- Department of Biological and Environmental Science, University of Jyväskylä, Jyväskylä, Finland
The ubiquitous presence of perfluorinated carboxylic acids (PFCAs) around the globe has attracted increasing attention, due to their persistency, bioaccumulation, and toxicity. Nevertheless, the ecotoxicological effects of the compounds on aquatic microorganisms has remained understudied. Hence, the present study focused on determining, and comparing, the effects of regulated long-chain PFCA, perfluorooctanoic acid (PFOA), and nonregulated short-chain PFCA, perfluorohexanoic acid (PFHxA), on the diversity, structure, microbial growth, and activity of a freshwater microbial community. In the experiment, lake water was incubated for a period of four weeks at three different concentrations of the studied PFCAs: 100 ng/L, 100 μg/L, and 10 mg/L. The results suggested that both compounds at high concentration (10 mg/L) altered the structure of the microbial community, but the diversity was not affected. Both compounds also decreased the microbial biovolume at higher concentrations and the increasing dose added to the significance of the impact, whereas inhibition of net microbial respiration could not be demonstrated. PFOA showed more potent toxicity towards the microbial community as it caused more significant structural changes to the community and significantly inhibited microbial growth even at the low 100 ng/L concentration. This study helps to better understand the ecotoxicity of PFCAs and to assess the environmental risks associated with their use. Additionally, these results can help policy makers to better assess the environmental risks posed by short-chain PFCAs on aquatic ecosystems.
1 Introduction
Microorganisms play a crucial part in the biogeochemical processes that sustain freshwater ecosystems (Cotner and Biddanda, 2002; Bissett et al., 2007; Briee et al., 2007), and a diverse microbial community is essential for maintaining the health of a well-functioning freshwater ecosystem (Echavarri-Bravo et al., 2015; Ren et al., 2019). However, anthropogenic emissions from industrial activities or modern lifestyles can adversely affect the ecological status of freshwater, directly by disturbing the microbial activities which are taking care of these vital biogeochemical processes (Gerbersdorf et al., 2011; Londono et al., 2017), or indirectly by causing changes to the composition of the microbial community (Zhang et al., 2019; Paumelle et al., 2021). Thus, how continuous exposure to anthropogenic pollutants affects the diversity, structure, and specific functions of the microbial communities is urgently needed.
One such anthropogenic pollutant is perfluorooctanoic acid (PFOA; C8), a member of persistent organic pollutants (POPs) called per- and polyfluoroalkyl substances (PFASs). PFASs possess unique chemical properties that have led to a wide range of applications for them in industrial and domestic products, e.g., lubricants and dirt-repellent coatings (Buck et al., 2011; Glüge et al., 2020). As a downside, the widespread use and chemical persistence has led to the ubiquitous presence of PFASs in the environment, wildlife, and humans (Abunada et al., 2020). In addition to their persistence (Wang et al., 2014), many of the PFASs are toxic (Henning and Fuchsman, 2021) bioaccumulative (Haukås et al., 2007), water-soluble (3M, 2001; Zhao et al., 2016), and readily transportable in the environment (Reinikainen et al., 2019). Since the toxicity of PFASs is highly related to the length of the carbon backbone (Latala et al., 2009), efforts towards using less-toxic short-chained PFASs have been made by regulations (USEPA, 2016). As a result, long-chained PFASs, such as PFOA, have been replaced with short-chain PFASs, such as perfluorohexanoic acid (PFHxA; C6) (Ritter, 2010; Buck et al., 2011).
PFOA and PFHxA are both members of a sub-group of PFASs called perfluoro carboxylic acids (PFCAs). PFCAs differ greatly from other well-studied organic pollutants (e.g., polychlorinated biphenyl) as they tend to have low octanol/water partition coefficients (logKow) resulting in lower affinity to sediments and soils and higher water solubility (Davis et al., 2007). Consequently, both PFOA and PFHxA are frequently detected from bodies of waters around the globe (Sun et al., 2018; Muir and Miaz, 2021; Sims et al., 2022), where they end up directly from point-sources or indirectly as a wet or dry fall (Knepper et al., 2012). So far, PFOA is generally detected more often, and in higher concentrations, from the surface waters compared to PFHxA (Eschauzier et al., 2012). However, this is likely to change in the future and sites where short-chain PFCAs dominate have already been reported (Zhao et al., 2016). Moreover, the ability of PFHxA to percolate through soils and to reach groundwaters is higher compared PFOA, as the logKow value of the PFHxA is lower than of the PFOA (Jing et al., 2009). Therefore, PFHxA is more mobile in the soils and has more potential to contaminate groundwater aquifers. As the documentation regarding the toxic effects of the short-chain PFCAs is increasing, it seems evident that there is no full certainty as to whether short-chain PFCAs can be considered as a safe long-term alternative for the long-chain PFCAs in terms of environment and human health. In fact, the 2018 ANNEX XV report of the European Chemical Agency (ECHA, 2018) suggested that PFHxA should be considered as a substance of very high concern (SVHC) and added to the list of Article 57 of Regulation No 1907/2006, stating that the level of concern for PFHxA should be equal with PFOA.
Previous toxicological studies concerning PFCAs, have mainly focused on humans (Alexander, 2001; Lau et al., 2007; Rand and Mabury, 2017), rodents (Kato et al., 2014), and aquatic organisms such as microalgae (Rodea-Palomares et al., 2012), invertebrates (Barmentlo et al., 2015), and rotifers (Wang et al., 2014). The few studies focusing on microorganisms have mainly investigated the effects on soil microbes. Thus, very little is known about the overall impacts of these compounds on aquatic microorganisms. A recent study by Zhang et al. (2019) provided the much-needed evidence that PFOA at high concentrations could affect the composition of microbial communities in freshwater ecosystems. However, similar studies on the effects of PFHxA, or any other short-chain PFCA, has not been conducted to date. Therefore, our study aims to determine and compare the ecotoxicological effects of PFOA and PFHxA on a freshwater microbial community during a four-week incubation experiment. The effects on the microbial community will be studied by measuring the following features: 1) structure and diversity via next-generation sequencing (NGS), 2) microbial growth by conducting a flow cytometric (FCM) bacterium count measurement, and 3) microbial activity by measuring cell respiration by taking weekly CO2 measurements during the incubation. For the first time, the effects of a long-chain PFCA on aquatic microbial community will be directly compared to the effects caused by a short-chain PFCA. The hypotheses are that both compounds significantly affect the diversity and structure of the microbial community at high concentrations and inhibit, both, the cellular respiration and microbial growth of the community. Also, it can be assumed that the obtained effects of the PFHxA are milder compared to effects of the PFOA.
2 Materials and Methods
2.1 Research Site and Sample Water
The studied water was collected as a compiled sample from the pelagic zone of Enonselkä Basin of Lake Vesijärvi, Central Finland (61°02′42″N, 25°35′10″E), during the summer season (August 2020) and stored at +6°C for 35 days prior to further analysis. Lake Vesijärvi is a well-studied medium-sized (110 km2) urban eutrophic, clear water lake with a total phosphorus content of 27 μg/L and watercolor of 10 mg Pt/L (Taipale et al., 2020). In turn, Enonselkä is the southernmost basin of the lake and has a mean depth of ca 6.8 m and a surface area of ca 26 km2.
The dissolved organic carbon (DOC) content, pH, and calculated specific UV absorbance at 254 nm (SUVA254) for the sample water were 5.1 mg/L, 7.9, and 1.9 (±0.3) L mg C−1m−1, respectively. For the analysis of DOC concentration, 15 ml of the sample water was pre-filtered through a 0.45 µm hydrophilic syringe filter (Biotech), and 80 µL of HCl (2 M) was added. The sample was then analyzed with TOC-L-CSH Total Organic Carbon Analyzer (Shimadzu, Kyoto, Japan). The equipment was calibrated by using standards with carbon concentrations of 0.3 and 30 mg/L. In turn, the SUVA254 parameter was used to describe the aromaticity of the lake water, and it was measured by using UV-1800 spectrophotometer (Shimadzu, Kyoto, Japan). As described by Taipale et al. (2019), the SUVA254 expressed as L mg C−1 m−1 is defined as the UV absorbance at 254 nm measured in inverse meters (m−1) divided by the DOC concentration (mg/L) and multiplied then with 100.
2.2 Sample Preparations and Experimental Set-Up
To start the experiments, the sample water was pre-filtered with 100 µm mesh into two 3 L Erlenmeyer flasks, and the water was stored in the absence of light at room temperature (20 ± 1°C) without shaking for seven days. Both PFOA (95%) and PFHxA (≥97%) were purchased from Sigma Aldrich. In order to study the impact of PFOA and PFHxA enrichment on the bacterial community in Lake Vesijärvi, the sample water was divided into 250 mL Wheaton glass bottles, and both were spiked with either PFOA or PFHxA with final concentrations of 100 ng/L, 100 μg/L, and 10 mg/L (treatments Hx1-3 and O1-3). This wide range of concentrations was chosen due to a wide range of concentrations reported in the literature. Both PFOA and PFHxA are ubiquitously present in water bodies at the low ng/L range (Eschauzier et al., 2012), and higher concentrations at µg/L and mg/L range have been reported on contaminated sites (Moody et al., 2003; Seyoum et al., 2020). Four replicates (A-D) for each treatment were made. Also, four replicates with no added PFOA or PFHxA were prepared to act as controls (cntrlA-D). All 28 samples were incubated for a period of 28 days at room temperature in the absence of light without shaking. The extended incubation period compared to the usual 21 days, was chosen to assure that any possible structural changes in the microbial community caused even by the lowest concentration would be detected. All the samples with their specific sample IDs are shown in Supplementary Table S1.
2.3 16S rRNA Gene Sequencing
A total of 31 samples were prepared for the sequencing using sample IDs given in Supplementary Table S1, three of the samples were taken from the unincubated initial water (startA-C) and 28 sub-samples from the incubated samples (control and PFCA treatments). Samples were prepared by filtering the water through a Supor® membrane syringe filter (0.2 µm/25 mm, Pall Corporation). The filtered sample volume for samples startA-C was 27 mL, for sample cntrlB 20 mL, and for the remaining samples 25 mL.
2.3.1 DNA Extraction, Amplification, and Library Preparation
The extraction of microbial DNA was performed through bead mill homogenization. For this, the filters were placed into pre-filled bead beating tubes with 750 µL of DNA/RNA Shield™ (Zymo Research) and homogenized with Bead Ruptor Elite 24 bead mill homogenizer (Omni International Inc., GA, United States) (v = 5.5 m/s, t = 40 s). Homogenized samples were stored at −80°C to wait for further analysis. Later, the samples were centrifuged at 4°C (5 min; 12,000 rpm) and 300 µL of the supernatant per sample was pipetted to a 96-well plate and the magnetic bead-based DNA/RNA isolation was performed with the chemagicTM 360 Instrument (PerkinElmer Inc., United States) following the instructions of chemagic Viral DNA/RNA Kit. The isolated DNA was stored at −20°C.
The first-round PCR was made by using gene-specific forward primer 515FB (GTGYCAGCMGCCGCGGTAA) and reverse primer 806R (GGACTACHVGGGTWTCTAAT), enabling the partial amplification of the target 16S ribosomal RNA (rRNA) gene. PCR-reactions were prepared on qPCR strips by mixing 2 µL of the template DNA with 23 µL of master mix consisting of 12.5 µL of SybrGreen/Fluorescein qPCR Maxima Master Mix (2x) (Thermo Scientific, Lithuania), 0.5 µL of each primer (10 µM), and 9.5 µL of sterile water, which was also used as a negative control (NTC). Reaction products were vortexed, spun down, and amplified with a qPCR instrument. Second-round PCR was made to add Ion Torrent sequencing adapters to the amplicon and followed the same steps as first-round PCR. 1 µL of the product of first-round PCR was mixed with 24 µL of master mix that consisted of 12.5 µL of the SybrGreen/Fluorescein qPCR Maxima Master Mix (2x), 1 µL of (1 µM) forward fusion primer M13-515FB (TGTAAAACGACGGCCAGT GTGYCAGCMGCCGCGGTAA), and 1 µL of (10 µM) reverse fusion primer P1-806R (CCTCTCTATGGGCAGTCGGTGAT GGACTACHVGGGTWTCTAAT). Additionally, 1 µL of barcoded IonA-M13 fusion primers (10 µM) were added to each PCR reaction so that they all included individual barcodes used for identifying the samples.
All amplifications were performed with CFX96TM qPCR instrument (Bio-Rad, CA, United States). The reaction conditions were 95 °C for 10 min (initial denaturation), followed by 35 cycles for 30 s (denaturation); 50°C, 30 s (annealing); 72°C, 1 min (extension), a 5 min final extension at 72°C, and 4°C maintenance. The SYBR-fluorophore curves of the qPCRs were checked with CFX Maestro software to verify amplifications were successful.
Second-round PCR products were purified with AMPureXP magnetic beads (Beckman Coulter, CA, United States) using manufacturers’ protocol. DNA concentration of the purified products were quantified with Qubit (Qubit Fluorometric Quantification, Thermo Fischer Scientific), using QubitTM 1x dsDNA HS Assay Kit (Life Technologies Co., UT, United States). A library for the sequencing was constructed by pooling 20 ng of DNA from each sample.
2.3.2 Ion Torrent Sequencing and Data Processing
The Ion Torrent sequencing of the library was made in the premises of the Department of Biological and Environmental Sciences of the University of Jyväskylä (Finland). The emulsion PCR was done on the Ion OneTouchTM 2 system following the Ion PGM Hi-Q View OT2 Kit (Life Technologies) according to instructions of the manufacturer (Life Technologies, United States). The resulted ion sphere particles (ISPs) were loaded and sequenced on an Ion 318TM Chip v2 using Hi-Q View Sequencing Kit (Life Technologies) that generated ca. 5.4 million reads with an average read length of 265 bp. For a reason, unknown, samples startB, cntrlB, and Hx1B were unsuccessfully sequenced and had to be discarded from further analysis.
The resulted data was processed in CLC Genomics Workbench 12 software (Qiaqen). The data were trimmed to discard the primers (515FB and 806r) and sequences shorter than 150 bp. Moreover, the sequences were trimmed to have a unified length of 260 bp, and the operational taxonomic unit (OTU) clustering was made based on SILVA 16S v132 99% database with a 99% similarity assumption, allowing the creation of new OTUs with 80% taxonomic similarity.
The effects on the microbial community structure were examined at the phylum, class, and family level. Family level changes were displayed visually by performing a multidimensional scaling (MDS) plot using. Furthermore, OTUs with abundance of <1% across the whole data, were excluded from the MDS plot.
2.4 Biovolume Assessment
A flow cytometric (FCM) bacterial count analysis was performed for the samples taken after the incubation. For this, the samples were prepared by following the InvitrogenTM Bacteria Counting Kit® (Thermo Fischer Scientific, United States) protocol of the manufacturer apart from not adding the microsphere standard suspension.
The samples were analyzed with a Guava® easyCyteTM HT flow cytometer (Luminex®, United States) following the instructions of the manufacturer. The data was processed with the software provided by the manufacturer, and the results (cells/ml) are expressed as the total number of bacterial cells in the sample using formula (1):
where TBCn is the total bacteria count of sample n, BVn, and BVblank, are the biovolume of sample n and blank (cells/ml), whereas 10 is a correction factor for dilution, and Vn is the total volume (mL) of sample n.
2.5 CO2 Measurements
For the analysis, a 10 mL air sample was taken from each treatment and injected into a helium-washed flat bottomed 12 mL Exetainer® vial (due to a systematic error, the sample volume in the first measurements was 11 mL). During the 28 days incubation, a total of four measurements were taken in seven days intervals. The samples were analyzed with an Agilent 7890B GC greenhouse gas chromatograph (Agilent Technologies, Palo Alto, CA, United States). The configuration of the equipment consisted of two separate channels with stainless steel packed columns (HayeSep Q 80/100, length 8 feet × inner diameter 1/8″) using three detectors: flame-ionization (FID), thermal conductivity (TCD), and micro electron capture (μ-ECD). Low CO2-level samples were converted to CH4 through the methanizer and measured by FID. The temperatures at the column and valve box during the runs were 60 °C.
To get the final CO2-amounts, the results given by the device had to be multiplied by a correction factor (CF) which was calculated by using formula (2):
From the results obtained, the average CO2 amounts for each week were calculated for the treatments, and a graph was formed for each treatment, showing the change in the amount of CO2 over time. The change in CO2 concentration observed during each week of the experiment was reported in both ppm and as percentage.
2.6 Statistical Testing
The statistical differences in the structures of the microbial communities at the family level (including only the families with a combined abundance of >1% across the whole data) were examined with permutational multivariate analysis of variance (PERMANOVA) using the Monte Carlo test. The multidimensional scaling (MDS) plot was created using Bray Curtis resemblance and a Pearson difference of 80% was used for the clustering. The inhibitory effect of the compounds on the growth of microbial biomass was statistically tested by comparing the treatments with the control, and the effect of dose and differences between compounds were examined with post-hoc tests. The statistical comparisons of the CO2 measurement results were made separately for the measurements of each week, possible outliers were discarded, Shapiro-Wilkin was used to check normality, and Levene’s test to check the homogeneity of variances.
The confidence level for all the tests was 95% (* p ≤ 0.05; ** p ≤ 0.01; *** p ≤ 0.001) and they were made with IBM® SPSS® Statistics (v.26) with the exception that the multidimensional scaling (MDS) and PERMANOVA were performed with PRIMER-E (v.7, Ivybridge, United Kingdom).
3 Results
3.1 The Compounds Impact on Microbial Diversity was not Significant
The operational taxonomic unit (OTU) clustering of the data based on SILVA database resulted in obtaining a total of 53,053 OTUs of which 97.6% were de novo OTUs based on 80% similarity assumption.
Interestingly, all the incubated treatments showed more taxonomic diversity than the unincubated treatment (Start), as the number of OTUs was 21.2–32.4% higher in the incubated treatments (Figure 1). Moreover, against the assumption, statistical comparison of diversity based on the number of OTUs showed no significant differences when comparing the control to PFHxA and PFOA treatments (ANOVA: p > 0.05). However, the results suggested that the PFOA slightly reduced the diversity since the number of OTUs in treatments O1, O2, and O3 were, respectively, 6.4, 1.6, and 4.2% lower than in the control, but the increasing dosage seemed to have no effect. More details about diversity results are represented in the Supplementary Material (Supplementary Table S2).
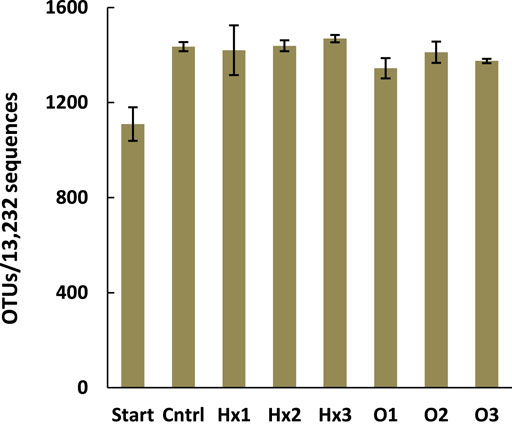
FIGURE 1. The mean number of operational taxonomic units (OTUs) in the treatments after an equal amount of 13,232 sequences were analyzed per treatment. Error bars represent the SDs and n = 4, except for treatments Cntrl and Hx1 n = 3 and for Start n = 2.
3.2 Both Compounds Caused Structural Changes on Microbial Community at Family Level
A total of seven phyla with a combined abundance of more than 1% were found in the total data. Gram-negative bacteria dominated, as only one of the major phyla was gram-positive. The most abundant phylum was Proteobacteria, followed by Planctomycetes, Verrucomicrobia, Bacteroidetes, and Actinobacteria, as their combined abundance in the whole dataset was 93.6% (Supplementary Figure S1). Similarly, a total of 11 classes were obtained with an abundance of >1%. Gammaproteobacteria was the most abundant across the data, followed by Alphaproteobacteria, Planctomycetacia, Verrucomicrobiae, and Bacteroidia, which together stood for 79.7% of the bacteria (Supplementary Figure S2).
At family level. a total of 432 families were obtained of which 26 had a combined abundance of more than 1% across the whole data. The bacteria belonging to these 26 most abundantly present families represented 68.0–76.6% of all the bacteria in the treatments. Moreover, in terms of the most abundant families, there were more differences between treatments than at the phylum and class level (Figure 2). For instance, in the control, the relative abundances of the families ranked as Burkholderiaceae > Opitutaceae > Phycisphaeraceae > Pirellulaceae > Gemmatimonadaceae, while in all PFCA treatments Pirellulaceae were more abundant than Phycisphaeraceae and Gemmatimonadaceae was relatively less abundant compared to other families such as Sporichthyaceae and Gemmataceae. However, Burkholderiaceae was the most abundantly present family in all treatments.
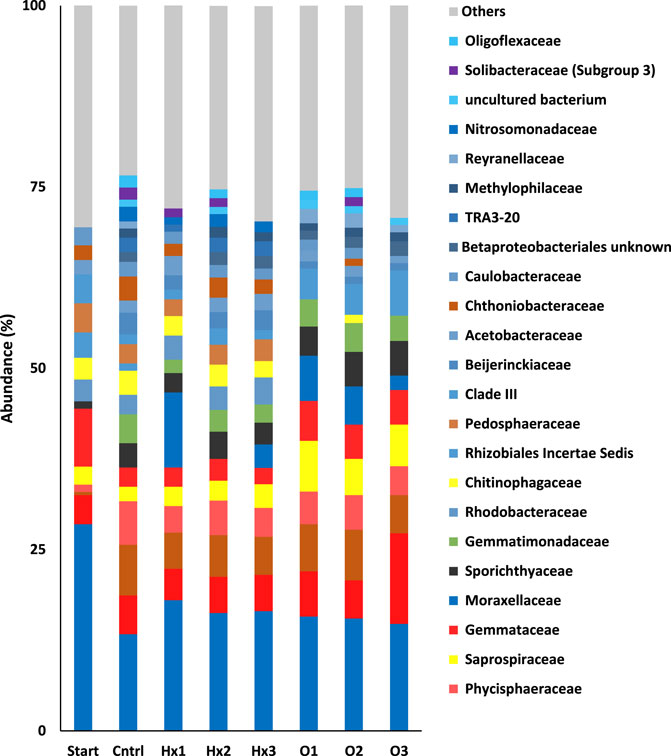
FIGURE 2. The relative mean abundances of the families in the treatments. Others represent the sum of abundance of families with a combined abundance less than 1% across the whole data. Note that the families belonging to same class share the common base color.
As expected, statistical testing revealed that both compounds significantly changed the structure of the microbial community at high concentrations (10 mg/L). When comparing the treatments Hx3 and O3 to control, they had p-values of 0.021 and 0.001 (PERMANOVA, Monte Carlo test), respectively. Moreover, for both compounds, the high concentration altered the microbial community structure most significantly. However, even the 100 ng/L concentration of PFOA significantly changed the microbial community structure, and the observed change was as significant as with the 100 μg/L concentration (PERMANOVA: p = 0.003 for both O1 and O2 compared to control). Surprising results were also obtained when comparing PFHxA treatments, as the microbial community at the highest concentration differed significantly from Hx2 (p = 0.015) but not from Hx1. Moreover, statistical testing showed the more potent toxicity of PFOA since the pair-wise comparison of Hx1 to O1, Hx2 to O2, and Hx3 to O3 had p-values of 0.031, 0.001, and 0.001, respectively.
Multidimensional scaling (MDS) showed excellent clustering of different treatments, as all of the PFOA treatments were clustered together and PFHxA treatments clustered with control (Figure 3). Only sample Hx1C separated from all the other incubated samples, primarily because of its substantially higher abundance of Moraxellaceae (Figure 2). Based on Pearson correlations, the most significant differences on the MDS1-axis were caused by the differences in abundances between families Burkholderiaceae, Sporichthyaceae, Opitutaceae, and Gemmatimonadaceae. Therefore, the most significant change during the incubation that separated the incubated treatments from the Start was the decreased abundance of Burholderiaceae, resulting in higher abundances of Sporichtyaceae, Opitutaceae, and Gemmatimonadaceae. Similarly, in the MDS2-axis, changes in the relative abundances in the families of Gemmataceae, Rhizobiales Incertae Sedis, Beijerinckiaceae, and Betaproteobacteriales most significantly explained changes in microbial communities between the treatments. Consequently, the most significant differences in the microbial communities of PFOA treatments compared to control and PFHxA treatments were their relatively higher quantities of Saprospiraceae, Sporichthyaceae, Rhizobiales Incertae Sedis, and Gemmataceae, which resulted in comparatively lower abundances of TRA3-20 and Beijerinckiarcaeae. Moreover, treatment O3 differed from O1 and O2 due to its higher abundance of Pirellulaceae. Thus, an elevated quantity of Saprospiraceae and Pirellulaceae in aquatic environments may indicate a high concentration of PFOA. Similarly, the significant difference between PFOA treatment Hx3 compared to Hx2, and control was caused mainly by differences in the abundances of Moraxellaceae, uncultured bacterium, Solibacteraceae (Subgroup 3), and Oligoflexaceae. Thus, a high level of Moraxellaceae may be an indicator of PFHxA in freshwater ecosystems.
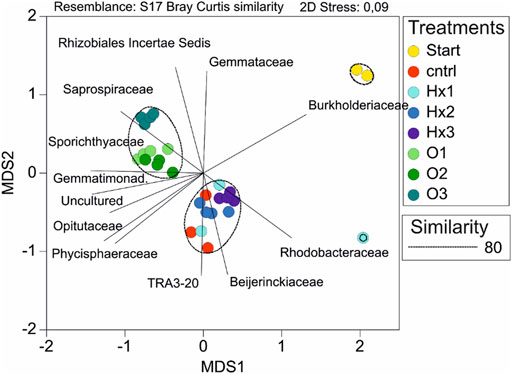
FIGURE 3. Multidimensional scaling (MDS) of the microbial community compositions of the treatments showed significant separation of samples as the 2D stress value was <0.1. Treatments are separated by the color and the shading of the same base color separates the dose of the individual PFCA. Moreover, the circles indicate the clustering of samples sharing similar microbial community structures under 80% similarity assumption and lines represent the families which most strongly explained the structural differences in microbial communities between treatments (Pearson correlation threshold value 0.8).
3.3 Both Compounds Inhibited the Microbial Growth
With flow cytometry (FCM), the highest total bacteria count (TBC) was obtained in the control (8.95 × 1010 cells), and the TBCs of the PFCA treatments were 18.1–55.2% lower compared to that (Supplementary Table S3). Compared to control, TBCs showed statistically significant inhibition with treatments Hx2, Hx3, and all PFOA treatments (Tukey’s HSD: p < 0.05, see Figure 4). Moreover, according to hypothesis, there was a negative correlation between the increasing concentration and TBC, with both compounds. Although with PFOA, the effect on TBC showed similar responses with both 100 ng/L and 100 μg/L concentrations (Figure 4).
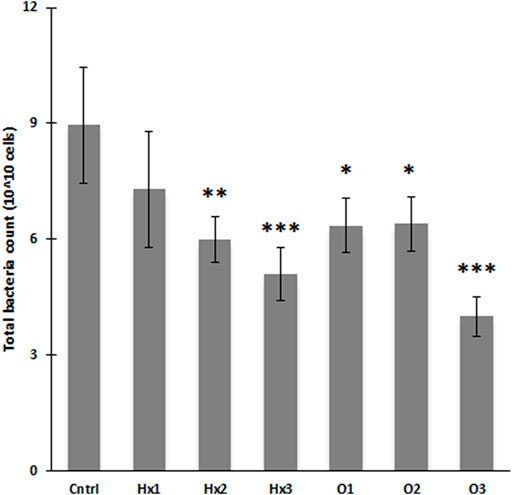
FIGURE 4. Flow cytometrically determined biovolumes as total bacteria counts for different treatments after the incubation (n = 4). Error bars represent the SDs and statistical significance deviation from control (* p ≤ 0.05; ** p ≤ 0.01; *** p ≤ 0.001).
Expectedly, PFOA decreased the number of microbes more effectively than PFHxA. The TBCs for 100 ng/L and 10 mg/L PFOA treatments were 13.0 and 21.6% lower than for PFHxA at same concentrations. Even though, at 100 μg/L the TBC of PFHxA was 6.7% higher than in the corresponding PFOA treatment, the difference was not significant (Tukey’s HSD: p > 0.05). Surprisingly, there was no statistical differences between the compounds, whereas the increasing dose had an expected effect. The observed negative correlation of TBC with the rising PFHxA concentration was statistically significant as 10 mg/L PFHxA treatment had significantly lower (-30.0%) TBC than 100 ng/L PFHxA treatment (Tukey’s HSD: p = 0.047), and PFOA at highest concentration (10 mg/L) showed significantly lower (−37.0 and -37.5%) TBCs than 100 ng/L and 100 μg/L PFOA treatments with p-values of 0.038 and 0.035, respectively (Tukey’s HSD).
3.4 Effects on the Microbial Respiration
The CO2 concentrations growth-curves (Figure 5) indicate that all treatments shared the same type of growth rate during the experiment. Moreover, Figure 5 well illustrates that the significant differences between the CO2 concentrations of the treatments occurred during the first week of incubation and remained approximately the same or leveled off during the experiment. In all treatments, the CO2 concentrations grew fastest during the first two weeks of incubation, after which the concentrations stopped growing or began to decrease.
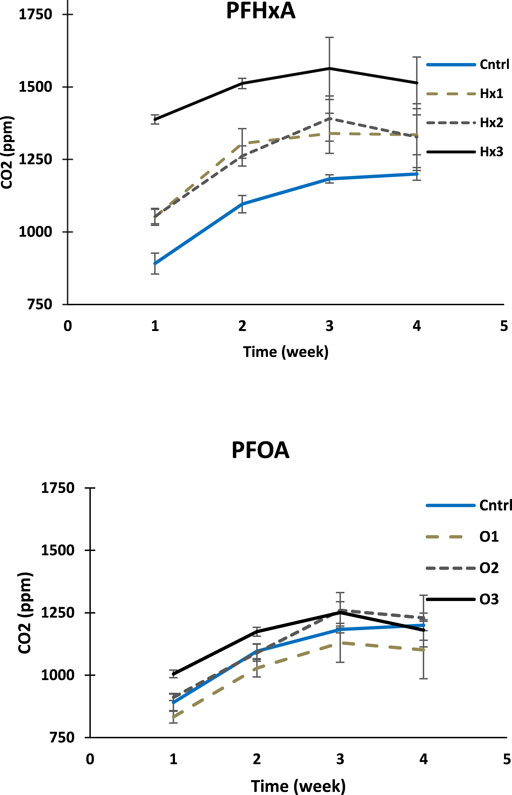
FIGURE 5. The CO2 concentrations growth curves are shown as each measuring week mean CO2 ppm-levels for PFHxA and PFOA treatments compared to control (the error bars represent SDs; n = 4). Note that both figures have the same scaling in the y-axis starting from 750 ppm.
As expected, the changes of CO2 ppm-levels in PFCA-treatments with the highest concentration were lower than the change in control at all weeks of the experiment, suggesting that the presence of the compounds have some inhibitory effect with high concentration (Supplementary Table S4). However, the statistical testing showed no significant inhibition during the experiment (Welch’s ANOVA, p > 0.05). Instead, the comparison of mean total CO2 amounts of the treatments showed that all PFHxA treatments and treatment O3 had significantly higher CO2 concentrations than control after the first week of incubation (Tukey’s HSD: p < 0.05). Notably, none of the PFCA treatments showed significantly lower total CO2 concentrations at any incubation stage compared to control.
Surprisingly, the CO2 concentrations in less toxic PFHxA treatments differed more from control than PFOA treatments. The total mean CO2 concentration of 100 ng/L PFHxA treatment was significantly higher than in control for the first two weeks, 100 μg/L treatment until the third week, and 10 mg/L treatment throughout the experiment (Supplementary Table S5). PFHxA seems to have an accelerating effect on microbial cell respiration during the first week, which positively correlates with the concentration of the compound.
The only PFOA treatment with a significantly different CO2 concentration compared to control during the incubation was 10 mg/L treatment after the first week having 12.8% more CO2 (p = 0.000). However, the difference leveled off by the end of the experiment, suggesting that the amount of CO2 had increased faster in control (4th-week p = 1.000). At other concentrations, the effect of PFOA varied. At 100 ng/L treatment, the total mean CO2 concentration was 4.5–8.3% lower than in control throughout the experiment, and at 100 μg/L treatment variably higher or lower at different stages of the experiment (Supplementary Table S4).
When comparing the compounds, the CO2 concentrations of the 100 ng/L and 10 mg/L PFOA treatments were significantly lower compared to the concentrations of PFHxA, at each week of the experiment, while the corresponding difference for 100 μg/L PFCA treatments was significant only for the first two weeks (Table). Moreover, contrary to the assumption, PFOA did not affect the cell respiration of the aquatic microbes more strongly than PFHxA (Welch’s ANOVA: p > 0.05).
Notably, sample Hx3A was discarded from statistical analysis as an outlier. The calculated mean CO2 concentrations and the obtained p-values of the post-hoc test comparing different treatments are summarized in Supplementary Tables S4,S5.
4 Discussion
4.1 PFCAs Impacts on the Diversity and Structure of the Microbial Community
Persistent organic pollutants (POPs) cause concern as they force organisms to adapt to their presence. Very little research has been done on the effects of perfluorinated alkyl acid (PFCAs) on the structure and diversity of freshwater microbial communities. Therefore, in this study, we investigated the structural changes in the microbial community of Lake Vesijärvi (Finland) caused by PFOA and PFHxA. Our results suggested that neither one of the compounds affected the diversity of the community but caused structural changes at high concentration (10 mg/L). However, PFOA showed a more substantial impact as it also affected the structure significantly with lower concentrations of 100 ng/L and 100 μg/L. Although more significant structural changes occurred at the highest concentrations, the significance of the increasing concentration was lower than expected.
Previous studies have suggested that PFOA could affect microbial community structure and diversity in freshwaters (Zhang et al., 2019), sediments (Sun et al., 2016), and soils (Qiao et al., 2018; Cai et al., 2020). However, some of the results of these earlier studies show inconsistencies with the present study. For instance, Zhang et al. (2019) obtained an evident increase of Proteobacteria (12.5–18.6%) that positively correlated with the concentration. In this study, the relative abundance of Proteobacteria was only increased by 1.4 and 3.0% with 100 ng/L and 100 μg/L PFOA concentrations and decreased by 8.9% with 10 mg/L concentration, showing a contrary response. Moreover, Zhang et al. (2019) obtained a significant increment in the relative abundance of Chloroflexi, which did not occur in the present study, whereas our results showed a decreased quantity of Planctomycetes, which did not occur in their results. Furthermore, as their results showed decreased relative abundances of Actinobacteria (26.5–38.8%) and Bacteroidetes (40.5–70.7%) in this study, their relative abundances increased 13.2–66.0 and 14.3–47.1%, respectively. The only similar response at the phylum level was the decreased relative quantity of Verrucomicrobia. However, the obtained decreasement by Zhang et al. (2019) was twofold higher than here. These results are very comparable in terms of doses, as Zhang et al. (2019) used almost similar concentrations (450 ng/L, 130 μg/L, and 5 mg/L) as used here. Some of the differences in the obtained results are most likely due to differences in the original compositions of the microbial communities. For instance, Chloroflexi was not among the most abundantly present phyla in the sampled water of this work and vice versa for Planctomycetes.
This was the first time when the impact of PFHxA on microbial community structure and diversity was assessed. Therefore, the obtained results cannot be similarly compared to earlier findings. However, as expected, the effects of PFHxA on the microbial community structure were milder than those caused by PFOA, and the significant changes were all caused by the high 10 mg/L concentration. In general, exposure to PFHxA increased the relative abundance of Proteobacteria, which led to decreased quantities of Planctomycetes, Verrucomicrobia, Gemmatimonadetes, and Acidobacteria.
The earlier study by Hu et al. (2014) suggested that different taxonomic groups of microorganisms show variating responses to PFOA toxicity. Since the results of our flow cytometric (FCM) biovolume assessment showed inhibition of the growth, it is likely that the structural changes here are the result of other bacterial selection by growth inhibition. Since Proteobacteria and Planctomycetes were the most abundant phyla in the studied water, changes in the relative abundances of families inside these phyla most significantly explained the differences between the microbial communities exposed to PFOA and PFHxA. An elevated abundance of strain Rhizobialez Insertae Sedis, Saprospiraceae, Pirellules, and Gemmataceae might indicate the presence of PFOA in aquatic environments, as they showed low sensitivity towards the toxic compound. In turn, Rhodobacteraceae and Saprospiraceae positively correlated with PFHxA suggesting that the families resist PFHxA contamination. Moreover, Moraxellaceae may express the presence of PFHxA. However, there was a high variance between the treatments and samples regarding the quantity of Moraxellaceae in PFHxA treatments.
The quality of the results and the value of the conclusions drawn from them are undermined because most operational taxonomic units (OTUs) were produced de novo with an assumption of 80% similarity (97.6% of all the OTUs). Moreover, discarding the families showing relative abundance of <1% across the whole data led to discarding rare families with a combined abundance of 23.4–32.0% in the treatments. Consequently, some significant groups abundantly present in some treatment may have gone unnoticed. The low similarity assumption, in turn, increases the probability that closely related bacteria have been incorrectly clustered to represent the same OTUs. This may also explain why the diversity-related changes observed in previous studies were not observed in this experiment. According to Sun et al. (2016) PFOA suppressed the diversity of a microbial community of river sediment when the concentration was higher than 100 ng/L. Similar finding was made by Zhang et al. (2019) who recorded significant reduction of microbial diversity of freshwater microbial community with PFOA at 130 μg/L and 5 mg/L but not with 450 ng/L.
Despite the inconsistencies with the earlier study, our results further confirmed the notion that the PFOA can alter the structure of a freshwater microbial community and that the effects of PFHxA are much milder. Furthermore, there was no significant difference between the changes produced by the highest and lowest concentrations of PFHxA. Besides, examining changes by comparing the abundance ratios of different bacterial species does not necessarily tell the whole truth about the significance of the changes. It would be important to investigate the effects of the structural changes on the microbial processes that maintain the aquatic ecosystem. Therefore, further research should focus on clarifying these issues. However, studies such as these should also be done to improve the overall picture of structural changes. Moreover, we find it important, that future studies would take sub-samples during the incubation period, in order to follow-up and to see the variation of the structural changes in the microbial community. This would also give additional information about the toxic potency of the compounds.
4.2 PFCAs Effects on the Microbial Growth
Previous studies investigating the growth inhibition by PFCAs have focused on acute responses with shorter incubation times and higher concentrations. Also, studies have targeted individual microorganisms, e.g., algal species, instead of the whole microbial community. For instance, Hu et al. (2014) reported that after 8-days exposure to PFOA (10–40 mg/L), the growth of freshwater microalgae C. reinhardtii was significantly inhibited, whereas growth of S. obliquus was only slightly suppressed, indicating variating responses depending on the organisms.
In the present study, microbial growth was examined flow cytometrically (FCM) and our experiment was able to show significant inhibition of microbial growth for both compounds. As expected, the increasing concentration added to the inhibition. However, contrary to assumption, the inhibitory effect of PFOA was not significantly more substantial than PFHxA’s, which raises concerns about the unregulated use of PFHxA. Notably, some inhibitory effects may have been “lost” if there were bacteria in the community whose growth was promoted by the compounds, a phenomenon that cannot be considered entirely impossible. Thus, we conclude that studies targeting the growth of model microorganisms (e.g., E. coli bacteria) would serve better to tell the responses on microbial growth.
4.3 PFCAs Effects on the Microbial Respiration
Microbial respiration is a key process affecting the aquatic life cycle of carbon and information about the effects of toxic chemicals on the process is scarce. Here, we investigated the effects of PFOA and PFHxA on aquatic microbial respiration, which according to our knowledge, have not been previously studied. On the other hand, few studies have focused on the ecotoxicological effects of PFOA on soil microbes, and, for instance, Xu et al. (2021) concluded that PFOA could inhibit soil respiration at high concentrations. Their notion is inconsistent with the observations of the present study as our results suggested that PFHxA and PFOA did not significantly inhibit the microbial respiration of lake microorganisms. Instead, PFHxA showed a significant stimulating effect.
The higher ppm levels observed in the 10 mg/L PFHxA treatment after the first week of incubation might be due to differences in the initial CO2 concentrations in the sample, as the base level was not measured. However, in this case, the results would have shown a lot more variation between the replicates within the same treatment. Consequently, it can be concluded that PFHxA is likely to have an acute accelerating impact on microbial respiration. During the first week the most sensitive bacteria may have died, and CO2 was released as decomposers decomposed the dead bacteria. Same may have happened with PFOA, but the inhibition of microbial degradation has been stronger, which kept the CO2 concentration low. Besides, a study conducted by He et al. (2016), demonstrated that on soils, PFOA inhibited beneficial microbial processes such as dehydrogenase and urase, leaving space to believe that it might also disturb the microbial degradation process on aquatic environments.
The obtained elevated CO2 concentrations with PFHxA exposure could also be a sign of structural changes. Hence, to better understand the effect of the compounds on aquatic microbial respiration, the possible accelerating effect of PFHxA should be investigated, focusing on the first week of the experiment with short measurement intervals, and also, by monitoring the changes in the microbial community throughout the incubation. Moreover, the effect of the compounds on different microorganisms might vary. If for some microbes, the effect of the compounds on respiration is stimulating and for some inhibitory, such responses of a single microorganism cannot be verified by examining changes in the total amount of carbon dioxide throughout the community, as the responses may cancel out each other. Consequently, we suggest the inhibitory effect to be studied for individual microorganisms separately.
5 Conclusion
The ecotoxicological effects of PFOA and PFHxA on aquatic microbes have been poorly studied. Here, we have investigated how the presence of the compounds affect the diversity and structure, biovolume, and activity of a freshwater microbial community as a whole. The phylum, class, and family level analysis of the bacterial community showed that both compounds with high concentration altered their structure but did not affect the diversity. However, the observed structural changes differed from earlier findings. The bacterial biovolume was inhibited by the compounds, while inhibition of activity via measuring cell respiration could not be demonstrated. In accordance with the hypothesis, PFOA showed more potent toxicity causing more structural changes to the microbial community than PFHxA.
Future experiments should aim to understand how the microorganisms adapt to the continuous presence of these compounds, and what are the overall impacts of these adaptations towards ecosystems. In general, there should be a shift from studying long-chain PFCA homologues to studying short-chain PFCA homologues, since the short-chain PFCAs concentrations in the environment are likely to exceed long-chain homologues in the future. Although, this study helped to better understand the ecotoxicity of PFCAs on aquatic microbes, the information about their physicochemical properties, toxicity, environmental behavior, and fate is scarce. Therefore, before the overall environmental risk of these compounds can be assessed in a comprehensive and reliable manner, their manufacture and use should be globally more regulated in efforts to minimize emissions to the environment.
Data Availability Statement
The authors of the study confirm that the data supporting the findings of this study are available within the article and its Supplementary Materials. The raw sequencing data has been deposited in the Sequenced Read Archive (SRA) of NCBI under accession number PRJNA825694.
Author Contributions
MT, ST, VP, and ML contributed to planning the research and designing the laboratory experiments. ML conducted the experiment and all the analyses, MT contributed to DNA analysis and Ion Torrent data analysis, ST contributed to MDS-plot, and JV contributed to CO2-measurements and flow cytometric measurements. ML compiled the data, performed the statistical tests, and wrote the initial version of the manuscript. All authors contributed to revising and commenting on the manuscript.
Funding
This research was supported by the Academy of Finland research grant 333564, awarded to ST, and by Work Scholarship granted for ML is 4221 by Maa-ja vesitekniikan tuki ry association.
Conflict of Interest
The authors declare that the research was conducted in the absence of any commercial or financial relationships that could be construed as a potential conflict of interest.
Publisher’s Note
All claims expressed in this article are solely those of the authors and do not necessarily represent those of their affiliated organizations, or those of the publisher, the editors and the reviewers. Any product that may be evaluated in this article, or claim that may be made by its manufacturer, is not guaranteed or endorsed by the publisher.
Acknowledgments
We are grateful to all the people who aided with the field and laboratory analyses. Foremost we wish to thank Jaakko Litmanen for collecting the sample water, Elina Virtanen for conducting the NGS run, and Hannu Pakkanen for his assistance with the CO2-measurements.
Supplementary Material
The Supplementary Material for this article can be found online at: https://www.frontiersin.org/articles/10.3389/fenvs.2022.888171/full#supplementary-material
References
Abunada, Z., Alazaiza, M. Y. D., and Bashir, M. J. K. (2020). An Overview of Per- and Polyfluoroalkyl Substances (PFAS) in the Envirnoment: Source, Fate, Risk and Regulations. Water 12 (20), 3590. doi:10.3390/w12123590
Alexander, B. H. (2001). “Mortality Study of Workers Employed at the 3M cottage grove Facility,” in Final Rep. Environ. Occup. Health Sch (Gottage Grove: Public Health Univ. Minn.), 143–146.
Barmentlo, S. H., Stel, J. M., van Doorn, M., Eschauzier, C., de Voogt, P., and Kraak, M. H. S. (2015). Acute and Chronic Toxicity of Short Chained Perfluoroalkyl Substances to Daphnia magna. Environ. Pollut. 198, 47–53. doi:10.1016/j.envpol.2014.12.025
Bissett, A., Burke, C., Cook, P. L. M., and Bowman, J. P. (2007). Bacterial Community Shifts in Organically Perturbed Sediments. Environ. Microbiol. 9 (1), 46–60. doi:10.1111/j.1462-2920.2006.01110.x
Briée, C., Moreira, D., and López-García, P. (2007). Archaeal and Bacterial Community Composition of Sediment and Plankton from a Suboxic Freshwater Pond. Res. Microbiol. 158, 213–227. doi:10.1016/j.resmic.2006.12.012
Buck, R. C., Franklin, J., Berger, U., Conder, J. M., Cousins, I. T., De Voogt, P., et al. (2011). Perfluoroalkyl and Polyfluoroalkyl Substances in the Environment: Terminology, Classification, and Origins. Integr. Environ. Assess. Manag. 7 (4), 513–541. doi:10.1002/ieam.258
Cai, Y., Chen, H., Yuan, R., Wang, F., Chen, Z., and Zhou, B. (2020). Metagenomic Analysis of Soil Microbial Community under PFOA and PFOS Stress. Environ. Res. 188, 109838. doi:10.1016/j.envres.2020.109838
Cotner, J. B., and Biddanda, B. A. (2002). Small Players, Large Role: Microbial Influence on Biogeochemical Processes in Pelagic Aquatic Ecosystems. Ecosystems 5, 105–121. doi:10.1007/s10021-001-0059-3
Davis, K. L., Aucoin, M. D., Larsen, B. S., Kaiser, M. A., and Hartten, A. S. (2007). Transport of Ammonium Perfluorooctanoate in Environmental media Near a Fluoropolymer Manufacturing Facility. Chemosphere 67, 2011–2019. doi:10.1016/j.chemosphere.2006.11.049
ECHA (2018). Annex XV – Identification of Undecafluorohexanoic Acid and its Ammonium Salt as SVHC. Available as pdf-file from: http://www.echa.europa.eu/documents/.
Echavarri-Bravo, V., Paterson, L., Aspray, T. J., Porter, J. S., Winson, M. K., Thornton, B., et al. (2015). Shifts in the Metabolic Function of a Benthic Estuarine Microbial Community Following a Single Pulse Exposure to Silver Nanoparticles. Environ. Pollut. 201, 91–99. doi:10.1016/j.envpol.2015.02.033
Eschauzier, C., de Voogt, P., Brauch, H.-J., and Lange, F. T. (2012). “Polyfluorinated Chemicals in European Surface Waters, Ground- and Drinking Waters,” in Polyfluorinated Chemicals and Transformation Products – Handbook of Environmental Chemistry (Springer-Verlag), 73–102. doi:10.1007/978-3-642-21872-9_5
Gerbersdorf, S. U., Hollert, H., Brinkmann, M., Wieprecht, S., Schüttrumpf, H., and Manz, W. (2011). Anthropogenic Pollutants Affect Ecosystem Services of Freshwater Sediments: the Need for a “Triad Plus X” Approach. J. Soils Sediments 11, 1099–1114. doi:10.1007/s11368-011-0373-0
Glüge, J., Scheringer, M., Cousins, I. T., DeWitt, J. C., Goldenman, G., Herzke, D., et al. (2020). An Overview of the Uses of Per- and Polyfluoroalkyl Substances (PFAS). Environ. Sci. Process. Impacts 22, 2345–2373. doi:10.1039/D0EM00291G
Haukås, M., Berger, U., Hop, H., Gulliksen, B., and Gabrielsen, G. W. (2007). Bioaccumulation of Per- and Polyfluorinated Alkyl Substances (PFAS) in Selected Species from the Barents Sea Food Web. Environ. Pollut. 148 (1), 360–371. doi:10.1016/j.envpol.2006.09.021
He, W., Megharaj, M., and Naidu, R. (2016). Toxicity of Perfluorooctanoic Acid towards Earthworm and Enzymatic Activities in Soil. Environ. Monit. Assess. 188, 424. doi:10.1007/s10661-016-5416-y
Henning, M. H., and Fuchsman, P. C. (2021). Ecological Risk Assessment of Per‐ and Polyfluorinated Alkyl Substances: Foreword. Integr. Environ. Assess. Manag. 17 (4), 670–672. doi:10.1002/ieam.4465
Hu, C., Luo, Q., and Huang, Q. (2014). Ecotoxicological Effects of Perfluorooctanoic Acid on Freshwater microalgaeChlamydomonas reinhardtiiandScenedesmus Obliquus. Environ. Toxicol. Chem. 33 (5), 1129–1134. doi:10.1002/etc.2532
Jing, P., Rodgers, P. J., and Amemiya, S. (2009). High Lipophilicity of Perfluoroalkyl Carboxylate and Sulfonate: Implications for Their Membrane Permeability. J. Am. Chem. Soc. 131, 2290–2296. doi:10.1021/ja807961s
Kato, H., Fujii, S., Takahashi, M., Matsumoto, M., Hirata-Koizumi, M., Ono, A., et al. (2014). Repeated Dose and Reproductive/developmental Toxicity of Perfluorododecanoic Acid in Rats. Environ. Toxicol. 30 (11), 1244–1263. doi:10.1002/tox.21996
Knepper, T. P., Lange, F. T., Barcelo, D., Bergmann, A., Brauch, H.-J., Buck, R. C., et al. (2012). Polyfluorinated Chemicals and Transformation Products. Handbook Environ. Chem. 17, 1–40. doi:10.1007/978-3-642-21872-9
Latala, A., Nedzi, M., and Stepnowski, P. (2009). Acute Toxicity Assessment of Perfluorinated Carboxylic Acids towards the Baltic Microalgae. Environ. Toxicol. Pharmacol. 22 (2), 167–171. doi:10.1016/j.etap.2009.03.010
Lau, C., Anitole, K., Hodes, C., Lai, D., Pfahles-Hutchens, A., and Seed, J. (2007). Perfluoroalkyl Acids: a Review of Monitoring and Toxicological Findings. Toxicol. Sci. 99, 366–394. doi:10.1093/toxsci/kfm128
Londono, N., Donovan, A. R., Shi, H., Geisler, M., and Liang, Y. (2017). Impact of TiO2 and ZnO Nanoparticles on an Aquatic Microbial Community: Effect at Environmentally Relevant Concentrations. Nanotoxicology 11 (9-10), 1140–1156. doi:10.1080/17435390.2017.1401141
Moody, C. A., Hebert, G. N., Strauss, S. H., and Field, J. A. (2003). Occurrence and Persistence of Perfluorooctanesulfonate and Other Perfluorinated Surfactants in Groundwater at a Fire-Training Area at Wurtsmith Air Force Base, Michigan, USAElectronic Supplementary Information (ESI) Available: Map of Location of Wurtsmith Air Force Base, Oscoda, MI and Surrounding States. See http://www.rsc.Org/suppdata/em/b2/b212497a/. J. Environ. Monitor. 5 (2), 341–345. doi:10.1039/b212497a
Muir, D., and Miaz, L. T. (2021). Spatial and Temporal Trends of Perfluoroalkyl Substances in Global Ocean and Coastal Waters. Environ. Sci. Technol. 55 (14), 9527–9537. doi:10.1021/acs.est.0c08035
Paumelle, M., Donnadieu, F., Joly, M., Besse-Hoggan, P., and Artigas, J. (2021). Effects of Sulfonamide Antibiotics on Aquatic Microbial Community Composition and Functions. Environ. Int. 146, 106198. doi:10.1016/j.envint.2020.106198
Qiao, W., Xie, Z., Zhang, Y., Liu, X., Xie, S., Huang, J., et al. (2018). Perfluoroalkyl Substances (PFASs) Influence the Structure and Function of Soil Bacterial Community: Greenhouse experiment. Sci. Total Environ. 642, 1118–1126. doi:10.1016/j.scitotenv.2018.06.113
Rand, A. A., and Mabury, S. A. (2017). Is There a Human Health Risk Associated with Indirect Exposure to Perfluoroalkyl Carboxylates (PFCAs)? Toxicology 375, 28–36. doi:10.1016/j.tox.2016.11.011
Reinikainen, J., Perkola, N., Takala, M., Äystö, L., and Ahkola, H. (2019). Perfluorattujen Alkyyliyhdisteiden Ympäristötutkimukset Ja Riskinarviointi, 21. Helsinki: Suomen ympäristökeskuksen raportteja.
Ren, Z., Qu, X., Peng, W., Yu, Y., and Zhang, M. (2019). Functional Properties of Bacterial Communities in Water and Sediment of the Eutrophic River-lake System of Poyang Lake, China. PeerJ 7, e7318. doi:10.7717/peerj.7318
Ritter, S. K. (2010). Fluorochemicals Go Short. Chem. Eng. News Archive 88 (5), 12–17. doi:10.1021/cen-v088n005.p012
Rodea-Palomares, I., Leganes, F., Rosal, R., and Fernandez-Pinas, F. (2012). Toxicological Interactions of Perfluorooctane Sulfonic Acid (PFOS) and Perfluorooctanoic Acid (PFOA) With Selected Pollutants. J. Hazard. Mater. 201–202, 209–218. doi:10.1016/j.jhazmat.2011.11.061
Seyoum, A., Pradhan, A., Jass, J., and Olsson, P.-E. (2020). Perfluorinated Alkyl Substances Impede Growth, Reproduction, Lipid Metabolism and Lifespan in Daphnia magna. Sci. Total Environ. 737, 139682. doi:10.1016/j.scitotenv.2020.139682
Sims, J. L., Stroski, K. M., Kim, S., Killeen, G., Ehalt, R., Simcik, M. F., et al. (2022). Global Occurrence and Probabilistic Environmental Health hazard Assessment of Per- and Polyfluoroalkyl Substances (PFASs) in Groundwater and Surface Waters. Sci. Total Environ. 816, 151535. doi:10.1016/j.scitotenv.2021.151535
Sun, R., Wu, M., Tang, L., Li, J., Qian, Z., Han, T., et al. (2018). Perfluorinated Compounds in Surface Waters of Shanghai, China: Source Analysis and Risk Assessment. Ecotoxicology Environ. Saf. 149, 88–95. doi:10.1016/j.ecoenv.2017.11.012
Sun, Y., Wang, T., Peng, X., Wang, P., and Lu, Y. (2016). Bacterial Community Compositions in Sediment Polluted by Perfluoroalkyl Acids (PFAAs) Using Illumina High-Throughput Sequencing. Environ. Sci. Pollut. Res. 23, 10556–10565. doi:10.1007/s11356-016-6055-0
Taipale, S. J., Peltomaa, E., Kukkonen, J. V. K., Kainz, M. J., Kautonen, P., and Tiirola, M. (2019). Tracing the Fate of Microplastic Carbon in the Aquatic Food Web by Compound-specific Isotope Analysis. Sci. Rep. 9, 19894. doi:10.1038/s41598-019-55990-2
Taipale, S., Peltomaa, E., and Salmi, P. (2020). Variation in ω-3 and ω-6 Polyunsaturated Fatty Acids Produced by Different Phytoplankton Taxa at Early and Late Growth Phase. Biomolecules 10 (4), 559. doi:10.3390/biom10040559
USEPA (2016). Health Effects Support Document for Perfluorooctanoic Acid (PFOA). Available at: https://www.epa.gov/sites/production/files/2016-05/documents/pfoa_hesd_final-plain.pdf (accessed April 20, 2020).
Wang, Y., Niu, J., Zhang, L., and Shi, J. (2014). Toxicity Assessment of Perfluorinated Carboxylic Acids (PFCAs) towards the Rotifer Brachionus Calyciflorus. Sci. Total Environ. 491-492, 266–270. doi:10.1016/j.scitotenv.2014.02.028
Xu, B., Yang, G., Lehmann, A., Riedel, S., and Rillig, M. C. (2021). Effects of Perfluoroalkyl and Polyfluoroalkyl Substances on Soil Structure and Function. bioRxiv. doi:10.1101/2021.10.26.465889
Zhang, D., Zhang, W., and Liang, Y. (2019). Bacterial Community of Freshwater Pond Responding to the Presence of Perfluorooctanoic Acid (PFOA). Environ. Technol. 9, 1–11. doi:10.1080/09593330.2019.1616828
Keywords: PFCAs, toxicity, micorbial community, rRNA gene sequencing, NGS
Citation: Laine MB, Vesamäki JS, Puupponen V-M, Tiirola M and Taipale SJ (2022) Comparing the Ecotoxicological Effects of Perfluorooctanoic Acid (PFOA) and Perfluorohexanoic Acid (PFHxA) on Freshwater Microbial Community. Front. Environ. Sci. 10:888171. doi: 10.3389/fenvs.2022.888171
Received: 02 March 2022; Accepted: 12 April 2022;
Published: 17 May 2022.
Edited by:
Pedro Neves Carvalho, Aarhus University, DenmarkReviewed by:
Jianfeng Feng, Nankai University, ChinaYunqiao Zhou, Institute of Tibetan Plateau Research (CAS), China
Copyright © 2022 Laine, Vesamäki, Puupponen, Tiirola and Taipale. This is an open-access article distributed under the terms of the Creative Commons Attribution License (CC BY). The use, distribution or reproduction in other forums is permitted, provided the original author(s) and the copyright owner(s) are credited and that the original publication in this journal is cited, in accordance with accepted academic practice. No use, distribution or reproduction is permitted which does not comply with these terms.
*Correspondence: Miikka B. Laine, bWliZWxhaW5Aanl1LmZp