- 1Department of Energy and Technology, Swedish University of Agricultural Sciences, Uppsala, Sweden
- 2Civil Engineering Department and the Future Water Institute, University of Cape Town, Cape Town, South Africa
Alkaline earth hydroxides are widely used in water and wastewater treatment. Within the emerging niche of source-separating sanitation, these chemicals have found a new application—to prevent urease-catalysed degradation of urea present in freshly excreted human urine. However, little is known about the dissolution behaviour of these hydroxides in biological fluids like human urine. Herein, we investigate the solubility of Mg(OH)2 and examine factors that govern its dissolution in different types of urine (real fresh urine, synthetic fresh urine, synthetic dephosphatised fresh urine and real fresh urine concentrated by CO2-free drying). We report experimentally determined as well as thermodynamically simulated data on Mg(OH)2 solubility, dissolution kinetics, and chemical speciation in urine. We find that it takes between 6 and 16 min for Mg(OH)2 to dissolve and the average solubility in real fresh urine at 25°C to be 650 mg L−1. We show that solubility is influenced mainly by concentration of organic compounds, soluble phosphate, and magnesium excreted in fresh urine. When fresh urine is supersaturated with Mg(OH)2, the pH increases to >10.5 and urease-catalysed degradation of urea is inhibited for >14 days. Removing 95% water present in urine increases the solubility of Mg(OH)2 to 16,240 mg L−1 but reduces pH to < 10. Because relative increase in Mg(OH)2 solubility decreases as more water is removed and the solubility is retrograde with respect to temperature, to increase the urine pH to >10 and prevent enzymatic ureolysis, the temperature must be kept < 29°C at 75% water removal and < 22°C at 95% water removal. We find this dissolution behaviour of Mg(OH)2 in concentrated urine solutions to be unlike other alkaline earth hydroxides. These findings have significant implications for the design of new sanitation systems that separately collect and recycle plant-essential nutrients present in human urine.
Introduction
Compounds containing the alkaline Earth metals magnesium and calcium are abundant in nature (Bray and Ghalayin, 2020) and used widely in the wastewater treatment sector for a variety of applications, including the removal of eutrophying nutrients (Ahmad et al., 2020), odours (Jefferson et al., 2002), organic pollutants (Nie et al., 2019), heavy metals (Dhakal et al., 2005), reactive dyes (Li et al., 2016) and microplastics (Zhang et al., 2021). Within the niche of source separation, where technologies are being developed to separately collect, treat, and recycle different fractions of domestic wastewater (Larsen et al., 2013), a new use for these chemicals has recently emerged. It involves using alkaline Earth monoxides and hydroxides to increase the pH of fresh source-separated human urine, which is usually < 7 at excretion (Rose et al., 2015), to >10 in order to prevent the natural degradation of urea that occurs in all sanitation systems (Chin and Kroontje, 1963; Höglund et al., 2000; Udert et al., 2003). Urea accounts for 75–90% of the urinary nitrogen excretion (Rose et al., 2015). In the presence of the enzyme urease (urea amidohydrolase, EC 3.5.1.5), urea is catalytically decomposed (KM = 0.2–32 mM and t1/2 = 20 µS) to ammonia and carbon dioxide (Dixon et al., 1980; Qin and Cabral, 2009). If the degradation of urea is prevented, then the majority of the urinary nitrogen is non-volatile and can be recovered as solids; e.g., by evaporating urine by alkaline dehydration (Simha et al., 2020b). A simple approach to inhibit urease is to increase the pH of urine, since alkaline conditions (pH > 9.1) affect the functioning of the mobile “flap” that caps the bi-nickel active site of the enzyme (Benini et al., 1999; Krajewska and Ciurli, 2005; Roberts et al., 2012; Mazzei et al., 2020).
In previous studies, both Mg(OH)2 and Ca(OH)2 have been used to alkalise fresh human urine and have been shown to increase the pH to >10 and >12.5, respectively (Randall et al., 2016; Vasiljev et al., 2022). Their use has even been piloted in field-scale implementation of new urine recycling technologies (Flanagan and Randall, 2018; Simha et al., 2020a; Riechmann et al., 2021). However, apart from a simulation of Ca(OH)2 solubility in fresh human urine by Randall et al. (2016), we know quite little about the dissolution behaviour of alkaline Earth hydroxides in biological fluids such as human urine.
In this study, we were interested in bridging this research gap. We performed experiments in parallel with thermodynamic simulations of the chemical speciation to systematically study the dissolution and quantify the solubility of Mg(OH)2 in different types of urine–real fresh urine, synthetic fresh urine, synthetic dephosphatised fresh urine and real fresh urine concentrated by evaporation in a CO2-free drying chamber. We identified factors that affect solubility, determined dissolution kinetics, quantified the concentration of major solids that form in alkalised urine, as well as assessed the accuracy of computer-simulated thermodynamic model. Our results have implications for the design of new source-separating sanitation systems that aim for recycling resources like plant-essential nutrients contained in wastewater, which is key to achieving multiple global Sustainable Development Goals.
Methodology
Materials
Light burned MgO of technical grade (≥97%, VWR Chemicals BDH®, United Kingdom) and high reactivity (citric acid neutralisation time of 45 ± 6 s) was used. Fresh human urine (20 persons, age 25–65) was collected using 500 ml sterile high-density polyethylene bottles, pooled, and used within 7 hours of donation. The samples represented urine collected over a working day (FU1 and FU3), first-morning urine (FU2), and a mixture of first-morning urine and day urine (FU4). Synthetic fresh urine (SU1-SU4) that mimicked the composition of real fresh urine was prepared by dissolving urea and inorganic compounds (NaCl, Na2SO4, KCl, MgCl2.6H2O, NaH2PO4, CaCl2, and NH4Cl) in Milli-Q water and adjusting the pH of the solution with NaOH (Table 1). When preparing synthetic dephosphatised fresh urine (SDU1-SDU4), NaH2PO4 was substituted with NaCl, to have the same sodium concentration as that measured for fresh human urine. To make concentrated fresh human urine, a sample of fresh human urine was dried in a CO2-free drying chamber till the urine weighed 1/8th of its original weight. The concentrated urine (CFU×8) was diluted with Milli-Q water to prepare CFU×2 and CFU×4. To make concentrated synthetic urine (CSU×2, CSU×4 and CSU×8), the same procedure as that for making fresh synthetic urine was followed but the concentration of urea and inorganic compounds in Milli-Q water was increased by two, four or eight times, respectively.
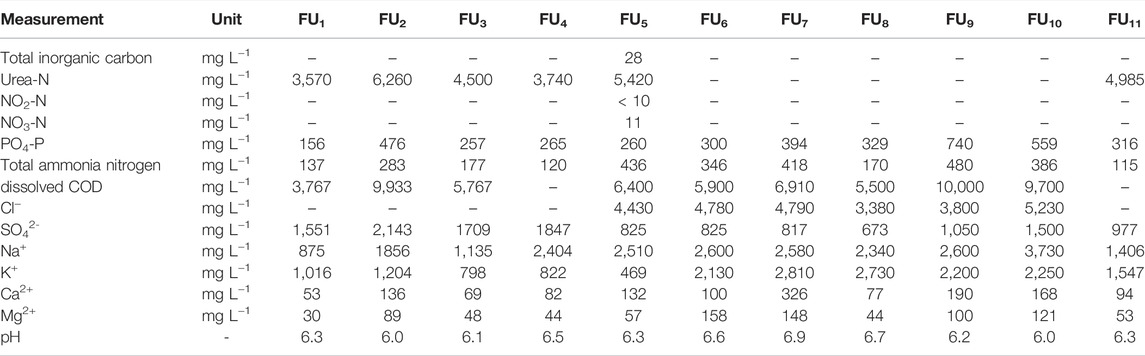
TABLE 1. Composition of fresh urine samples. The compositions FU1, FU2, FU3 and FU4 were measured in this study, while FU5, FU6, FU7 and FU8 were taken from Randall et al. (2016), FU9 from Udert et al. (2006), FU10 from Udert et al. (2003) and FU11 from Vasiljev et al. (2022).
Solubility of Mg(OH)2 in Human Urine
To study the dissolution of Mg(OH)2 in urine, flasks containing 250 ml fresh urine were dosed with 2 g MgO L−1, covered with parafilm, and placed at room temperature (23 ± 2°C) over a magnetic stirrer set to 700 rpm for 60 min. Pre-trials indicated that at these experimental conditions (MgO dosage, mixing speed and duration), less than 15 min was required for electrical conductivity (EC) of urine to increase and equilibrate. The pH, electrical conductivity, and temperature of urine were monitored at 60 s intervals for the first 15 min, and thereafter at every 15 min interval. The pH was measured using a single junction gel electrode (13-620-AE6, Fisher Scientific United States) connected to a benchtop pH meter (AE150 accumet, Fisher Scientific, United States), while the EC and temperature were monitored with a probe (TetraCon 325, WTW, Germany) connected to a handheld meter (Cond 340i, WTW, Germany). After 60 min, the urine was filtered using a 0.45 μm pore size filter paper (Merck KGaA, Germany) placed in a vacuum filtration system (Uni-Crown, Taiwan) that was set to a pressure of 1.5 atm. A representative sample of the filtered urine was acidified to a pH of < 2 by adding 1 M H2SO4 and stored at 4°C for further analysis.
To determine the effect of organic substances and soluble phosphate present in urine on the solubility of Mg(OH)2, the dissolution experiments were repeated using synthetic fresh human urine and synthetic dephosphatised fresh human urine, respectively.
To determine the effect of water removal (i.e., concentrating urine) on the solubility of Mg(OH)2, the dissolution experiments were repeated with concentrated real fresh urine and concentrated synthetic fresh urine. All the experiments were performed in triplicate and average values along with their standard deviation are reported.
Inhibition of Urease
Flasks containing 250 ml fresh human urine or fresh human urine dosed with 2 g MgO L−1 were contaminated with 7.3 mg urease L−1 (lyophilised urease from Canavalia ensiformis with activity of ≥5 U mg−1; 108,489 urease, Merck, Germany), covered with parafilm, and placed over a magnetic stirrer set to 700 rpm at room temperature (23 ± 2°C). The flasks were monitored for 14 days, and daily measurements were made for pH and total ammonia concentration in urine. As control, flasks containing fresh urine and fresh urine saturated with Mg(OH)2, both without any urease contamination were also monitored. The concentration of urease was fixed based on the enzymatic activity reported by the manufacturer (5 µmol ammonia mg−1 min−1), a total hydrolysis time of 14 days, and assuming that fresh urine contained 10 g urea L−1.
Analytical Methods
The concentration of total ammonia nitrogen, total nitrogen, and chemical oxygen demand was determined colorimetrically using Spectroquant® test kits according to the instructions of the manufacturer (Merck KGaA, Germany) and a Spectroquant® photometer (NOVA 60 A, Merck KGaA, Germany). The concentration of phosphorus, potassium, calcium, magnesium, sodium, and sulphur was determined by inductively coupled plasma-optical emission spectrometry (Optima Avio 200, PerkinElmer, United States), prior to which urine samples were digested with 65% HNO3 and diluted with Milli-Q water.
Calculations
The solubility of Mg(OH)2 in urine was calculated using Eq. 1, whereas the MgO dosage required to saturate fresh human urine with Mg(OH)2 was calculated using Eq. 2.
where
The concentration factor, which can be defined either as the ratio of volume of water present in urine initially to the volume of water left after evaporation (Randall and Nathoo, 2015), or the proportion of ion concentration in urine after evaporation versus the ion concentration in fresh urine (Pronk et al., 2006), was calculated using Eq. 3.
The subscripts i and f refer to fresh urine and concentrated urine, respectively, whereas C and A refer to cations and anions.
Modelling of Dissolution Kinetics and Chemical Speciation
To determine the pH of human urine as function of time, the experimental data was fitted to the pseudo second order kinetic model (Eq. 4).
where k2 (min−1) is the rate constant, and pHt and pHeq are the pH of urine at time t and at equilibrium, respectively. To evaluate goodness of fit of the model, the correlation coefficient (R2; Eq. 5) and normalised standard deviation ΔpH (%; Eq. 6) were calculated.
where pHt,cal and pHt,exp are the calculated and experimental pH of urine at time t,
The software OLI Stream Analyzer (OLI Systems Inc., 2020) was used (using the Mixed Solvent Electrolyte model option) to simulate the pH, chemical speciation, and major solids formed in urine at thermodynamic equilibrium. The pH predicted by the software was matched with the experimentally determined pH using Eq. 4, and used to estimate the kinetics of chemical speciation, i.e., time required for Mg(OH)2 to dissolve and for major solids to form in urine. The simulations were made for different compositions of real and synthetic urine used in this study (FU1-FU4), as well as for urine compositions taken from literature (FU5-FU11).
Statistical Analyses
The experiment data was tested for normality and homogeneity of variance, after which one way analysis of variance at 95% confidence interval was performed to assess whether the initial composition of fresh urine and the type of urine (fresh, synthetic or concentrated) had a significant influence on the pH, EC and elemental composition of the saturated urine as well as the solubility of Mg(OH)2. When significant differences were found, a post-hoc test (Tukey’s honest significant difference) was performed at 95% confidence interval. In addition, exploratory principal component analysis was conducted to identify variables that explained the variance in the solubility data, following which linear regression analysis was performed on the variables of interest. All the statistical analyses were carried out in RStudio version 1.2.5042 and R version 4.0.0 (RStudio Team, 2016).
Results
Inhibition of Enzymatic Urea Hydrolysis
The effect of introducing urease to fresh urine, with and without alkalisation by Mg(OH)2 are shown in Figure 1. Without any Mg(OH)2, urea is quickly hydrolysed to ammonia (1860 mg L−1 after 14 days), which increases the pH of urine to 8.6. In fresh urine treated with Mg(OH)2, the concentration of total ammonia nitrogen increased from 100 mg L−1 to 128 mg L−1 after 14 days. This corresponds to a urea hydrolysis rate of just 0.5%. As fresh urine was dosed with excess MgO (2 g L−1), it was saturated with Mg(OH)2 and maintained a pH of >10.5 for 14 days.
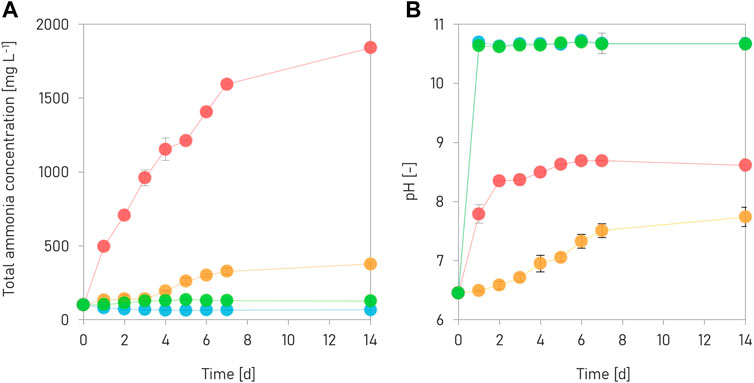
FIGURE 1. The (A) concentration of total ammonia nitrogen (TAN) and the (B) pH is shown over time (days) for fresh human urine, with (red) and without (orange) contamination by jack bean urease and for fresh human urine alkalised using Mg(OH)2, with (green) and without (blue) contamination by jack bean urease. Data points are average values of three replicates and error bars show the standard deviation.
Simulation of Mg(OH)2 Dissolution
When MgO is added to fresh urine, it hydrates to Mg(OH)2. As Mg(OH)2 dissolves in urine, the concentration of Mg2+(aq) and OH−(aq) increases (Eq. 7), resulting in a corresponding increase in the urine pH (Figure 2). Depending on the composition of urine, when the pH is between 6.8 and 7.4, the concentration of Mg2+(aq) and
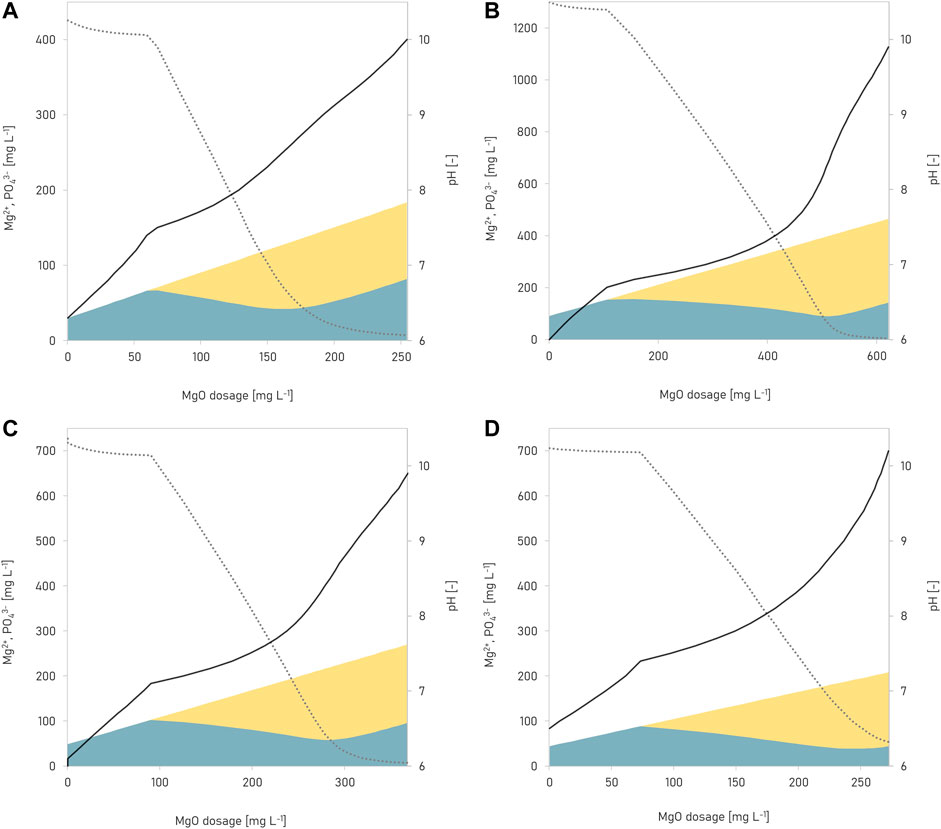
FIGURE 2. The concentration of Mg2+(aq) (blue), Mg2+(s) (yellow) and
At 25°C, between 80 and 700 mg Mg(OH)2 L−1 dissolves, depending on the composition of urine (Figure 3A). The average simulated Mg(OH)2 solubility is 365 mg L−1, making it sparingly soluble in fresh human urine. The dissolution of Mg(OH)2 increases the pH of urine to 10 ± 0.15 at 25°C (Figure 3B). The overall dosage of MgO needed to saturate fresh urine with Mg(OH)2 at 25°C varies between 250 and 970 mg L−1 (Figure 3C).
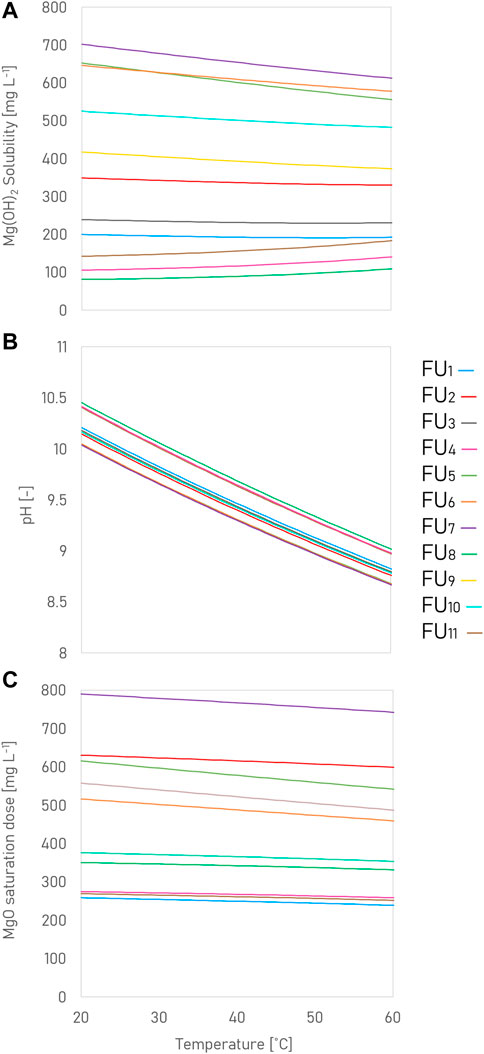
FIGURE 3. The simulated (A) solubility of Mg(OH)2, (B) pH and (C) MgO dosage needed to saturate fresh human urine at different temperatures and for different urine compositions. The compositions are described in detail in Table 1.
The dissolution of Mg(OH)2 is exothermic, so it has retrograde solubility in fresh urine. Increasing the temperature decreases Mg(OH)2 solubility (Figure 3A) as well as the pH of urine (Figure 3B). The solubility of Mg(OH)2 in concentrated fresh urine is higher than its solubility in fresh urine (Figure 4A) and there is a linear relationship between solubility and urine concentration factor (Figure 5). At 25°C and CF = 32, the solubility of Mg(OH)2 in concentrated fresh urine is 16,240 mg L−1, which is 27 times higher than the solubility in fresh urine (Supplementary Figure S1). As a result, at a given temperature, the higher the concentration factor, the lower is the pH of fresh urine when it is saturated with Mg(OH)2 (Figure 4B). Only when >98% of the water is removed from urine, i.e., concentration factors above 60, the minerals arcanite and halite are predicted to precipitate (Figure 5).
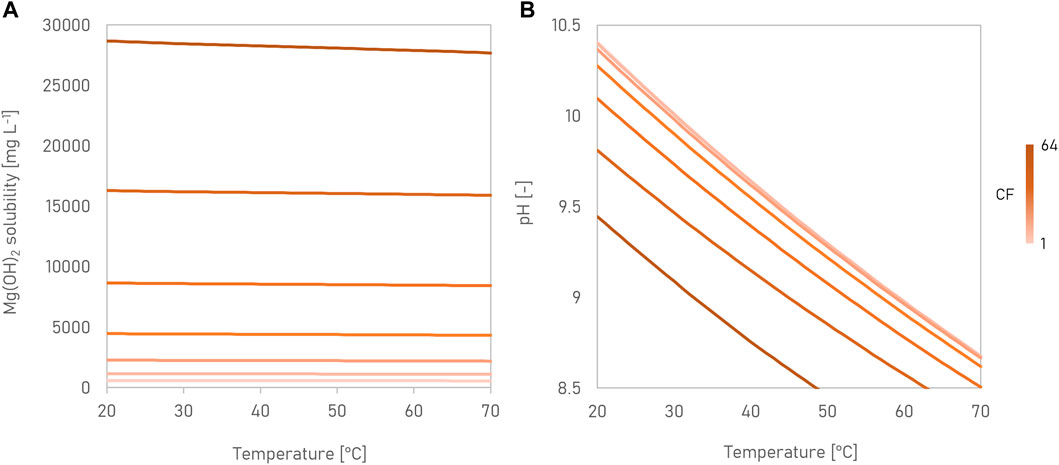
FIGURE 4. The simulated (A) solubility of Mg(OH)2 and (B) pH of fresh human urine at different concentration factors and temperatures.
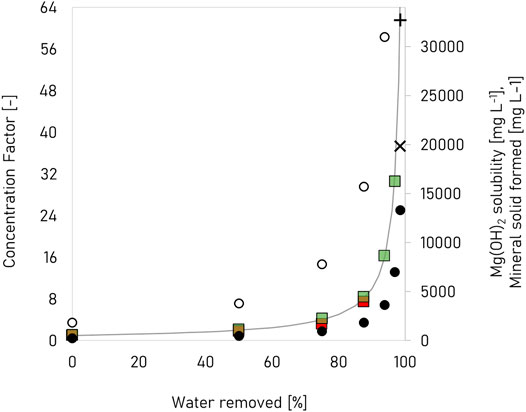
FIGURE 5. The concentration factor (-) obtained as function of water removed (%) from urine. Shown on the secondary y-axis are simulated (green squares) and experimentally determined (red squares) solubility of Mg(OH)2, as well as the simulated amount of struvite (○), hydroxyapatite (∙), halite (+) and arcanite (✕) that form in urine.
Experimentally Determined Mg(OH)2 Solubility
The solubility of Mg(OH)2 in real fresh urine at 23 ± 2°C was measured to be between 495 mg L−1 (FU1) and 940 mg L−1 (FU2), varying with urine composition (Figure 6). According to the mass balance (Supplementary Figure S2), 60% (±2) of the total ammonia initially present in fresh urine, which accounted for 10% (±5%) of the total nitrogen, precipitated when the urine was saturated with Mg(OH)2. In addition, 94% (±3) of the total phosphorus, 69% (±23) of the calcium, and 55% (±9) of the sulphur also precipitated. The solubility of Mg(OH)2 in real fresh urine increased when the urine was concentrated by evaporation (Figure 5). At CF = 8, the solubility was 3,980 mg L−1 or about seven times higher than the solubility in unconcentrated fresh urine, whereas the pH of urine decreased from 10.6 to 9.8. The relative increase in Mg(OH)2 solubility for concentrated fresh synthetic urine was lower than the relative increase in solubility for real urine. The pH of synthetic fresh urine saturated with Mg(OH)2 was >11, but the pH dropped to 10.3 (±0.1) at CF=16 (Supplementary Figure S3).
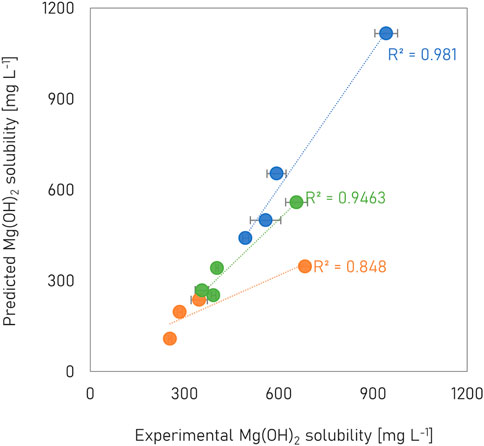
FIGURE 6. The experimentally determined solubility and the simulated solubility of Mg(OH)2 in real fresh urine (blue), synthetic fresh urine (orange) and dephosphatised synthetic fresh urine (green). Data points for experimental solubility are average values of three replicates, with error bars showing the standard deviation. Dashed lines represent linear fit to the experimental data.
The deviation between the Mg(OH)2 solubility determined in experiments and the thermodynamic simulations were less than 5% (Figure 6), suggesting that the thermodynamic model accurately predicted the chemical speciation in urine. In fact, the experimentally measured EC in urine and the simulated Mg(OH)2 solubility curve had the same trend–increasing at first, then decreasing, and finally equilibrating. In comparison to real fresh urine, the solubility of Mg(OH)2 at 23 ± 2 °C was less in synthetic (393 ± 180 mg L−1) and dephosphatised synthetic urine (452 ± 126 mg L−1), and the deviation between the simulated and experimental values was much larger (>28%).
Simulation of Mg(OH)2 Dissolution Kinetics
By matching the experimentally determined pH with the thermodynamically simulated pH of urine, we could also simulate the kinetics of Mg(OH)2 dissolution. We found that it took between 6 and 16 min for brucite (Mg(OH)2) to dissolve (Figure 7), which compares well with the time required for the pH to increase to >10 (Figure 8) and EC to equilibrate (Supplementary Figure S4). In addition to brucite, struvite and hydroxyapatite were the only two minerals predicted to form. All the urine solutions were supersaturated with respect to struvite in 10 min, while hydroxyapatite supersaturation required less than 2 min. It is likely that both the minerals precipitated in 15 min as the change in EC of urine is less than 5% after this (Supplementary Figure S4).
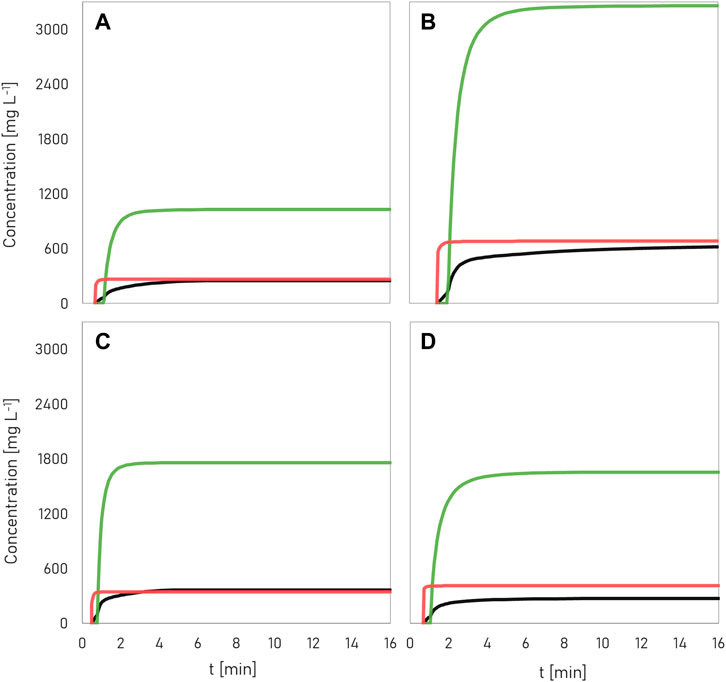
FIGURE 7. Kinetics of dissolution of Mg(OH)2 (black line) and formation of hydroxyapatite (red line) and struvite (green line) in fresh urine dosed with 2 g MgO L−1 at 25°C. Four different urine compositions were used: FU1 (A), FU2 (B), FU3 (C) and FU4 (D).
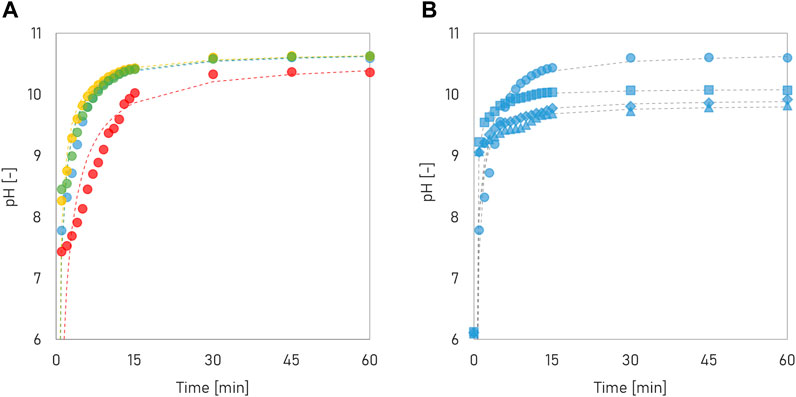
FIGURE 8. Mg(OH)2 dissolution kinetics in (A) fresh urine and (B) concentrated fresh urine. Data points show experimentally determined average pH values and dashed lines represent first order kinetic fit by linear regression and plotted using rate constants shown in Table 2. For fresh urine, four urine compositions were used: FU1 (blue), FU2 (red), FU3 (yellow) and FU4 (green). For concentrated fresh urine, FU1 was dehydrated to make CFU×2 (▪), CFU×4 (◆) and CFU×8 (▲).
Experimentally Determined Mg(OH)2 Dissolution Kinetics
In experiments, after 60 min of mixing at 700 rpm, the pH of real fresh urine treated with Mg(OHJ2 reached >10.5 (Figure 8A) and remained stable during 14 days of storage in a closed flask (Figure 1). To reach pH 10, the average time required was 8.9 ± 5.7 min. Increasing the urine concentration factor reduced the pH but increased the time required to achieve saturation (Table 2). The Mg(OH)2 dissolution kinetics in both fresh urine and concentrated fresh urine was well described (R2 = >0.99 and ΔpH = <5%) by the pseudo-second order rate equation (Figures 8A,B) (Table 2). Similar trends were seen when the experiments were repeated with synthetic fresh urine and synthetic concentrated urine, although the pH of urine was higher, and the time required to reach equilibrium was less in comparison to real fresh urine.
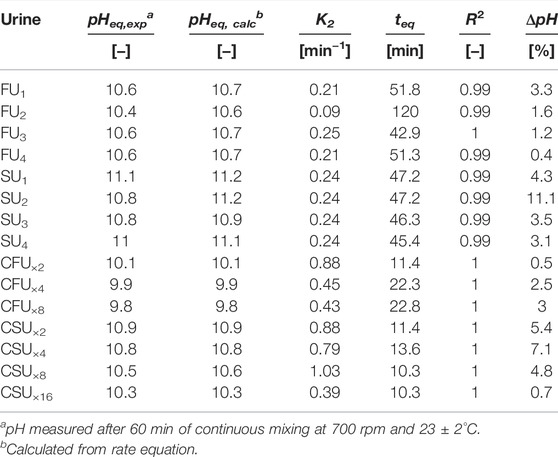
TABLE 2. Dissolution kinetics of Mg(OH)2 in real fresh urine (FU), synthetic fresh urine (SU), concentrated fresh urine (CFU) and concentrated fresh synthetic urine (CSU).
Discussion
Factors Affecting the Solubility of Mg(OH)2 in Urine
The results of our study demonstrated that Mg(OH)2, an alkaline Earth metal compound, is sparingly soluble in freshly excreted human urine. Despite a low solubility of < 1 g Mg(OH)2 L−1 at 25°C, fresh urine saturated with Mg(OH)2 has pH > 10.5, which we also demonstrated as being sufficient alkalinity to inhibit the enzymatic degradation of urea (Figure 1). Yet, many factors determine the solubility of this compound in human urine. First, fresh urine with different compositions had different solubilities. According to descriptive principal component analysis, it is the concentration of magnesium (R2=0.95; p < 0.0001) and phosphate (R2=0.93; p < 0.0001) and to a lesser extent calcium (R2=0.89; p < 0.001) and total ammonia (R2=0.88; p < 0.001) that is initially present in urine that explain this variability (>94% of total variance) and has the largest influence on Mg(OH)2 solubility (Supplementary Figure S5). This is apparent because Mg(OH)2 solubility is a direct reflection of the concentration of Mg2+(aq) (Eq. 1), which is influenced by the concentration of Mg2+ removed from urine as a precipitate (Figure 2).
Secondly, the solubility is retrograde with respect to the temperature of urine, increasing significantly at lower temperatures (R2=0.97; p < 0.001). The hydration of MgO(s) is exothermic, with reaction enthalpy of −81 kJ mol−1 (Kato et al., 1996). The dissolution of Mg(OH)2 is also exothermic, with solution enthalpy of −152 kJ mol−1 (Tahiri et al., 2003). The overall enthalpy change is about eight times more if urine is dosed with CaO (Long et al., 2017) as the solubility of Ca(OH)2 in fresh urine is 5 g L−1 at 25°C (Randall et al., 2016). Due to the low solubility of Mg(OH)2, the temperature of urine dosed with 2 g MgO L−1 and kept in an uninsulated flask increases by just 2°C over 60 min (Supplementary Figure S6). The rate of increase in the urine temperature depends on the hydration kinetics of MgO and the dissolution kinetics of Mg(OH)2. According to Kato et al. (1996), the hydration of MgO involves rapid physical adsorption of water to produce an intermediate (MgOH2O), followed by chemical hydration which is the rate-controlling process. A fraction of MgO remains inert to hydration because of sintering during calcination (Strydom et al., 2005).
Thirdly, we found a large effect on solubility from organic substances present in urine. On average, the solubility of Mg(OH)2 was 40% lower in synthetic fresh urine than in real fresh urine. The synthetic urine that we prepared contained only urea, which has no COD (Li et al., 2012), whereas in real human urine, the concentration of organic substances is about 10 g COD L−1 (Udert et al., 2006) and there can be hundreds of metabolic breakdown products (Bouatra et al., 2013). Organic compounds in urine also have pH-dependent solubility. Increasing the pH of urine from < 7 to >10 increases the solubility of creatinine but decreases the solubility of uric acid. We also know that some organic substances in urine co-precipitate by adhesion to mineral colloids and that the sediment at the bottom of tanks storing hydrolysed urine contain 0.39–0.65 g VS. g TS−1 (Höglund et al., 2000). On the other hand, Curtin et al. (2016) showed that solubility and degradation of organic matter increases when soil is treated with Ca(OH)2. It seems that the presence and/or degradation of organic substances and the change in their solubility also affects the solubility of Mg(OH)2 in urine.
Lastly, we found that the more concentrated the urine, the higher is the solubility of Mg(OH)2. However, the relative increase in the Mg(OH)2 solubility is lower at a higher concentration factor, as is the pH of the urine saturated with Mg(OH)2. Increasing the temperature reduces the pH of urine further. For instance, at 50°C and concentration factor of 16, the pH of urine saturated with Mg(OH)2 drops to < 9. To increase the urine pH to ≥10 and prevent ureolysis, the temperature must be < 30°C at CF=1 and < 22°C at CF=16, respectively (Supplementary Figure S7). In contrast, the solubility of Ca(OH)2 and the pH of urine increase with concentration factor since soluble sulphate in urine precipitates as gypsum at CF < 60 and anhydrite at CF > 60. In contrast, no ammonia nitrogen can be recovered when fresh urine is alkalised using Ca(OH)2 (Riechmann et al., 2021), since all of the phosphate in urine precipitates as hydroxyapatite (Ca10(PO4)6(OH)2) (Randall et al., 2016). At CF > 100 or when 99% water is removed from urine dosed with Mg(OH)2, the fraction of soluble phosphate in urine that is precipitated as struvite increases and hydroxyapatite decreases (Supplementary Figure S8). This suggests that there is higher potential to recover ammonia nitrogen excreted in fresh urine as struvite at high concentration factors. Depending on the composition of urine, between 22 and 100% of the ammonia nitrogen can be recovered as struvite when fresh urine is alkalised using Mg(OH)2 (Figure 9).
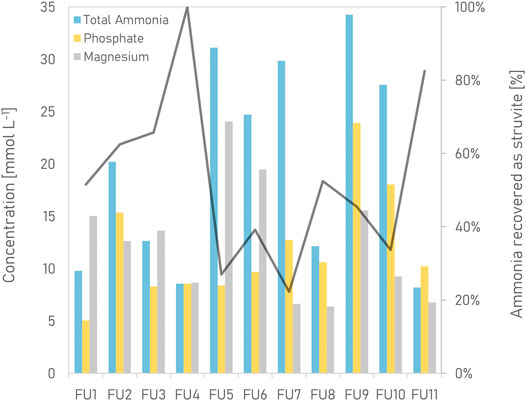
FIGURE 9. Concentration of total ammonia and soluble phosphate in freshly excreted urine and concentration of total magnesium in fresh urine saturated with Mg(OH)2 at 25°C is shown for different urine compositions. The fraction of ammonia nitrogen that can be recovered from saturated urine as struvite is shown on the secondary y-axis. See Table 1 for the urine compositions.
Conclusion
In this study, we developed experimental and simulated data on the dissolution of Mg(OH)2 in human urine. Our results showed that Mg(OH)2 is particularly well suited to prevent the urease-catalysed degradation of urea and inhibit ureolysis in the long-term (>14 days). A dosage of < 1 g MgO L−1 is needed to increase the pH of fresh urine to >10 and saturate it with Mg(OH)2. We identified and quantified the influence of a range of factors on the solubility of Mg(OH)2 in urine. We found that the composition (e.g., initial total ammonia and phosphate concentration) and type of urine (fresh or concentrated), and the choice of the dissolution conditions (temperature, pH, time) have significant effect on solubility, dissolution kinetics, and MgO dosage required to saturate urine with Mg(OH)2. These results have implications for the use of alkaline Earth monoxides and hydroxides in sanitation systems based on source-separation that aim to recycle plant-essential nutrients present in wastewater.
Data Availability Statement
The original contributions presented in the study are included in the article/Supplementary Material, further inquiries can be directed to the corresponding author.
Author Contributions
All authors contributed to the study conceptualization and design, manuscript revision, read and approved the submitted version. PS and CD performed the experiments, physicochemical analyses, and formal data analysis. DR modeled the chemical speciation of urine using OLI. BV supervised the study, provided resources and acquired funding. PS wrote the first draft of the manuscript. All authors contributed to manuscript revision, read and approved the submitted version.
Funding
The study was funded by a grant from the Swedish Research Council for the project “Urintorkning processoptimering och underliggande processer” (Grant number 2019–00986). DR was supported through the FLAIR Fellowship by the Royal Society and the African Academy of Sciences.
Conflict of Interest
The authors declare that the research was conducted in the absence of any commercial or financial relationships that could be construed as a potential conflict of interest.
Publisher’s Note
All claims expressed in this article are solely those of the authors and do not necessarily represent those of their affiliated organizations, or those of the publisher, the editors, and the reviewers. Any product that may be evaluated in this article, or claim that may be made by its manufacturer, is not guaranteed or endorsed by the publisher.
Supplementary Material
The Supplementary Material for this article can be found online at: https://www.frontiersin.org/articles/10.3389/fenvs.2022.889119/full#supplementary-material
References
Ahmad, S. Z. N., Hamdan, R., Al‐Gheethi, A., Alkhadher, S., and Othman, N. (2020). Removal of Phosphate from Wastewater by Steel Slag with High Calcium Oxide Column Filter System; Efficiencies and Mechanisms Study. J. Chem. Technol. Biotechnol. 95 (12), 3232–3240. doi:10.1002/jctb.6501
Benini, S., Rypniewski, W. R., Wilson, K. S., Miletti, S., Ciurli, S., and Mangani, S. (1999). A New Proposal for Urease Mechanism Based on the crystal Structures of the Native and Inhibited Enzyme from Bacillus Pasteurii: Why Urea Hydrolysis Costs Two Nickels. Structure 7 (2), 205–216. doi:10.1016/s0969-2126(99)80026-4
Bouatra, S. F., Aziat, R., Mandal, A. C., Guo, M. R., Wilson, C., and Knox, T. C. (2013). The Human Urine Metabolome. PLoS One 8(9), e73076.
Bray, E. L., and Ghalayin, Z. T. (2020). “Magnesium Compounds,” in 2017 Minerals Yearbook (Reston, Virginia: U.S. Department of the Interior & U.S. Geological Survey).
Chin, W.-t., and Kroontje, W. (1963). Urea Hydrolysis and Subsequent Loss of Ammonia1. Soil Sci. Soc. America J. 27 (3), 316–318. doi:10.2136/sssaj1963.03615995002700030030x
Curtin, D., Peterson, M. E., and Anderson, C. R. (2016). pH-Dependence of Organic Matter Solubility: Base Type Effects on Dissolved Organic C, N, P, and S in Soils with Contrasting Mineralogy. Geoderma 271, 161–172. doi:10.1016/j.geoderma.2016.02.009
Dhakal, R. P., Ghimire, K. N., and Inoue, K. (2005). Adsorptive Separation of Heavy Metals from an Aquatic Environment Using orange Waste. Hydrometallurgy 79 (3-4), 182–190. doi:10.1016/j.hydromet.2005.06.007
Dixon, N. E., Riddles, P. W., Gazzola, C., Blakeley, R. L., and Zerner, B. (1980). Jack Bean Urease (EC 3.5.1.5). V. On the Mechanism of Action of Urease on Urea, Formamide, Acetamide, N-Methylurea, and Related Compounds. Can. J. Biochem. 58 (12), 1335–1344. doi:10.1139/o80-181
Flanagan, C. P., and Randall, D. G. (2018). Development of a Novel Nutrient Recovery Urinal for On-Site Fertilizer Production. J. Environ. Chem. Eng. 6 (5), 6344–6350. doi:10.1016/j.jece.2018.09.060
Höglund, C., Vinnerås, B., Stenström, T. A., and Jönsson, H. (2000). Variation of Chemical and Microbial Parameters in Collection and Storage Tanks for Source Separated Human Urine. J. Environ. Sci. Health A 35 (8), 1463–1475. doi:10.1080/10934520009377047
Jefferson, B., Hurst, A., Stuetz, R., and Parsons, S. A. (2002). A Comparison of Chemical Methods for the Control of Odours in Wastewater. Process Saf. Environ. Prot. 80 (2), 93–99. doi:10.1205/095758202753553211
Kato, Y., Yamashita, N., Kobayashi, K., and Yoshizawa, Y. (1996). Kinetic Study of the Hydration of Magnesium Oxide for a Chemical Heat Pump. Appl. Therm. Eng. 16 (11), 853–862. doi:10.1016/1359-4311(96)00009-9
Krajewska, B., and Ciurli, S. (2005). Jack Bean (Canavalia Ensiformis) Urease. Probing Acid-Base Groups of the Active Site by pH Variation. Plant Physiol. Biochem. 43 (7), 651–658. doi:10.1016/j.plaphy.2005.05.009
Larsen, T. A., Udert, K. M., and Lienert, J. (2013). Source Separation and Decentralization for Wastewater Management. London, U.K.: IWA Publishing.
Li, H., Liu, S., Zhao, J., and Feng, N. (2016). Removal of Reactive Dyes from Wastewater Assisted with Kaolin clay by Magnesium Hydroxide Coagulation Process. Colloids Surf. A: Physicochemical Eng. Aspects 494, 222–227. doi:10.1016/j.colsurfa.2016.01.048
Li, L., Zhang, S., Li, G., and Zhao, H. (2012). Determination of Chemical Oxygen Demand of Nitrogenous Organic Compounds in Wastewater Using Synergetic Photoelectrocatalytic Oxidation Effect at TiO2 Nanostructured Electrode. Analytica Chim. Acta 754, 47–53. doi:10.1016/j.aca.2012.10.008
Lin, J., and Wang, L. (2009). Comparison between Linear and Non-linear Forms of Pseudo-first-order and Pseudo-second-order Adsorption Kinetic Models for the Removal of Methylene Blue by Activated Carbon. Front. Environ. Sci. Eng. China 3 (3), 320–324. doi:10.1007/s11783-009-0030-7
Long, X. F., Dai, L., Lou, B., and Wu, J. (2017). The Kinetics Research of Thermochemical Energy Storage System Ca(OH)2/CaO. Int. J. Energ. Res. 41 (7), 1004–1013. doi:10.1002/er.3688
Mazzei, L., Musiani, F., and Ciurli, S. (2020). The Structure-Based Reaction Mechanism of Urease, a Nickel Dependent Enzyme: Tale of a Long Debate. J. Biol. Inorg. Chem. 25 (6), 829–845. doi:10.1007/s00775-020-01808-w
Nie, M., Deng, Y., Nie, S., Yan, C., Ding, M., Dong, W., et al. (2019). Simultaneous Removal of Bisphenol A and Phosphate from Water by Peroxymonosulfate Combined with Calcium Hydroxide. Chem. Eng. J. 369, 35–45. doi:10.1016/j.cej.2019.03.046
Pronk, W., Biebow, M., and Boller, M. (2006). Electrodialysis for Recovering Salts from a Urine Solution Containing Micropollutants. Environ. Sci. Technol. 40 (7), 2414–2420. doi:10.1021/es051921i
Qin, Y., and Cabral, J. M. S. (2009). Review Properties and Applications of Urease. Biocatal. Biotransformation 20 (1), 1–14. doi:10.1080/10242420210154
Randall, D. G., Krahenbuhl, M., Kopping, I., Larsen, T. A., and Udert, K. M. (2016). A Novel Approach for Stabilizing Fresh Urine by Calcium Hydroxide Addition. Water Res. 95, 361–369. doi:10.1016/j.watres.2016.03.007
Randall, D. G., and Nathoo, J. (2015). A Succinct Review of the Treatment of Reverse Osmosis Brines Using Freeze Crystallization. J. Water Process Eng. 8, 186–194. doi:10.1016/j.jwpe.2015.10.005
Riechmann, M. E., Ndwandwe, B., Greenwood, E. E., Reynaert, E., Morgenroth, E., and Udert, K. M. (2021). On-site Urine Treatment Combining Ca(OH)2 Dissolution and Dehydration with Ambient Air. Water Res. X 13, 100124. doi:10.1016/j.wroa.2021.100124
Roberts, B. P., Miller, B. R., Roitberg, A. E., and Merz, K. M. (2012). Wide-open Flaps Are Key to Urease Activity. J. Am. Chem. Soc. 134 (24), 9934–9937. doi:10.1021/ja3043239
Rose, C., Parker, A., Jefferson, B., and Cartmell, E. (2015). The Characterization of Feces and Urine: A Review of the Literature to Inform Advanced Treatment Technology. Crit. Rev. Environ. Sci. Technol. 45 (17), 1827–1879. doi:10.1080/10643389.2014.1000761
Simha, P., Karlsson, C., Viskari, E.-L., Malila, R., and Vinnerås, B. (2020a). Field Testing a Pilot-Scale System for Alkaline Dehydration of Source-Separated Human Urine: A Case Study in Finland. Front. Environ. Sci. 8, 570637. doi:10.3389/fenvs.2020.570637
Simha, P., Lalander, C., Nordin, A., and Vinnerås, B. (2020b). Alkaline Dehydration of Source-Separated Fresh Human Urine: Preliminary Insights into Using Different Dehydration Temperature and media. Sci. Total Environ. 733, 139313. doi:10.1016/j.scitotenv.2020.139313
Strydom, C. A., Van der Merwe, E. M., and Aphane, M. E. (2005). The Effect of Calcining Conditions on the Rehydration of Dead Burnt Magnesium Oxide Using Magnesium Acetate as a Hydrating Agent. J. Therm. Anal. Calorim. 80 (3), 659–662. doi:10.1007/s10973-005-0710-x
Tahiri, I. A., Matiullah, M., and Subhani, M. S. (2003). Molten Ba(OH)2·8H2O as an Etchant for CR-39 Detector. Radiat. Measurements 37 (3), 205–210. doi:10.1016/s1350-4487(03)00030-1
Udert, K. M., Larsen, T. A., Biebow, M., and Gujer, W. (2003). Urea Hydrolysis and Precipitation Dynamics in a Urine-Collecting System. Water Res. 37 (11), 2571–2582. doi:10.1016/s0043-1354(03)00065-4
Udert, K. M., Larsen, T. A., and Gujer, W. (2006). Fate of Major Compounds in Source-Separated Urine. Water Sci. Technol. 54 (11-12), 413–420. doi:10.2166/wst.2006.921
Vasiljev, A., Simha, P., Demisse, N., Karlsson, C., Randall, D. G., and Vinnerås, B. (2022). Drying Fresh Human Urine in Magnesium-Doped Alkaline Substrates: Capture of Free Ammonia, Inhibition of Enzymatic Urea Hydrolysis & Minimisation of Chemical Urea Hydrolysis. Chem. Eng. J. 428, 131026. doi:10.1016/j.cej.2021.131026
Keywords: free ammonia, nutrient recycling, solubility, source separating sanitation, urease, wastewater
Citation: Simha P, Deb CK, Randall DG and Vinnerås B (2022) Thermodynamics and Kinetics of pH-dependent Dissolution of Sparingly Soluble Alkaline Earth Hydroxides in Source-Separated Human Urine Collected in Decentralised Sanitation Systems. Front. Environ. Sci. 10:889119. doi: 10.3389/fenvs.2022.889119
Received: 03 March 2022; Accepted: 17 March 2022;
Published: 12 April 2022.
Edited by:
Amin Mojiri, Hiroshima University, JapanReviewed by:
Salem S. Abu Amr, Karabük University, TurkeyMohammed Bashir, Tunku Abdul Rahman University, Malaysia
Copyright © 2022 Simha, Deb, Randall and Vinnerås. This is an open-access article distributed under the terms of the Creative Commons Attribution License (CC BY). The use, distribution or reproduction in other forums is permitted, provided the original author(s) and the copyright owner(s) are credited and that the original publication in this journal is cited, in accordance with accepted academic practice. No use, distribution or reproduction is permitted which does not comply with these terms.
*Correspondence: Prithvi Simha, UHJpdGh2aS5TaW1oYUBzbHUuc2U=, cHJpdGh2aS5zaW1oYUBtZXNwb20uZXU=