- 1Earth and Environmental Sciences, Rensselaer Polytechnic Institute, Troy, NY, United States
- 2Earth and Atmospheric Sciences, Indiana University, Bloomington, IN, United States
- 3Institute for Arctic Biology, University of Alaska Fairbanks, Fairbanks, AK, United States
- 4Center for Genomics and Bioinformatics, Indiana University, Bloomington, IN, United States
- 5O’Neill School of Public and Environmental Affairs, Indiana University, Bloomington, IN, United States
Lakes are currently responsible for a significant amount of total natural methane emission. Microbial oxidation of methane plays a central role in Arctic carbon cycling, potentially reducing methane emissions from lakes, though little is known about methane cycling in the water column of Arctic lakes. We previously detected surprisingly large enrichments of heavy carbon and hydrogen isotopes of methane in three small lakes in Greenland suggesting unusually efficient methanotrophic communities in these Arctic lakes. Using stable isotope and 16S rRNA gene sequencing we determined carbon and hydrogen isotopes and microbial community composition down the water column of Teardrop lake, under open-water conditions. We found that isotopic values of methane in Teardrop lake were again highly enriched 13C and 2H at 4 m depth with −13.2‰ and −27.1‰ values for carbon and hydrogen isotopes, respectively. Methane concentrations slightly increased at the depth interval with isotope enrichment, not typical of classic methanotrophy. Consistent with isotopic enrichment of the heavy isotopes we detected the highest relative abundance of putative methanotrophs, in particular Methylovulum at 4 m. The highest relative abundance of putative methanogens was detected at 3 m as well as at 5 m. At the same depth interval, temperature and oxidation reduction potential also increase, supporting increased microbial activity within the water column. Based on geochemical and microbial observations, we suggest that the methane cycling in Teardrop lake is decoupled from a traditional depth dependent model where the dominant source of methane is in the anoxic sediments. Instead, methane in the water column is likely from a combination of anoxic sediment, littoral transport and oxic methanogenesis in the mid-water column, and recycling of carbon within the water column is leading to extreme isotope enrichments. Thus, understanding linkages between depth-dependent microbial dynamics and methane biogeochemistry are necessary to constrain the sensitivity of the methane cycle to future climate change.
1 Introduction
Lakes are currently responsible for 6%–16% of total natural emissions of methane (Bastviken et al., 2004; Rosentreter et al., 2021), an important greenhouse gas, with about 25% greater radiative forcing than CO2 on a per molecule basis over a period of 100 years (Whalen, 2005; Etminan et al., 2016). As the climate continues to warm, methane production and emissions from freshwater environments are expected to increase (Tan and Zhuang, 2015). In the Arctic, small lakes (< 10 km2) represent approximately half of the total area of surface water environments (Abnizova et al., 2012) and emit more methane per unit area than larger lakes (Downing, 2010). Microbial production of methane by methanogens is dependent upon anoxia, temperature and the amount and quality of organic substrates (Liikanen et al., 2003; Kankaala et al., 2006; Duc et al., 2010; Borrel et al., 2011). Microbial oxidation of methane plays a central role in carbon cycling, potentially reducing emissions of methane from lakes where warming climate has increased biogenic methane production. In northern lakes, up to 88% of the methane produced is oxidized by methanotrophs (Lopez Bellido et al., 2009), lowering concentrations of methane in surface waters, and thereby attenuating the positive feedback potential from biogenic methane.
In lakes, methane is primarily produced in anoxic sediments by methanogenic archaea. A depth-dependent model for methane cycling assumes methane is predominantly produced within anoxic sediments and is exported into the water column by ebullition or diffusion. However, recent studies suggest that methane is also produced in oxic conditions leading to an oversaturation in oxic waters (Keppler et al., 2006; Tang et al., 2016; Günthel et al., 2019; Bizic et al., 2020). For example, in Lake Cromwell, oxic methane production accounted for 20% of methane emissions (Bogard et al., 2014). Additionally, methane has the potential to be introduced into the lake water column by lateral transport from peripheral water bodies, littoral sediments and in situ biological production in addition to profundal sediments (Günthel et al., 2019). Therefore, it is possible then to have cyclic production and consumption of methane happening simultaneously within the water column.
The fraction of in-lake methane production that is oxidized before emission varies greatly from negligible to nearly complete. Methane oxidation rates in aquatic systems are likely dependent on a suite of environmental variables, including methane concentration, dissolved O2 concentration, water temperature and light. Incubation experiments suggest that methane concentration is often a major factor affecting methane oxidation rate (Loften et al., 2014; Shelley et al., 2014). However, methanotrophs can also adapt to low methane concentrations (Mau et al., 2013). In many lakes, the highest methanotrophic activity is associated with depths where dissolved O2 concentrations are very low, suggesting partial inhibition of methanotrophic activity at high O2 concentrations (Kankaala et al., 2013; Blees et al., 2014; Martinez-Cruz et al., 2015). Several studies have shown no effect of temperature on methane oxidation rate; however, others suggest that methane oxidation is temperature dependent (Duc et al., 2010; Shelley et al., 2014). Additionally, light has been suggested to inhibit methane oxidation in the euphotic zone (Murase and Sugimoto, 2005), potentially leading to increased methane emissions. Recent studies found a decline of methane emissions with increasing dissolved organic carbon concentration and lake color, potentially the result of increased methanotrophic activity under low light (Rasilo et al., 2015). However, in shallow lakes with light penetration below the oxycline, increased methane oxidation can occur due to coupling with in situ production of oxygen by photosynthesis, known as cryptic photosynthetic oxygen production (Milucka et al., 2015; Oswald et al., 2015, 2016; Brand et al., 2016). The localized photosynthetic oxygen production might in turn support methanotrophy that suppresses methane emissions.
In freshwater environments, methane oxidation is carried out by type I and type II methanotrophs, primarily by aerobic methane oxidizing bacteria, such as Methylobacter, Methylosoma, Methylovulum, Methylosoma, Methylosarcina and Methylomicrobium (He et al., 2012; Oswald et al., 2017). Methanotrophs have been observed throughout the water column and sediments and their abundance is influenced by methane concentration, temperature, depth, dissolved oxygen, dissolved phosphate concentration and season. Abundance of methanotrophs generally increases with depth and relative abundance is higher in the winter compared to the summer (Samad and Bertilsson, 2017). While microbial communities in aquatic polar environments are receiving increased interest (Comeau et al., 2012; Stoeva et al., 2014; Vick-Majors et al., 2014; Osudar et al., 2016; Ruuskaanen et al., 2018; Bomberg et al., 2019; Butler et al., 2019; Vigneron et al., 2019; Møller et al., 2020; Somers et al., 2020; Emerson et al., 2021), most studies focusing on the role of microbial communities in methane cycling are based on lake sediments (He et al., 2012, 2022; Martinez-Cruz et al., 2017; Colby et al., 2020; Møller et al., 2020; Zandt et al., 2020). For example, carbon acquisition in lake sediments is driven predominantly by Methylobacter, Methylosoma, Methylosoma (He et al., 2012), and Methylobacter has been shown to acquire carbon in anaerobic sediments (Martinez-Cruz et al., 2017). Microbial methane cycling in the water column is less well understood.
Isotopic signatures of methane are useful indicators of microbial methane processes, including microbial methane oxidation. During anoxic biogenic methanogenesis a kinetic isotope effect yields methane that is depleted in 13C and 2H relative to precursor substrates (Whiticar et al., 1986; Balabane et al., 1987; Valentine et al., 2004). However, oxic methane production, such as methyl-phosphonate demethylation, has been shown to have minimal fractionation (Taenzer et al., 2020), whereas isotopic fractionation from other oxic methane production pathways have not been reported (Bizic et al., 2020; Wang et al., 2021). Methanotrophy exhibits a kinetic isotope effect that leaves the residual methane pool isotopically enriched in 13C and 2H (Coleman et al., 1981; Happell et al., 1994; Templeton et al., 2006; Kinnaman et al., 2007). The isotope discrimination during oxidation can yield residual methane pools that are enriched by < 1‰–30‰ for δ13CCH4 and 3‰–200‰ for δ2HCH4 (Coleman et al., 1981; Happela et al., 1994; Bastviken et al., 2002).
Isotopic measurements of C and H of dissolved methane in small Greenlandic lakes under both ice-free and ice-covered conditions from 2013–2014 revealed surprisingly large ranges for both δ13C and δ2H. Under open-water conditions, δ13C ranged from −68.7‰ to +7.4‰ and δ2H from −370‰ to +250‰, while under ice covered conditions, δ13C ranged from −72.2‰ to −33.2‰ and δ2H from -388‰ to -29‰. The most enriched 13C and 2H isotopic values were observed in a single lake, Teardrop lake, under open-water conditions, coinciding with a metalimnetic oxygen minimum at 4 m depth in summer 2013. The enrichment in 13C and 2H was associated with low methane concentrations, as is characteristic with consumption of methane by microbial oxidation of methane (Coleman et al., 1981; Happell et al., 1994; Templeton et al., 2006; Kinnaman et al., 2007). Isotope excursions have been demonstrated in other Arctic lakes at mid-water column depths, with carbon isotope values as high as +1.4‰ reported in shallow Canadian lakes (Thottathil et al., 2018, 2019). Similarly, in these lakes, the most enriched carbon isotope values of methane were associated with low methane concentrations near the oxycline, supporting the potential of highly efficient methanotrophic communities at intermediate water depth.
In order to study water column methane oxidation in greater detail, we returned to Teardrop lake in summer 2015 and expanded the scope of the investigation to include more detailed water column chemistry, stable isotopic signatures of dissolved methane, and a characterization of the microbial communities with depth. We have had success in identifying spatial agreement between methane, sulfur and nitrogen gradients and changes in the microbial community using amplicon-based sequencing targeting the 16S rRNA genes and aqueous geochemistry in these lakes (Schütte et al., 2016). Our objectives were to answer the following questions: 1) is extreme isotopic enrichment 13C and 2H of dissolved methane observed in subsequent years, or was the phenomenon in 2013 a unique occurrence? And 2) if enrichment in 13C and 2H is observed, what microbial communities are present? Could microbial community composition explain the isotope enrichment in 13C and 2H of dissolved methane? Climate change has the potential to increase methane emissions from freshwater systems, which would in turn induce further climate change, resulting in a positive climate feedback. Understanding linkages between microbial communities and methane biogeochemistry are necessary to constrain the sensitivity of the methane cycle to future climate predictions.
2 Materials and methods
2.1 Site description
The ice-free margin of southwest Greenland is characterized by a low continental climate and is strongly influenced by the high-pressure system over the Greenland Ice Sheet (Bennike, 2000). In Kangerlussuaq, the average annual temperature is −5°C from 2008 to present, the maximum temperature measured was 15.4°C in June of 2018, and the minimum temperature measured was −44.9°C in February of 2015. The average rainfall is 243 mm. Teardrop lake (informal name) is located ∼5 km from the terminal moraine of the Russell Glacier (Figure 1). It is a relatively small lake, with a surface area of 0.97 ha, volume of 34,000 m3 and maximum depth of 5.25 m. Teardrop is a moderately brackish, mesotrophic lake, with mean conductivity of 2,740 μS cm−1and mean dissolved organic carbon of 97.6 mg L−1 (Cadieux et al., 2016). Under open-water conditions in 2013, the pH was alkaline and buffered, ranging from 8.98 to 9.3. Currently, Teardrop lake is a hydrologically closed basin, with no direct inflow or outflow channel. Due to continuous permafrost, groundwater seepage is assumed to be limited. While many lakes in the Kangerlussuaq region are cold monomictic (Anderson et al., 2001), Teardrop lake is a dimictic lake, exhibiting ice cover from late October to early June (Cadieux et al., 2016).
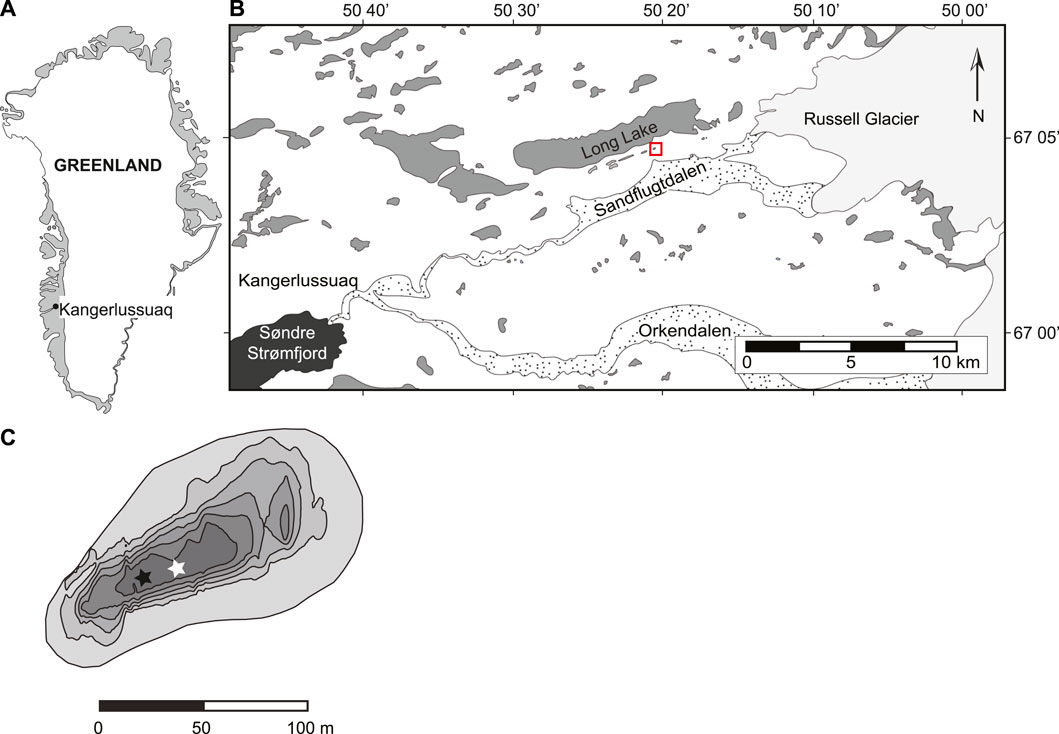
FIGURE 1. Figure 1: Maps of (A) Greenland showing Kangerlussuaq; (B) zoom in of Kangerlussuaq region. Red box highlights Teardrop lake; (C) Teardrop lake bathymetry (contour interval = 1 m) showing sample locations. White star shows East sampling location (discussed in paper); black star shows West sampling location. Modifed from Cadieux et al., 2016.
2.2 Sampling and in situ analysis
Water samples and measurements were taken at two depth profiles located in the deepest basin of Teardrop lake (Figure 1C) in August of 2015. Profiles were similar between the two locations, noted East and West (Supplementary Figures S1,S2). Herein, we will present findings from the East sampling station located in the center of the basin at the maximum depth point. Dissolved oxygen (D. O., mg L−1), pH, specific conductivity (μS cm−1) and oxidation reduction potential (ORP, mV) were measured using a YSI 6039 Data Sonde (Yellow Springs Inc., Yellow Springs, OH, United States) deployed at 0.5 m depth intervals down to maximum depth. Photosynthetically active radiation (PAR) was measured using a Li-Cor LI193 Spherical Quantum Sensor. Water samples were taken with a submersible pump at 0.5 m–1 m intervals throughout the water column at each location, with the exception for methane, where only one sample was obtained from the well-mixed epilimnion and the remainder of the water column was sampled at 0.5 m intervals.
For methane, 1 L of water at each interval was immediately stripped in the field using a headspace-equilibrium technique to extract methane gas from water (Westendorp, 1985). Headspace gas was displaced into a Cali-5-Bond bag using surficial water (Cadieux et al., 2016, 2017). For chlorophyll, 250 ml of water was filtered onto a 0.7 nm glass filter, and the filter was transferred to an amber bottle and remained frozen until analysis. Additionally, 1 L of water was collected into sterile HDPE Nalgene bottles and filtered through 0.22 μm Isopore Membrane Filters (Millipore SiGMa, Burlington, MA) for microbial analysis. Filters were frozen until analysis.
2.3 Chemical analysis
Chlorophyll-a concentrations were determined using acetone pigment extraction in aqueous acetone solution and spectrophotometric determination of chlorophyll a with pheophytin correction at Indiana University (APHA, 2012). Concentrations of dissolved aqueous methane were measured on a Gas Chromatography Flame Ionization Detector (GC-FID) at Indiana University. Stable C and H isotopes of methane were analyzed using continuous-flow gas chromatography-oxidation/pyrolysis isotope ratio mass spectrometry (GC-ox/pyr-IRMS) with a laboratory-built gas pre-concentrator interfaced with a ThermoFinnigan GCC and Delta Plus XP IRMS at the Stable Isotope Research Facility (SIRF) at Indiana University in Bloomington Indiana (Cadieux et al., 2016). Carbon isotope values are referenced to Vienna Pee Dee Belemnite standard (VPDB) and hydrogen isotope values are referenced to Vienna Standard Mean Ocean Water (VSMOW) by comparison with pure methane where the isotopic composition has been measured by off-line combustion. Instrument uncertainty of δ13C and δ2H from the GC-C-IRMS was calculated as 0.4‰ and 10‰ respectively, which is one standard deviation for repeated measurements of the gas standards analyzed during sample runs.
2.4 Microbial community composition
Genomic DNA was extracted using the PowerWater DNA isolation kit (MOBIO laboratories, Carlsbard, CA). Amplification of the 16S rRNA genes was performed using the forward primer 515f and a barcoded reverse primer 806r (Caporaso et al., 2012, http://www.earthmicrobiome.org). Each reaction was 25 μl in volume with 5 μl 5′ HF Buffer (New England BioLabs), 1.5 μl of 50 mM MgCl2, 0.5 ml 10 mM dNTPs, 0.5 μl of each 10 mM primer (Integrated DNA Technologies, Coraville, IA) 0.25 μl of 2,000 U/ml Phusion DNA Polymerase (New England Biolabs), 10 ng–20 ng of DNA, and 15.75 μl PCR water. Amplification was performed using an initial incubation step at 94°C for 3 min followed by 35 cycles of 94°C for 45 s, 50°C for 1 min, 72°C for 1.5 min and a final extension step at 72°C for 10 min. PCR amplicons were cleaned using the PCR purification kit (QIAgen, Valencia, CA). Amplicons were pooled in an equimolar mixture and the pool was cleaned an additional time using the Agencourt AMPure XP (Beckman Coulter, Brea, CA). Sequences were generated on the MiSeq Illumina platform using MiSeq Reagent Kit v2 (300 cycles, PE 300 bp, Illumina Inc., San Diego, CA) and custom-made sequencing primers (http://www.earthmicrobiome.org).
After quality control amplicon sequence variants (ASVs) were generated using the DADA2 (Callahan et al., 2016) subcommand from within QIIME2 (Bolyen et al., 2019) release 2018.2 with the parameters “--p-trunc-len-f 200- --p-trunc-len-r 95”. A portion of the Mothur MiSeq SOP (Kozich et al., 2013) was then followed to align reads to the RDP training set v.9 and remove reads identified as anything other than bacteria or archaea. Remaining reads were imported back into QIIME2 and chimeras were removed using the “vsearch uchime-denovo” subcommand. ASVs were classified using the “classify-sklearn” command in QIIME2 against release 138 of the Silva SSU database (Quast et al., 2013) and a rooted tree was generated using the phylogeny subpackage with default parameters. Shannon entropy and Faith pd diversity measures were generated within QIIME 2. We used these sequence data to assess changes in microbial community composition based on differences in relative abundances with depth in Teardrop lake. Recent studies have pointed out that using differences in relative abundances without determining changes in total abundance through e.g., qPCR can be misleading when inferring changes in microbial community composition in the environment (Aitchison 1982; Gloor et al., 2017; Knight et al., 2018; Morton et al., 2019). An increase in the relative abundance of e.g., methanotrophs can be associated with low absolute numbers of methanotrophs at a particular location suggesting that the relevance of this function may still be limited. Furthermore, changes in the relative abundance of one taxa results in compensatory changes in at least one other taxa as the relative abundances of the microbial taxa are not independent from one another (Aitchison 1982; Gloor et al., 2017). Many different underlying scenarios for changes in the microbial community lead to the same relative abundances of taxa (Morton et al., 2019). However, in this study we assessed the changes in microbial community composition in combination with changes in multiple geochemical parameters. This approach has allowed us to investigate linkages between inferred microbial functional groups and corresponding geochemical processes.
Phytoplankton samples were concentrated via sedimentation and settling using an Utermoehl settling chamber. Taxa present were identified, and plankton enumeration was done using a nanoplankton counting chamber at 200x magnification ensuring a minimum of 200 organisms were counted for each sample depth. Colony forming phytoplankton were enumerated and individual cells were counted from a minimum of 10 organisms to generate an average cell count. This multiplication factor was used to generate cells per milliliter (APHA, 2012).
3 Results
At the time of sampling in August 2015, the temperature in the lake ranged from 14.1°C at the surface to 10.2°C at the bottom, with a slight increase of 0.6°C from 3.5 m–4.0 m depth (Figure 2A). The lake was well-mixed from surface to 3.5 m depth, with uniform pH, specific conductivity, D. O and ORP. At 3.5 m depth, pH decreased from 9.3 to 8.7 and D.O. decreased from 12.6 mg L−1 to 0.6 mg L−1 (115%–5.3%). Specific conductivity increased with depth from 1,545 μS cm−1 to 2,318 μS cm−1. ORP also increased from 121.1 mV to 136.2 mV at 4.5 m, but decreased to 120.7 mV at the sediment water interface (Figure 2B). Light penetrated through the entire water column, with 1.3% of PAR surface irradiance remaining at the sediment water interface (Figure 3C). Chlorophyll concentrations were highest at the sediment water interface, and varied throughout the water column, ranging from 0.74 μg/L to 3.9 μg/L.
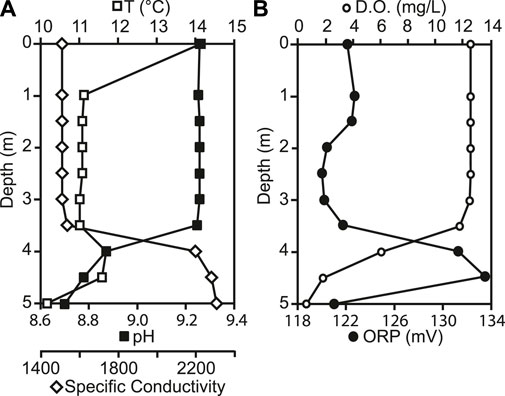
FIGURE 2. (A) Changes in temperature (T), pH, specific conductivity, (B) dissolved oxygen (D. O.), and Redox Potential (ORP) down the water column.
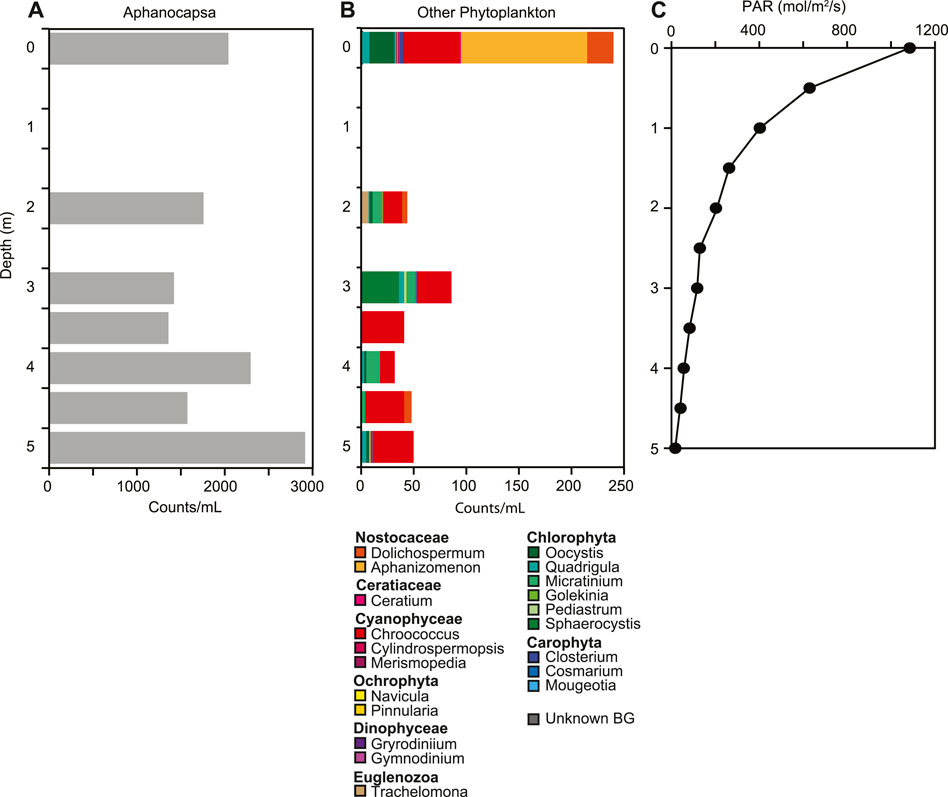
FIGURE 3. Changes in phytoplankton (A,B) and (C) photosynthetically active radiation (PAR) down the water column. Aphanocapsa was the most dominant phytoplankton. Phytoplankton were determined by visual analysis and counting.
The overall microbial community composition changed throughout the water column with two distinct changes, one at 3 m and one below 4.5 m (Figure 4). Both Shannon diversity and Faith pd indicated highest diversity of the microbial community at 5 m; microbial diversity and community composition were similar at the West and East depth profiles (Supplementary Figures S3-S5). These shifts in community composition are consistent with changes in ORP and dissolved oxygen (Figure 2B). The community composition in the upper water column showed higher relative abundances of Luteolibacter, Polynucleobacter, Burkholderiaceae, Candidatus Limnoluna, and hgcl clade (family Sporichthyaceae) among others (Figure 4). At 3 m GKS98 freshwater group, family Alcaligenaceae increased in relative abundance and taxa such as NS11-12 marine group (order Sphingobacteriales), Pseudarcicella, Flavobacterium, and Algoriphagus decreased in relative abundance. Below 3 m besides an increase in the relative abundance of Pedobacter and a decrease in the relative abundance of Polynucleobacter the community composition was surprisingly similar to the composition in the upper water column. The most distinct change in microbial community composition occurred below 4.5 m with a sharp decrease in the number of taxa with a relative abundance of over 1% and a decrease in the relative abundance of Luteolibacter, Pedobacter, NS11-12 marine group, Pseudarcicella, and Sporichthyaceae. In contrast the relative abundance of Hgcl clade increased. The phytoplankton population was dominated by Cyanobacteria, in particular Aphanocapsa, with concentrations increasing from 1.4 × 104 cells/ml at 3.5 m to 2.9 × 104 cells/ml at 5.0 m (Figure 3A,B). Other cyanobacteria exhibited lower concentrations ranging from 50 cells/ml to just below 250 cells/ml. The most abundant other cyanobacteria included Dolichospermum, Aphanizomenon, and Chroococcus at the surface, with Chroococcus being the most abundant throughout the water column. The second most abundant group of phytoplankton was Chlorophyta with Oocystis the most abundant at the surface. Sphaerocystis showed an increase in abundance at 3 m and the Micratinium increased from 2 m–4 m.
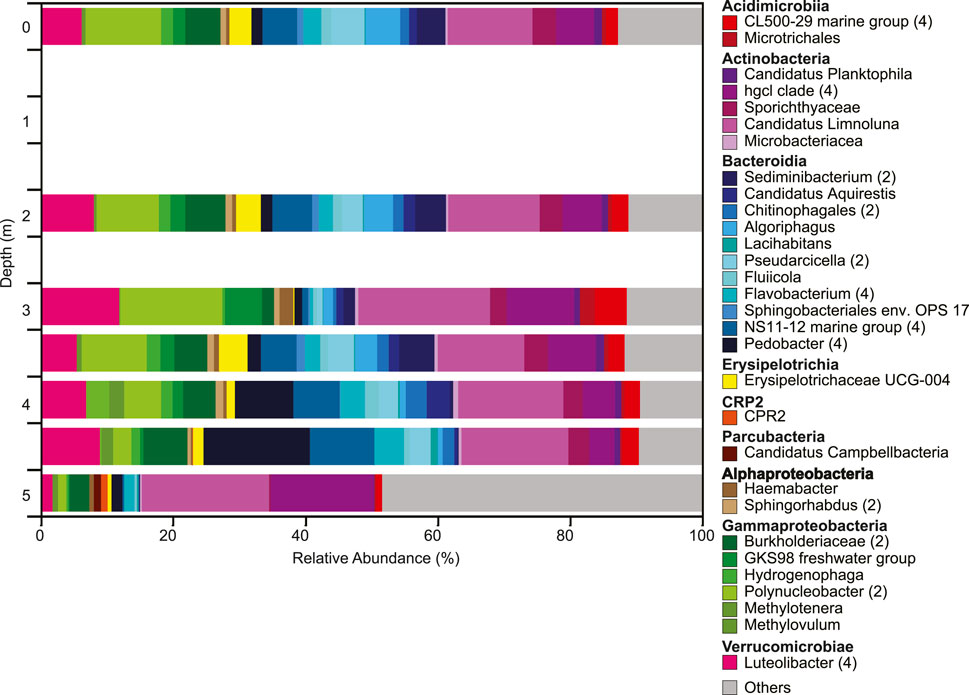
FIGURE 4. Depth profile of microbial community composition down the water column based on changes in the relative abundances of 16S rRNA gene sequences. All ASVs with a relative abundance > 1% are shown. ASVs with identical identification were combined into the same taxonomic group. ASVs with a relative abundance < 1% are combined in the category ‘others’. The number of ASVs included in each taxonomic group is included with the number given after the taxonomic name. If no number is given, the group only contains one ASV.
Methane concentrations were greatest at the sediment-water interface (27.2 μM) and decreased up the water column to 4.0 μM (Figure 5C). A slight increase in concentration occurred from 4.5 m to 3.5 m. Corresponding with the increase in methane concentration at 4.0 m is an isotope enrichment for both δ13C (−13.2‰) and δ2H (−101‰). Carbon and hydrogen isotope values of methane are statistically significantly related throughout the water column (Figure 5C). The putative methanotrophs we detected included Methylovulum, Methylobacter, Crenothrix, and Methylomonaceae (Figure 5A; Supplementary Figure S6). Consistent with an increased isotope enrichment of δ13C at 4 m we detected the highest relative abundance of putative methanotrophs (0.038). The combined geochemical evidence of isotope enrichment and redox change supports the increase in the relative abundances of putative methanotrophs and methanotrophy. This lessens the concern of solely using relative abundances to assess changes in microbial community composition (Aitchison 1982; Gloor et al., 2017; Knight et al., 2018; Morton et al., 2019). Differences in methanotrophs with depth was in particular due to changes in the relative abundance of Methylovulum, making up 90% of all the putative methanotrophs detected at 4 m. Below 4 m the overall relative abundance of putative methanotrophs decreased to below 1%, but the relative abundance of Methylobacter and Crenothrix increased. The overall relative abundance of putative methanogens ranged from 0.07%–0.22% across the water column (Figure 5B, Supplementary Figure S7). Methanobacterium, Methanosaeta, and Methanoregula made up among others the community of putative methanogens throughout the water column. Surprisingly, the highest relative abundance of putative methanogens was detected at 3 m and also at 5 m with Methanobacterium making up most of the relative abundance of putative methanogens at 3 m and Methanobacterium, Methanosaeta, Methanospirillum, Methanoregula, and Methanolinea being the putative methanogens with the highest relative abundance.
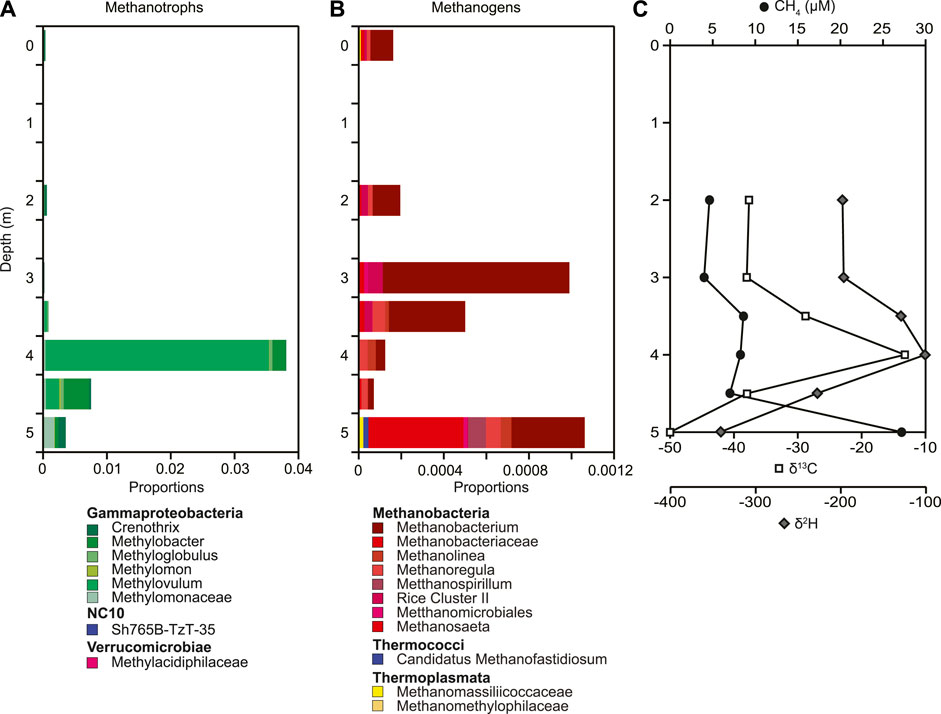
FIGURE 5. Depth profiles of (A) putative methanogen relative abundances, (B) putative methanotroph relative abundances based on 16S rRNA gene sequences, as well as (C) concentration and stable C and H isotope composition of dissolved methane down the water column. The number of ASVs included in each taxonomic group is included with the number given after the taxonomic name. If no number is given, the group only contains one ASV.
Nitrite is the most abundant nitrogen species throughout the water column, with median concentration 295.5 μM, 20 times greater than nitrate concentrations (median 14.5 μM, Figure 6D). Nitrogen concentrations were overall uniform throughout the water column, with a slight increase in nitrite from 3.5 m to 4.0 m depth, and slight decrease in both nitrate and ammonia from 3.5 m to 4.0 m depth. Putative denitrifiers overall had the highest relative abundances (0.4–0.5, Figure 6B) followed by putative dissimilatory nitrate reducers ranging in relative abundance between < 0.001 and 0.009 at 5 m (Figure 6C, Supplementary Figure S9) and putative ammonia oxidizers ranging from < 0.001 to 0.001 (Figure 6A, Supplementary Figure S10). Consistent with the uniform concentrations of nitrate throughout the water column, the relative abundances of putative denitrifiers did not change with depth (Figure 6B, Supplementary Figure S9). In contrast, putative ammonia oxidizers showed the highest relative abundances at 3.5 m and 4.5 m (Figure 6A). The decrease in the relative abundances of putative ammonia oxidizers and dissimilatory nitrate reducers (DNRA) at 4.0 m corresponds to the shift in nitrogen concentration at that depth (Figure 6C, Supplementary Figure S11). The geochemical evidence supports changes in microbial functional groups involved in nitrogen cycling throughout the water column in Teardrop lake. This lessens the concern of solely using relative abundances to assess changes in the microbial community composition (Aitchison 1982; Gloor et al., 2017; Knight et al., 2018; Morton et al., 2019). We did detect very low relative abundances of Candidatus Anammoximicrobium up to 3.5 m and again at 5.0 m indicating that the potential for anaerobic ammonia oxidation exists in Teardrop lake.
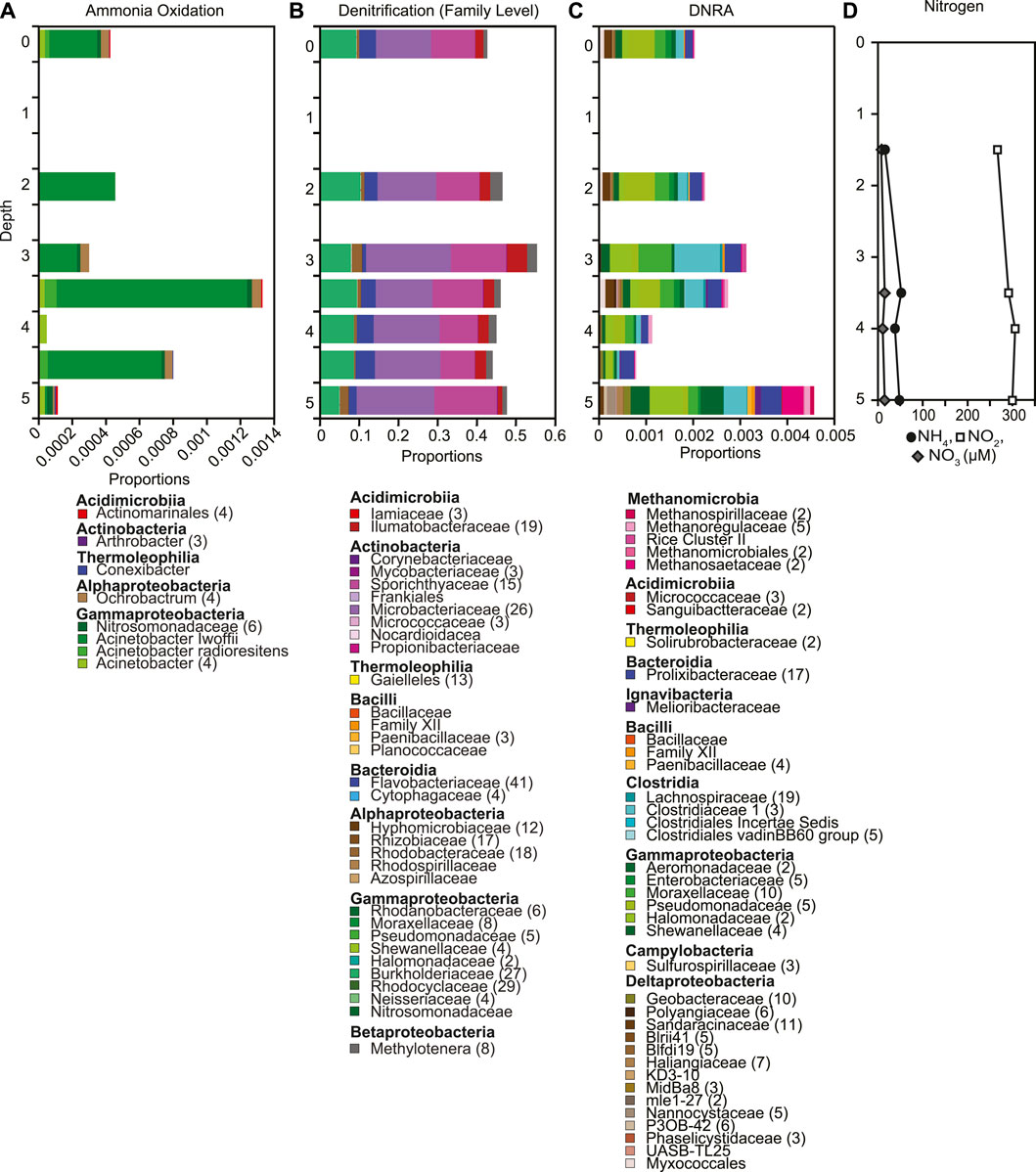
FIGURE 6. Depth profiles of putative (A) ammonia oxidizers, (B) denitrifiers, (C) DNRA based on 16S rRNA gene sequences, as well as (D) concentrations of nitrate, nitrite and ammonia down the water column. (A–C). The number of ASVs included in each taxonomic group is included with the number given after the taxonomic name. If no number is given, the group only contains one ASV.
4 Discussion
4.1 Geochemical, isotopic and genomic evidence of traditional methane cycling
The traditional conceptual model of methane cycling in northern lakes involves methane production in anoxic sediments that is transported vertically up the water column by ebullition and diffusion, with oxidative removal along the transport path that generates a concentration profile of dissolved methane that decreases up the water column (Wik et al., 2016; Thottathil and Prairie, 2021). Our results for dissolved methane in Teardrop lake exhibited this general pattern - methane concentrations decreased from a maximum just above the sediment/water interface to a minimum in the upper water column. Oxidative removal of methane along the path was also likely. Dissolved oxygen was clinograde, reaching hypoxic conditions at the sediment-water interface. Oxidation of methane by aerobic methanotrophic bacteria was likely occurring throughout the water column as evidenced by the inverse relationship between dissolved oxygen and dissolved methane concentrations throughout, the change in methane isotopic signature, and the presence of methanotrophs throughout the water column (Figure 5). Dissolved oxygen concentrations were sufficient to support methanotrophy throughout the water column which is consistent with an increase in relative abundance of putative methanotrophs we observed below 3.5 m (Figure 5). Additionally, a strong positive correlation was observed between δ13C and δ2H of dissolved methane (n = 12, R = 0.93, p < 0.001; Supplementary Figure S12), characteristic of residual methane following aerobic methanotrophic activity (Whiticar and Faber, 1985; Happell et al., 1994; Whiticar, 1999; Bastviken et al., 2002; Alstad and Whiticar, 2011; Wik et al., 2020). The average slope of enrichment of 13C and 2H of dissolved methane, calculated as δ2H/δ13C (5.2) is similar to the slope of projected oxidation predicted by previous experimental studies, which range from 5.9 to 13 (Coleman and Risatti, 1981; Whiticar, 1999; Kinnaman et al., 2007). These results are consistent with previous findings from Teardrop lake, other Greenlandic lakes in this area (Cadieux et al., 2016), and other Arctic freshwater environments (Wik et al., 2020).
Putative methanotrophic communities were present throughout the water column of Teardrop lake and were similar to other lacustrine systems. Gammaproteobacteria, the dominant putative methanotrophs observed here, were also the most abundant taxa in high oxidation zones in several other lacustrine systems (Carini et al., 2005; Blees et al., 2014; Oswald et al., 2015, 2016; Zigah et al., 2015). In neighboring Potentilla lake, where microbial communities were collected under ice in late winter, we detected high relative abundances of Gammaproteobacteria known to be type I methanotrophs, including Crenothrix and Methylobacter, which dominated between 4 m and 5 m depth (Schütte et al., 2016). In Teardrop lake, Methylovulum was the dominant type I methanotroph, and exhibited the highest relative abundance at 4 m. Beijerinckiaceae (Alphaproteobacteria) including Methylobacterium were present in low relative abundance in Teardrop lake. However, Tamas et al. (2014) reported that members of Beijerinckiaceae lost their capabilities of methanotrophy and are not methanotrophs. Others report that Methylobacterium is methylotrophic and not methanotrophic (Dedysh et al., 2004; Theisen and Murrell, 2005). Most other studies of Arctic lakes described methanotroph community composition mainly in lake sediments rather than from the water column (He et al., 2012, 2022). Consistent with our results, He et al. (2012) found the methanotrophic community in an Arctic lake in Alaska to be dominated by members of the Gammaproteobacteria. In contrast, studies from boreal lake systems found that Gammaproteobacteria, in particular Candidatus Methylumidiphilus within the order Methylococcales, had high relative abundances throughout the lake, but Alphaproteobacteria known to be type II methanotrophs had high relative abundance in surface water where methane concentrations were comparatively low (0 μM–1 μM) (Martin et al., 2021). In comparison, methane concentrations in Teardrop lake were elevated throughout the water column (2 μM–27 μM), consistent with the ubiquitous presence of type I methanotrophs, which have been suggested to have lower methane affinity compared to type II methanotrophs (Martin et al., 2021).
4.2 Decoupled methane cycle at intermediate depth
Coexisting with a traditional methane cycle functioning throughout the water column, we found evidence of methane cycling centered between 3 and 5 m depth that may be decoupled from the full water column cycle. Extreme isotope enrichment of 13C and 2H of dissolved methane centered at 4 m depth may result from methanogenesis coupled tightly to methanotrophy in what approaches closed-system characteristics. Microbial oxidation of methane under these conditions leads to a systematic enrichment in both 13C and 2H of the residual un-oxidized methane pool (Whiticar and Faber, 1985; Happell et al., 1994; Whiticar, 1999; Bastviken et al., 2002; Alstad and Whiticar, 2011; Wik et al., 2020). At the time of sampling in August 2015, dissolved methane in Teardrop lake at 3 m–4 m depth was isotopically enriched by as much as 36‰ for 13C and 279‰ for 2H relative to bottom waters at 5 m depth. The lake was weakly thermally stratified and likely approaching holomixis. However, a slight increase in temperature was observed at 4 m depth, potentially supporting increased microbial processes leading to a thermal discontinuity or temporary biogenic meromixis. Oxidation-reduction potential was also elevated within this zone by 15 mV. A subsurface maximum in the isotopic enrichment of 13C and 2H in dissolved methane is an unusual pattern relative to other local lakes that we first observed in Teardrop lake in 2013 (Cadieux et al., 2016). However, this pattern has recently been reported in a German lake (Lake Stechlin; Hartmann et al., 2018), as well as group of northern freshwater lakes in the Canadian Shield (i.e., Morency Lake, Croche Lake, Cromwell Lake, Gelil Lake), with δ13C mid-depth values as high as +1.7‰ and is attributed to localized elevated methanotrophic activity (Thottathil et al., 2019).
Photosynthesis in the euphotic zone of lakes is known to fuel aerobic methane oxidation as the addition of dissolved oxygen can stimulate methane oxidation and rates of oxygen production can be of the same magnitude as methane oxidation (Oswald et al., 2015). In Teardrop lake, the entire water column was euphotic, with photosynthesis likely occurring at all depths, including 3.5 m–4.5 m depth. Additionally, we observed concentrated layers of potential microbial growth suspended throughout the lake at mid-depths, supported by increased phytoplankton counts at 3 m (Figure 3). These suspended microbial communities could contribute to enhanced oxygenic photosynthesis. At the major methane isotope excursion at 4 m, there was no observable increase in dissolved oxygen, as oxygen is saturated and not a limiting factor. However, there was a 10% increase in ORP to +134 mV between 4.5 m and 3.5 m confirming sufficient concentration of dissolved oxygen to meet terminal electron acceptor demand from aerobic methanotrophy.
Corresponding with the extreme isotope enrichment in 13C and 2H of methane at 4 m was an increase in the relative abundance of putative methanotrophs. The putative methanotrophic community was dominated by type I methanotrophs belonging to the Gammaproteobacteria. Despite being microaerophilic, incubation studies suggest that Gamma-MOB also have a high tolerance for dissolved oxygen (Reis et al., 2020). Methylovulum had the highest relative abundance at 4 m in Teardrop lake. The change in relative abundances of putative methanotrophs with depth was consistent with changes in putative methylotrophic bacteria. Methylotrophic bacteria such as Methylotenera and Methylobacterium were present throughout the water column, but showed a significant increase at 4 m consistent with the increase in methanotrophs (i.e., relative abundance of 0.002 at 3 m to 0.312 at 4 m for Methylotenera; Supplementary Figure S8). Recent studies have shown that methylotrophs can co-feed with methanotrophs (Krause et al., 2016). The presence of methylotrophs can lead to a change in gene expression and the production of a different enzyme that results in the release of methanol that methylotrophs can then metabolize (Krause et al., 2016). Metagenomic analysis of methanotrophs in Arctic lake sediments showed that methanotrophs also have the capability to produce methanol (He et al., 2022). This suggests that methylotrophs in Teardrop lake have the capability to co-feed with methanotrophs and that the increase in relative abundance of methanotrophs at 4 m may help account for the increase of methylotrophs at that depth.
In Teardrop lake, methane concentrations increased at the interval corresponding with extreme isotope enrichment of 13C and 2H, which is not typical of classic methanotrophy. Production and accumulation of methane in oxic waters, known as the “methane paradox,” has been documented in other lakes, globally (Keppler et al., 2006; Bogard et al., 2014; Tang et al., 2014, 2016; Günthel et al., 2019; Bizic et al., 2020; Bizic 2021; Wang et al., 2021) and could lead to a mid-water-depth increase in methane concentrations. Putative methanogens were identified both above and below the zone of 13C and 2H isotope enrichment of methane at 3 m and 5 m, though relative abundances were overall low. Methanogenesis in oxic water has been demonstrated to be driven by acetoclastic production (Bogard et al., 2014). We only detected two ASVs classified as Methanosaeta known to be capable of acetoclastic production with increased relative abundance at 5 m (Fournier and Gogarten 2008). All other putative methanogens we detected carry out hydrogenotrophic and/or methylotrophic methanogenesis (Vanwonterghem et al., 2016). Isotopic composition of methane above and below the interval of isotopic excursion ranged between −37‰ and −38‰ for δ13C and −100‰ to −227‰ for δ2H, which is closer to the isotopic range from acetoclastic methanogenesis than to that from hydrogenotrophic methanogenesis (Whiticar 1999). Additionally, accumulation of methane in oxygenated freshwater systems, though lower than archaeal methanogenic production rates, has been associated with the presence of phytoplankton including cyanobacteria, which readily convert fixed inorganic carbon into methane under both light and dark conditions (Bizic et al., 2020, 2021; Hartmann et al., 2020; Bizic 2021). Potential pathways include association of archaeal methanogens with cyanobacteria (Berg et al., 2014), demethylation of methylophosphates (Yao et al., 2016) under phosphorus starvation (Bizic et al., 2020), and a connection to the photosynthetic electron transfer chain in cyanobacteria (Bizic et al., 2020, 2021). In Teardrop lake, phytoplankton were present throughout the water column, with highest abundance especially of the cyanobacteria, Anabaena, at the surface, but a marked increase of Aphanocapsa at 4 m and other phytoplankton at 3 m depth. If methanogenesis in the oxic water column is associated with algal activity, the increased abundance of more labile organic matter could potentially promote methanogenesis. Microbial degradation of methylphosphate to methane has been shown to result in minimal fractionation (Taenzer et al., 2020), but isotopic fractionation from other oxic methane production pathways has not been reported (Bizic et al., 2020; Wang et al., 2021). Therefore, we are unable to use isotopic signatures of methane to confirm if oxic methane production is occurring in Teardrop Lake.
In addition to mid-depth methanogenesis, methane in surface intervals may also derive from different source areas than the anoxic sediments of deeper waters. One possibility is lateral transport of methane from littoral sediments, which has been reported in other lakes, and can amount to 30%–40% of methane emitted during the summer stratification (Günthel et al., 2020). Though in lower relative abundance, we detected putative methanogens throughout the water column (Figure 5). Teardrop lake is a hydrologically closed basin, with no inputs from other water bodies. Lateral methane source areas are limited to shallow littoral sediments. Carbon isotopic composition of porewater methane collected from littoral sediments in 2013 ranged from −48.6 to −36.5‰ (Cadieux et al., 2016), within the range of epilimnetic waters in Teardrop lake. The dissolved methane in profundal sediments of Teardrop lake was more depleted in 13C and enriched in 2H relative to the methane in littoral sediments, suggesting different microbial production pathways (Cadieux et al., 2016). Partial oxidation of porewater methane or more isotopically enriched methanogenic substrates in littoral sediments can also contribute to differences in the isotopic composition of dissolved methane between littoral and profundal sediments in small Arctic lakes (Thompson et al., 2018).
Biogeochemical cycles in lakes are often coupled, and driven by underlying microbial community interactions (Wetzel, 2001). In particular, methane and nitrogen cycling are known to be coupled (Bodelier and Steenbergh, 2014; Zhu et al., 2016; Jing et al., 2020). Under anaerobic conditions, methane oxidation occurs using alternative electron acceptors such as nitrate and nitrite instead of oxygen (Valentine, 2002; Oswald et al., 2017). Nitrogen concentrations have been found to both inhibit and stimulate methanotrophic activity and methane oxidation. For example, ammonium-based fertilization has been found to stimulate the growth and activity of methane oxidizers in rice patties (Bodelier et al., 2000; Krüger and Frenzel, 2003), however, ammonium concentrations > 4 mM have also been found to repress methane oxidation in littoral lake sediments (Bosse et al., 1993). Ammonium concentrations in Teardrop lake are 3 orders of magnitude below the threshold for inhibition and unlikely to influence methane oxidation. Methanotrophic bacteria have also been suggested to co-oxidize ammonia when nitrite is present (Harrits and Hanson, 1980). Nitrite concentrations in Teardrop lake exceeded nitrate concentrations, which has also been reported in other local Greenlandic lakes (Cadieux et al., 2016, 2017; Schütte et al., 2016). In Teardrop lake, nitrite concentrations increased slightly at 4 m where extreme isotope enrichment of 13C and 2H was observed associated with a drop in the relative abundance of putative ammonia oxidizers (Figure 6A), but concentrations remained overall stable throughout the water column. Additionally, the presence of nitrate has been suggested to stimulate methane oxidation rates under oxic conditions, with Methylotenera being capable of denitrification (van Grinsven et al., 2020). Relative proportions of putative denitrifiers were overall constant in Teardrop lake, though the putative methylotroph Methylotenera increased at 4 m–2.5% (Supplementary Figure S9). This increase in the relative abundance of Methylotenera was mostly driven by one ASV. Despite not finding clear linkages between methane and nitrogen biogeochemical cycles in Teardrop lake, it is possible that the extreme isotope enrichment of 13C and 2H in dissolved methane resulted from alternative co-cycling of nutrients.
4.3 Implications
Arctic lakes are likely to represent a growing net source of methane to the atmosphere with rapid warming of northern landscapes (Wik et al., 2016; Li and Xue, 2021). Measurements of global atmospheric δ13C-CH4 suggest a rapidly increasing microbial source (Lan et al., 2021) that may account for up to 85% of the recent growth in atmospheric methane (Tollefson, 2022). Identification and inclusion of the key biogeochemical processes in climate models is a significant challenge (Lan et al., 2021). In the last 10 years, the methane paradox has been unfolding in the literature, demonstrating that methane in oxic water is not exclusively from transport from anoxic sources, and oxic methane production may account for 18%–90% of surface emissions (Keppler et al., 2006; Grossart et al., 2011; Bogard et al., 2014; Tang et al., 2014, 2016; Günthel et al., 2019, 2020). We suggest that, in Teardrop lake, methane in the water column may derive from a combination of sources: anoxic sediments, lateral transport from littoral areas and oxic methanogenesis in the mid-water column. Additionally, there is evidence for tight carbon recycling within a limited depth interval of the water column, leading to extreme isotope enrichment in 13C and 2H of the dissolved methane pool. Our work from Teardrop lake highlights the challenge in predicting potential methane emissions from small, shallow water bodies. Most conceptual models of greenhouse gas emissions from northern landscapes utilize large lakes and assume methane emissions from conventional anoxic sources (IPCC 2013; Saunois et al., 2016). Feedbacks involving small lakes have been lacking (Rasilo et al., 2015). As permafrost thaw rapidly expands with warming climate, smaller thermokarst lakes are increasing in number and area in the Arctic (Abnizova et al., 2012; Gao et al., 2013). It is important to integrate methane cycling of small Arctic lakes into the conceptual modeling framework for greenhouse gas emissions from the terrestrial biosphere.
Data availability statement
Data is available through the National Center for Biotechnology Information Sequence Read Archive. BioProject ID is PRJNA879411.
Author contributions
SC, US, and JW contributed to conception and design of the study. JW collected field samples. SC performed chemical and isotopic analyses. US performed microbial analyses. SP performed chlorophyll and plankton analyses. CH performed bioinformatics. SC and US wrote the first draft of the manuscript. SC, US, JW, SP and CH wrote sections of the manuscript. All authors contributed to manuscript revision, read, and approved the submitted version.
Funding
The Funding for this project is from NASA Astrobiology Science and Technology for Exploring Planets (ASTEP) Program #NNX11AJ01G.
Acknowledgments
Robert Whitticar and Lisa Pratt for assistance with collecting field samples.
Conflict of interest
The authors declare that the research was conducted in the absence of any commercial or financial relationships that could be construed as a potential conflict of interest.
Publisher’s note
All claims expressed in this article are solely those of the authors and do not necessarily represent those of their affiliated organizations, or those of the publisher, the editors and the reviewers. Any product that may be evaluated in this article, or claim that may be made by its manufacturer, is not guaranteed or endorsed by the publisher.
Supplementary material
The Supplementary Material for this article can be found online at: https://www.frontiersin.org/articles/10.3389/fenvs.2022.884133/full#supplementary-material.
References
Abnizova, A., Siemens, J., Langer, M., and Boike, J. (2012). Small ponds with major impact: The relevance of ponds and lakes in permafrost landscapes to carbon dioxide emissions. Glob. Biogeochem. Cycles 26, 4237. doi:10.1029/2011GB004237
Aitchison, J. (1982). The statistical analysis of compositional data. J. R. Stat. Soc. Ser. B 44, 139–160. doi:10.1111/j.2517-6161.1982.tb01195.x
Alstad, K. P., and Whiticar, M. J. (2011). Carbon and hydrogen isotope ratio characterization of methane dynamics for Fluxnet Peatland Ecosystems. Org. Geochem. 42 (5), 548–558. doi:10.1016/j.orggeochem.2011.03.004
American Public Health Association (2012). Standard methods for the examination of water and wastewater. 22nd Edition. Washington, D.C: American Public Health Association, American Water Works Association and the Water Environment Federation.
Anderson, N. J., Harriman, R., Ryves, D. B., and Patrick, S. T. (2001). Dominant factors controlling variability in the ionic composition of West Greenland lakes. Arct. Antarct. Alp. Res. 33, 418–425. doi:10.1080/15230430.2001.12003450
Balabane, M., Galimov, E. M., Hermann, M., and Letolle, R. (1987). Hydrogen and carbon isotope fractionation during experimental production of bacterial methane. Org. Geochem. 11, 115–119. doi:10.1016/0146-6380(87)90033-7
Bastviken, D., Cole, J., Pace, M., and Tranvik, L. (2004). Methane emissions from lakes: Dependence of lake characteristics, two regional assessments, and a global estimate. Glob. Biogeochem. Cycles 18. doi:10.1029/2004GB002238
Bastviken, D., Ejlertsson, J., and Tranvik, L. (2002). Measurement of methane oxidation in lakes: A comparison of methods. Environ. Sci. Technol. 36, 3354–3361. doi:10.1021/es010311p
Bennike, O. (2000). Palaeoecological studies of Holocene lake sediments from west Greenland. Palaeogeogr. Palaeoclimatol. Palaeoecol. 155, 285–304. doi:10.1016/s0031-0182(99)00121-2
Berg, A., Lindblad, P., and Svensson, B. H. (2014). Cyanobacteria as a source of hydrogen for methane formation. World J. Microbiol. Biotechnol. 30 (2), 539–545. doi:10.1007/s11274-013-1463-5
Bizic, M., Klintzsch, T., Ionescu, D., Hindiyeh, M. Y., Günthel, M., Muro-Pastor, A. M., et al. (2020). Aquatic and terrestrial cyanobacteria produce methane. Sci. Adv. 6 (3), eaax5343. doi:10.1126/sciadv.aax5343
Bizic, M. (2021). Phytoplankton photosynthesis: An unexplored source of biogenic methane emission from oxic environments. J. Plankton Res. 43 (6), 822–830. doi:10.1093/plankt/fbab069
Blees, J., Niemann, H., Weck, C. B., Zopfi, J., Schubert, C. J., Kirf, M. K., et al. (2014). Micro-aerobic bacterial methane oxidation in the chemocline and anoxic water column of deep south-Alpine Lake Lugano (Switzerland). Limnol. Oceanogr. 59 (2), 311–324. doi:10.4319/lo.2014.59.2.0311
Bodelier, P. L. E., Roslev, P., Henckel, T., and Frenzel, P. (2000). Stimulation by ammonium-based fertilizers of methane oxidation in soil around rice roots. Nature 403, 421–424. doi:10.1038/35000193
Bodelier, P. L. E., and Steenbergh, A. K. (2014). Interactions between methane and the nitrogen cycle in light of climate change. Curr. Opin. Environ. Sustain. 9 (10), 26–36. doi:10.1016/j.cosust.2014.07.004
Bogard, M. J., del Giorgio, P. A., Boutet, L., Chaves, M. C., Prairie, Y. T., Merante, A., et al. (2014). Oxic water column methanogenesis as a major component of aquatic CH4 fluxes. Nat. Commun. 5, 5350. doi:10.1038/ncomms6350
Bolyen, E., Rideout, J. R., Dillon, M. R., Bokulich, N. A., Abnet, C. C., Al-Ghalith, G. A., et al. (2019). Reproducible, interactive, scalable and extensible microbiome data science using QIIME 2. Nat. Biotechnol. 37, 852–857. doi:10.1038/s41587-019-0209-9
Bomberg, M., Claesson, L. L., Lamminmäki, T., and Kontula, A. (2019). Highly diverse aquatic microbial communities separated by permafrost in Greenland show distinct features according to environmental niches. Front. Microbiol. 10, 1583. doi:10.3389/fmicb.2019.01583
Borrel, G., Jezequel, D., Biderre-Petit, C., Morel-Desrosiers, N., Morel, J. P., Peyret, P., et al. (2011). Production and consumption of methane in freshwater lake ecosystems. Res. Microbiol. 162, 832–847. doi:10.1016/j.resmic.2011.06.004
Bosse, U., Frenzel, P., and Conrad, R. (1993). Inhibition of methane oxidation by ammonium in the surface layer of a littoral sediment. FEMS Microbiol. Ecol. 13, 123–134. doi:10.1111/j.1574-6941.1993.tb00058.x
Brand, A., Bruderer, H., Oswald, K., Guggenheim, C., Schubert, C. J., and Wehrli, B. (2016). Oxygenic primary production below the oxycline and its importance for redox dynamics. Aquat. Sci. 78, 727–741. doi:10.1007/s00027-016-0465-4
Butler, T. M., Wilhelm, A. C., Dwyer, A. C., Webb, P. N., Baldwin, A. L., and Techtmann, S. M. (2019). Microbial community dynamics during lake ice freezing. Sci. Rep. 9, 6231. doi:10.1038/s41598-019-42609-9
Cadieux, S. B., White, J. R., and Pratt, L. M. (2017). Exceptional summer warming leads to contrasting outcomes for methane cycling in small Arctic lakes of Greenland. Biogeosciences 14, 559–574. doi:10.5194/bg-14-559-2017
Cadieux, S. B., White, J. R., Sauer, P. E., Peng, Y., Goldman, A. E., and Pratt, L. M. (2016). Large fractionations of C and H isotopes related to methane oxidation in Arctic lakes. Geochimica Cosmochimica Acta 187, 141–155. doi:10.1016/j.gca.2016.05.004
Callahan, B., McMurdie, P., Rosen, M., Han, A. W., Johnson, A. J. A., and Holmes, S. P. (2016). DADA2: High-resolution sample inference from Illumina amplicon data. Nat. Methods 13, 581–583. doi:10.1038/nmeth.3869
Caporaso, J. G., Lauber, L. C., Walters, W. A., Berg-Lyons, D., Huntley, J., Fierer, N., et al. (2012). Ultra-high-throughput microbial community analysis on theIllumina HiSeq and MiSeq platforms. ISME J. 6, 1621–1624. doi:10.1038/ismej.2012.8
Carini, S., Bano, N., LeCleir, G., and Joye, S. B. (2005). Aerobic methane oxidation and methanotroph community composition during seasonal stratification in Mono Lake, California (USA). Environ. Microbiol. (7), 1127–1138. doi:10.1111/j.1462-2920.2005.00786.x
Colby, G. A., Ruuskanen, M. O., St. Pierre, K. A., Louis, St.V. L., Poulain, A. J., and Aris-Brosou, S. (2020). Warming climate is reducing the diversity of dominant microbes in the largest high Arctic lake. Front. Microbiol. 11, 561194. doi:10.3389/fmicb.2020.561194
Coleman, D. D., Risatti, J. B., and Schoell, M. (1981). Fractionation of carbon and hydrogen isotopes by methane-oxidizing bacteria. Geochimica Cosmochimica Acta 45, 1033–1037. doi:10.1016/0016-7037(81)90129-0
Coleman, D. D., Risatti, J. B., and Schoell, M. (1981). Fractionation of carbon and hydrogen isotopes by methane-oxidizing bacteria. Geochimica Cosmochimica Acta 45, 1033–1037. doi:10.1016/0016-7037(81)90129-0
Comeau, A., Harding, T., Galand, P. E., Vincent, W. F., and Lovejoy, C. (2012). Vertical distribution of microbial communities in a perennially stratified Arctic lake with saline, anoxic bottom waters. Sci. Rep. 2, 604. doi:10.1038/srep00604
Dedysh, S. N., Dunfield, P. F., and Trotsenko, Y. A. (2004). Methane utilization by Methylobacterium species: New evidence but still no proof for an old controversy. Int. J. Syst. Evol. Microbiol. 54 (6), 1919–1920. doi:10.1099/ijs.0.63493-0
Downing, J. A. (2010). Emerging global role of small lakes and ponds: Little things mean a lot. Limnetica 29, 9–24. doi:10.23818/limn.29.02
Duc, N. T., Crill, P., and Bastviken, D. (2010). Implications of temperature and sediment characteristics on methane formation and oxidation in lake sediments. Biogeochemistry 100, 185–196. doi:10.1007/s10533-010-9415-8
Emerson, J. B., Varner, R. K., Wik, M., Parks, D. H., Neumann, R. B., Johnson, J. E., et al. (2021). Diverse sediment microbiota shape methane emission temperature sensitivity in Arctic lakes. Nat. Commun. 12, 5815. doi:10.1038/s41467-021-25983-9
Etminan, M., Myhre, G., Highwood, E. J., and Shine, K. P. (2016). Radiative forcing of carbon dioxide, methane and nitrous oxide: A significant revision of the methane radiative forcing. Geophys. Res. Lett. 43 (24), 2614–12623. doi:10.1002/2016gl071930
Fournier, G. P., and Gogarten, J. P. (2008). Evolution of acetoclastic methanogenesis in methanosarcina via horizontal gene transfer from cellulolytic clostridia. J. Bacteriol. 190 (3), 1124–1127. doi:10.1128/JB.01382-07
Gao, X., Schlosser, C. A., Sokolov, A., Anthony, K. W., Zhuang, Q., and Kicklighter, D. (2013). Permafrost degradation and methane: Low risk of biogeochemical climate-warming feedback. Environ. Res. Lett. 8 (3), 035014. doi:10.1088/1748-9326/8/3/035014
Gloor, G. B., Macklaim, J. M., Pawlowsky-Glahn, V., and Egozcue, J. J. (2017). Microbiome datasets are compositional: And this is not optional. Front. Microbiol. 8, 2224. doi:10.3389/fmicb.2017.02224
Grossart, H. P., Frindte, K., Dziallas, C., Eckert, W., and Tang, K. W. (2011). Microbial methane production in oxygenated water column of an oligotrophic lake. Proc. Natl. Acad. Sci. U. S. A. 108, 19657–19661. doi:10.1073/pnas.1110716108
Günthel, M., Donis, D., Kirillin, G., Ionescu, D., Bizic, M., McGinnis, D. F., et al. (2019). Contribution of oxic methane production to surface methane emission in lakes and its global importance. Nat. Commun. 10, 5497. doi:10.1038/s41467-019-13320-0
Günthel, M., Klawonn, I., Woodhouse, J., Bizic, M., Ionescu, D., Ganzert, L., et al. (2020). Photosynthesis-driven methane production in oxic lake water as an important contributor to methane emission. Limnol. Oceanogr. 65, 2853–2865. doi:10.1002/lno.11557
Happell, J. D., Chanton, J. P., and Showers, W. S. (1994). The influence of methane oxidation on the stable isotopic composition of methane emitted from Florida swamp forests. Geochimica Cosmochimica Acta 58, 4377–4388. doi:10.1016/0016-7037(94)90341-7
Harrits, S. M., and Hanson, R. S. (1980). Stratification of aerobic methane-oxidizing organisms in lake mendota, madison, Wisconsin1. Limnol. Oceanogr. 25 (3), 412–421. doi:10.4319/lo.1980.25.3.0412
Hartmann, J. F., Gentz, T., Schiller, A., Greule, M., Grossart, H.-P., Ionescu, D., et al. (2018). A fast and sensitive method for the continuous in situ determination of dissolved methane and its δ13C-isotope ratio in surface waters. Limnol. Oceanogr. Methods 16, 273–285. doi:10.1002/lom3.10244
Hartmann, J. F., Günthel, M., Klintzsch, T., Kirillin, G., Grossart, H.-P., Keppler, F., et al. (2020). High spatiotemporal dynamics of methane production and emission in oxic surface water. Environ. Sci. Technol. 54, 1451–1463. doi:10.1021/acs.est.9b03182
He, R., Wang, J., Pohlman, J. W., Jia, Z., Chu, Y. X., Wooller, M. J., et al. (2022). Metabolic flexibility of aerobic methanotrophs under anoxic conditions in Arctic lake sediments. ISME 16 (1), 78–90. doi:10.1038/s41396-021-01049-y
He, R., Wooller, M. J., Pohlman, J. W., Quensen, J., Tiedje, J. M., and Leigh, M. B. (2012). Shifts in identity and activity of methanotrophs in Arctic lake sediments in response to temperature changes. Appl. Environ. Microbiol. 78 (13), 4715–4723. doi:10.1128/AEM.00853-12
Jing, H., Wang, R., Jiang, Q., Zhang, Y., and Peng, X. (2020). Anaerobic methane oxidation coupled to denitrification is an important potential methane sink in deep-sea cold seeps. Sci. Total Environ. 748, 142459. doi:10.1016/j.scitotenv.2020.142459
Kankaala, P., Huotari, J., Peltomaa, E., Saloranta, T., and Oiala, A. (2006). Methanotrophic activity in relation to methane efflux and total heterotrophic bacterial production in a stratified, humic, boreal lake. Limnol. Oceanogr. 51, 1195–1204. doi:10.4319/lo.2006.51.2.1195
Kankaala, P., Huotari, J., Tulonen, T., and Ojala, A. (2013). Lake-size dependent physical forcing drives carbon dioxide and methane effluxes from lakes in a boreal landscape. Limnol. Oceanogr. 38, 1915–1930. doi:10.4319/lo.2013.58.6.1915
Keppler, F., Hamilton, J. T., Brass, M., and Rockmann, T. (2006). Methane emissions from terrestrial plants under aerobic conditions. Nature 439, 187–191. doi:10.1038/nature04420
Kinnaman, F. S., Valentine, D. L., and Tyler, S. C. (2007). Carbon and hydrogen isotope fractionation associated with the aerobic microbial oxidation of methane, ethane, propane and butane. Geochimica Cosmochimica Acta 71, 271–283. doi:10.1016/j.gca.2006.09.007
Knight, R., Vrbanac, A., Taylor, B. C., Aksenov, A., Callewaert, C., Debeliu, J., et al. (2018). Best practices for analysing microbiomes. Nat. Rev. Microbiol. 16, 410–422. doi:10.1038/s41579-018-0029-9
Kozich, J. J., Westcott, S. L., Baxter, N. T., Highlander, S. K., and Schloss, P. D. (2013). Development of a dual-index sequencing strategy and curation pipeline for analyzing amplicon sequence data on the MiSeq Illumina sequencing platform. Appl. Environ. Microbiol. 79 (17), 5112–5120. doi:10.1128/aem.01043-13
Krause, S. M., Johnson, T., Samadhi Karunaratne, Y., Fu, Y., Beck, D. A., Chistoserdova, L., et al. (2016). Lanthanide-dependent cross-feeding of methane-derived carbon is linked by microbial community interactions. Proc. Natl. Acad. Sci. U. S. A. 114 (2), 358–363. doi:10.1073/pnas.1619871114
Krüger, M., and Frenzel, P. (2003). Effects of N-fertilization on CH4 oxidation and production, and consequences for CH4 emissions from microcosms and rice fields. Glob. Change Biol. 9, 773–784. doi:10.1046/j.1365-2486.2003.00576.x
Lan, X., Basu, S., Schwietzke, S., Bruhwiler, L. M., Dlugokencky, E. J., Michel, S. E., et al. (2021). Improved constraints on global methane emissions and sinks using δ13C‐CH4. Glob. Biogeochem. Cycles 35 (6), e2021GB007000. doi:10.1029/2021gb007000
Li, L., and Xue, B. (2021). Methane emissions from northern lakes under climate change: A review. SN Appl. Sci. 3 (12), 883–912. doi:10.1007/s42452-021-04869-x
Liikanen, A., Huttunen, J. T., Murtoniemi, T., Tanskanene, H., Vaisanen, T., Silvola, J., et al. (2003). Spatial and seasonal variation in greenhouse and nutrient dynamics and their interactions in the sediment of a boreal eutrophic lake. Biogeochemistry 65, 83–103. doi:10.1023/a:1026070209387
Loften, D. D., Whalen, S. C., and Hershey, A. E. (2014). Effect of temperature on methane dynamics and evaluation of methane oxidation kinetics in shallow Arctic Alaskan lakes. Hydrobiologia 721, 209–222. doi:10.1007/s10750-013-1663-x
López Bellido, J., Tulonen, T., Kankaala, P., and Ojala, A. (2009). CO2 and CH4 fluxes during spring and autumn mixing periods in a boreal lake (Pääjärvi, southern Finland). J. Geophys. Res. 114, G04007. doi:10.1029/2009JG000923
Martin, G., Rissanen, A. J., Garcia, S. L., Mehrshad, M., Buck, M., and Peura, S. (2021). Candidatus Methylumidiphilus drives peaks in methanotrophic relative abundance in stratified lakes and ponds across northern landscapes. Front. Microbiol. 12, 669937. doi:10.3389/fmicb.2021.669937
Martinez-Cruz, K., Lewis, M-C., Herriott, I. C., Sepulveda-Jauregui, A., Walter Anthony, K., Thalasso, F., et al. (2017). Anaerobic oxidation of methane by aerobic methanotrophs in sub-Arctic lake sediments. Sci. Total Environ. 607-608, 23–31. doi:10.1016/j.scitotenv.2017.06.187
Martinez-Cruz, K., Sepulveda-Jauregui, A., Walter Anthony, K., and Thalasso, F. (2015). Geographic and seasonal variation of dissolved methane and aerobic methane oxidation in Alaskan lakes. Biogeosciences 12, 4595–4606. doi:10.5194/bg-12-4595-2015
Mau, S., Blees, J., Helmke, E., Niemann, H., and Damm, E. (2013). Vertical distribution of methane oxidation and methanotrophic response to elevated methane concentrations in stratified waters of the Arctic fjord Storfjorden (Svalbard, Norway). Biogeosciences 10, 6267–6278. doi:10.5194/bg-10-6267-2013
Milucka, J., Kirf, M., Lu, L., Krupke, A., Lam, P., Littmann, S., et al. (2015). Methane oxidation coupled to oxygenic photosynthesis in anoxic waters. ISME J. 9, 1991–2002. doi:10.1038/ismej.2015.12
Møller, T. E., van der Bilt, G. G. M., Roerdink, D. L., and Jørgensen, S. L. (2020). Microbial community structure in Arctic lake sediments reflect variations in holocene climate conditions. Front. Microbiol. 11, 1520. doi:10.3389/fmicb.2020.01520
Morton, J. T., Marotz, C., Washburne, A., Silverman, J., Zaramela, L. S., Edlund, A., et al. (2019). Establishing microbial composition measurement standards with reference frames. Nat. Commun. 10, 2719. doi:10.1038/s41467-019-10656-5
Murase, J., and Sugimoto, A. (2005). Inhibitory effect of light on methane oxidation in the pelagic water column of a mesotrophic lake (Lake Biwa, Japan). Limnol. Oceanogr. 50, 1339–1343. doi:10.4319/lo.2005.50.4.1339
Osudar, R., Liebner, S., Alawi, M., Yang, S., Bussmann, I., and Wagner, D. (2016). Methane turnover and methanotrophic communities in arctic aquatic ecosystems of the Lena Delta, Northeast Siberia. FEMS Microbiol. Ecol. 92 (8), fiw116. doi:10.1093/femsec/fiw116
Oswald, K., Graf, J., Littmann, S., Tienken, D., Brand, A., Wehrli, B., et al. (2017). Crenothrix are major methane consumers in stratified lakes. ISME J. 11, 2124–2140. doi:10.1038/ismej.2017.77
Oswald, K., Jegge, C., Tischer, J., Berg, J., Brand, A., Miracle, M. R., et al. (2016). Methanotrophy under versatile conditions in the water column of the ferruginous meromictic Lake La Cruz (Spain). Front. Microbiol. 7, 1762. doi:10.3389/fmicb.2016.01762
Oswald, K., Milucka, J., Brand, A., Littmann, S., Wehril, B., Kuypers, M. M. M., et al. (2015). Light-dependent aerobic methane oxidation reduces methane emissions from seasonally stratified lakes. PLoS ONE 10 (7), e0132574. doi:10.1371/journal.pone.0132574
Quast, C., Pruesse, E., Yilmaz, P., Gerken, J., Schweer, T., Yarza, P., et al. (2013). The SILVA ribosomal RNA gene database project: Improved data processing and web-based tools. Nucleic Acids Res. 41 (D1), D590–D596. doi:10.1093/nar/gks1219
Rasilo, T., Prairie, Y. T., and Del Giorgio, P. A. (2015). Large-scale patterns in summer diffusive CH4 fluxes across boreal lakes, and contribution to diffusive C emissions. Glob. Change Biol. 21, 1124–1139. doi:10.1111/gcb.12741
Reis, P. C. J., Thottathil, S. D., Ruiz-Gonzales, C. l., and Prairie, Y. T. (2020). Niche separation within aerobic methanotrophic bacteria across lakes and its link to methane oxidation rates. Environ. Microbiol. 22 (2), 738–751. doi:10.1111/1462-2920.14877
Rosentreter, J. A., Borges, A. V., Deemer, B. R., Holgerson, M. A., Liu, S., Song, C., et al. (2021). Half of global methane emissions come from highly variable aquatic ecosystem sources. Nat. Geosci. 14, 225–230. doi:10.1038/s41561-021-00715-2
Ruuskanen, M. O., St. Pierre, K. A., Louis, St.V. L., Aris-Brosou, S., and Poulain, A. J. (2018). Physicochemical drivers of microbial community structure in sediments of lake hazen, nunavut, Canada. Front. Microbiol. 9, 1138. doi:10.3389/fmicb.2018.01138
Samad, M. S., and Bertilsson, S. (2017). Seasonal variation in abundance and diversity of bacterial methanotrophs in five temperate lakes. Front. Microbiol. 8, 142. doi:10.3389/fmicb.2017.00142
Saunois, M., Bousquet, P., Poulter, B., Peregon, A., Ciais, P., Canadell, J. G., et al. (2016). The global methane budget 2000-2012. Earth Syst. Sci. Data 8, 697–751. doi:10.5194/essd-8-697-2016
Schütte, U. M. E., Cadieux, S. B., Hemmerich, C., Pratt, L. M., and White, J. R. (2016). Unanticipated geochemical and microbial community structure under seasonal ice cover in a dilute, dimictic Arctic lake. Front. Microbiol. 7, 1035. doi:10.3389/fmicb.2016.01035
Shelley, F., Grey, J., and Trimmer, M. (2014). Widespread methanotrophic primary production in lowland chalk rivers. Proc. R. Soc. B 281, 20132854. doi:10.1098/rspb.2013.2854
Somers, D. J., Strock, K. E., and Saros, J. E. (2020). Environmental controls on microbial diversity in arctic lakes of west Greenland. Microb. Ecol. 80, 60–72. doi:10.1007/s00248-019-01474-9
IPCC (2013). Climate change 2013: The physical science basis. Contribution of working group I to the fifth assessment report of the intergovernmental panel on climate change. Editors T. F. Stocker, D. Qin, G.-K. Plattner, M. Tignor, S. K. Allen, J. Boschunget al. (Cambridge, United Kingdom and New York, NY, USA: Cambridge University Press), 1535. doi:10.1017/CBO9781107415324
Stoeva, M. K., Aris-Brosou, S., Che´telat, J., Hintelmann, H., Pelletier, P., and Poulain, A. J. (2014). Microbial community structure in lake and wetland sediments from a high arctic polar desert revealed by targeted transcriptomics. PLoS ONE 9 (3), e89531. doi:10.1371/journal.pone.0089531
Taenzer, L., Carini, P. C., Masterson, A. M., Bourquie, B., Gaube, J. H., and Leavitt, W. D. (2020). Microbial methane from methylphosphonate isotopically records source. Geophys. Res. Lett. 74. doi:10.1029/2019GL085872
Tamas, I., Smirnova, A. V., He, Z., and Dunfield, P. F. (2014). The (d)evolution of methanotrophy in the beijerinckiaceae – a comparative genomics analysis. ISME J. 8, 369–382. doi:10.1038/ismej.2013.145
Tan, Z., and Zhuang, Q. (2015). Arctic lakes are continuous methane sources to the atmosphere under warming conditions. Environ. Res. Lett. 10, 054016. doi:10.1088/1748-9326/10/5/054016
Tang, K. W., McGinnis, D. F., Frindte, K., Brüchert, V., and Grossart, H.-P. (2014). Paradox reconsidered: Methane oversaturation in well-oxygenated lake waters. Limnol. Oceanogr. 59, 275–284. doi:10.4319/lo.2014.59.1.0275
Tang, W. K., McGinnis, D. F., Ionescu, D., and Grossart, H.-P. (2016). Methane production in oxic lake waters potentially increases aquatic methane flux to air. Environ. Sci. Technol. Lett. 3, 227–233. doi:10.1021/acs.estlett.6b00150
Templeton, A. S., Chu, K.-H., Alvarez-Cohen, L., and Conrad, M. E. (2006). Variable carbon isotope fractionation expressed by aerobic CH4-oxidizing bacteria. Geochimica Cosmochimica Acta 70, 1739–1752. doi:10.1016/j.gca.2005.12.002
Theisen, A. R., and Murrell, J. C. (2005). Facultative methanotrophs revisited. J. Bacteriol. 187 (13), 4303–4305. doi:10.1128/JB.187.13.4303-4305.2005
Thompson, H. A., Pratt, L. M., and White, J. R. (2018). Spatial variation in stable isotopic composition of organic matter of macrophytes and sediments from a small Arctic Lake in West Greenland. Arct. Antarct. Alp. Res. 50 (1), ID1420282. doi:10.1080/15230430.2017.1420282
Thottathil, S. D., and Prairie, Y. T. (2021). Coupling of stable carbon isotopic signature of methane and ebullitive fluxes in northern temperate lakes. Sci. Total Environ. 777, 146117. doi:10.1016/j.scitotenv.2021.146117
Thottathil, S. D., Reis, P. C. J., del Giorgio, P. A., and Prairie, Y. T. (2018). The extent and regulation of summer methane oxidation in northern lakes. J. Geophys. Res. Biogeosci. 123, 3216–3230. doi:10.1029/2018JG004464
Thottathil, S. D., Reis, P. C. J., and Prairie, Y. T. (2019). Methane oxidation kinetics in northern freshwater lakes. Biogeochemistry 143, 105–116. doi:10.1007/s10533-019-00552-x
Tollefson, J. (2022). Scientists raise alarm over 'dangerously fast' growth in atmospheric methane. Nature. Epub ahead of print. doi:10.1038/d41586-022-00312-2
Valentine, D. L. (2002). Biogeochemistry and microbial ecology of methane oxidation in anoxic environments: A review. Ant. Van Leeuwenhoek 81, 271–282. doi:10.1023/A:1020587206351
Valentine, D. L., Chidthaisong, A., Rice, A., Reeburgh, W. S., and Tyler, S. C. (2004). Carbon and hydrogen isotope fractionation by moderately thermophilic methanogens 1. Geochimica Cosmochimica Acta 68, 1571–1590. doi:10.1016/j.gca.2003.10.012
van Grinsven, S., Sinninghe Damsté, J. S., Harrison, J., and Villanueva, L. (2020). Impact of electron acceptor availability on methane-influenced microorganisms in an enrichment culture obtained from a stratified lake. Front. Microbiol. 11, 715. doi:10.3389/fmicb.2020.00715
Vanwonterghem, I., Evans, P., Parks, D. H., Jensen, P. D., Woodcroft, B. J., Hugenholtz, P., et al. (2016). Methylotrophic methanogenesis discovered in the archaeal phylum Verstraetearchaeota. Nat. Microbiol. 1, 16170. doi:10.1038/nmicrobiol.2016.170
Vick-Majors, T., Priscu, J. C., and Amaral-Zettler, L. A. (2014). Modular community structure suggests metabolic plasticity during the transition to polar night in ice-covered Antarctic lakes. ISME J. 8, 778–789. doi:10.1038/ismej.2013.190
Vigneron, A., Lovejoy, C., Cruaud, P., Kalenitchenko, D., Culley, A., and Vincent, W. F. (2019). Contrasting winter versus summer microbial communities and metabolic functions in a permafrost thaw lake. Front. Microbiol. 10, 1656. doi:10.3389/fmicb.2019.01656
Wang, Q., Alowaifeer, A., Kerner, P., Balasubramanian, N., Patterson, A., Christian, W., et al. (2021). Aerobic bacterial methane synthesis. Proc. Natl. Acad. Sci. U. S. A. 118, e2019229118. doi:10.1073/pnas.2019229118
Westendorp, R. G. (1985). A quantitation method for dynamic headspace analysis using multiple runs. J. Chromatogr. Sci. 23, 521–524. doi:10.1093/chromsci/23.11.521
Wetzel, R. G. (2001). Limnology: lake and river ecosystems. 3rd ed. San Diego: Elsevier. doi:10.1016/C2009-0-02112-6
Whalen, S. C. (2005). Biogeochemistry of methane exchange between natural wetlands and the atmosphere. Environ. Eng. Sci. 22, 73–94. doi:10.1089/ees.2005.22.73
Whiticar, M. J. (1999). Carbon and hydrogen isotope systematics of bacterial formation and oxidation of methane. Chem. Geol. 161, 291–314. doi:10.1016/S0009-2541(99)00092-3
Whiticar, M. J., and Faber, E. (1985). Methane oxidation in sediment and water column environments – isotope evidence. Org. Geochem. 10, 759–768. doi:10.1016/s0146-6380(86)80013-4
Whiticar, M. J., Faber, E., and Schoell, M. (1986). Biogenic methane formation in marine and freshwater environments: CO2 reduction vs. acetate fermentation-isotope evidence. Geochim. Cosmochim. Acta 50, 693–709. doi:10.1016/0016-7037(86)90346-7
Wik, M., Thornton, B. F., Varner, R. K., McCalley, C., and Crill, P. M. (2020). Stable methane isotopologues from northern lakes suggest that ebullition is dominated by sub‐lake scale processes. J. Geophys. Res. Biogeosci. 125 (10), e2019JG005601. doi:10.1029/2019jg005601
Wik, M., Varner, R. K., Anthony, K. W., MacIntyre, S., and Bastviken, D. (2016). Climate-sensitive northern lakes and ponds are critical components of methane release. Nat. Geosci. 9 (2), 99–105. doi:10.1038/ngeo2578
Yao, M., Henny, C., and Maresca, J. A. (2016). Freshwater bacteria release methane as a byproduct of phosphorus acquisition. Appl. Environ. Microbiol. 82 (23), 6994–7003. doi:10.1128/AEM.02399-16
Zandt, M. H., Frank, J., Yilmaz, P., Cremers, G., Jetten, M. S. M., and Welte, C. U. (2020). Long-term enriched methanogenic communities from thermokarst lake sediments show species-specific responses to warming. FEMS Microbes 1 (1). doi:10.1093/femsmc/xtaa008
Zhu, J., Wang, Q., Yuan, M., Tan, G. A., Sun, F., Wang, C., et al. (2016). Microbiology and potential applications of aerobic methane oxidation coupled to denitrification (AME-D) process: A review. Water Res. 90, 203–215. doi:10.1016/j.watres.2015.12.020
Keywords: methane oxidation, methanotrophs, isotopes, lake, arctic, methane cycling
Citation: Cadieux SB, Schütte UME, Hemmerich C, Powers S and White JR (2022) Exploring methane cycling in an arctic lake in Kangerlussuaq Greenland using stable isotopes and 16S rRNA gene sequencing. Front. Environ. Sci. 10:884133. doi: 10.3389/fenvs.2022.884133
Received: 25 February 2022; Accepted: 06 September 2022;
Published: 19 October 2022.
Edited by:
Yves T Prairie, Université du Québec à Montréal, CanadaReviewed by:
Danny Ionescu, Leibniz-Institute of Freshwater Ecology and Inland Fisheries (IGB), GermanyYongcui Deng, Nanjing Normal University, China
Copyright © 2022 Cadieux, Schütte, Hemmerich, Powers and White. This is an open-access article distributed under the terms of the Creative Commons Attribution License (CC BY). The use, distribution or reproduction in other forums is permitted, provided the original author(s) and the copyright owner(s) are credited and that the original publication in this journal is cited, in accordance with accepted academic practice. No use, distribution or reproduction is permitted which does not comply with these terms.
*Correspondence: Sarah B. Cadieux, Y2FkaWVzQHJwaS5lZHU=