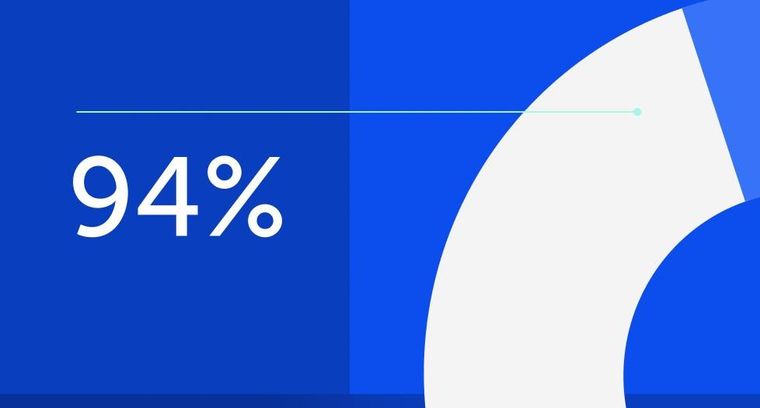
94% of researchers rate our articles as excellent or good
Learn more about the work of our research integrity team to safeguard the quality of each article we publish.
Find out more
ORIGINAL RESEARCH article
Front. Environ. Sci., 13 May 2022
Sec. Toxicology, Pollution and the Environment
Volume 10 - 2022 | https://doi.org/10.3389/fenvs.2022.875512
This article is part of the Research TopicChallenges in Characterizing Nano- to Macro-Plastics and Adhered Substances in the Aquatic EnvironmentView all 7 articles
Contamination by microplastics (particles < 1 mm) is a growing and alarming environmental problem in freshwater systems. Evidence suggests that industrial effluents could be one of the critical point sources of microplastics and other pollutants, and their interaction can cause organismal stress and affect host and environmental microbial communities. We tested the individual and combined effects of microplastics and other pollutants on host survival and host associated (commensal) bacterial diversity. We exposed Daphnia magna to 1 µm microplastic beads with a concentration of approximately 1820 particles/ml and chromium (VI) simultaneously with treatments of 2 and 5 ppm for 72 h. DNA extraction was done to amplify and sequence the ribosomal Bacterial 16S from both the water and the Daphnia. Daphnia experienced low mortality in treatments microplastics (13.3%) and 2 ppm chromium VI (30%) individually. However, the combination of microplastics and 2 ppm chromium (VI) increased the mortality to 74.4%. In the treatments with 5 ppm of chromium (VI) mortality rose to 100% after 30 h of exposure. Microbial diversity changed in response to microplastics, chromium (VI), and both combined exposure. Microplastics and toxic metals can cause dysbiosis of freshwater environmental microbiota, whole host microbiota, and host survival. This work stresses the importance to assess how pollutants’ individual and joint effects could affect organisms including their microbiome.
The small plastic fragments, beads, granules and fibers below 1 mm in diameter are specifically called microplastics (MPs here after) (Santos et al., 2009; Cole et al., 2015; Silva et al., 2019). MPs can be specifically manufactured (Napper et al., 2015; Cole et al., 2016) or they are the consequence of mechanical, biological or photodegradation and subsequent fragmentation of large plastic items (Ma et al., 2016; de Sá et al., 2018; Athey et al., 2020). One of the major concerns about MPs is that they are highly bioavailable in marine environments, fresh water and terrestial ecosystems (Cole et al., 2014; Huerta Lwanga et al., 2016; Wagner and Lambert 2018). In fact, a large variety of organism has been reported to ingest and accumulate MPs, from zooplankton to fish (Santos et al., 2009; Cole et al., 2015; Mattsson et al., 2015). MPs can have a negative effect on organisms, compromising the individual fitness by reducing feeding, energetic reserves, growth rate, fecundity, and survival (Cole et al., 2016; Huerta Lwanga et al., 2016; Jeong et al., 2016; Foley et al., 2018). Moreover, the bioavailability of MPs and their possible interactions with different pollutants in the environment (Rochman et al., 2014), might open the possibility of synergic toxic action of MPs with other pollutants such as toxics metals. The synergic effects of MPs and metal ions on organism fitness and its associated microbes remain mostly unexplored, constituting one of the new frontiers in toxicology.
Toxic metal ions such as hexavalent chromium [hereafter, Cr (VI)] are known to be highly carcinogenic, nephrotoxic, hepatotoxic, neurotoxic, teratogenic, and mutagenic (Zeng et al., 2016; Bojarski et al., 2021; Guo et al., 2021). Compared with organic pollutants, metal ions cannot be biodegraded into more harmless compounds. Chromium in aquatic environments exist in two different states, trivalent chromium [Cr (III)] and Cr (VI), which is more harmful to human health (Barnhart 1997; Khezami and Capart 2005; Guo et al., 2021). Many industrial processes release Cr (VI) in aquatic environments, including tanning, electroplate manufacturing of dye, metal finishing, and production of paint and paper (Pérez-Candela et al., 1995; Vargas and Dussán 2016; Guo et al., 2021). The strong oxidizing property and the ability of Cr (VI) to cross biological membranes is a threat to many organisms living in polluted environments, environmental microorganisms, and host associated microbes (Babich et al., 1980; Breton et al., 2013; Hose et al., 2016; Assefa and Köhler 2020; Bojarski et al., 2021).
Host associated microbes play a role in host immune response, development and function of vital organs (e.g., the brain), and production of key elements (e.g., vitamin B12) (Bennett et al., 2015; Marchesi et al., 2015; Bharwani et al., 2016). Environmental anthropogenic pollutants can negatively affect host physiology, host microbiome, and multiple metabolic pathway because these chemical can act as carcinogens, endocrine disruptors and neurotoxins (Assefa and Köhler 2020; Häder et al., 2020). For example, toxic metals can induce structural damage in microorganisms, direct damage on biological membranes, deplete antioxidants and cause protein dysfunction via oxidation (Babich et al., 1980; Breton et al., 2013; Lemire et al., 2013; Assefa and Köhler 2020). Therefore, exposure to Cr (VI) in host-microbiome is likely to cause dysbiosis affecting host functions (Assefa and Köhler 2020). Similarly, MP ingestion can also have possible stressful effects on the host and in its associated microorganisms (Aljaibachi and Callaghan 2018; Jin et al., 2019; Silva et al., 2019; Wang et al., 2019). Recent studies showed that MPs can be colonized and be used as substrate for some microorganism in the environment (Didier et al., 2017; Jacquin et al., 2019). In this way, ingesting MPs could result in a new resource for members outside of the autochthonous gut microbial communities, affecting bacterial gut colonization in the gut environment. Therefore, the effects on the host responses and host metabolism changed by external stress factors on the microbiota cannot be ignored.
In this study, we tested the effect of microplastics and toxic metals on Daphnia magna mortality and the changes in the composition of free-living and host associated microorganism in the presence and absence of MPs and Cr (VI). It has been shown that plastic can both enhance the effects of other micropollutants such as toxic metals, and enhance the growth of new bacterial strains due to the use of MPs as carbon source (Zettler et al., 2013; Rochman et al., 2014; Horton et al., 2018; Felten et al., 2020). Therefore we hypothesize a additive effect where mortality of D. magna will increase due to the combination of MPs and Cr (VI). We also hypothesize that the microbial communities in the water and in the host will change in response to the presence of MPs. In the treatments with Cr (VI) we expect mortality close to the 50% of the population because the concentrations used in this experiment are lower than the LC50 of D. magna and other water invertebrates (Kim et al., 2002; Vargas and Dussán 2016; Zhou et al., 2021). As Cr (VI) is a very toxic pollutant we expected a decrease in microbial diversity.
River water was collected from River Fyrisån, Uppsala Sweden (59°50′31.3″N 17°39′22.9″E). The water was collected in 20 L plastics containers from the river at both 50 cm and 3 m depth using a ruttner sampler near the shore on both sides of the river. The plastics containers and other intruments were acid-washed and/or rinsed meticulously with MilliQ prior to use. The water was filtered with 165 µm mesh to remove phytoplankton, zooplankton and other big particles. In the lab the water from the two depths was mixed and filtered again with a 47 mm binder free glass fiber filter for further use.
D. magna were grown in dechlorinated tap water in a 70 L tank in aerated water. Temperature was 20 ± 1˚C, and photoperiod was 16 h L: 8 h D. They were fed with Raphidocelis subcapitata algae. The algae were grown in Modified Wright’s Cryptophyte MWC medium (Guillard and Lorenzen 1972), This laboratory cultured D. magna population had been kept for 5 years and was used as model organism in the experiments.
The study was carried out at room temperature (20°C ± 1) in glass vessels with a 12 h L: 12 h D photoperiod. The effect of MPs and Cr (VI) were tested in a fully factorial two-way design with two levels of MPs (with or without) and three levels of Cr (VI) (0 ppm, 2 and 5 ppm) resulting in six treatments that were replicated 5 times (Supplementary Figure S1). To perform the experiment, 165 ml glass vessels were used containing 110 ml of river water with 20 individuals of Daphnia in each replicate. The water was collected downstream Uppsala river near to the water treatment plant. Even if the contamination might be higher at this point in the river the water there is relatively clean. We decided to work with water that is closer to human activity to have more realistic conditions. Therefore we decided to work with a very low concentration of MPs. The MPs treatment had a concentration of 1820 particles/mL (1 × 10−9 g/ml) of 1 µm microplastic polystyrene-based latex spheres Fluoresbrite® Yellow Green Microspheres (CAS# 0009003536, Polysciences, Inc.) diluted in fresh water with a total volume of 110 ml. This concentration is way lower than what has been previously reported, and similar or lower to what has been found in contaminated rivers (Klein et al., 2015; Mani et al., 2015; Lu et al., 2018; Jin et al., 2019; Costa et al., 2020; Varg et al., 2021). MPs were centrifuged at 6,000 rpm and resuspended in freshwater to remove the leached dye in the solution that could have an extra effect not related to the MPs. The MPs came packed with deionized water only. We used those particles because the have been used as biomarkers (Rillig and Bonkowski 2018). Other reports suggested toxicity from additives such as surfactants instead of the microplastics themselves (Heinlaan et al., 2020). In our case, the particles are inert and non-toxic, as additional compounds such as surfactants do not accompany the MPs. The concentrations of Cr (VI) treatments (2 and 5 ppm) where chosen base on previous studies of Cr (VI) in aquatic invertebrates (Kühn et al., 1989; Kim et al., 2002; Vargas and Dussán 2016). Analytical grade K2CrO4 (Sigma-Aldrich) was used to archive the final concentration of 2 and 5 ppm in a total volume of 110 ml of river water. The combined Cr (VI) 2 ppm-MPs and Cr (VI) 5 ppm- MPs treatments consist on the aforementioned concentration of Cr (VI) and a concentration of 1,820 particles/ml of 1 µm of microplastic. Before placing the D. magna in the treatment vessel, 10 ml of the experimental solution was remove from the vessel and stored for determination of the Cr (VI) concentration leaving a total of 100 ml of treatment solution vessel. The experiment lasted 72 h and one daphnia was sacrificed from each treatment at hour 40 for sequencing of the microbiome. At the end of the experiment, the water from each treatment was filtered with 0.2 µm filter for further microbial analysis. Mortality was checked at 12, 18, 24, 28, 30, 40, 52, 66 and 72 h.
DNA was extracted from the whole daphnia samples after being rinsed with Milli Q water to remove the microbes coming from the water. DNA was also extracted from the 0.2 µm filters to obtain only DNA of the water microbiota. In both cases the DNA was extracted using DNeasy Powersoil (Qiagen, No./ID: 12888-10) as recommended in the manufacture’s protocol with an additional incubation at 65°C for 10 min after adding the C1 solution and additional 30 min of the bead homogenizer step. The 16S ribosomal RNA gene was amplified in a two-step PCR using primer pair 515F and 805R that flanks the hypervariable region V4. For the first step, PCRs were performed in triplicate using Phusion High-Fidelity DNA polymerase (Thermo Fisher Scientific, No./ID: F-530XL). Thirty cycles were performed following Phusion polymerase protocol. Negative controls were run during DNA extraction and 16S PCR amplification to check for contamination. Triplicate PCR products of each sample were pooled and subsequently purified using AMPure XP magnetic beads for Purification (Beckman Coulter, No/ID: A63882). For the second step, Illumina adaptor sequences and barcodes were attached to the PCR primers to provide each sample with a unique identifier. Samples were then purified again using magnetic beads, and the DNA concentration of each samples was measured using Quant-iT PicoGreen dsDNA Assay kit (Thermo Fisher Scientific, No/ID: P7589). An equal concentration of DNA from each sample was pooled and run through agarose gel. Then, 400–500 bp band was excised and purified using the QIAquick gel extraction kit (Qiagen, No/ID: 28104). PCR products were sequenced on IlluminaMiSeq to obtain 250 bp paired-end reads at Science for Life Laboratory (SciLifeLab, Uppsala, Sweden).
The effects of the different exposure treatments on the D. magna mortality at 12, 18, 24, 28, 30, 40, 52, 66 and 72 h were analyzed using Generalized Least Squares fit model (GLS). Percentage mortality of D. magna was entered as response, time was entered as a covariate, and the exposures to MPs (two level factor: presence/absence) and Cr (VI) (three level factor 0, 2, 5 ppm) were entered as fixed factors. Finally, to account for repeated measurements an autocorrelation structure of order one was entered, with time as a continuous covariate and D. magna vessel as a grouping effect. The model was performed using the packages nlme (Pinheiro et al., 2020) for R statistical Computing Language 3.6.2. Additionally, two quasipoisson Generalized Linear Model (GLM) was used to test the mortality due to the exposure to MPs and Cr (VI) in the individual times 40 and 72 h. Post-hoc test were performed to in all cases to observe differences between treatments using the functions emtrends and emmeans of the R package emmeans (Lenth 2020).
The amplicon sequence variant (ASV) Table from the D. magna mirobiome was created using Demultiplexed data from the SciLifeLab using the R package DADA2 and following the pipeline 1.8 (Callahan et al., 2016). Taxa diversity and the relative abundant phyla were calculated using the R package lattice (Sarkar, 2008) and MASS (Venables and Ripley, 2002). A phylogenetic, Shanon and Chao diversity analysis were performed to obtain ASV Abundance and ASV abundance and evenness as alpha diversity measurements using the R packages fossil (Vavrek 2011), vegan (Oksanen et al., 2018), ape (Paradis and Schliep 2019) and picante (Kembel et al., 2010). To test the effect of the treatments on the microbiome, linear models were carried out using the diversity indexes as response variable and presence/absence of MPs (2 level factor) and Cr (VI) (3 level factor). Daphnia’s phylogenetic and Chao indexes were log 10 transformed to meet the requirements of the test. A post-hoc test was carried out for pairwise comparisons using emmeans R package. Moreover, a Principal Coordinates Analysis (PCoA) was performed to visualize the beta diversity among treatments. The effect of treatments were then tested with a Permutational Multivariate Analysis of Variance Using Distance Matrices (ADONIS) using the vegan package in R (Oksanen et al., 2018), the ASV Bray-Curtis distance matrix was used as response variable and MPs and Cr (VI) as fixed factors. To investigate changes in the four main microbial phyla a Multivariate Analysis of Variance (MANOVA) was performed using relative abundance of the four main phyla as response variable and MPs and Cr (VI) as fixed factors. To Observe the univariate changes of the four main phyla Generalized linear models (GLM) were performed using the relative abundance of each phyla as response variable and MPs and Cr (VI) as fixed factors. All the statistical analyses were executed in R statistical Computing Language 3.6.2 (R Core Team 2020). The phylogenetic tree, and the taxonomy plots were created using Qiime 1.9.9 (Caporaso et al., 2012).
10 ml of the solution in each treatment and replicate was used to measure the Cr (VI) concentration at the beginning and at the end of the experiment using a Metrohm ion chromatography (IC) system (883 Basic IC Plus and 919 Autosampler Plus). The IC was set as follow: Metrosep A Supp 4/5-guard column and a Metrosep A Supp 5 analytical column (150 × 4.0 mm). The separations were performed using a carbonate eluent (3.2 mM Na2CO3 + 1.0 mM NaHCO3) and a flow rate of 0.7 ml/min.
The control treatments and the MPs treatment showed similar mean mortality rates (13.3 and 14.4%, respectively), at the end of the experimental time. Daphnia mortality was 100% after 30 h in both 5 ppm Cr (VI) treatments [5 ppm Cr (VI) alone and MPs + 5 ppm Cr (VI)], with both having similar trends over time (Figure 1). In the time 40 h we observed that the treatments of 5 ppm Cr (VI) and MPs + 5 ppm Cr (VI) have high mortality mainly driven by Cr (VI) (Table 1; Figure 1). The post hoc test showed that all treatments that contain 5 ppm Cr (VI) were different from the other treatments but not between each other. At 72 h, the mean mortality of Daphnia in 2 ppm Cr (VI) concentration was 40% while MPs + 2 ppm Cr (VI) showed a mean mortality of 74.4%. The GLS model that tested the effects of the MPs and Cr (VI) across time showed that Cr (VI) and time significantly affect the Daphnia mortality. The two-way interaction of MPs and time, and Cr (VI) and time are also significant but there is no significant interaction between MPs and Cr (VI) (Table 1). The post hoc test for the aforementioned model showed that there were significant differences between select treatments (Supplementary Table S1). However, the model that tested only at time 72 h showed significant effects in the mortality of Daphnia for all the factors: MPs, Cr (VI) and the two way interaction MPs + Cr (VI) (Table 1; Figure 1). The contrast of the post hoc test at 72 h showed significant differences between all the treatments except for the control and MPs, MPs combined with 2 ppm of Cr (VI) and 5 ppm of Cr (VI) alone and with MPs, and MPs combined with 5 ppm of Cr (VI) and 5 ppm of Cr (VI). To see the post hoc tests, see Supplementary Tables S1–S3.
FIGURE 1. Mortality of D. Magna exposed to MPs, two different concentration of Cr (VI), and the combined treatment of MPs with both 2 and 5 ppm of Cr (VI) over the course of 72 h. The control treatment were not exposed to either MP or any concentration of Cr (VI).
TABLE 1. Results for the models testing the effects of exposure to MPs, and to Cr (VI) and their interaction on the mortality of D. magna. The first model is a Generalized Least Squares fitted linear model (GLS) that includes D magna ID as random effects and MPs, Cr (VI) and Time as a fixed factor. The second and third models are Generalized Linear Model (GLM) as a subset of the first model taking into account only the time 40 and 72 h, respectively. Significant and marginally non-significant p-values are highlighted in bold.
The sequencing generated an average of 31091 reads per sample and 1834401 reads in total. The microbiome in the daphnia and in the water shows four main phyla in order of abundance: Proteobacteria, Bacteroidetes, Actinobacteria, and Verrucomicrobia for the daphnia and Bacteroidetes, Proteobacteria, Actinobacteria, and Tenericutes for the water (Supplementary Figure S2).
Alpha diversity (Chao, Shannon, as well as phylogenetic diversity), in both water and in Daphnia decreased in the presence of Cr (VI) (Figure 2; Table 2). The pairwise comparison of the post hoc test in the daphnia showed only significant differences in the phylogenetic diversity between the treatments (Figure 2, Supplementary Table S4). On the other hand, in the water the post hoc test did not show significant differences in the phylogenetics diversity but in the Chao and Shannon diversity: (Figure 2, Supplementary Table S4)
FIGURE 2. Changes in microbial diversity indexes, in Daphnia (left panel) and in the water (right panel), measured as Chao index (A,B), Shannon index (C,D), Phylogenetic diversity index (E,F), to the exposure to MP and/or Cr (VI). The control replicates were not exposed to either MP or Cr (VI). Error bars represent standard error. The violin shape represents the kernel density, showing the probability density of the data. Significant differences between treatments were tested using post-hoc Tukey tests and are indicated with asterisks (∗∗∗: p-value < 0.001; ∗∗: 0.001 < p-value < 0.01; ∗: p-value < 0.05; •: 0.05 < p-value < 0.09).
TABLE 2. Results of the model testing effects of exposure to MPs as a binomial factor (presence-absence), exposure to Cr (VI) as a three level factor (0, 2 and 5 ppm), and the two-way interaction on the diversity indexes (Chao, Shannon and Phylogenetic). The results for the PERMANOVA of the microbial composition are also included at the end of the table. Significant and marginally non-significant p-values are highlighted in bold.
The Daphnia microbiome showed separation in four apparent clusters 1) for the control, 2) for the MPs, 3) for the treatments with 2 ppm Cr (VI) and 4) for the treatments with 5 ppm Cr (VI) (Figure 3). The Daphnia microbial beta diversity was significantly affected by the exposure to Cr (VI), and by the two way interaction of MP × Cr (VI). MP showed a marginal non-significant effect on beta-diversity of the Daphnia microbiome (Table 2). In the water the microbiome showed two clusters 1) for the control and MPs and 2) for all the rest of the treatments that contain Cr (VI) (Figure 3). The water beta diversity was significantly affected only by the Cr (VI) exposure (Table 2).
FIGURE 3. Principal Coordinates Analysis showing the clusters of microbial composition in the Daphnia (A) and in the water (B). The microbial composition is clustered according to experimental treatment.
The main microbial phyla in Daphnia was significantly affected by MP, Cr (VI) and the interaction between MP × Cr (VI) (Table 3). On the other hand the four main microbial phyla in the water were only affected by the exposure of Cr (VI) (Table 3). Looking at the individual microbial phyla in Daphnia; the relative abundance of Proteobacteria increased in the presence of Cr (VI) whereas Bacteroidetes was affected both by the exposure to Cr (VI) and by the interaction of MP × Cr (VI) (Table 4). There was also a non-significant effect from MP exposure on Bacteroidetes. Both Actinobacteria and Verrucomicrobia relative abundances was negatively affected by the exposure of MPs alone and Cr (VI) alone (Table 4). The post hoc test showed differences between treatments in all the four main phyla (Figure 4). In the water, only Cr (VI) exposure affected Bacteroidetes, Proteobacteria and Actinobacteria whereas Tenericutes was not affected by any of the factors (Table 4). The post hoc showed significant differences between treatments only in Bacteroidetes and Proteobacteria (Figure 5).
TABLE 3. Results of the model testing effects of exposure to MPs as a binomial factor (presence-absence), exposure to Cr(VI) as a three level factor (0, 2 and 5 ppm), and the two-way interaction on the relative abundance of the four main microbiota phyla in the Daphnia and in the water (MANOVA). Significant and marginally non-significant p-values are highlighted in bold.
TABLE 4. Results of the model testing effects of exposure to MPs as a binomial factor (presence-absence), exposure to Cr(VI) as a three level factor (0, 2 and 5 ppm), and the two-way interaction on the relative abundance of the individual four main microbiota phyla. Significant and marginally non-significant p-values are highlighted in bold.
FIGURE 4. Relative abundance of the four top phyla in the Daphnia microbiome, Proteobacteria (A), Bacteroidetes (B), Actinobacteria (C), and Verrucomicrobia (D) for the different experimental treatments. Error bars represent standard error. The violin shape represents the kernel density, showing the probability density of the data. Significant differences between treatments were tested using post-hoc Tukey tests and are indicated with asterisks (∗∗∗: p-value < 0.001; ∗∗: 0.001 < p-value < 0.01; ∗: p-value <0.05; •: 0.05 < p-value < 0.09).
FIGURE 5. Relative abundance of the four top phyla in the wáter microbiome, Proteobacteria (A), Bacteroidetes (B), Actinobacteria(C), and Verrucomicrobia(D) for the different experimental treatments. Error bars represent standard error. The violin shape represents the kernel density, showing the probability density of the data. Significant differences between treatments were tested using post-hoc Tukey tests and are indicated with asterisks (∗∗∗: p-value < 0.001; ∗∗: 0.001< p-value < 0.01; ∗: p-value < 0.05; •: 0.05< p-value < 0.09).
The concentration of Cr (VI) in the treatments with Cr (VI) alone decreased between an average of 1.8% for the 2 ppm Cr (VI) treatment and an average of 5.6% for the 5 ppm Cr (VI) treatment. When the MPs are in the solution with Cr (VI) there is an apparent increase of Cr (VI) of an average of 16.4% for the MPs + 2 ppm Cr (VI) treatment and 11.1% for the MPs + 5 ppm Cr (VI) treatment (Figure 6).
FIGURE 6. Residual Cr (VI) concentration in each treatment. Cr (VI) was measured at the beginning and at the end of the experiment and then subtracted to obtain the residual concentration of Cr (VI). The treatments Control and MPs were also measured but Cr (VI) was not found in the samples as expected. Error bars represent standard deviation.
We show here that effects of the polystyrene-based latex sphere MPs on the mortality of D. magna are low and similar to the control treatments. This is not surprising because MPs depending on the material can be inert particles that do not have a direct toxic effect (Cole et al., 2015; Carbery et al., 2018; Wagner and Lambert 2018). However it has been found previously that MPs can have negative effects on different life history traits of D. magna (Ma et al., 2016; Rist et al., 2017; Felten et al., 2020). For example, Aljaibachi and Callaghan (2018) showed that MPs affects reproduction and mortality depending on food availability. Nevertheless, the effects that the MPs might have depend highly on the MPs material (Ma et al., 2016; Aljaibachi and Callaghan 2018; Lei et al., 2018). For example, Lei et al. (2018) found that polyvinyl chloride MPs have direct toxic effects on Zebra fish. Another important factor that might contribute to the negative effects of MPs is the size of the MPs. Ma et al. (2016) found that nano-plastics tend to be more harmful than MPs to D. magna. In the same way, the shape can also influence the negative effect of the MPs. In our experiment we use 1 μm MP with very defined spherical shape that could explain the low mortality that we had in our experiment. However we cannot discard the possibility that spherical MPs could cause some physical damage. MPs with more needle-like shapes could attach easily to internal and external surfaces. Depending on the size and shape MPs could generate physical irritation as indirect effects. Smaller angular shapes could be more difficult to dislodge than smooth spherical shapes blocking important organs such as gills and the digestive track (Wagner and Lambert 2018).
The toxicity of Cr (VI) have been highly studied (Barnhart 1997; Kim et al., 2002; Hose et al., 2016; Bojarski et al., 2021). The mortality from Cr (VI) that we found in this experiment is in line with other studies on aquatic invertebrates (Kim et al., 2002; Vargas and Dussán 2016; Zhou et al., 2021). It is interesting to see how 5 ppm of Cr (VI) resulted in 100% mortality of D. magna at 40 h while 2 ppm of Cr (VI) resulted in almost no mortality for that time. But highly increase few hours after showing the effect that a chronic exposure of a pollutant such as Cr (VI) can have (Zhou et al., 2021). Similar effects of 2 ppm of Cr (VI) in a different organism have been reported before in another study that used sublethal concentration of Cr (VI) to test the activity of a surface bacterial protein in Cr (VI) adsorption and in the mortality of mosquito born disease larvae (Vargas and Dussán 2016).
We showed here that MPs can enhance the negative effect of sublethal concentration of Cr (VI). At 5 ppm of Cr (VI) the concentration of Cr (VI) was so high that it was hiding the effects that the MPs might have when combined with Cr (VI). However, for the 2 ppm concentration of Cr (VI), the synergistic effect of MP and CR (VI) became clear, especially at 72 h. Similar effects with other micropollutants have been reported before. For example Felten et al. (2020) showed that MPs increase the negative effect of a pyrethroid pesticide in D. magna. This agrees with the known interaction between MPs and hydrophobic organic chemicals (HOCs) such as pyrethroids, making HOCs more bioavailable, working as a vector for HOCs to further ingestion (Rochman et al., 2014). The interactions and the effects that the MPs might have depend on the chemical/toxicant that the MPs are interacting with (Wagner and Lambert 2018; Felten et al., 2020). Here we show that the association of polystyrene-based latex sphere MPs with metal ions such are Cr (VI) can highly increase Cr (VI) toxicity on D. magna. Even though unchanged and cleaning effects (i.e., MPs adsorbing toxicant and consequently reducing exposure) have been reported before. These studies that have been focused on HOCs (Koelmans et al., 2013; Horton et al., 2018; Liu et al., 2020; Menéndez-Pedriza and Jaumot 2020), might explain the observed phenomena of Cr(VI) increment in the presence of MPs. In this scenario, MPs and D. magna works as adsorbent up taking Cr(VI) that could not be measured at the beginning of the experiment. In this way, Cr(VI) could be released after achieving the maximum adsorption capacity as part of the adsorption-desorption process and be measured at the end of the experiment (Gupta and Rastogi 2008; Janmohammadi et al., 2021). Studies on MPs and metals are scarce and this would need more attention before any conclusions could be drawn.
Studies of host-microbiota exposed to toxic metals are scarce but this exposure is likely to exert an unbalance on the resident microbial taxa in the host (Breton et al., 2013; Richardson et al., 2018; Assefa and Köhler 2020). In our experiment is clear that the microbial alfa diversity of Daphnia was mainly affected by the Cr(VI) exposure. This effects can be explained by that Cr(VI) can change the micro environmental conditions such as oxygen availability, redox potential leading to a changes in microbial communities to more Cr(VI) resistance bacteria (Assefa and Köhler 2020). However, a previous study testing the effects of toxic metals in gut microbiome found that chromium had a weak impact on the gut microbiome, especially compared to other metals such as arsenic, cadmium, and nickel but still impacting the host physiology (Richardson et al., 2018). Our result show that both host mortality and microbiome are highly affected by the exposure of Cr(VI). The differences between the study of Richardson et al. (2018) and our experiment can be a result of the intrinsic differences between hosts. In our case we work with wild aquatic invertebrate, D. magna, while they work with laboratory raised rats. Another possibility for the difference is that the chromium oxidation state used in their experiment is different compared to the Cr(VI) used in our study. This might result in lower toxicity as well as lower changes in the host-microbiome. Surprisingly, we did not find significant changes in the alpha microbial diversity to the exposure of MPs. Previous reports have found changes in the alpha microbial diversity in response to MPs exposure on free living microorganism (Zettler et al., 2013; Jin et al., 2019). However, we did find significant changes in microbial beta diversity to the exposure of MPs, Cr(VI) and the combined exposure of MPs + Cr(VI). This indicates that the overall microbial community is changing in response to the effect of MPs and/or Cr(VI). Similarly, we also found drastic changes in the relative abundance of the main phyla. These changes in each phylum can be due to the increase of bacteria with resistance mechanism to tolerate Cr(VI) or with the ability to use MPs as a carbon source. For example, the phylum Verrucomicrobia increase in the presence of MPs but decrease in presence of Cr(VI). Therefore, the removal or retention of properties in the microbiota might be highly influenced by the pollutant itself (Breton et al., 2013), however we should not discard the selection pressures that are exert by the host to maintain certain bacterial properties (Foster et al., 2017). In this way both host and pollutant might exert selective pressure on the microbial communities.
The water microbiome showed effects only to the exposure of Cr(VI). As mentioned before, Cr(VI) is highly toxic and can have huge influence on the bacterial phyla composition (Breton et al., 2013). Free-living bacteria have been differentiated from bacteria that is forming a biofilm on plastics (Didier et al., 2017; Jacquin et al., 2019). However, we did not observe any significant changes in the water microbiome to the exposure of MP. The lack of these effects can be related to the microbes that were originally in the water where we used river water collected next to the treatments plant effluents that have some degree of pollution. Even if this pollution is low, it might be that the microbes in the water were already able to colonize or degrade MPs decreasing the impact of the MPs disturbance in the water microbiome.
In summary, we tested direct mortality effects of 1 µm polystyrene-based latex sphere MPs, Cr(VI), and the combination of MPs and Cr(VI) in D. magna as well as the effects on the Daphnia microbiome and the water microbiome. We found that a synergistic interaction between MPs and Cr(VI) affecting the Daphnia mortality. MPs and Cr(VI) also influenced host microbial community composition. The results provide novel insights related to pollutant effects directly on mortality and on host-microbiome. We suggest that future studies should be focused on the effects of metal co-ions interactions together with MPs as well as the effects on the functional content of host-microbiome and its changes due to pollutant exposure, through metatranscriptomics tools. Therefore in the future, we hope that host-microbiome and the interaction with anthropogenic pollutants can be studied at the functional level. In this way for example it will be possible to test if the changes of bacterial taxa are related to loss of function or if the host is still keeping bacteria with similar traits and with the ability to tolerate the pollutant in the environment.
The datasets presented in this study can be found in online repositories. The names of the repository/repositories and accession number(s) can be found below: https://www.ebi.ac.uk/ena, PRJEB45679.
JV Conceived the project and designed the experiment. JV Performed the experiment as well as the DNA extraction and DNA library preparation. CB and JV Performed the IC measurements. JV Performed the microbime analyses and the statistical analyses with advise from RS. JV wrote the paper. CB and RS Contributed and approved to the final version of the manuscript.
Financial support for this study came from Linnéska stipendiestilftelsen and the Department of Ecology and Genetics.
We confirm that this manuscript has not been published elsewhere and is not under consideration by another journal. All authors have approved the manuscript and agree with submission to Frontiers in Environmental Science. We declare no conflict of interest associated with this publication.
All claims expressed in this article are solely those of the authors and do not necessarily represent those of their affiliated organizations, or those of the publisher, the editors and the reviewers. Any product that may be evaluated in this article, or claim that may be made by its manufacturer, is not guaranteed or endorsed by the publisher.
We want to thank Christoffer Mattson, and David Outomuro for their invaluable help with the water collection and microcosm set up. Sequencing was performed by the SNP and SEQ Technology Platform in Uppsala. The facility is part of the National Genomics Infrastructure (NGI) Sweden and Science for Life Laboratory. The SNP and SEQ Platform is also supported by the Swedish Research Council and the Knut and Alice Wallenberg Foundation.
The Supplementary Material for this article can be found online at: https://www.frontiersin.org/articles/10.3389/fenvs.2022.875512/full#supplementary-material
Aljaibachi, R., and Callaghan, A. (20182018). Impact of Polystyrene Microplastics on Daphnia Magna Mortality and Reproduction in Relation to Food Availability. PeerJ.
Assefa, S., and Köhler, G. (2020). Intestinal Microbiome and Metal Toxicity. Curr. Opin. Toxicol. 19, 21–27. doi:10.1016/j.cotox.2019.09.009
Athey, S. N., Albotra, S. D., Gordon, C. A., Monteleone, B., Seaton, P., Andrady, A. L., et al. (2020). Trophic Transfer of Microplastics in an Estuarine Food Chain and the Effects of a Sorbed Legacy Pollutant. Limnol. Oceanogr. Lett. 5, 154–162. doi:10.1002/lol2.10130
Babich, H., Stotzky, G., and Ehrlich, H. L. (1980). Environmental Factors that Influence the Toxicity of Heavy Metal and Gaseous Pollutants to Microorganisms. CRC Crit. Rev. Microbiol. 8, 99–145. doi:10.3109/10408418009081123
Barnhart, J. (1997). Occurrences, Uses, and Properties of Chromium. Regul. Toxicol. Pharmacol. 26, S3–S7. doi:10.1006/rtph.1997.1132
Bennett, B. J., Hall, K. D., Hu, F. B., Mccartney, A. L., and Roberto, C. (2015). Nutrition and the Science of Disease Prevention: A Systems Approach to Support Metabolic Health. Ann. N.Y. Acad. Sci. 1352, 1–12. doi:10.1111/nyas.12945
Bharwani, A., Mian, M. F., Foster, J. A., Surette, M. G., Bienenstock, J., and Forsythe, P. (2016). Structural & Functional Consequences of Chronic Psychosocial Stress on the Microbiome & Host. Psychoneuroendocrinology 63, 217–227. doi:10.1016/j.psyneuen.2015.10.001
Bojarski, B., Buchko, O., Kondera, E., Ługowska, K., Osikowski, A., Trela, M., et al. (2021). Effects of Embryonic Exposure to Chromium (VI) on Blood Parameters and Liver Microstructure of 1-Day-Old Chickens. Poult. Sci. 100, 366–371. doi:10.1016/j.psj.2020.10.016
Breton, J., Daniel, C., Dewulf, J., Pothion, S., Froux, N., Sauty, M., et al. (2013). Gut Microbiota Limits Heavy Metals Burden Caused by Chronic Oral Exposure. Toxicol. Lett. 222, 132–138. doi:10.1016/j.toxlet.2013.07.021
Callahan, B. J., Mcmurdie, P. J., Rosen, M. J., and Han, A. W. and (2016). Dada2. Nat. Methods 13, 581–583. doi:10.1038/nmeth.3869
Caporaso, J. G., Lauber, C. L., Walters, W. A., Berg-Lyons, D., Huntley, J., Fierer, N., et al. (2012). Ultra-high-throughput Microbial Community Analysis on the Illumina HiSeq and MiSeq Platforms. ISME J. 6, 1621–1624. doi:10.1038/ismej.2012.8
Carbery, M., O’Connor, W., and Palanisami, T. (2018). Trophic Transfer of Microplastics and Mixed Contaminants in the Marine Food Web and Implications for Human Health. Environ. Int. 115, 400–409. doi:10.1016/j.envint.2018.03.007
Cole, M., Lindeque, P., Fileman, E., Halsband, C., and Galloway, T. S. (2015). The Impact of Polystyrene Microplastics on Feeding, Function and Fecundity in the Marine Copepod Calanus helgolandicus.
Cole, M., Lindeque, P. K., Fileman, E., Clark, J., Lewis, C., Halsband, C., et al. (2016). Microplastics Alter the Properties and Sinking Rates of Zooplankton Faecal Pellets.
Cole, M., Webb, H., Lindeque, P. K., Fileman, E. S., Halsband, C., and Galloway, T. S. (2014). Isolation of Microplastics in Biota-Rich Seawater Samples and Marine Organisms. Sci. Rep. 4, 1–8. doi:10.1038/srep04528
Costa, E., Piazza, V., Lavorano, S., Faimali, M., Garaventa, F., and Gambardella, C. (2020). Trophic Transfer of Microplastics from Copepods to Jellyfish in the Marine Environment. Front. Environ. Sci. 8, 1–7. doi:10.3389/fenvs.2020.571732
de, Sá., Oliveira, M., Ribeiro, F., Rocha, T. L., and Futter, M. N. (2018). Studies of the Effects of Microplastics on Aquatic Organisms: What Do We Know and where Should We Focus Our Efforts in the Future? Sci. Total Environ. 645, 1029–1039. doi:10.1016/j.scitotenv.2018.07.207
Didier, D., Anne, M., and Alexandra, T. H. (2017). Plastics in the North Atlantic Garbage Patch: A Boat-Microbe for Hitchhikers and Plastic Degraders. Sci. Total Environ. 599600, 1222–1232.
Felten, V., Toumi, H., Masfaraud, J. F., Billoir, E., Camara, B. I., and Férard, J. F. (2020). Microplastics Enhance Daphnia Magna Sensitivity to the Pyrethroid Insecticide Deltamethrin: Effects on Life History Traits. Sci. Total Environ. 714, 136567. doi:10.1016/j.scitotenv.2020.136567
Foley, C. J., Feiner, Z. S., Malinich, T. D., and Höök, T. O. (2018). A Meta-Analysis of the Effects of Exposure to Microplastics on Fish and Aquatic Invertebrates. Sci. Total Environ. 631–632, 550–559. doi:10.1016/j.scitotenv.2018.03.046
Foster, K. R., Schluter, J., Coyte, K. Z., and Rakoff-Nahoum, S. (2017). The Evolution of the Host Microbiome as an Ecosystem on a Leash. Nature 548, 43–51. doi:10.1038/nature23292
Guillard, R. R., and Lorenzen, C. J. (1972). Yellow‐green Algae with Chlorophyllide C1, 2. J. Phycol. 8, 10–14. doi:10.1111/j.0022-3646.1972.00010.x
Guo, C., Ding, L., Jin, X., Zhang, H., and Zhang, D. (2021). Application of Response Surface Methodology to Optimize Chromium (VI) Removal from Aqueous Solution by Cassava Sludge-Based Activated Carbon. J. Environ. Chem. Eng. 9, 104785. doi:10.1016/j.jece.2020.104785
Gupta, V. K., and Rastogi, A. (2008). Sorption and Desorption Studies of Chromium(VI) from Nonviable Cyanobacterium Nostoc Muscorum Biomass. J. Hazard. Mater. 154, 347–354. doi:10.1016/j.jhazmat.2007.10.032
Häder, D. P., Banaszak, A. T., Villafañe, V. E., Narvarte, M. A., González, R. A., and Helbling, E. W. (2020). Anthropogenic Pollution of Aquatic Ecosystems: Emerging Problems with Global Implications. Sci. Total Environ. 713, 136586. doi:10.1016/j.scitotenv.2020.136586
Heinlaan, M., Kasemets, K., Aruoja, V., Blinova, I., Bondarenko, O., Lukjanova, A., et al. (2020). Hazard Evaluation of Polystyrene Nanoplastic with Nine Bioassays Did Not Show Particle-specific Acute Toxicity. Sci. Total Environ. 707, 136073. doi:10.1016/j.scitotenv.2019.136073
Horton, A. A., Vijver, M. G., Lahive, E., Spurgeon, D. J., Svendsen, C., Heutink, R., et al. (2018). Acute Toxicity of Organic Pesticides to Daphnia Magna Is Unchanged by Co-exposure to Polystyrene Microplastics. Ecotoxicol. Environ. Saf. 166, 26–34. doi:10.1016/j.ecoenv.2018.09.052
Hose, G. C., Symington, K., Lott, M. J., and Lategan, M. J. (2016). The Toxicity of Arsenic(III), Chromium(VI) and Zinc to Groundwater Copepods. Environ. Sci. Pollut. Res. 23, 18704–18713. doi:10.1007/s11356-016-7046-x
Huerta Lwanga, E., Gertsen, H., Gooren, H., Peters, P., Salánki, T., Van Der Ploeg, M., et al. (2016). Microplastics in the Terrestrial Ecosystem: Implications for Lumbricus Terrestris (Oligochaeta, Lumbricidae). Environ. Sci. Technol. 50, 2685–2691. doi:10.1021/acs.est.5b05478
Jacquin, J., Cheng, J., Odobel, C., Pandin, C., Conan, P., Pujo-Pay, M., et al. (2019). Microbial Ecotoxicology of Marine Plastic Debris: A Review on Colonization and Biodegradation by the “Plastisphere. Front. Microbiol. 10, 1–16. doi:10.3389/fmicb.2019.00865
Janmohammadi, M., Baghdadi, M., Adyel, T. M., and Mehrdadi, N. (2021). Waste Plastic Filter Modified with Polyaniline and Polypyrrole Nanoparticles for Hexavalent Chromium Removal. Sci. Total Environ. 752, 141850. doi:10.1016/j.scitotenv.2020.141850
Jeong, C. B., Won, E. J., Kang, H. M., Lee, M. C., Hwang, D. S., Hwang, U. K., et al. (2016). Microplastic Size-dependent Toxicity, Oxidative Stress Induction, and P-JNK and P-P38 Activation in the Monogonont Rotifer (Brachionus Koreanus). Environ. Sci. Technol. 50, 8849–8857. doi:10.1021/acs.est.6b01441
Jin, Y., Lu, L., Tu, W., Luo, T., and Fu, Z. (2019). Impacts of Polystyrene Microplastic on the Gut Barrier, Microbiota and Metabolism of Mice. Sci. Total Environ. 649, 308–317. doi:10.1016/j.scitotenv.2018.08.353
Kembel, S. W., Cowan, P. D., Helmus, M. R., Cornwell, W. K., Morlon, H., Ackerly, D. D., et al. (2010). Picante: R Tools for Integrating Phylogenies and Ecology. Bioinformatics 26, 1463–1464. doi:10.1093/bioinformatics/btq166
Khezami, L., and Capart, R. (2005). Removal of Chromium(VI) from Aqueous Solution by Activated Carbons: Kinetic and Equilibrium Studies. J. Hazard. Mater. 123, 223–231. doi:10.1016/j.jhazmat.2005.04.012
Kim, S. D., Park, K. S., and Gu, M. B. (2002). Toxicity of Hexavalent Chromium to Daphnia Magna: Influence of Reduction Reaction by Ferrous Iron. J. Hazard. Mater. 93, 155–164. doi:10.1016/s0304-3894(02)00057-2
Klein, S., Worch, E., and Knepper, T. P. (2015). Occurrence and Spatial Distribution of Microplastics in River Shore Sediments of the Rhine-Main Area in Germany. Environ. Sci. Technol. 49, 6070–6076. doi:10.1021/acs.est.5b00492
Koelmans, A. A., Besseling, E., Wegner, A., and Foekema, E. M. (2013). Plastic as a Carrier of POPs to Aquatic Organisms: A Model Analysis. Environ. Sci. Technol. 47, 7812–7820. doi:10.1021/es401169n
Kühn, R., Pattard, M., Pernak, K. D., and Winter, A. (1989). Results of the Harmful Effects of Water Pollutants to Daphnia Magna in the 21 Day Reproduction Test. Water Res. 23, 501–510. doi:10.1016/0043-1354(89)90142-5
Lei, L., Wu, S., Lu, S., Liu, M., Song, Y., Fu, Z., et al. (2018). Microplastic Particles Cause Intestinal Damage and Other Adverse Effects in Zebrafish Danio rerio and Nematode Caenorhabditis elegans. Sci. Total Environ., 6191–6208. doi:10.1016/j.scitotenv.2017.11.103
Lemire, J. A., Harrison, J. J., and Turner, R. J. (2013). Antimicrobial Activity of Metals: Mechanisms, Molecular Targets and Applications. Nat. Rev. Microbiol. 11, 371–384. doi:10.1038/nrmicro3028
Liu, P., Zhan, X., Wu, X., Li, J., Wang, H., and Gao, S. (2020). Effect of Weathering on Environmental Behavior of Microplastics: Properties, Sorption and Potential Risks. Chemosphere 242, 125193. doi:10.1016/j.chemosphere.2019.125193
Lu, L., Wan, Z., Luo, T., Fu, Z., and Jin, Y. (2018). Polystyrene Microplastics Induce Gut Microbiota Dysbiosis and Hepatic Lipid Metabolism Disorder in Mice. Sci. Total Environ. 631–632, 449–458. doi:10.1016/j.scitotenv.2018.03.051
Ma, Y., Huang, A., Cao, S., Sun, F., Wang, L., Guo, H., et al. (2016). Effects of Nanoplastics and Microplastics on Toxicity, Bioaccumulation, and Environmental Fate of Phenanthrene in Fresh Water. Environ. Pollut. 219, 166–173. doi:10.1016/j.envpol.2016.10.061
Mani, T., Hauk, A., Walter, U., and Burkhardt-Holm, P. (2015). Microplastics Profile along the Rhine River. Sci. Rep. 5, 1–7. doi:10.1038/srep17988
Marchesi, J. R., Adams, D. H., Fava, F., a Hermes, G. D., Hirschfield, G. M., Hold, G., et al. (2015). The Gut Microbiota and Host Health: a New Clinical Frontier. Gut, 1–10. doi:10.1136/gutjnl-2015-309990
Mattsson, K., Ekvall, M. T., Hansson, L., Linse, S., Malmendal, A., and Cedervall, T. (2015). Altered Behavior, Physiology, and Metabolism in Fish Exposed to Polystyrene Nanoparticles.
Menéndez-Pedriza, A., and Jaumot, L. (2020). Microplastics : A Critical Review of Sorption Factors. Toxics 8, 1–40. doi:10.3390/toxics8020040
Napper, I. E., Bakir, A., Rowland, S. J., and Thompson, R. C. (2015). Characterisation, Quantity and Sorptive Properties of Microplastics Extracted from Cosmetics. Mar. Pollut. Bull. 99, 178–185. doi:10.1016/j.marpolbul.2015.07.029
Paradis, E., and Schliep, K. (2019). Ape 5.0: An Environment for Modern Phylogenetics and Evolutionary Analyses in R. Bioinformatics 35, 526–528. doi:10.1093/bioinformatics/bty633
Pérez-Candela, M., Martín-Martínez, J. M., and Torregrosa-Maciá, R. (1995). Chromium(VI) Removal with Activated Carbons. Water Res. 29, 2174–2180. doi:10.1016/0043-1354(95)00035-j
R Core Team (2020). A Language and Environment for Statistical Computing. Vienna, Austria: R Foundation for Statistical Computing.
Richardson, J. B., Dancy, B. C. R., Horton, C. L., Lee, Y. S., Madejczyk, M. S., Xu, Z. Z., et al. (2018). Exposure to Toxic Metals Triggers Unique Responses from the Rat Gut Microbiota. Sci. Rep. 8, 1–12. doi:10.1038/s41598-018-24931-w
Rillig, M. C., and Bonkowski, M. (2018). Microplastic and Soil Protists: A Call for Research. Environ. Pollut. 241, 1128–1131. doi:10.1016/j.envpol.2018.04.147
Rist, S., Baun, A., and Hartmann, N. B. (2017). Ingestion of Micro- and Nanoplastics in Daphnia Magna – Quantification of Body Burdens and Assessment of Feeding Rates and Reproduction. Environ. Pollut. 228, 398–407. doi:10.1016/j.envpol.2017.05.048
Rochman, C. M., Hentschel, B. T., and Teh, S. J. (2014). Long-Term Sorption of Metals Is Similar Among Plastic Types : Implications for Plastic Debris in Aquatic Environments, 9. doi:10.1371/journal.pone.0085433
Santos, I. R., Friedrich, A. C., and Ivar do Sul, J. A. (2009). Marine Debris Contamination along Undeveloped Tropical Beaches from Northeast Brazil. Environ. Monit. Assess. 148, 455–462. doi:10.1007/s10661-008-0175-z
Silva, C. J. M., Silva, A. L. P., Gravato, C., and Pestana, J. L. T. (2019). Ingestion of Small-Sized and Irregularly Shaped Polyethylene Microplastics Affect Chironomus Riparius Life-History Traits. Sci. Total Environ. 672, 862–868. doi:10.1016/j.scitotenv.2019.04.017
Varg, J. E., Kunce, W., Outomuro, D., Svanbäck, R., and Johansson, F. (2021). Single and Combined Effects of Microplastics, Pyrethroid and Food Resources on the Life-History Traits and Microbiome of Chironomus Riparius. Environ. Pollut. 289. doi:10.1016/j.envpol.2021.117848
Vargas, J. E., and Dussán, J. (2016). Adsorption of Toxic Metals and Control of Mosquitos- Borne Disease by Lysinibacillus Sphaericus: Dual Benefits for Health and Environment. Biomed. Environ. Sci. 29, 187–196.
Vavrek, M. J. (2011). Fossil: Palaeoecological and Palaeogeographical Analysis Tools. Palaeontol. Electron. 14.
Wagner, M., and Lambert, S. (2018). Freshwater Microplastics. Page Freshwater Microplastics the Handbook of Environmental Chemistry.
Wang, J., Liu, X., Li, Y., Powell, T., Wang, X., Wang, G., et al. (2019). Microplastics as Contaminants in the Soil Environment: A Mini-Review. Sci. Total Environ. 691, 848–857. doi:10.1016/j.scitotenv.2019.07.209
Zeng, X., Xu, X., Boezen, H. M., and Huo, X. (2016). Children with Health Impairments by Heavy Metals in an E-Waste Recycling Area. Chemosphere 148, 408–415. doi:10.1016/j.chemosphere.2015.10.078
Zettler, E. R., Mincer, T. J., and Amaral-Zettler, L. A. (2013). Life in the “Plastisphere”: Microbial Communities on Plastic Marine Debris. Environ. Sci. Technol. 47, 7137–7146. doi:10.1021/es401288x
Keywords: microplastics, toxic metals, microbiota, host-microbiome, ecotoxicology, multiple stressors
Citation: Varg JE, Bergvall C and Svanbäck R (2022) The Stressful Effects of Microplastics Associated With Chromium (VI) on the Microbiota of Daphnia Magna. Front. Environ. Sci. 10:875512. doi: 10.3389/fenvs.2022.875512
Received: 14 February 2022; Accepted: 25 April 2022;
Published: 13 May 2022.
Edited by:
Sadasivam Anbumani, Indian Institute of Toxicology Research (CSIR), IndiaReviewed by:
Qiqing Chen, East China Normal University, ChinaCopyright © 2022 Varg, Bergvall and Svanbäck. This is an open-access article distributed under the terms of the Creative Commons Attribution License (CC BY). The use, distribution or reproduction in other forums is permitted, provided the original author(s) and the copyright owner(s) are credited and that the original publication in this journal is cited, in accordance with accepted academic practice. No use, distribution or reproduction is permitted which does not comply with these terms.
*Correspondence: Javier Edo Varg, amVkb3ZhcmdAZ21haWwuY29t
Disclaimer: All claims expressed in this article are solely those of the authors and do not necessarily represent those of their affiliated organizations, or those of the publisher, the editors and the reviewers. Any product that may be evaluated in this article or claim that may be made by its manufacturer is not guaranteed or endorsed by the publisher.
Research integrity at Frontiers
Learn more about the work of our research integrity team to safeguard the quality of each article we publish.