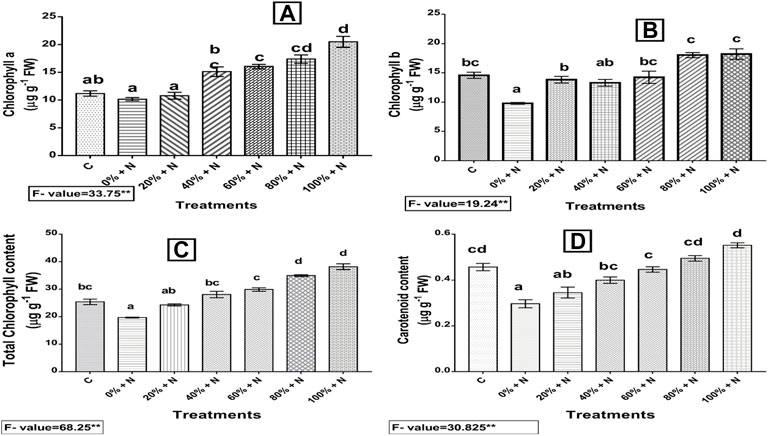
FIGURE 4. Effect of vermicompost on photosynthetic pigments: (A) Chl a, (B) Chl b, (C) total Chl, and (D) carotenoid content of nematode-infected tomato plants. A bar with distinct letters (a–d) denotes a significant difference (p ≤ 0.01) among the treatments, whereas one with the same letter represents a nonsignificant difference. N denotes nematode-infected plants, whereas C denotes control (untreated and uninfected) plants. 0–100% denote the Vcom concentrations. FW denotes fresh weight of tomato plant.
3.7 Osmolyte (Proline Content)
Proline levels in Vcom-treated tomato plants were reported to be significantly (p ≤ 0.01) higher than in the control plants. The Proline content of nematode-infected untreated tomato plants was reduced by around 5.67% as compared to the control. However, when 100% Vcom-treated plants were compared to infected and untreated plants, then 55.67% elevation was seen (Figure 5A).
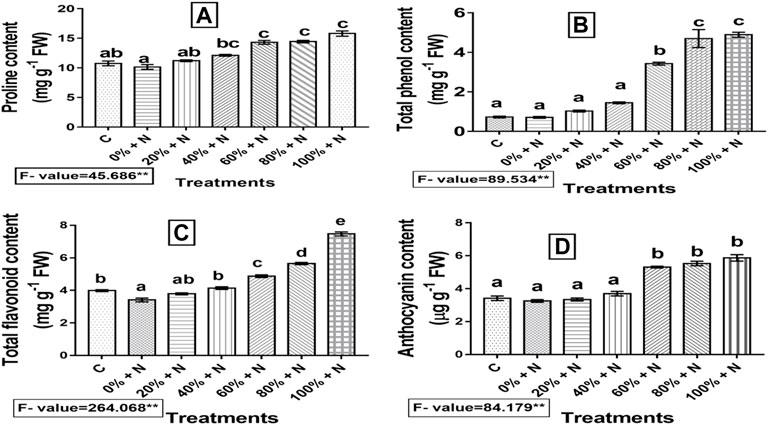
FIGURE 5. Effect of vermicompost on (A) proline content, (B) total phenolic content, (C) total flavonoid content, and (D) anthocyanin content of nematode-infected tomato plants. A bar with distinct letters (a–e) denotes a significant difference (p ≤ 0.01) among the treatments, whereas one with the same letter represents a nonsignificant difference. N denotes nematode-infected plants, whereas C denotes control (untreated and uninfected) plants. 0–100% denote the Vcom concentrations. FW denotes fresh weight of tomato plant.
3.8 Secondary Metabolites
When the plants were infected with nematodes, the levels of total phenolic content, total flavonoid content, and anthocyanin content were found to decrease by 2.74, 14.29, and 4.68%, respectively, as compared to the control. Furthermore, when nematode-infested vermicompost-enriched tomato plants were compared to the controls, their levels were significantly (p ≤ 0.01) higher. As nematode-infected tomato plants were treated with the highest concentration of Vcom, anthocyanin content was increased by 80.06%, whereas phenolic and flavonoid contents were increased by 5.73 and 1.18 times, respectively, when compared with untreated and nematode-stressed tomato plants (Figures 5B–D).
4 Discussion
The present study investigates the potential of vermicompost in boosting plant growth even during biotic stress. When Vcom-treated plants were compared to control seedlings, the overall growth of the plant was improved. Nematode stress caused a significant reduction in plant growth and in their bioactive components such as photosynthetic pigments and enzymatic activity and other metabolites. Earlier studies had shown stunted growth of tomato plants under nematode stress (Kaur et al., 2013; Tikoria et al., 2022). When compared to control seedlings, M. incognita–infected tomato plants had reduced root length, shoot length, root weight, and shoot weight by 22, 5.4, 13, and 5%, respectively (Sharma et al., 2020). Nematode infection reduced plant root and shoot length by 43.2 and 21.5%, respectively, in tomato plants as compared to non-stressed plants. The reduction in plant biomass (fresh weight) of these infected plants was also seen by 35.1% (Khanna et al., 2019). The present study also displays the potential of vermicompost to promote growth. It was also shown earlier that Vcom-treated potato plants (Solanum tuberosum) significantly increased in height and number of leaves in the range of 14–35% as compared to the control (Ezzat et al., 2019). Vermicompost resulted in significant increases of 26, 13, 78, and 57% in yield attributes, overall biomass, shoot biomass, and root biomass in a meta-analysis (Blouin et al., 2019). Another study reveals an increase of 80 and 96%, respectively, in the length of shoot and number of leaves (Rekha et al., 2018). In a comparable research, Vcom-treated pineapple plants increased in plant height and number of leaves by 21 and 2% times, respectively (Mahmud et al., 2020). In field tests, the application of Vcom derived from weeds reduced the root gall index when compared to the control (You et al., 2018). In 14-day-old nematode-stressed tomato plants, Vcom decreased gall development by 48 and 77%, respectively, when compared to inorganic fertilizers and compost and by 17 and 59% in 30-day-old nematode-stressed tomato plants (Xiao et al., 2016).
In our investigation, gaseous exchange parameters such as net photosynthetic rate, intercellular CO2 concentration, stomatal conductance, and transpiration rate were shown to be reduced after nematode exposure but were enhanced after treatment with vermicompost. These results are consistent with a prior study, which discovered that Vcom-derived humic acid produces stomatal opening equivalent to auxin and is involved in stomatal conductance and electron inflow (Azcona et al., 2011; Lotfi et al., 2018). In a similar research, Vcom-treated soybean plants increased stomatal conductance, transpiration rate, and photosynthetic rate by 37, 39, and 62%, respectively (Shahrusvand et al., 2020). Also, the Kinnow Mandarin plant showed a significant increase in photosynthetic parameters like rate of photosynthesis, stomatal conductance, and transpiration rate when these plants were treated with a combination of vermicompost and nitrogen (Pareek et al., 2017). Because of its water-holding capacity and the presence of microorganisms such as mycorrhizal fungi, vermicompost enhances the quantity of water entering the roots, so the water levels rise, and so does the rate of transpiration (Beykkhormizi et al., 2016). Vermicompost has a great capacity for soil aeration, adequate drainage, and reservoirs, and it boosts the CO2 requirement for photosynthesis by restricting the closing of the stomata (Singh et al., 2020). Furthermore, vermicompost has been shown to boost CO2 generation in the rhizosphere. The rise in photosynthesis rate seen in the current experiment as a result of the application of vermicompost is not surprising. Reduced stomatal conductance reduces leaf internal carbon dioxide concentration and, as a result, the activity of Rubisco and photosynthesis rate also decreased (Rahbarian et al., 2011). As a result, any factor that raises stomatal conductance can also raise the photosynthesis rate.
In our study, the protein content and enzyme activities of CAT, SOD, POD, GST, APOX, and PPO were shown to be reduced after nematode exposure but enhanced after treatment with vermicompost. Protein content decreased by 46% after M. incognita infection in tomato plants (Bali et al., 2020). Chickpea plants subjected to water stress have higher protein levels after being supplemented with vermicompost than the controls. Because nitrogen-containing heterocycles are the basic materials needed for protein production, a rise in protein leaf tissue is directly tied to an increasing nitrogen intake by plants (Hosseinzadeh et al., 2016). CAT, APOX, and PPO are physiological parameters that represent plant health (Ezzat et al., 2019). The level of both CAT and APOX was reduced when soil salinity and water stress wheat plants were treated with different concentrations of vermicompost (Hafez et al., 2021). CAT functions as an antioxidant enzyme, eliminating and cleaning generated H2O2 from peroxisomes and reducing the harmful effects of ROS (Rahbarian et al., 2011). SOD is the initial line of defense against radicals in plants and is among the most essential superoxide refiners. Superoxide is converted to H2O2 and O2 as a result of this enzyme activity (Armand et al., 2016). Vcom-treated potato plants significantly increase the activity of CAT, APOX, and PPO in the range of 14–35% as compared to the control. Maximum activity was found in those plants which received maximum concentration of vermicompost (Ezzat et al., 2019).
Nonenzymatic antioxidants such as glutathione, ascorbic acid, and tocopherol were also altered in plants exposed to nematode infection. All of these contents were reduced after nematode infection but significantly increased after vermicompost administration. In a similar research, ascorbic acid and glutathione content levels dropped after nematode inoculation, but they increased significantly in epibrassinolide-treated seedlings (Jasrotia and Ohri 2017). Ascorbic acid transports antioxidants and electrons and functions as an enzyme cofactor and maintains physiological and signaling pathways governed by phytohormones and directly neutralizes reactive oxygen species (ROS) through the use of secondary antioxidants during the lowering of the oxidized form of tocopherol (Akram et al., 2017). Tocopherol is an important plant molecule that works as a cell signaling modulator in a variety of physiological processes, such as mitosis (Mohamed et al., 2019). Glutathione is broadly distributed in many plant tissues and has a contributing role in ROS biotransformation. Furthermore, it aids in the sequestration of heavy metals in vacuoles by the creation of phytochelatins (Sharma and Dietz 2006).
MDA and H2O2 levels were observed to decrease with increasing vermicompost content in the current study. The levels of both MDA and H2O2 were elevated after nematode infection. Vermicompost treatments to these nematode-stressed tomato plants significantly reduced the elevated levels of MDA and H2O2. These findings are consistent with earlier studies which have reported similar findings. After 20 days of infection with M. incognita, the MDA concentration of stressed tomato plants was 10% lower than that in healthy plants (Udalova and Zinovieva 2019). In another study, plants infected with the pine wood nematode (Bursaphelenchus xylophilus) showed a gradual and considerable rise in MDA content (Silva et al., 2021). The H2O2 levels in mango ginger plants grown in heavily deteriorated soil are much greater than in those grown in healthy soil (Singh et al., 2019). The formation of H2O2 has been linked to enhanced pathogenicity of B. xylophilus (Zhang et al., 2019). However, if plant cells are subjected to extreme stress, they may accumulate malondialdehyde (MDA), a byproduct of cell wall lipid peroxidation (Silva et al., 2021). Vcom significantly reduces the elevated levels of MDA and H2O2 induced by nematode stress. Esringü et al. (2016) and García et al. (2016) also discovered a reduction in MDA levels in Hungarian vetch and Oryza sativa after treatment with vermicompost-generated humic acid, respectively. Similarly, 35.60 and 12.47% reduction was observed in MDA and H2O2 levels after tomato seeds were primed with vermicompost extract (Tikoria et al., 2022).
The present study revealed a reduction in the number of photosynthetic pigments in S. lycopersicum seedlings infected with M. incognita. The findings are consistent with a report where M. incognita–stressed tomato plants had a considerable drop in chlorophyll a (10–20%) and carotenoid (16–20%) levels (Udalova and Zinovieva, 2019). In another study, following nematode infection, chlorophyll a, chlorophyll b, and carotenoid levels were lowered by 10, 18, and 20%, respectively (Udalova et al., 2020). In our study, this reduction in photosynthetic pigments was overcome by the application of vermicompost. Enhancements in these pigments were directly proportional to the concentration of vermicompost. The number of photosynthetic pigments such as chlorophyll a, chlorophyll b, total chlorophyll, and carotenoids rose considerably with increasing Vcom dosages (Singh et al., 2019). Hosseinzadeh and Ahmadpour (2018) did a similar study to demonstrate the decline of chlorophyll a, chlorophyll b, total chlorophyll, and carotenoids concentration under extreme water stress. They also discovered that the use of vermicompost was beneficial in combating this decline. The usage of vermicompost boosted the carotenoid content through boosting nitrogen, phosphorus, and potassium absorption, as well as the involvement of these elements in the formation of photosynthetic organs (Mahmud et al., 2020). In a related study, Vcom-treated soybean plants boosted their chlorophyll a, chlorophyll b, total chlorophyll, and carotenoid content by 42.33, 40.65, 41.52, and 46.21%, respectively (Shahrusvand et al., 2020). Increased photosynthetic rate most likely resulted in enhanced protein content and other growth indices. This may be determined based on a rise in the level of all photosynthetic pigments (Singh et al., 2019).
Proline content was reduced following nematode infection in our study, but it was dramatically enhanced after Vcom supplementation. An elevated proline level in relation to stress is an evidence of a plant’s reaction to water deficiency stress. Plants that are more resistant to stress have a greater ability to synthesize proline (Hosseinzadeh et al., 2016). A high concentration of proline in cells during stress conditions shields cells and avoids toxicity from damaging plant cells (Rahbarian et al., 2011). Our study coincides with an earlier report which reveals that after Vcom treatment, proline content significantly increases with an increase in the concentration of Vcom (Singh et al., 2019). Because proline is a nitrogen-containing molecule, higher N absorption by plants leads to increased proline production, which results in increased proline concentration in plants (Furlan et al., 2020). In our investigation, we discovered a substantial rise in total phenol, flavonoid, and anthocyanin content after Vcom treatment. The total phenol and flavonoid contents were increased after vermicompost treatment by 36 and 37%, respectively, in Dracocephalum moldavica L. under moisture stress (Rahimi et al., 2021). Furthermore, an RKN-related study in Oryza sativa revealed higher levels of total phenols in plants. They revealed phenol-induced lignifications in plant epidermal areas as a chronic and inducible post-penetration strategy for nematode resistance. In another study, an elevation in anthocyanin levels in Arabidopsis thaliana attacked with nematodes was seen as an essential antioxidant in shielding the plants from infestation (Labudda et al., 2018). The total flavonoid content of tomato plants exhibited an extremely significant rise after 7 days of M. incognita infection (Jasrotia and Ohri, 2017). After vermicompost application, the anthocyanin and flavonoid levels of Hibiscus sabdariffa L. increased in a similar way (Ghayoumi-Mohammadi and Asadi-Gharneh, 2019).
5 Conclusion
The current investigation demonstrates the impact of neem-based vermicompost in boosting tomato plant growth under nematode stress. The protein content and anti-oxidative enzyme levels in tomato seedlings increased considerably after the administration of vermicompost. In addition to enzymes, nonenzymatic antioxidants such as ascorbic acid and glutathione concentrations increased in tomato plants fed with vermicompost. Vermicompost also aided in the reduction of MDA and H2O2 levels, both of which are considered plant stress signals. As a consequence, the findings of this study reveal that neem-based vermicompost has a potential role in reducing the oxidative stress caused by nematode invasion and increasing plant tolerance by boosting plant growth even during nematode stress.
Data Availability Statement
The original findings given in the study are provided in the article/Supplemental Material. Further inquiries should be made to the corresponding authors.
Author Contributions
Conceptualization and validation: RT, AK, and PO. Methodology: RT. Supervision and investigation: PO and AK.
Conflict of Interest
The authors declare that the research was conducted in the absence of any commercial or financial relationships that could be construed as a potential conflict of interest.
Publisher’s Note
All claims expressed in this article are solely those of the authors and do not necessarily represent those of their affiliated organizations, or those of the publisher, the editors, and the reviewers. Any product that may be evaluated in this article, or claim that may be made by its manufacturer, is not guaranteed or endorsed by the publisher.
Acknowledgments
The authors would like to express their gratitude toward the Council of Scientific and Industrial Research (CSIR), New Delhi, India [File no. 09/254 (0287)/2018-EMR-I] for their financial support in completing this study.
References
Abd-Elgawad, M. M. M. (2016). Biological Control Agents of Plant-Parasitic Nematodes. Egypt. J. Biol. Pest Control 26, 423–429.
Aebi, H. (1974). “Catalase,” in Methods of Enzymatic Analysis. Editor H. U. Bergmeyer (New York and London: Academic Press), 673–684. doi:10.1016/b978-0-12-091302-2.50032-3
Akram, N. A., Shafiq, F., and Ashraf, M. (2017). Ascorbic Acid-A Potential Oxidant Scavenger and its Role in Plant Development and Abiotic Stress Tolerance. Front. Plant Sci. 8, 613. doi:10.3389/fpls.2017.00613
Amiri, H., Ismaili, A., and Hosseinzadeh, S. R. (2017). Influence of Vermicompost Fertilizer and Water Deficit Stress on Morpho-Physiological Features of Chickpea (Cicer arietinumL.Cv. Karaj). Compost Sci. Util. 25, 152–165. doi:10.1080/1065657X.2016.1249313
Anwar, S. A., and McKenry, M. V. (2012). Incidence and Population Density of Plant-Parasitic Nematodes Infecting Vegetable Crops and Associated Yield Losses in Punjab, Pakistan. Pak. J. Zool. 44, 327–333.
Armand, N., Amiri, H., and Ismaili, A. (2016). Interaction of Methanol Spray and Water-Deficit Stress on Photosynthesis and Biochemical Characteristics ofPhaseolus vulgarisL. Cv. Sadry. Photochem Photobiol. 92, 102–110. doi:10.1111/php.12548
Arnon, D. I. (1949). Copper Enzymes in Isolated Chloroplasts. Polyphenoloxidase in Beta Vulgaris. Plant Physiol. 24, 1–15. doi:10.1104/pp.24.1.1
Azcona, I., Pascual, I., Aguirreolea, J., Fuentes, M., García‐Mina, J. M., and Sánchez‐Díaz, M. (2011). Growth and Development of Pepper Are Affected by Humic Substances Derived from Composted Sludge. Z. Pflanzenernähr. Bodenk. 174, 916–924. doi:10.1002/jpln.201000264
Bali, S., Kaur, P., Jamwal, V. L., Gandhi, S. G., Sharma, A., Ohri, P., et al. (2020). Seed Priming with Jasmonic Acid Counteracts Root Knot Nematode Infection in Tomato by Modulating the Activity and Expression of Antioxidative Enzymes. Biomolecules 10, 98. doi:10.3390/biom10010098
Bates, L. S., Waldren, R. P., and Teare, I. D. (1973). Rapid Determination of Free Proline for Water-Stress Studies. Plant Soil 39, 205–207. doi:10.1007/bf00018060
Bernard, G. C., Egnin, M., and Bonsi, C. (2017). “The Impact of Plant-Parasitic Nematodes on Agriculture and Methods of Control,” in Nematology - Concepts, Diagnosis and Control. Editor M. M. Shah (London, United Kingdom: InTech), 121–151. doi:10.5772/intechopen.68958
Beykkhormizi, A., Abrishamchi, P., Ganjeali, A., and Parsa, M. (2016). Effect of Vermicompost on Some Morphological, Physiological and Biochemical Traits of Bean (Phaseolus vulgarisL.) under Salinity Stress. J. Plant Nutr. 39, 883–893. doi:10.1080/01904167.2015.1109104
Blouin, M., Barrere, J., Meyer, N., Lartigue, S., Barot, S., and Mathieu, J. (2019). Vermicompost Significantly Affects Plant Growth. A Meta-Analysis. Agron. Sustain. Dev. 39, 1–15. doi:10.1007/s13593-019-0579-x
Chinoy, J. J. (1962). Formation and Utilization of Ascorbic Acid in Shoot Apex of Wheat: as Factor of Growth and Development. Indian J. Plant Physiol. 5, 172–201.
Esringü, A., Kaynar, D., Turan, M., and Ercisli, S. (2016). Ameliorative Effect of Humic Acid and Plant Growth-Promoting Rhizobacteria (PGPR) on Hungarian Vetch Plants under Salinity Stress. Commun. Soil Sci. Plant Analysis 47, 602–618. doi:10.1080/00103624.2016.1141922
Ezzat, A. S., Badway, A. S., and Abdelkader, A. E. (2019). Sequenced Vermicompost, glycine Betaine, Proline Treatments Elevate Salinity Tolerance in Potatoes. Middle East J. Agric. Res. 8, 126–138.
Fu, J., and Huang, B. (2001). Involvement of Antioxidants and Lipid Peroxidation in the Adaptation of Two Cool-Season Grasses to Localized Drought Stress. Environ. Exp. Bot. 45, 105–114. doi:10.1016/S0098-8472(00)00084-8
Furlan, A. L., Bianucci, E., Giordano, W., Castro, S., and Becker, D. F. (2020). Proline Metabolic Dynamics and Implications in Drought Tolerance of Peanut Plants. Plant Physiology Biochem. 151, 566–578. doi:10.1016/j.plaphy.2020.04.010
García, A. C., Santos, L. A., de Souza, L. G. A., Tavares, O. C. H., Zonta, E., Gomes, E. T. M., et al. (2016). Vermicompost Humic Acids Modulate the Accumulation and Metabolism of ROS in Rice Plants. J. Plant Physiology 192, 56–63. doi:10.1016/j.jplph.2016.01.008
Ghayoumi-Mohammadi, N., and Asadi-Gharneh, H. A. (2019). Effects of Foliar Application and Use of Vermicompost on Quantitative and Qualitative Characteristics of Roselle (Hibiscus sabdariffa L.). Iran. J. Med. Aromat. Plants Res. 34, 881–887. doi:10.22092/ijmapr.2019.115698.215
Habig, W. H., and Jakoby, W. B. (1981). [51] Assays for Differentiation of Glutathione S-Transferases. Methods Enzymol. 77, 398–405. doi:10.1016/S0076-6879(81)77053-8
Hafez, E. M., Omara, A. E. D., Alhumaydhi, F. A., and El‐Esawi, M. A. (2021). Minimizing Hazard Impacts of Soil Salinity and Water Stress on Wheat Plants by Soil Application of Vermicompost and Biochar. Physiol. Plant. 172, 587–602. doi:10.1111/ppl.13261
Hosseinzadeh, S. R., and Ahmadpour, R. (2018). Evaluation of Vermicompost Fertilizer Application on Growth, Nutrient Uptake and Photosynthetic Pigments of Lentil (Lens Culinaris Medik.) under Moisture Deficiency Conditions. J. Plant Nutr. 41, 1276–1284. doi:10.1080/01904167.2018.1450419
Hosseinzadeh, S. R., Amiri, H., and Ismaili, A. (2016). Effect of Vermicompost Fertilizer on Photosynthetic Characteristics of Chickpea (Cicer Arietinum L.) under Drought Stress. Photosynt. 54, 87–92. doi:10.1007/s11099-015-0162-x
Jacoby, R., Peukert, M., Succurro, A., Koprivova, A., and Kopriva, S. (2017). The Role of Soil Microorganisms in Plant Mineral Nutrition-Current Knowledge and Future Directions. Front. Plant Sci. 8, 1617. doi:10.3389/fpls.2017.01617
Jangra, M., Sindhu, S., Sonika, R. G., and Batra, V. (2019). Studies on Efficacy of Vermicompost for the Management of Polyphagotarsonemus Latus (Banks) (Acari: Tarsonemidae) Infesting Chilli (Capsicum Annuum L.) in Haryana Effect of Organic Manure (FYM) in Management Practices of Chilli Mite. Polyphagotarsonemus Latus. J. Pharm. Innov. 8, 86–89.
Jasrotia, S., and Ohri, P. (2017). 24-Epibrassinolide Reduces Stress in Nematode-Infected Tomato (Solanum lycopersicum L.) Plants Cultured In Vitro. Vitro Cell. Dev.Biol.-Plant 53, 538–545. doi:10.1007/s11627-017-9859-9
Joshi, R., Singh, J., and Vig, A. P. (2015). Vermicompost as an Effective Organic Fertilizer and Biocontrol Agent: Effect on Growth, Yield and Quality of Plants. Rev. Environ. Sci. Biotechnol. 14, 137–159. doi:10.1007/s11157-014-9347-1
Kaur, R., Ohri, P., and Bhardwaj, R. (2013). Effect of 28-homobrassinolide on Susceptible and Resistant Cultivars of Tomato after Nematode Inoculation. Plant Growth Regul. 71, 199–205. doi:10.1007/s10725-013-9820-9
Khanna, K., Sharma, A., Ohri, P., Bhardwaj, R., Abd_Allah, E. F., Hashem, A., et al. (2019). Impact of Plant Growth Promoting Rhizobacteria in the Orchestration of Lycopersicon esculentum Mill. Resistance to Plant Parasitic Nematodes: A Metabolomic Approach to Evaluate Defense Responses under Field Conditions. Biomolecules 9, 676. doi:10.3390/biom9110676
Kono, Y. (1978). Generation of Superoxide Radical during Autoxidation of Hydroxylamine and an Assay for Superoxide Dismutase. Archives Biochem. Biophysics 186, 189–195. doi:10.1016/0003-9861(78)90479-4
Kumar, K. B., and Khan, P. A. (1982). Peroxidase and Polyphenol Oxidase in Excised Ragi (Eleusine Corocana Cv PR 202) Leaves during Senescence. Indian J. Exp. Biol. 20, 412–416.
Kumar, K. R., Nattuthurai, K. N., and Gurusamy, R. (2011). Influence of Vermicompost in Root-Knot Nematode Management as a Function of Soil Fortification. Elixir Agric. 38, 4210–4213.
Kumari, M., Sharma, M. K., and Baheti, B. L. (2020). Biological Control of Root-Knot Nematode , Meloidogyne incognita Infecting Tomato ( Solanum lycopersicum ). J. Entomol. Zool. Stud. 8, 35–39.
Labudda, M., Różańska, E., Czarnocka, W., Sobczak, M., and Dzik, J. M. (2018). Systemic Changes in Photosynthesis and Reactive Oxygen Species Homeostasis in Shoots ofArabidopsis Thalianainfected with the Beet Cyst nematodeHeterodera Schachtii. Mol. Plant Pathol. 19, 1690–1704. doi:10.1111/mpp.12652
Lamaison, J., and Carnet, A. (1990). Main Flavonoids content of Crataegus Monogyna Jacq and Crataegus Laevigata (Poiret D. C) flowers depending on the Vegetation (en). Pharm. Acta Helv. 65, 315–320.
Lim, S. L., Wu, T. Y., Lim, P. N., and Shak, K. P. Y. (2015). The Use of Vermicompost in Organic Farming: Overview, Effects on Soil and Economics. J. Sci. Food Agric. 95, 1143–1156. doi:10.1002/jsfa.6849
Lotfi, R., Kalaji, H. M., Valizadeh, G. R., Behrozyar, E., Hemati, A., Gharavi-Kochebagh, P., et al. (2018). Effects of Humic Acid on Photosynthetic Efficiency of Rapeseed Plants Growing under Different Watering Conditions. Photosynt. 56, 962–970. doi:10.1007/s11099-017-0745-9
Lowry, O., Rosebrough, N., Farr, A. L., and Randall, R. (1951). Protein Measurement with the Folin Phenol Reagent. J. Biol. Chem. 193, 265–275. doi:10.1016/s0021-9258(19)52451-6
Mahmud, M., Abdullah, R., and Yaacob, J. S. (2020). Effect of Vermicompost on Growth, Plant Nutrient Uptake and Bioactivity of Ex Vitro Pineapple (Ananas Comosus Var. MD2). Agronomy 10, 1333. doi:10.3390/agronomy10091333
Malik, C. P., and Singh, M. B. (1980). Plant Enzymology and Histo-Enzymology. New Delhi: Kalyani Publishers.
Mancinelli, A. L. (1990). Interaction between Light Quality and Light Quantity in the Photoregulation of Anthocyanin Production. Plant Physiol. 92, 1191–1195. doi:10.1104/pp.92.4.1191
Martinek, R. G. (1964). Method for the Determination of Vitamin E (Total Tocopherols) in Serum. Clin. Chem. 10, 1078–1086. doi:10.1093/clinchem/10.12.1078
Mohamed, A. S., Ali, A. A. M., and Ibrahim, D. S. S. (2019). Potential of Vermicompost and Vermicompost Tea to Improve Yield and Quality of Kalamata Olive Trees Infected with Root-Knot Nematode, Meloidogyne incognita. World J. Agric. Sci. 15, 414–424. doi:10.5829/idosi.wjas.2019.414.424
Mokrini, F., Janati, S., Houari, A., Essarioui, A., Bouharroud, R., and Mimouni, A. (2018). Management of Plant Parasitic Nematodes by Means of Organic Amendment. Rev. Mar. Sci. Agron. Vét. 6 (3), 337–344.
Mukhtar, T., Hussain, M. A., Kayani, M. Z., and Aslam, M. N. (2014). Evaluation of Resistance to Root-Knot Nematode (Meloidogyne incognita) in Okra Cultivars. Crop Prot. 56, 25–30. doi:10.1016/j.cropro.2013.10.019
Mukhtar, T. (2018). Management of Root-Knot Nematode, Meloidogyne incognita, in Tomato with Two Trichoderma Species. Pjz 50, 1589–1592. doi:10.17582/journal.pjz/2018.50.4.sc15
Nakano, Y., and Asada, K. (1981). Hydrogen Peroxide Is Scavenged by Ascorbate-specific Peroxidase in Spinach Chloroplasts. Plant Cell. Physiol. 22, 867–880.
Ntalli, N., Adamski, Z., Doula, M., and Monokrousos, N. (2020). Nematicidal Amendments and Soil Remediation. Plants 9, 429. doi:10.3390/plants9040429
Nunes da Silva, M., Santos, C. S., Cruz, A., López-Villamor, A., and Vasconcelos, M. W. (2021). Chitosan Increases Pinus pinaster Tolerance to the Pinewood Nematode (Bursaphelenchus Xylophilus) by Promoting Plant Antioxidative Metabolism. Sci. Rep. 11, 1–10. doi:10.1038/s41598-021-83445-0
Pareek, P. K., Bhatnagar, P., Bola, P. K., and Kumar, S. (2017). Chemical Science Review and Letters Impact of Nitrogen and Vermicompost Interaction on Photosynthetic Efficiency Parameters of Kinnow Mandarin in Jhalawar District. Chem. Sci. Rev. Lett. 6, 806–811.
Pütter, J. (1974). “Peroxidases,” in Methods of Enzymatic Analysis. Editor H. U. Bergmeyer (New York: USA Academic Press), 685–690. doi:10.1016/b978-0-12-091302-2.50033-5
Rahbarian, R., Khavari-Nejad, R., Ganjeali, A., Bagheri, A., and Najafi, F. (2011). Drought Stress Effects on Photosynthesis, Chlorophyll Fluorescence and Water Relations in Tolerant and Susceptible Chickpea (Cicer Arietinum L.) Genotypes. Acta Biol. Cracoviensia Ser. Bot. 53, 47–56. doi:10.2478/v10182-011-0007-2
Rahimi Jahangirlou, M., Akbari, G. A., Alahdadi, I., Soufizadeh, S., Kumar, U., and Parsons, D. (2021). Phenotypic Traits, Grain Yield and Yield Components of Maize Cultivars under Combinations of Management Practices in Semi-arid Conditions of Iran. Int. J. Plant Prod. 15, 459–471. doi:10.1007/s42106-021-00151-7
Ramakrishnan, S., and Mahadevaswamy, M. (2012). Efficacy of Vermicompost against Root-Knot Nematodes, Meloidogyne Spp. In Flue Cured virginia (FCV) Tobacco. Indian J. Nematol. 42, 143–145.
Ramrao, D., and Pathak, K. (2013). Impact of Vermicompost on Nematode Population at Different Moisture Levels. Indian J. Nematol. 43, 115–122.
Rekha, G. S., Kaleena, P. K., Elumalai, D., Srikumaran, M. P., and Maheswari, V. N. (2018). Effects of Vermicompost and Plant Growth Enhancers on the Exo-Morphological Features of Capsicum Annum (Linn.) Hepper. Int. J. Recycl Org. Waste Agric. 7, 83–88. doi:10.1007/s40093-017-0191-5
Sedlak, J. (1982). Changes of Glutathione and Protein Bound SH-Groups Concentration in Rat Adrenals under Acute and Repeated Stress. Endocrinol. Exp. 16, 103–109.
Seman, A., Awol, S., and Mashilla, D. (2020). Integrated Management of Meloidogyne incognita in Tomato (Solanum lycopersicum) through Botanical and Intercropping. Afr. J. Agric. Res. 15, 492–501. doi:10.5897/ajar2019.14040
Shahrusvand, S., Eisvand, H. R., Firouzabadi, F. N., and Feizian, M. (2020). Soybean Photosynthesis Responses, Yield, and Grain Quality Affected by Vermicompost and Sulfur. Iran. J. Plant Physiol. 10, 3233–3241.
Sharma, N., Khanna, K., Manhas, R. K., Bhardwaj, R., Ohri, P., Alkahtani, J., et al. (2020). Insights into the Role of Streptomyces Hydrogenans as the Plant Growth Promoter, Photosynthetic Pigment Enhancer and Biocontrol Agent against Meloidogyne incognita in Solanum lycopersicum Seedlings. Plants 9, 1109. doi:10.3390/plants9091109
Sharma, S. S., and Dietz, K.-J. (2006). The Significance of Amino Acids and Amino Acid-Derived Molecules in Plant Responses and Adaptation to Heavy Metal Stress. J. Exp. Bot. 57, 711–726. doi:10.1093/jxb/erj073
Singh, A., Karmegam, N., Singh, G. S., Bhadauria, T., Chang, S. W., Awasthi, M. K., et al. (2020). Earthworms and Vermicompost: an Eco-Friendly Approach for Repaying Nature's Debt. Environ. Geochem. Health 42, 1617–1642. doi:10.1007/s10653-019-00510-4
Singh, A., and Singh, G. S. (2017). Vermicomposting: A Sustainable Tool for Environmental Equilibria. Environ. Qual. Manag. 27, 23–40. doi:10.1002/tqem.21509
Singh, P. K., Sahu, P., Pratap, S. G., and Tandon, P. K. (2019). Effect of Vermicompost Developed from Municipal Solid Waste on Rhizome Yield, Photosynthetic Pigments and Metabolism of Mango Ginger (Curcuma Amada Roxb.) Grown in Highly Degraded Sodic Soil. Hort. J. 3, 252–259. doi:10.15406/hij.2019.03.00139
Surendra, K. G., Gamini, S., Bhumesh, K. V., and Aditi, N. P. (2014). Status of Root-Knot Nematode (Meloidogyne Species) Disease in Vegetable Crops of Some Districts of Central Plain Region of Chhattisgarh State, India. Afr. J. Microbiol. Res. 8, 1663–1671. doi:10.5897/ajmr2014.6691
Tikoria, R., Kaur, A., and Ohri, P. (2022). Potential of Vermicompost Extract in Enhancing the Biomass and Bioactive Components along with Mitigation of Meloidogyne incognita-induced Stress in Tomato. Environ. Sci. Pollut. Res. 8. doi:10.1007/s11356-022-19757-z
Udalova, Z. V., Folmanis, G. E., Fedotov, M. A., Pelgunova, L. A., Krysanov, E. Y., Khasanov, F. K., et al. (2020). Effects of Silicon Nanoparticles on Photosynthetic Pigments and Biogenic Elements in Tomato Plants Infected with Root-Knot Nematode Meloidogyne incognita. Dokl. Biochem. Biophys. 495, 329–333. doi:10.1134/S1607672920060150
Udalova, Z. V., and Zinovieva, S. V. (2019). Effect of Salicylic Acid on the Oxidative and Photosynthetic Processes in Tomato Plants at Invasion with Root-Knot Nematode Meloidogyne incognita (Kofoid Et White, 1919) Chitwood, 1949. Dokl. Biochem. Biophys. 488, 350–353. doi:10.1134/S160767291905017X
Velikova, V., Yordanov, I., and Edreva, A. (2000). Oxidative Stress and Some Antioxidant Systems in Acid Rain-Treated Bean Plants. Plant Sci. 151, 59–66. doi:10.1016/s0168-9452(99)00197-1
Williamson, V. M. (1998). Root-knot Nematode Resistance Genes in Tomato and Their Potential for Future Use. Annu. Rev. Phytopathol. 36, 277–293. doi:10.1146/annurev.phyto.36.1.277
Xiao, Z., Liu, M., Jiang, L., Chen, X., Griffiths, B. S., Li, H., et al. (2016). Vermicompost Increases Defense against Root-Knot Nematode (Meloidogyne incognita) in Tomato Plants. Appl. Soil Ecol. 105, 177–186. doi:10.1016/j.apsoil.2016.04.003
You, X., Tojo, M., Ching, S., and Wang, K.-H. (2018). Effects of Vermicompost Water Extract Prepared from Bamboo and Kudzu against Meloidogyne incognita and Rotylenchulus Reniformis. Rotylenchulus Reniformis. J. Nematol. 50, 569–578. doi:10.21307/jofnem-2018-054
Keywords: vermicompost, root-knot nematode, plant growth, biotic stress, bioactive components
Citation: Tikoria R, Kaur A and Ohri P (2022) Modulation of Various Phytoconstituents in Tomato Seedling Growth and Meloidogyne incognita–Induced Stress Alleviation By Vermicompost Application. Front. Environ. Sci. 10:891195. doi: 10.3389/fenvs.2022.891195
Received: 07 March 2022; Accepted: 06 June 2022;
Published: 13 July 2022.
Edited by:
Sharanpreet Singh, Shri Guru Arjun Dev Group of Institutes, IndiaReviewed by:
Shivika Datta, Doaba College Jalandhar, IndiaSwarndeep Hundal, Punjab Agricultural University, India
Copyright © 2022 Tikoria, Kaur and Ohri. This is an open-access article distributed under the terms of the Creative Commons Attribution License (CC BY). The use, distribution or reproduction in other forums is permitted, provided the original author(s) and the copyright owner(s) are credited and that the original publication in this journal is cited, in accordance with accepted academic practice. No use, distribution or reproduction is permitted which does not comply with these terms.
*Correspondence: Puja Ohri, b2hyaV9wdWphXzExQHJlZGlmZm1haWwuY29t