- 1Department of Biotechnology, University of Kotli Azad Jammu and Kashmir, Kotli, Pakistan
- 2School of Natural Sciences, National University of Sciences and Technology, Islamabad, Pakistan
- 3Department of Earth and Environmental Sciences, Bahria University, Islamabad, Pakistan
The extensive and unchecked application of chlorpyrifos against crop insects has caused contamination of various ecosystems, such as soil, sediments, and water, posing harm to plants, animals, useful arthropods, and humans. The present study aimed at evaluating the ability of proto-type constructed wetland to biodegrade chlorpyrifos and its major metabolites especially 2-hydroxy-3, 5, 6-trichloropyridine/ol (TCP) using chlorpyrifos-degrading indigenous bacterial strains, namely, Acinetobacter baumanni and Bacillus cibi with Canna spps. and indigenous Mentha spps. as a bacterial–plant consortium. Soil and plant samples were collected at regular time intervals for 12 weeks; analytes were extracted using the toluene method and evaluated through gas chromatography–mass spectrometry (GC-MS). In case of wetland vegetation with Canna and Mentha, 2-hydroxy-3, 5, 6-trichloropyridine (TCP, m/z = 198) and 2- hydroxypyridine (m/z = 97) with deprotonated molecular ions at m/z = 69 (M-H)−were detected as the intermediate metabolites, while in the bacterial–plant consortium, instead of TCP, 3, 5, 6-trichloro-2-methoxypyridine (TMP, m/z = 212) was formed along with di-ethylthiophosphate (DETP, m/z = 169). Based on the metabolite analysis using GC-MS, the biodegradation pathway for chlorpyrifos degradation through bacterial–plant consortia is predicted. The constructed wetland with the bacterial–plant consortium showed its potential to either bypass TCP generation, or TCP may have been immediately biodegraded by the plant part of the consortium. The designed constructed wetland provided a novel remedial measure to biodegrade chlorpyrifos without producing harmful metabolites.
1 Introduction
Chlorpyrifos is a broad-spectrum pesticide, extensively used against pests in agricultural (cotton, grains, and fruits) and urban (lawns, commercial, and domestic buildings) settings. It belongs to the organo-phosphate class, under the chemical name O, O-diethyl O-(3, 5, 6-trichloro-2- pyridinyl)-phosphorothioate (C9H11Cl3NO3PS) having low water solubility (2 mg/L) but soluble in organic solvents (Tariq et al., 2007).
Less than 1% of chlorpyrifos (CP) is applied to the target organisms, and most of the remaining chlorpyrifos ends up contaminating the atmosphere, soil, and water (Shi et al., 2019). Therefore, long-term and irregular applications of chlorpyrifos have resulted in large-scale pollution of soil, groundwater, sediment, and air. It eradicates non-targeted organisms along with the targeted ones including fish, useful arthropods, plants, animals, and humans. Its exposure leads to acetylcholine accumulation leading to high irritation and nerve compression (Gilani et al., 2016). This nerve compression leads to seizures and finally death of insects and mammals. Furthermore, CP and its metabolites are associated with endocrine disruption (Ur-Rehman et al., 2021; Ramos et al., 2019). This non-targeted biocidal activity on these organisms may also be responsible for the loss of biodiversity and overall environmental quality deterioration (Eskenazi et al., 1999; Matthews, 2006; Benachour et al., 2007).
The half-life of CP in water is 50 days (Dores & De-Lamonica-Freire, 2001), while in soil it varies from 60 to 120 days, although it can deviate from 2 weeks to over 1 year, depending on the soil type, climate, and other environmental conditions (Uniyal et al., 2021). Therefore, it has been detected as the second most common pesticide in food and water (John and Shaika, 2015). In aquatic environments, its metabolites, for e.g., 3, 5, 6-trichloropyridinol (TCP) and diethyl chlorpyrifos (DEC) are found, among which TCP has been documented as more toxic, persistent, and mobile than its parent compound CP, by the US-EPA with a half-life ranging from 65 to 360 days in soil (El-Hellow et al., 2013; Sud et al., 2020). The long half-life and antimicrobial nature pose a hurdle for the complete remediation of CP through microorganisms, leading to the accumulation of TCP which results in the loss of soil biodiversity.
There are numerous methods available for detoxification of chlorpyrifos including chemical treatment (Rayment and Higginson, 1992), photodecomposition, volatilization, and incineration, but most of them are not applicable for complete removal of contamination at low concentration due to their inefficiency, expensive, and environmentally unfriendly nature (Abraham et al., 2013). In the past few years, physicochemical (advanced oxidation process) and biological treatment approaches have been widely employed for pesticide removal. Being a cost-effective and eco-friendly method (Walkley and Black, 1934), bioremediation (microbial and phyto-degradation) of environmental pollutants, especially pesticides have been a focus to improve environmental quality in general and soil quality in particular (Nandhini et al., 2021). Chlorpyrifos, previously shown to be resistant to enhanced degradation, has now been proved to undergo enhanced microbe-mediated decay (John and Shaike, 2015). Several bacterial genera, especially Bacillus and Acinetobacter, Pseudomonas, Flavobacterium, Sphingomonas, and Agrobacterium sp. have been reported to biodegrade CP (Alizadeh et al., 2018; Anwar et al., 2009; Pino and Peñuela, 2011; Maya et al., 2011; Fulekar & Geetha, 2008; Yang et al., 2006). Among these reported bacterial species, Acinetobacter is reported as an efficient biodegrader of various organophosphates and is able to use those organophosphates as a sole carbon and energy source (Sabit et al., 2011). Therefore, microbial degradation is proven as a major factor determining the fate of organophosphate pesticides in the environment. In addition to microbial degradation, indigenous vegetation is reported to be involved in the degradation of CP. Plants may serve as a means to enhance the bioremediation process of contaminated soils as they can absorb and accumulate a variety of xenobiotics and metals from polluted soils and even degrade them, but the uptake process of organic pollutants and metals by plant roots is affected by several factors (Chandra et al., 2021). The herbaceous plants derived from local wetland species showed good growth when used to biodegrade chlorinated perchloro-ethylene (PCE) or its by-products (Avsar et al., 2007). Sometimes, the extended root system of plants in the soil apparently sustains microbial communities which are responsible for both anaerobic and aerobic biodegradative activity against such contaminants (Amon et al., 2007). These plants enhance the bioremediation process by release of exudates and enzymes such as carbohydrates, carboxylic acid, and amino acids that stimulate both microbial and biochemical activity in surrounding soil and mineralization of pollutants in rhizosphere soil (Tarla et al., 2020). The constant supply of carbon compounds from plant roots to rhizosphere microbes acts as fuels for complex interactions among rhizosphere organisms including those between microorganisms and plants (Daane et al., 2001). This consortium when exists as a land transition between terrestrial and aquatic systems, termed as wetland, plays an important role in the environment rehabilitation through natural decomposition or degradation (Terry and Bañuelos, 1999; Mejáre and Bülow, 2001). In addition to natural wetlands, lab-scale constructed wetland offers an option for ex situ bioremediation of contaminants, displaying a considerable potential to mitigate pesticide load including CP (Schulz and Peall, 2001). Plants in constructed wetlands also serve to stabilize the bed surface, increases porosity throughout the wetland volume for aerobic bacteria thriving in the soil, thus contributing toward the biodegradation process. Approximately, 92% removal of different pesticides from wastewater has been reported by Cooper et al. (2016) when a three-stage bio-bed was used as wetland. Retention capability was assessed by Schulz and Peall (2001) when no pesticide was detected in the outlet of a constructed wetland, while Gregoire et al. (2009) mentioned almost 80% removal efficiency for pesticide flux. Tang et al. (2019) reported a 98% CP removal with Cyperus alternifolius, Canna indica, Iris pseudacorus, Juncus effusus, and Typha orientalis in recirculating vertical flow constructed wetland systems. In a similar study, a constructed wetland (CW) cultivated with Polygonum punctatum, Cynodon spp., and Mentha aquatic showed approximately 98% removal efficiency of CP by Souza et al. (2017).
It has been shown that multiple bacterial species co-exist, not as isolated pockets of pure cultures, but as complex communities known as biofilms, which are capable of maximizing nutrient utilization and redox environments, etc., for example, some members of a community may convert plant exudates into a form available to other member of the community (Nottingham and Messer, 2021). Bacteria were isolated from the rhizosphere of Phragmites australis growing in the distillery effluent contaminated site mostly present in the lower region of roots, capable for the bioremediation of distillery wastewater contaminated sites (Chandra & Chaturvedi, 2002).
Taking into account the CP biodegradation process, the major CP metabolite, TCP, being antimicrobial and having a higher water-soluble nature, makes it more mobile in various environmental matrices (Bose et al., 2021). Furthermore, the produced TCP, as a result of microbial biodegradation, in turn proves lethal for the biodegrading microorganisms resulting not only in decline in the biodegradation efficiency but also in the loss of diversity of soil microbial fauna (Abraham et al., 2013). In addition, studies concerning its fate and degradation in the soil are very limited. So, its further degradation is crucial to alleviate its concentration to prevent its magnification in the environment, thus mitigating the pollution and toxicity posed by TCP in particular and other metabolites of CP in general.
The CP biodegradation efficiency in a proto-type CW is influenced by many independent environmental factors such as bacterial species, nature of the pesticide, hydraulic retention time (HRT), and the plant species (deMatos et al., 2009; Tu et al., 2018). As reported by Romos et al. (2019) for pesticides with short aquatic half-lives, wetland systems require to exhibit much longer residence times (RTs). So, in the present study, the bacterial–plant consortium was used to 1) observe the potential of constructed wetland for bioremediation of CP and its major metabolites, especially TCP using the indigenous plant–bacterial consortium; 2) compare the biodegradation of chlorpyrifos (CP) and its metabolites using wetland vegetation alone and plant–bacterial consortium for possible biodegradation pathway analysis.
2 Materials and Methods
2.1 Chemicals and Reagents
Analytical-grade chemicals and reagents were purchased from Sigma-Aldrich, while commercial chlorpyrifos (48% w/v) was purchased from standard commercial suppliers. Minimal salt medium (MSM; pH 6.8–7.0) was prepared using the chemicals as described: dextrose, 1.0 g/L; K2HPO4, 7.0 g/L; KH2PO4, 2.0 g/L; sodium citrate, 0.5 g/L; MgSO4.7H2O, 0.1 g/L; and (NH4)2SO4, 1.0 g/L (Parmar et al., 2014).
2.1.1 Soil Parameter Analysis
Different parameters of soil such as pH, soil nutrients, soil texture, and soil nitrogen contents were analyzed as described in the following sections.
2.1.2 pH Measurement
In a 100-ml bottle, about 10 g of air-dried soil was weighed, and 25 ml of distilled water was added and shaken for about 1 h. After shaking, the glass electrode was dipped in soil suspension and pH of soil was recorded (Ohtsu et al., 1994).
2.1.3 Organic Matter Determination
To the air-dried and sieved 1 g soil, 10 ml of k2Cr2O7 solution (1 N) was added. Then, 20 ml of concentrated H2SO4 was added and mixed well, and the mixture was allowed to stand for about half an hour. On the other hand, 25–30 drops of diphenylamine indicator and 0.2 g of sodium fluoride were added to distilled water, and the solution was titrated with ferrous ammonium sulfate solution. A color change from dull green to brilliant green was noticed. A blank sample (without soil) was also run in the same way (Walkely–Black method).
2.1.4 Total Nitrogen
One gram of dried soil was shifted to a digestion tube along with 10 ml of H2SO4 and 5 g of catalyst mixture and heated to 100°C with an increase in temperature up to 400°C. The sample was noted, and the cooled sample was shifted into a distillation unit with 40 ml NaOH (40%) and 20 ml boric acid (4%) as an indicator, and any color change was noted. The distillate was titrated with sulfuric acid (0.02 N). A blank sample was also run using the same method (AOAC, 1995).
2.1.5 Total Phosphorous
Dried soil (2.5 g) was added to 0.5 g activated charcoal. To this mixture, 50 ml NaHCO3 (0.5 M) solution was added and shaken for almost half an hour and filtered through the Whatman filter paper. The filtrate was acidified by adding H2SO4 (5N) and ascorbic acid with distilled water as an added solvent. A blank sample was also run with the similar conditions and compared with the test sample (Koenig & Johnson, 1942).
2.1.6 Determination of Potassium
Five gram of soil was weighed with 25 ml of ammonium acetate (NH4OAc) solution, then shaken for five minutes, and filtered through the Whatman filter paper. Potassium extract was measured (Black, 1965).
2.2 Screening and Isolation of Chlorpyrifos-Degrading Bacteria
To isolate the potential bacterial strains, soil samples from selected chlorpyrifos-infested sites were collected from Kotli, Azad Jammu, and Kashmir-Pakistan (33.2896 ºN 73.7414 ºE). This particular field of choice has been exposed to continuous applications of chlorpyrifos for a considerable period of time. The soil samples were obtained from 5 to 10 cm layers below soil surface as described by Wang et al. (2021). One gram soil sample was mixed in distilled water (10 ml), and 1 ml of the solution was spread on the nutrient agar media through the spread plate method. The plates were incubated at 37°C for 24 h. Morphologically distinct colonies were selected and purified through the streak plate method.
2.2.1 Enrichment of the Bacterial Strains
Isolated strains were selected by subjecting them to the increasing concentrations of chlorpyrifos (250, 500, 750, and 1,000 ug/L). For this purpose, the isolated strains were grown in MSM medium (pH 7.0) on a shaker (IRMECO, I 3000, Germany) at 200 rpm for a period of 72 h as described by Abraham et al. (2013) with some modification. CP degradation was observed in the first set of experiments by bacterial isolates in minimal salt media (MSM) in different concentrations and combinations with CP as the sole carbon source along with positive and negative controls.
2.3 Proto-Type Constructed Wetland
A prototype wetland was constructed using pots filled with coarse and fine gravel, coarse gravel (20–30 mm diameter), fine gravel (2–10 mm diameter), sand, and soil from a specific site (Figures 1A,B). Prior to hand milling, soil was air-dried and screened through a sieve (2 mm pore size). The local plants, namely, Canna spps. and Mentha spps. (indigenous mint), were selected for constructed wetland (CW) establishment due to their ubiquitous abundance in the region, especially on the sampling site and along the river. The use of indigenous species is preferred as they do not pose any negative impact on micro-flora and has better survival chance (Farhan et al., 2021). A total of twelve (12) CW arrangements were maintained under different experimental conditions, namely, control, with isolated bacterial strains in soil alone, with plant and soil alone, and with a mix bacterial–plant consortium, and spiked with predefined CP concentrations.
2.3.1 Extraction of Chlorpyrifos From Soil
After a set retention time, the remaining chlorpyrifos was extracted from the CW soils using the toluene method as described by Reddy et al. (2013). A measured quantity of soil (12.5 g) was added to 20 ml toluene in 50-ml Teflon centrifuge tubes and kept on a horizontal shaker (IRMECO, OS 10, Germany) at 150 rpm for 4 h at 25°C. The tubes were then centrifuged at 4000 rpm for 10 min (Reddy et al., 2013). The resultant extract was filtered through anhydrous sodium sulfate (Na2SO4). For GC-MS analysis, 1 ml of toluene was added to the filtrate.
2.3.2 GC-MS Analysis for Chlorpyrifos Biodegradation
Degradation of chlorpyrifos to its metabolites was confirmed by GC-MS (Perkin Elmer, MS Claurus SQ 8S, GC Claurus 590, USA) equipped with a HP-5MS capillary column (30 m, 0.025 mm i.d) in helium carrier gas (1 ml per min) and with a splitless injection system. Initially, the column was maintained for 5 min at 90°C and then increased to 290°C at a rate of 8°C per min and held at 290°C for 5 min (Reddy et al., 2013). The injector and interface temperature were kept at 280°C and the source temperature at 250°C. A mass spectrum was obtained by the electron impact (EI) at 70 eV.
3 Results and Discussion
3.1 Soil Analysis
Soil used in the constructed wetland was analyzed for different parameters such as saturation, pH, texture, organic matter, nitrogen content, phosphorous, and potassium. Soil saturation was 51% with basic pH (8.12) with a texture of clayey loam with 0.059%, 16, and 131 ppm of nitrogen, phosphorous, and potassium contents, respectively.
3.2 Isolation and Identification of Pesticide-Degrading Bacteria
In the present study, a bioremediation system was developed by isolating CP-degrading indigenous bacterial cultures, and their subsequent application in the indigenous plants using constructed wetland (CW). A total of two morphologically distinct CP-tolerant bacterial strains MBT035 and MBT037 were isolated and identified through ribotyping (16s rRNA) by Macrogen (Macrogen Inc., South Korea) as Acinetobacter baumanni and Bacillus cibi, respectively.
The CW establishment was applied in two combinations: a) soil and plants only and b) soil, plant, and bacterial consortium, to observe the effect of the bacterial isolate alone, plants alone, and in consortium. The CP removal efficiency in constructed wetland was analyzed by GC-MS, in which MS spectra of the extracted samples showed an effective biodegradation using constructed wetland when cultivated with indigenous Canna spps. and Mentha spps. and bioaugmented with Acinetobacter baumanni and Bacillus cibi (CP-degrading bacterial isolates). By observing the chromatograph, it is evident that chlorpyrifos was metabolized to produce various intermediates of different m/z in various combinations of CWs, that is, plant alone and in the bacterial–plant consortium. The results are described in the following sections:
3.2.1 Wetland With Indigenous Plants
The wetland vegetation, Cannas spps. and Mentha spps., in a CW was used to monitor the biodegradation of CP through the phyto-degradation process. Several characteristic peaks of different metabolites of chlorpyrifos’s biodegradation were observed in the GC-MS chromatogram. Among them, the most documented intermediate and degradation product, TCP (3, 5, 6-tricloro-2-piridinol) was detected, which was confirmed by the m/z peak of m/z = 198 (Figure 2).This product has already been documented in case of bacterial biodegradation by various researchers (Abraham et al., 2013; Zhu et al., 2019). But, it is also reported as a more toxic pollutant than the parent compound CP in the environment, especially for the microbes in the soil (antimicrobial agent). This may be a possible reason for resistance to enhanced microbial biodegradation for CP biodegradation. In the current study, TCP was also reported when indigenous plant vegetation was used. But, a good growth response to this toxic TCP was shown by the wetland vegetation. This toxic antimicrobial compound seemed either non-toxic to the indigenous plants or the plants might have coped with the toxicity posed by this particular intermediate of CP biodegradation. The study endorses the hypothesis of plant degradation potential for TCP.
Further degradative product, diethyl acid phosphate (DEP) with a molecular ion at m/z 152.98 [M - 1]- was also detected which was further metabolized into a molecule of m/z = 98, identified as H3PO4. These results were found in accordance with Shi et al. (2019).
TCP was further degraded to form 2-hydroxypyridine (m/z = 97) with deprotonated molecular ions at m/z = 69 (M-H)− as described by Uniyal et al. (2021).The results were found in accordance with Tang et al. (2019) who reported a decrease in the half-life of TCP when Canna indica was used as wetland vegetation with approximately 33 percent removal. These results showed efficient biodegradation of CP and its metabolites, especially TCP in proto-type constructed wetland when Canna and Mentha spps. were used.
3.2.2 Soil, Plant, and Bacterial Consortium
In the second phase of the experimentation setup of the plant–bacterial consortium, when wetland vegetation, i.e., Canna spps. and Mint spps., was used along with the indigenous bacterial strains, a CP metabolite with m/z = 212 was detected corresponding to TMP (3, 5, 6-trichloro-2-methoxypyridine). In addition to TMP, another metabolite (m/z = 169) was also detected and found out to be diethyl-thio-phosphate (DETP) a CP hydrolysis product mentioned by Bicker et al. (2005) in his studies (Figure 3). As described by Chen et al. (2012), the degrading microorganisms tend to metabolize chlorpyrifos by hydrolysis to form diethyl-thio-phosphoric acid (DETP) along with TCP. On the other hand, the bacterial–plant consortium showed good growth performance, depicting a good consortium establishment between plants and isolated strains (Figure 4). When metabolites of the biodegradation in both the experimental designs, i.e., wetland vegetation alone and plant–bacterial consortium, were compared, this TCP was not detected in plant–bacterial association rather TMP was detected. It is already described by Shi et al. (2019) that TCP can further generate TMP. The transient appearance of TCP and its subsequent degradation to TMP may be attributed to the mutual interaction and biodegradation ability of the plant–bacterial consortium. It may further be inferred from the GC-MS chromatogram that the formation of TCP may be either bypassed, or it was synthesized for a very short period of time and immediately biodegraded by the plant part of the consortium. The synthesis of TMP in place of TCP was found against the findings of Chen et al. (2012) as in most of the cases reported to date, the bacterial isolates tended to transform chlorpyrifos to yield TCP, which in turn accumulated in the batch cultures or soils and posed toxic effects on the microbial culture. So, the enhanced microbial degradation could not occur owing to its antimicrobial properties (Chen et al., 2012).
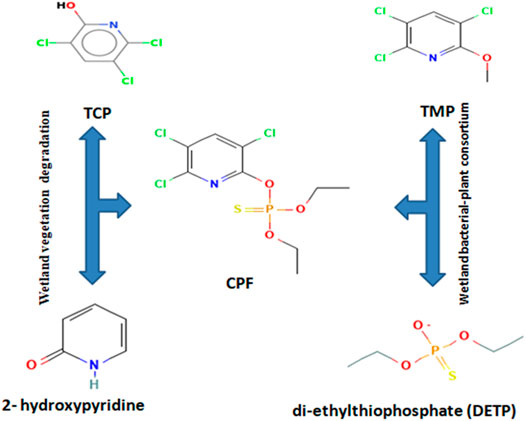
FIGURE 4. Proto-type constructed wetland with indigenous bacterial strains and Canna and Mentha spps.
During the study, a good adaptation of the bacterial isolates to the applied CP was observed in the plant–bacterial consortium, since the bacterial number increased with increasing concentration of the pesticide to the soil. This could be reasoned to the absence of TCP in the bacterial–plant consortium which may be the reason for prolonged survival and good growth of the bacterial–plant consortium. An overall 96% of CP removal was observed in the bacterial–plant consortium. These results were found against the findings of Chen et al. (2012) who reported formation of TCP along with DETP.
Thus, the bacterial augmentation in the experimental soil with wetland vegetation eventually caused higher degradation, suggesting the compatibility of the augmented culture with the indigenous wetland vegetation. The dominant removal process was reported to occur through microbial degradation in wetland technology as described by Liu et al. (2019). In a similar study, Singh et al. (2003) observed maximum biodegradation of CP using Pseudomonas putida after 90 days in basic soils. These results were also found in accordance with Souza et al. (2017) and Uniyal et al. (2021).
3.3 Proposed Biodegradation Pathway for CP
The insecticide CPF mostly undergoes hydrolysis to 3, 5, 6-trichloro-2-pyridinol (TCP), diethyl-thio-phosphoric acid (DETP), and negligible amounts of other intermediate products (Das and Adhya, 2015).TCP is a major metabolite of CP, and under all pathways, it is observed to undergo ring cleavage to form smaller organic and inorganic molecules as described by Sud et al. (2020). The degradation pathway of chlorpyrifos begins with cleavage of the phosphorus ester bond to yield 3, 5, 6-trichloro-2-pyridinol (TCP) (Lee et al., 2012). Based on the GC-MS analysis, the possible degradation pathway in case of wetland vegetation is proposed via hydrolysis as: CP (m/z = 358 > TCP (m/z = 198) >2- hydroxypyridine (m/z = 198) > 69. While in case of bacterial–plant association, CP (m/z = 358)>TMP (m/z = 212>TCP-2H (m/z = 196) > DETP (m/z = 169) is suggested (Figure 5).
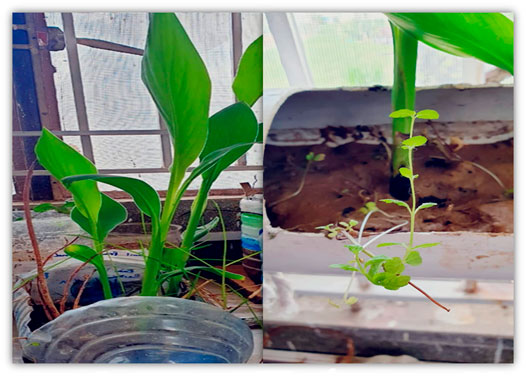
FIGURE 5. Proposed biodegradative pathway for chlorpyrifos in wetland vegetation and bacterial–plant association.
4 Conclusion
The present study was designed to observe the potential of constructed wetland using indigenous bacterial–plant association for the chlorpyrifos biodegradation. Therefore, a bioremediation system was developed by isolating CP-degrading indigenous bacterial cultures and their subsequent application in couple with indigenous plants using constructed wetland.
1. Two morphologically distinct CP-biodegrading bacterial strains Acinetobacter baumanni (MBT035) and Bacillus cibi (MBT037) were isolated and purified.
2. A pro-type constructed wetland (CW) with indigenous Canna spps. and Mentha spps. and bioaugmented with Acinetobacter baumanni and Bacillus cibi showed enhanced biodegradation of chlorpyrifos (CP) up to 96%.
3. TCP (3, 5, 6-tricloro-2-piridinol, m/z = 198) was observed when indigenous plant vegetation was used in CW which was further degraded to form 2-hydroxypyridine (m/z = 97) with de-protonated molecular ions at m/z = 69 (M-H)−.
4. In case of the bacterial–plant consortium, instead of TCP, a 3, 5, 6-trichloro-2-methoxypyridine (TMP, m/z = 212) was detected along with diethyl-thio-phosphate (DETP, m/z = 169).
5. The results of the present study showed good potential constructed wetland with Canna spps. and Mentha spps. and bioaugmented with Acinetobacter baumanni and Bacillus cibi.
5 Future Prospects
The current study promotes further research on plant–microbe joint combined remediation and examines the different behaviors. Following future prospects may be considered:
1. The fate of the other metabolites of CP and their toxicity may be monitored in the plants and animal species thriving in soil or environment.
2. Attempts could be made to optimize the bacterial–plant consortium for maximum and efficient bioremediation of the CP as well other environmental contaminants.
Data Availability Statement
The original contributions presented in the study are included in the article/Supplementary Materials, further inquiries can be directed to the corresponding author.
Author Contributions
TA performed the experiments and wrote the manuscript. SR designed the experiments, administered the project, and wrote the manuscript. AS conceived the idea and designed the experiments. HN assisted in metabolite analysis. AF assisted in manuscript writing and editing. AJ assisted in the analysis of the results and review of the manuscript. SJ reviewed the manuscript.
Conflict of Interest
The authors declare that the research was conducted in the absence of any commercial or financial relationships that could be construed as a potential conflict of interest.
Publisher’s Note
All claims expressed in this article are solely those of the authors and do not necessarily represent those of their affiliated organizations, or those of the publisher, the editors, and the reviewers. Any product that may be evaluated in this article, or claim that may be made by its manufacturer, is not guaranteed or endorsed by the publisher.
Acknowledgments
The authors are thankful to the technical support provided by the National University of Sciences and Technology (NUST), Islamabad, Pakistan.
References
Abraham, J., Shanker, A., and Silambarasan, S. (2013). Role of Gordonia Sp JAAS1 in Biodegradation of Chlorpyrifos and its Hydrolysing Metabolite 3,5,6-Trichloro-2-Pyridinol. Lett. Appl. Microbiol. 57, 510–516. doi:10.1111/lam.12141
Amon, J. P., Agrawal, A., Shelley, M. L., Opperman, B. C., Enright, M. P., Clemmer, N. D., et al. (2007). Development of a Wetland Constructed for the Treatment of Groundwater Contaminated by Chlorinated Ethenes. Ecol. Eng. 30, 51–66. doi:10.1016/j.ecoleng.2007.01.008
Anwar, S., Liaquat, F., Khan, Q. M., Khalid, Z. M., and Iqbal, S. (2009). Biodegradation of Chlorpyrifos and its Hydrolysis Product 3,5,6-Trichloro-2-Pyridinol by Bacillus Pumilus Strain C2A1. J. Hazard. Mater. 168, 400–405. doi:10.1016/j.jhazmat.2009.02.059
Aoac, A. (1995). Official Methods of Analysis. 16th Ed. Washington DC, USA: Association of official analytical chemists. Sci. Educ.
Avsar, Y., Tarabeah, H., Kimchie, S., and Ozturk, I. (2007). Rehabilitation by Constructed Wetlands of Available Wastewater Treatment Plant in Sakhnin. Ecol. Eng. 29, 27–32. doi:10.1016/j.ecoleng.2006.07.008
Benachour, N., Moslemi, S., Sipahutar, H., and Seralini, G. (2007). Cytotoxic Effects and Aromatase Inhibition by Xenobiotic Endocrine Disrupters Alone and in Combination☆. Toxicol. Appl. Pharmacol. 222, 129–140. doi:10.1016/j.taap.2007.03.033
Bicker, W., Lämmerhofer, M., and Lindner, W. (2005). Determination of Chlorpyrifos Metabolites in Human Urine by Reversed-Phase/weak Anion Exchange Liquid Chromatography-Electrospray Ionisation-Tandem Mass Spectrometry. J. Chromatogr. B 822, 160–169. doi:10.1016/j.jchromb.2005.06.003
Black, C. A. (1965). Methods of Soil Analysis Part I Am. Soc. Madison, Wis.: American Society of Agronomy.
Bose, S., Kumar, P. S., and Vo, D.-V. N. (2021). A Review on the Microbial Degradation of Chlorpyrifos and its Metabolite TCP. Chemosphere 283, 131447. doi:10.1016/j.chemosphere.2021.131447
Chandra, R., Bharagava, R. N., and Yadav, S. (2021). Wetland Rhizosphere Bacteria: An Eco-Friendly Tool for Bioremediation of Environmental Pollutants.
Chandra, R., and Chaturvedi, S. (2002). “Isolation and Characterization of Bacterial Population from Rhizosphere of Cyperus Papyrus Growing in Distillery Effluent Contaminated Site,” in 2 nd International conference on plants and environmental pollution held on 2002 at NBRI (India: Lucknow). Abstract no. SIII/P-5.
Chen, S., Liu, C., Peng, C., Liu, H., Hu, M., and Zhong, G. (2012). Biodegradation of Chlorpyrifos and its Hydrolysis Product 3,5,6-Trichloro-2-Pyridinol by a New Fungal Strain Cladosporium Cladosporioides Hu-01. PLoS One 7, e47205. doi:10.1371/journal.pone.0047205
Cooper, R. J., Fitt, P., Hiscock, K. M., Lovett, A. A., Gumm, L., Dugdale, S. J., et al. (2016). Assessing the Effectiveness of a Three-Stage On-Farm Biobed in Treating Pesticide Contaminated Wastewater. J. Environ. Manage. 181, 874–882. doi:10.1016/j.jenvman.2016.06.047
Daane, L. L., Harjono, I., Zylstra, G. J., and Häggblom, M. M. (2001). Isolation and Characterization of Polycyclic Aromatic Hydrocarbon-Degrading Bacteria Associated with the Rhizosphere of Salt Marsh Plants. Appl. Environ. Microbiol. 67, 2683–2691. doi:10.1128/aem.67.6.2683-2691.2001
Das, S., and Adhya, T. K. (2015). Degradation of Chlorpyrifos in Tropical rice Soils. J. Environ. Manage. 152, 36–42. doi:10.1016/j.jenvman.2015.01.025
Dores, E. F. G. d. C., and De-Lamonica-Freire, E. M. (2001). Contaminação Do ambiente aquático por pesticidas. Estudo de caso: águas usadas para consumo humano em Primavera Do Leste, Mato Grosso - análise preliminar. Quím. Nova 24, 27–36. doi:10.1590/S0100-40422001000100007
El-Helow, E. R., Badawy, M. E. I., Mabrouk, M. E. M., Mohamed, E. A. H., and El-Beshlawy, Y. M. (2013). Biodegradation of Chlorpyrifos by a Newly IsolatedBacillus subtilisStrain, Y242. Bioremediation J. 17, 113–123. doi:10.1080/10889868.2013.786019
Eskenazi, B., Bradman, A., and Castorina, R. (1999). Exposures of Children to Organophosphate Pesticides and Their Potential Adverse Health Effects. Environ. Health Perspect. 107, 409–419. doi:10.1289/ehp.99107s3409
Farhan, M., Ahmad, M., Kanwal, A., Butt, Z. A., Khan, Q. F., Raza, S. A., et al. (2021). Biodegradation of Chlorpyrifos Using Isolates from Contaminated Agricultural Soil, its Kinetic Studies. Sci. Rep. 11, 1–14. doi:10.1038/s41598-021-88264-x
Fulekar, M. H., and Geetha, M. (2008). Bioremediation of Chlorpyrifos by Pseudomonas aeruginosa Using Scale up Technique. J. Appl. Biosci. 12, 657–660.
Gilani, R. A., Rafique, M., Rehman, A., Munis, M. F. H., Rehman, S. U., and Chaudhary, H. J. (2016). Biodegradation of Chlorpyrifos by Bacterial genusPseudomonas. J. Basic Microbiol. 56, 105–119. doi:10.1002/jobm.201500336
Gregoire, C., Elsaesser, D., Huguenot, D., Lange, J., Lebeau, T., Merli, A., et al. (2009). Mitigation of Agricultural Nonpoint-Source Pesticide Pollution in Artificial Wetland Ecosystems - A Review. Clim. Change Intercropping, Pest Control. Beneficial Microorg., 293–338. doi:10.1007/978-90-481-2716-0_11
John, E. M., and Shaike, J. M. (2015). Chlorpyrifos: Pollution and Remediation. Environ. Chem. Lett. 13, 269–291. doi:10.1007/s10311-015-0513-7
Koenig, R., and Johnson, C. (1942). Colorimetric Determination of Phosphorus in Biological Materials. Ind. Eng. Chem. Anal. Ed. 14, 155–156. doi:10.1021/i560102a026
Lee, K. Y., Strand, S. E., and Doty, S. L. (2012). Phytoremediation of Chlorpyrifos byPopulusandSalix. Int. J. Phytoremediation 14, 48–61. doi:10.1080/15226514.2011.560213
Liu, T., Xu, S., Lu, S., Qin, P., Bi, B., Ding, H., et al. (2019). A Review on Removal of Organophosphorus Pesticides in Constructed Wetland: Performance, Mechanism and Influencing Factors. Sci. Total Environ. 651, 2247–2268. doi:10.1016/j.scitotenv.2018.10.087
Matos, A., Freitas, W., and Lo Monaco, P. (2009). Capacidade extratora de diferentes espécies vegetais cultivadas em sistemas alagados utilizados no tratamento de águas residuárias da suinocultura. Ambi-Agua 4, 31–45. doi:10.4136/ambi-agua.84
Matthews, G. A. (2006). Pesticides: Health, Safety and the Environment. Chichester, UK: Blackwell Publishing.
Maya, K., Singh, R. S., Upadhyay, S. N., and Dubey, S. K. (2011). Kinetic Analysis Reveals Bacterial Efficacy for Biodegradation of Chlorpyrifos and its Hydrolyzing Metabolite TCP. Process Biochem. 46, 2130–2136. doi:10.1016/j.procbio.2011.08.012
Mejáre, M., and Bülow, L. (2001). Metal-binding Proteins and Peptides in Bioremediation and Phytoremediation of Heavy Metals. Trends Biotechnol. 19, 67–73. doi:10.1016/S0167-7799(00)01534-1
Nandhini, A. R., Muthukumar, H., and Gummadi, S. N. (2021). Chlorpyrifos in Environment and Foods: A Critical Review of Detection Methods and Degradation Pathways. Environ. Sci. Process. Impacts. doi:10.1039/D1EM00178G
Nottingham, E. R., and Messer, T. L. (2021). A Literature Review of Wetland Treatment Systems Used to Treat Runoff Mixtures Containing Antibiotics and Pesticides from Urban and Agricultural Landscapes. Water 13, 3631. doi:10.3390/w13243631
Parmar, K. J., Tomar, R. S., Parakhia, M. V., Malviya, B. J., Rathod, V. M., Thakkar, J. R., et al. (2014). Isolation and Bio-Analytical Characterization of Chloropyrifos Degrading Bacteria. J.Cell Tissue Res. 14, 4641.
Pino, N., and Peñuela, G. (2011). Simultaneous Degradation of the Pesticides Methyl Parathion and Chlorpyrifos by an Isolated Bacterial Consortium from a Contaminated Site. Int. Biodeterioration Biodegradation 65, 827–831. doi:10.1016/j.ibiod.2011.06.001
Ramos, A., Whelan, M. J., Guymer, I., Villa, R., and Jefferson, B. (2019). On the Potential of On-Line Free-Surface Constructed Wetlands for Attenuating Pesticide Losses from Agricultural Land to Surface Waters. Environ. Chem. 16, 563–576. doi:10.1071/en19026
Rayment, G. E., and Higginson, F. R. (1992). Australian Laboratory Handbook of Soil and Water Chemical Methods. c1992 xvii, 330 p.. Melbourne: Inkata press.
Reddy, A., Madhavi, V., Reddy, K. G., and Madhavi, G. (2013). Remediation of Chlorpyrifos Contaminated Soils by Laboratory-Synthesized Zero-Valent Nano Iron Particles: Effect of pH and Aluminium Salts. J. Chem. 2013, 1–7. doi:10.1155/2013/521045
Sabit, H. H., Said, O. A., Shamseldin, A. F., and &Elsayed, K. (2011). Molecular Identification of Acinetobacter Isolated from Egyptian Dumpsite as Potential Bacteria to Degrade Malathion. Int. J. Acad. Res. 3, 84–90.
Schulz, R., and Peall, S. K. C. (2001). Effectiveness of a Constructed Wetland for Retention of Nonpoint-Source Pesticide Pollution in the Lourens River Catchment, South Africa. Environ. Sci. Technol. 35, 422–426. doi:10.1021/es0001198
Shi, T., Fang, L., Qin, H., Chen, Y., Wu, X., and Hua, R. (2019). Rapid Biodegradation of the Organophosphorus Insecticide Chlorpyrifos by Cupriavidus Nantongensis X1T. Ijerph 16, 4593. doi:10.3390/ijerph16234593
Singh, B. K., Walker, A., Morgan, J. A. W., and Wright, D. J. (2003). Effects of Soil pH on the Biodegradation of Chlorpyrifos and Isolation of a Chlorpyrifos-Degrading Bacterium. Appl. Environ. Microbiol. 69, 5198–5206. doi:10.1128/AEM.69.9.5198-5206.2003
Souza, T. D. D., Borges, A. C., Matos, A. T. D., Mounteer, A. H., and Queiroz, M. E. L. R. d. (2017). Removal of Chlorpyrifos Insecticide in Constructed Wetlands with Different Plant Species. Rev. Bras. Eng. Agríc. Ambient. 21, 878–883. doi:10.1590/1807-1929/agriambi.v21n12p878-883
Sud, D., Kumar, J., Kaur, P., and Bansal, P. (2020). Toxicity, Natural and Induced Degradation of Chlorpyrifos. J. Chil. Chem. Soc. 65, 4807–4816. doi:10.4067/S0717-97072020000204807
Tang, X.-Y., Yang, Y., McBride, M. B., Tao, R., Dai, Y.-N., and Zhang, X.-M. (2019). Removal of Chlorpyrifos in Recirculating Vertical Flow Constructed Wetlands with Five Wetland Plant Species. Chemosphere 216, 195–202. doi:10.1016/j.chemosphere.2018.10.150
Tariq, M. I., Afzal, S., Hussain, I., and Sultana, N. (2007). Pesticides Exposure in Pakistan: a Review. Environ. Int. 33, 1107–1122. doi:10.1016/j.envint.2007.07.012
Tarla, D. N., Erickson, L. E., Hettiarachchi, G. M., Amadi, S. I., Galkaduwa, M., Davis, L. C., .., , Nurzhanova, A., and Pidlisnyuk, V. (2020). Phytoremediation and Bioremediation of Pesticide-Contaminated Soil. Appl. Sci. 10 (4), 1217. doi:10.3390/app10041217
Terry, N., and Bañuelos, G. (1999). Phytoremediation of Contaminated Soil and Water. Lewis Publishers is an imprint of CRC Press LLC. Printed in the United States of Florida, America. doi:10.1201/9780367803148
Tu, Y., Jiang, L., and Li, H. (2018). Non-persistent Pesticides Removal in Constructed Wetlands. AIP Conf. Proc. 1944, 020045. AIP Publishing LLC. doi:10.1063/1.5029761
Ubaid Ur Rahman, H., Asghar, W., Nazir, W., Sandhu, M. A., Ahmed, A., and Khalid, N. (2021). A Comprehensive Review on Chlorpyrifos Toxicity with Special Reference to Endocrine Disruption: Evidence of Mechanisms, Exposures and Mitigation Strategies. Sci. Total Environ. 755, 142649–649. doi:10.1016/j.scitotenv.2020.142649
Uniyal, S., Sharma, R. K., and Kondakal, V. (2021). New Insights into the Biodegradation of Chlorpyrifos by a Novel Bacterial Consortium: Process Optimization Using General Factorial Experimental Design. Ecotoxicology Environ. Saf. 209, 111799–799. doi:10.1016/j.ecoenv.2020.111799
Walkley, A., and Black, I. A. (1934). An Examination of the Degtjareff Method for Determining Soil Organic Matter, and a Proposed Modification of the Chromic Acid Titration Method. Soil Sci. 37, 29–38. doi:10.1097/00010694-193401000-00003
Wang, Y., Zhang, W., Zhang, Z., Wang, W., Xu, S., and He, X. (2021). Isolation, Identification and Characterization of Phenolic Acid‐degrading Bacteria from Soil. J. Appl. Microbiol. 131 (1), 208–220. doi:10.1111/jam.14956
Yang, C., Liu, N., Guo, X., and Qiao, C. (2006). Cloning of Mpd Gene from a Chlorpyrifos-Degrading Bacterium and Use of This Strain in Bioremediation of Contaminated Soil. FEMS Microbiol. Lett. 265, 118–125. doi:10.1111/j.1574-6968.2006.00478.x
Keywords: chlorpyrifos, bioremediation, crop insects, constructed wetland, biodegradation pathway
Citation: Aziz T, Rasheed S, Shah AH, Nasir H, Fariq A, Jamil A and Jannat S (2022) Bioremediation Potential of Plant-Bacterial Consortia for Chlorpyrifos Removal Using Constructed Wetland. Front. Environ. Sci. 10:880807. doi: 10.3389/fenvs.2022.880807
Received: 21 February 2022; Accepted: 07 April 2022;
Published: 26 May 2022.
Edited by:
Mazhar Iqbal Zafar, Quaid-i-Azam University, PakistanReviewed by:
Muhammad Afzal, King Saud University, Saudi ArabiaVineet Kumar, National Environmental Engineering Research Institute (CSIR), India
Muhammad Saqib Nawaz, King Abdullah University of Science and Technology, Saudi Arabia
Copyright © 2022 Aziz, Rasheed, Shah, Nasir, Fariq, Jamil and Jannat. This is an open-access article distributed under the terms of the Creative Commons Attribution License (CC BY). The use, distribution or reproduction in other forums is permitted, provided the original author(s) and the copyright owner(s) are credited and that the original publication in this journal is cited, in accordance with accepted academic practice. No use, distribution or reproduction is permitted which does not comply with these terms.
*Correspondence: Sajida Rasheed, ZHJzYWppZGExNDJAZ21haWwuY29t