- 1College of Resources and Environment, Xinjiang Agricultural University, Urumqi, China
- 2Suzhou Agricultural Ecological Environment Station, Suzhou, China
- 3College of Resources and Environmental Sciences, China Agricultural University, Beijing, China
- 4Xinjiang Huier Agriculture Company Ltd., Changji, China
Phosphorus (P) fertilizer is generally applied to enhance the soil P pool and meet crop demand, but most of the added P is absorbed by soil. This study aimed to explore the importance of P fractions and adsorption–desorption characteristics on the demand for P fertilizer to determine the optimum P fertilizer amount for a cotton field under mulched drip irrigation in Xinjiang, China. A 4-year experiment (2016–2019) was conducted in a cotton field to evaluate the effects of five P fertilizer addition levels (0, 75, 150, 300, and 450 kg P2O5 ha−1 year−1) on inorganic P fractions and P adsorption–desorption properties at different soil depths and explore the effects of soil properties on P adsorption. The 4-year continuous P fertilization enhanced the inorganic P content and altered the proportions of various P forms. A large proportion of P accumulated in soil was transformed into Ca8-P, followed by Ca2-P, mainly in the surface layer. The accumulation and transformation of P in fertilized soil reduced P adsorption and enhanced P desorption. Soils with higher P application levels had higher inorganic P accumulation with lower adsorption and higher desorption. The total P and Olsen-P in a 0- to 5-cm soil layer; Ca8-P and calcium carbonate (CaCO3) in a 5- to 10-cm soil layer; Olsen-P, Ca10-P, clay, and cation exchange capacity in a 10- to 20-cm soil layer; and Olsen-P in a 20- to 40-cm soil layer significantly affected the P adsorption–desorption (p < 0.05). The application rate of 75–150 kg P2O5 ha−1 year−1 produced lower inorganic P accumulation, favorable adsorption–desorption properties, and high cotton yield, and thus could be considered the optimal P fertilizer application level.
Introduction
Phosphorus (P) accumulation in soil is often associated with intensive agricultural production (MacDonald, et al., 2011; Mai et al., 2018), in which high amounts of P fertilizer are applied to overcome soil P deficiency. However, soils receiving huge fertilizer input have accumulated P to a level beyond the agronomic optimum required for satisfactory crop production (Dou et al., 2009). Therefore, it is important to explore P fertilizer application levels and P dynamics in soil to achieve efficient management of P fertilizer in agricultural production. Both transformation of P forms and P adsorption–desorption behavior are key aspects of soil P dynamics (Bai et al., 2017; Yan et al., 2017).
Soil inorganic P, the main component of the soil P pool in calcareous soil, can be separated into the following six forms: Ca2-P, Ca8-P, Fe-P, Al-P, occluded P (O-P), and Ca10-P (Jiang and Gu, 1989). These different forms of inorganic P in soil are in the process of continuous transformation. Soil physicochemical properties, environmental factors, and management measures are the main factors affecting the transformation and availability of inorganic P (Jiao et al., 2020). Addition of P fertilizer affects the status of soil P fractions and total P (TP) accumulation by directly or indirectly changing soil properties (Yan et al., 2017). Li W. W. et al. (2020) showed that the proportion of P fractions was in an order of moderate-activity P sources (Ca8-P, Fe-P, Al-P) > potential P sources (O-P, Ca10-P) > available P source (Ca2-P) in a 15-year experiment of P fertilization on greenhouse vegetables in calcareous soil. Lv and Yang (2019) showed that Fe-P and Ca10-P were the main inorganic P fractions present after long-term fertilization of black soil. Wang et al. (2020) observed that the increasing P application rate led to an increase in Al-P, Fe-P, and Ca-P proportions in yellow-mud paddy soil. In general, there is an increase in the proportion of inorganic labile and moderate labile P fractions but a decrease or stable trend in the proportion of low labile fractions to TP with the history of long-term P fertilization (Pavinato et al., 2009; Li W. W. et al., 2020). However, there are significant differences in P fraction proportions among different regions or soil types, and further research is needed to explore the transformation of inorganic P fractions under drip irrigation in arid regions.
The P adsorption–desorption reaction of soil affects the P availability of the crop directly (Bera et al., 2006; Yang et al., 2019) and can determine the key process controlling the utilization efficiency of P fertilizer (McLaughlin et al., 2011). It is generally accepted that the soil properties such as pH, soil organic matter (SOM), clay, and calcium carbonate (CaCO3) contents affect the P adsorption–desorption characteristics of soil (Bai et al., 2017; Zhang Y. et al., 2018; Yan et al., 2018; Yang et al., 2019). Prakash et al. (2017) pointed out that the existing soil P, clay, and CaCO3 contents were the main factors affecting soil P adsorption–desorption. The Langmuir and Freundlich equations can provide a suitable description of P adsorption properties of soil. Some P sorption parameters can be determined via these equations, which can express the capacity of soil for P adsorption (Yan et al., 2018; Yang et al., 2019; Sun et al., 2020). In general, soils with a high P application have less capacity to retain P at a higher P adsorption saturation (Yan et al., 2018; Wang et al., 2019), posing a greater risk for P leaching than soils receiving limited or no P application (McDowell, 2012). Zhang Y. et al. (2018) found that a fine-textured soil had stronger P absorption and weaker desorption ability with a higher maximum P buffer capacity, but a coarse-textured soil had the opposite absorption–desorption ability. However, most reports of the effect of some soil properties (e.g., pH, SOM, and CaCO3) on P adsorption are contradictory (Carreira et al., 2006; Bai et al., 2017; Yang et al., 2019). The relationship between soil properties and P adsorption capacity, though highly studied, does not tell a straightforward story. Accordingly, researchers are interested in P adsorption–desorption in soil and factors controlling P binding at both the local and international scales.
Low availability of inorganic P and adsorption–desorption properties in soil are often the limiting factors for P utilization efficiency and crop productivity (Sun et al., 2020; Yang et al., 2020). Phosphorus is the second nutrient limiting factor for cotton yield under film drip irrigation (Chen et al., 2020). Therefore, the objective of this study was to explore P dynamics of soil in cotton fields under medium- and short-term fertilization management with drip irrigation under film in the arid area. The specific objectives were to 1) investigate effects of different P application levels on the transformation of inorganic P forms under mulched drip irrigation, 2) evaluate P adsorption–desorption properties under mulched drip irrigation, 3) determine the influence and contribution of different soil properties on the characteristics of P adsorption behavior in a 4-year field assessment, and 4) identify the optimization of P fertilizer application to resolve agronomic P imbalances in the cotton field.
Materials and Methods
Experimental Site
The field experiment was conducted in Manas County, Xinjiang, Northwest China (86°44′N, 44°79′E). The site has a temperate continental climate with an average annual temperature of 7.2°C, 2,500–3,000 annual sunshine hours, 160–180 frost-free days per year, and an average annual precipitation of 173.3 mm. The mean daily temperature and precipitation from April to September in 2016–2019 are presented in Supplementary Figure S1. The soil type at the experimental field is a gray desert soil with a loam texture. The soil physicochemical properties are shown in Table 1. Cotton “XinLuZao57,” the main cultivated cotton genotype in the region, was selected for planting during this study.
Experimental Design
The experiment was conducted for 4 years (2016–2019). Five P fertilization treatments were set in randomized blocks with three replicates: P0 (control without any applications of P fertilization), P75 (75 kg P2O5 ha−1 year−1), P150 (150 kg P2O5 ha−1 year−1), P300 (300 kg P2O5 ha−1 year−1), and P450 (450 kg P2O5 ha−1 year−1). There were 15 plots in total, and the size of each plot was 8.8 × 6 m. The five P fertilizer treatments were applied along with nitrogen fertilizer (300 kg N ha−1 year−1) and potash fertilizer (75 kg K2O ha−1 year−1). The P fertilizer (superphosphate), 40% of the nitrogen fertilizer (urea), and 50% of the potash fertilizer (potassium sulfate) were applied on the surface and then incorporated into the soil by ploughing before sowing as a basal dressing. The remainder of the N fertilizer and potash fertilizer was applied with irrigation. Cotton was normally sown in mid-April, and the other field management practices were consistent with the local cotton agronomic practices.
Soil Sampling and Analysis
Soil samples were collected at 0 to 40 cm depth with 20-cm increments to analyze the properties of background before fertilization in April 2016. The soil profile was sampled at 0–5, 5–10, 10–20, and 20–40 cm in the cotton flowering and boll set (maximum efficiency stage of P nutrition in cotton) stages annually, with a total of 80 soil samples. Each mixed soil sample consisted of five parallel samples from a plot, which were air-dried under natural conditions and sequentially sieved through 2-, 1-, 0.25-, and 0.15-mm sieves after the removal of gravel, film, roots, and other debris.
Soil physicochemical properties were measured using the methods outlined by Bao (2000). Briefly, the soil pH was determined in a 1:5 soil:water suspension using a pH meter (FE28-TRIS pH), and the salinity was measured by weighing the dry residue after the evaporation of suspension on a sand bath. The SOM was determined using the KCr2O7 redox titration method. Soil available P (Olsen-P) and TP were determined by the NaHCO3 (pH 8.5) extraction method and H2SO4–HClO4 digestion, respectively. The soil mechanical composition was determined by using the pipette method and divided into clay (<0.002 mm), silt (0.002–0.05 mm), and sand (0.05–2 mm). The CaCO3 content was determined by the gasometric method. The cation exchange capacity (CEC) was measured using the NaOAc method.
Determination of Soil Inorganic Phosphorus Fractions
Soil inorganic P fractions were measured by using the sequential fractionation method developed for calcareous soils (Jiang and Gu, 1989). A 0.5 g air-dried soil sample (0.15 mm) was placed in a 50-ml plastic centrifuge tube and successively extracted with 25 ml each of 0.25 mol L−1 NaHCO3, 0.5 mol L−1 NH4Ac, 0.5 mol L−1 NH4F, 0.1 mol L−1 NaOH–Na2CO3, 0.3 mol L−1 Na3C6H5O7 (20 ml) + 0.5 g Na2S2O4 + 0.5 mol L−1 (5 ml) NaOH, and 0.25 mol L−1 H2SO4 in that order, and inorganic P concentrations in the extracts were assayed by using the molybdenum blue colorimetric method (Murphy and Riley, 1962). The results were the average of three replications. In this article, these inorganic P fractions are referred to as Ca2-P, Ca8-P, Al-P, Fe-P, O-P, and Ca10-P, respectively.
Phosphorus Adsorption and Desorption Experiments
In the adsorption experiment, 2q air-dried soil samples (0.25 mm) were placed in a 50-ml plastic centrifuge tube, and 40 ml P standard solutions (prepared by Ca(H2PO4)2H2O) of various concentrations (0, 5, 10, 20, 30, 40, 50, 75, 100, 150, and 200 mg L−1) were added. The P standard solutions contained 0.01 mol L−1 CaCl2, and each series was performed in triplicate. Two drops of toluene were added to inhibit microbial activities. The tubes were capped and placed at a constant temperature of 25°C in a shaker at 200 rpm for 2 h. The mixtures were then intermittently shaken for 24 h at intervals of 12 h for 30 min each time. Thereafter, the suspensions were centrifuged at 2,200 ×g for 10 min, and after filtration, the P concentration in the equilibrium solution was determined by using the molybdenum blue colorimetric method. The quantity of P adsorbed was the difference between P added and P remaining in the filtrate.
For the desorption experiment, the residual soils obtained from the adsorption experiment were washed twice with 30 ml saturated NaCl to remove adsorbents. Subsequently, a 40 ml of 0.02 mol L−1 KCl was added to the tubes and shaken at 200 rpm for 1 h at 25°C in a thermostat shaker. The tubes were periodically shaken twice a day for 2 days (30 min each time). The suspensions were centrifuged for 10 min at 2,200 ×g. The content of P desorbed was measured by using the molybdenum blue colorimetric method (Bao, 2000).
Adsorption and Desorption Models for Phosphorus
The adsorption models can quantitatively illustrate the equilibrium characteristics of P adsorption onto the particle surface of soil. In the present study, P adsorption was modeled with the Langmuir and Freundlich equations. The Langmuir equation is as follows:
where C is the P concentration (mg L−1) in the adsorption equilibrium solution, Q is the amount of P adsorbed in each soil sample (mg kg−1), KL is a constant associated with the adsorption energy of P (L mg−1), and Qm is the maximum adsorption capacity of P (mg kg−1). In addition, the maximum P buffer capacity (MBC, mg kg−1) of soil was calculated from the product of Langmuir parameters Qm and KL. The degree of P saturation (DPS, %) was calculated from the ratio of available P (Olsen-P) to P adsorption maximum (Qm).
The Freundlich equation is as follows:
where KF is the Freundlich affinity coefficient (L kg−1) and n is a constant related to adsorption intensity.
Statistical Analysis
The data were preprocessed using Microsoft Excel spreadsheets (MS. Co., Redmond, WA, United States). Means and standard deviations for each variable were calculated. Differences in P fractions, P adsorption parameters, and soil properties between the five treatments were analyzed statistically using the one-way analysis of variance, and significant differences between individual treatments were determined by Tukey’s post hoc testing. Linear regression analysis was applied to the Langmuir and Freundlich isotherms of P adsorption on soil with different P contents. All statistical analyses were conducted using SPSS 19.0 (SPSS Inc., Chicago, IL, United States) with a significance threshold of p < 0.05. Redundancy analysis (RDA) was conducted to study the relationship between P adsorption parameters and different soil properties using Canoco 5 software package (Microcomputer Power, Ithaca, NY, United States). Curve fitting and figure creation were conducted using Origin 2018 software package (OriginLab Co., Northampton, MA, United States).
Results
Inorganic Phosphorus Fractions
The contents of inorganic P fractions in the soils are shown in Figure 1. The Ca-P content was the highest among the fractions, accounting for more than 82% of total inorganic P (Figure 2), whereas the contents of Al-P, Fe-P, and O-P were lower. The highest content of Ca-P was in the form of Ca10-P, followed by Ca8-P and Ca2-P. Inorganic P fraction contents increased holistically with the increase in the P application rate and exhibited much higher levels in the P300 or P450 treatments than those under the P0 treatment. Inorganic P fraction contents generally declined along the soil profiles. And these inorganic P fraction contents increased in all depths over time, whereas those under the P0 treatment decreased over time. The analysis of the proportion of different P fractions in total inorganic P (Figure 2) shows that the proportions of Ca2-P, Ca8-P, Al-P, and Fe-P increased initially and then decreased as the P application rate increased. The proportions of Ca2-P and Ca8-P peaked under the P300 treatment, whereas the proportions of Al-P and Fe-P peaked under the P150 treatment. The proportions of O-P and Ca10-P decreased to a nadir under the P300 treatment initially and then increased as the P application rate increased. The proportions of Ca2-P and Ca8-P decreased gradually as the experiment time increased under the P0 treatment but increased to different degrees under other treatments (P75, P150, P300, and P450), whereas the opposite tendency was shown for the variation of the Ca10-P proportion. The proportions of Al-P, Fe-P, and O-P showed irregular change with small variances.
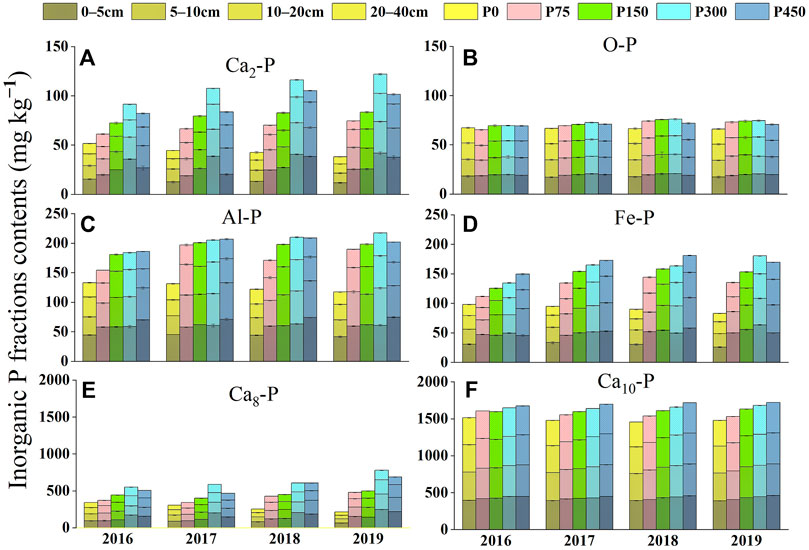
FIGURE 1. Inorganic P fractions (A) Ca2-P, (B) O-P, (C) Al-P, (D) Fe-P, (E) Ca8-P, and (F) Ca10-P contents in different treatments in 2016–2019.
Soil P Adsorption Characteristics
The P-adsorption isotherms are shown in Figure 3, which were similar among treatments, and the adsorption capacity first increased rapidly and then slowly with the increase in the equilibrium solution P concentration in 2016–2019 under the five fertilization treatments and at the four soil depths. Among them, the P-adsorption isotherms in 2016 did not reach the plateau of the isotherms (Figures 3A–D); therefore, a higher initial P concentration was confidently added in other years, enabling the isotherms to curve.
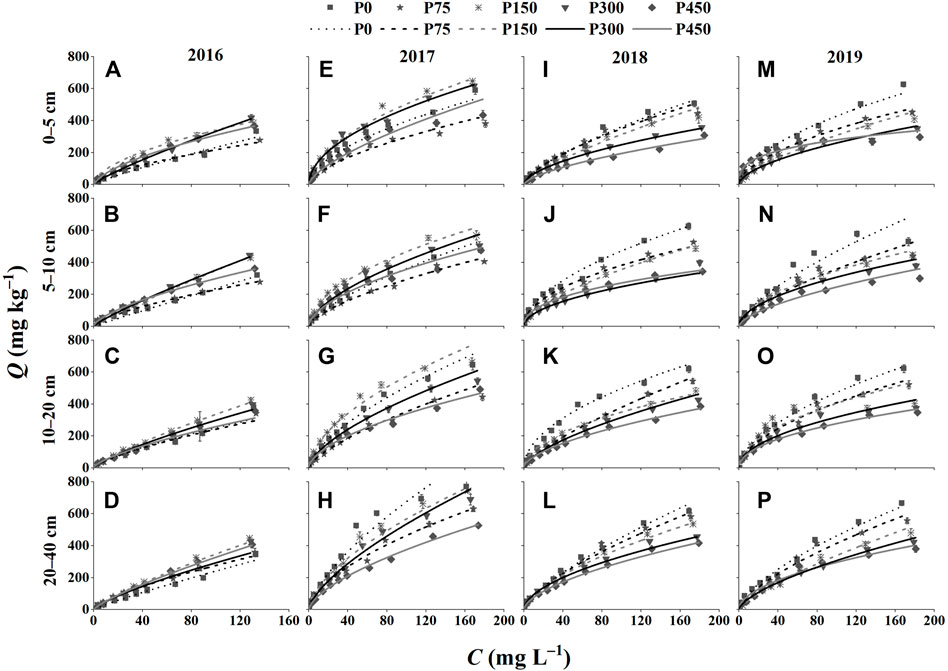
FIGURE 3. Phosphate isothermal adsorption curves of different treatments in 2016–2019. (A–D) 0–5, 5–10, 10–20, and 20–40 cm in 2016; (E–H) 0–5, 5–10, 10–20, and 20–40 cm in 2017; (I–L) 0–5, 5–10, 10–20, and 20–40 cm in 2018; and (M–P) 0–5, 5–10, 10–20, and 20–40 cm in 2019. C, the P concentration in the adsorption equilibrium solution; Q, the equilibrium adsorption capacity.
Different fertilization treatments showed a marked influence on the P adsorption isotherms. The amount of P absorbed from soils in 2016 increased first and then decreased with the increase in the P application rate, that is, the amount of P absorbed from soils was in an order of P150 and P300 > P450 > P0 and P75 (Figures 3A–D). Moreover, there was little discrepancy in the amount of soil P adsorption among treatments in 2016. The P adsorption in 2017 was relatively low in P75 and P450, and the capacity of P150 was higher than that of other treatments (Figures 3E–H). The adsorption capacity of soil P in 2018 and 2019 decreased in the order P0 > P75 > P150 > P300 > P450 (Figures 3I–P), showing a decrease in P adsorption with an increasing P application rate. Compared with the adsorption characteristics among treatments in 2016 (Figures 3A–D), there were significant differences in the P adsorption capacity among treatments in 2017, 2018, and 2019 (Figures 3E–P). Moreover, the depth of soil layers had a certain influence on the P adsorption capacity. The soil P adsorption capacity of each treatment increased gradually with the increase in soil depth (Figure 3). In addition, the P0 and P75 treatments caused increases in the soil P adsorption capacity for 4 years; however, the soil P adsorption capacity of the P150, P300, and P450 treatments decreased with prolonged fertilization, especially in the P450 treatment.
In order to better quantify the variation of adsorbed P in different treatments, the Freundlich and Langmuir P adsorption models were fitted, and the parameters are listed in Supplementary Tables S1, S2. The optimal model was the Freundlich equation, with the correlation coefficient of fitting (R2) in the range of 0.916–0.998 (Supplementary Table S1). The n values obtained from the Freundlich model were greater than 1 (Supplementary Table S1). The correlation of fitting for the Langmuir equation was poor compared with that of the Freundlich model, but it also reached a significant level (R2 = 0.518–0.994; Supplementary Table S2). The parameters of Qm and KL fitted by the Langmuir equation along with MBC and DPS related to the Langmuir equation are important indicators of the ability of soil P adsorption. The results showed that the value of KL was positive (Supplementary Table S2), indicating that the adsorption reaction was spontaneous. The 4 years of P application could reduce the value of Qm and enhance the DPS (Supplementary Table S3). Moreover, the adsorption of P0 increased depending on the shortage of soil P for years as Qm was in the range of 303–833 mg kg−1 under P fertilizer treatments at all four depths in 2019 compared with 769–1,000 mg kg−1 for the soils under the P0 treatment in 2019 (Supplementary Table S2).
Soil P Desorption Characteristics
As shown in Figure 4, the P desorption capacity increased substantially at a lower initial P concentration, and then the increase in P desorption tended to be slower or near steady as the initial P concentration increased. In addition, the order of P desorption capacity in different fertilization treatments was opposite to that of adsorption. In general, the P desorption capacity increased as the P application rate increased and decreased gradually with the increase in soil depth. Moreover, as the number of fertilization years increased, the P desorption capacity decreased in the P0 and P75 treatments but increased in the P150, P300, and P450 treatments.
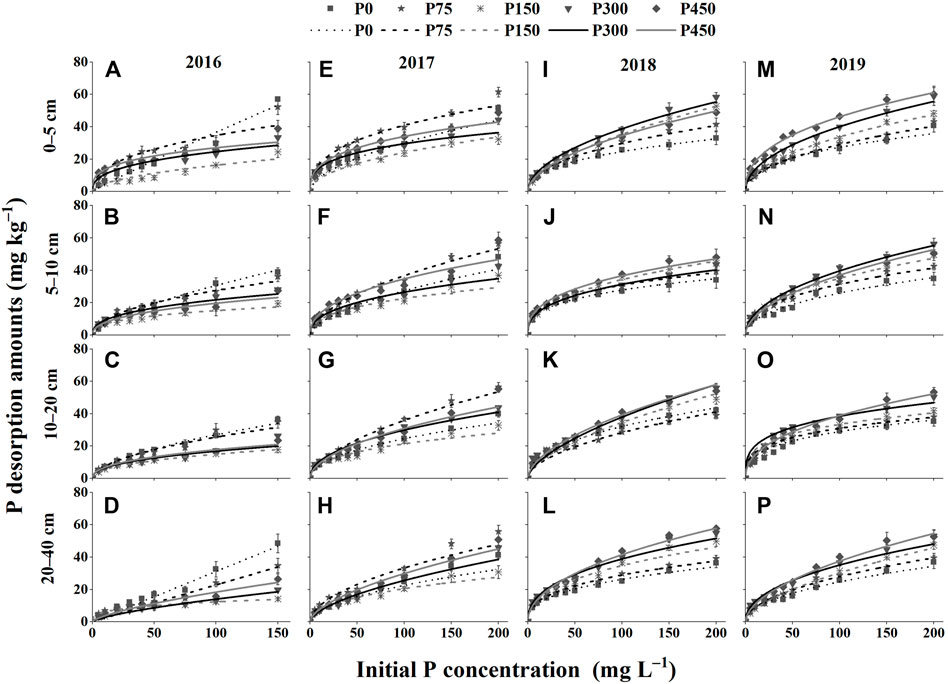
FIGURE 4. Phosphate isothermal desorption curves of different treatments in 2016–2019. (A–D) 0–5, 5–10, 10–20, and 20–40 cm in 2016; (E–H) 0–5, 5–10, 10–20, and 20–40 cm in 2017; (I–L) 0–5, 5–10, 10–20, and 20–40 cm in 2018; and (M–P) 0–5, 5–10, 10–20, and 20–40 cm in 2019.
As shown in Figure 5, the P desorption rate first decreased and then gradually leveled off. In addition, the order of the P desorption rate in different fertilization treatments was opposite to that of the P adsorption capacity. In general, the P desorption rate increased as the P application rate increased, and decreased gradually with the increase in soil depth. Moreover, there was a decrease in the P desorption rate under the P0 and P75 treatments but an increase in those of the P150, P300, and P450 treatments as the number of P fertilization years increased.
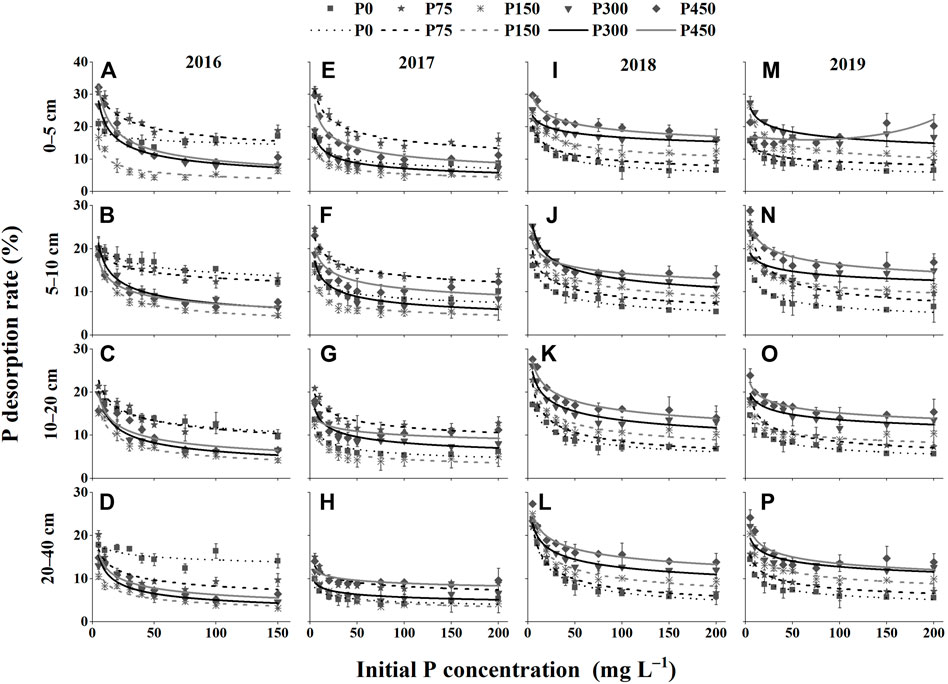
FIGURE 5. Phosphate desorption rate of different treatments in 2016–2019. (A–D) 0–5, 5–10, 10–20, and 20–40 cm in 2016; (E–H) 0–5, 5–10, 10–20, and 20–40 cm in 2017; (I–L) 0–5, 5–10, 10–20, and 20–40 cm in 2018; and (M–P) 0–5, 5–10, 10–20, and 20–40 cm in 2019.
Soil Properties
The average values of soil physicochemical properties in the 4 years of fertilization treatment are shown in Table 2. The P application treatments had no significant effect on pH, SOM content, and salinity of soil in the cotton field, but a slight decrease in pH and SOM content and a small increase in salinity were shown as P application levels increased. Soils under P application treatments exhibited substantial increases in Olsen-P and TP contents, and the largest increase was observed in the P450 treatment when compared with the P0 treatment. By contrast, CaCO3 and CEC were not significantly affected by P fertilization. Moreover, the mechanical composition of soil changed under P application, as indicated by the significant increase in sand and a decrease in clay and silt (p < 0.05). In addition, there were some differences in soil properties among different layers, and the history of P fertilization can also contribute to further effects on soil properties.
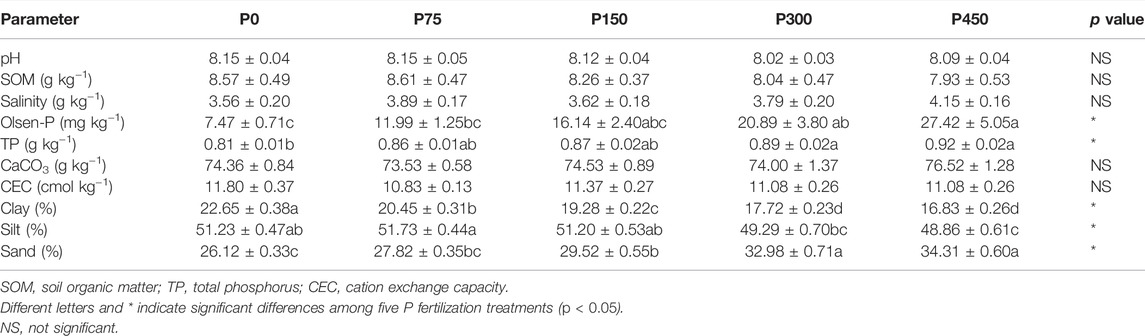
TABLE 2. Mean values of soil properties (0–40 cm) of different treatments under 4-year fertilization.
Response of P Adsorption–Desorption Parameters to Soil Properties
The RDA showed that the selected soil variables could explain 96.41, 85.41, 96.01, and 95.67% of the total variance observed in the characteristic parameters of P adsorption and desorption for the 0–5, 5–10, 10–20, and 20–40 cm soil depths, respectively (Figure 6). At 0–5 cm soil depth (Figure 6A), TP and Olsen-P were the major factors explaining 49.7% (p = 0.008) and 15.1% (p = 0.008) of the variance, respectively. In the 5- to 10-cm soil layer (Figure 6B), Ca8-P and CaCO3 were the main factors explaining 56.2% (p = 0.004) and 17.5% (p = 0.018) of the variance, respectively. In the 10- to 20-cm soil layer, Olsen-P (p = 0.002), Ca10-P (p = 0.010), clay (p = 0.022), and CEC (p = 0.032) accounted for the majority of the variance (91.5%) (Figure 6C), whereas Olsen-P was the main explanatory factor in the 20- to 40-cm soil layer, explaining 62.2% (p = 0.002) of the variance (Figure 6D).
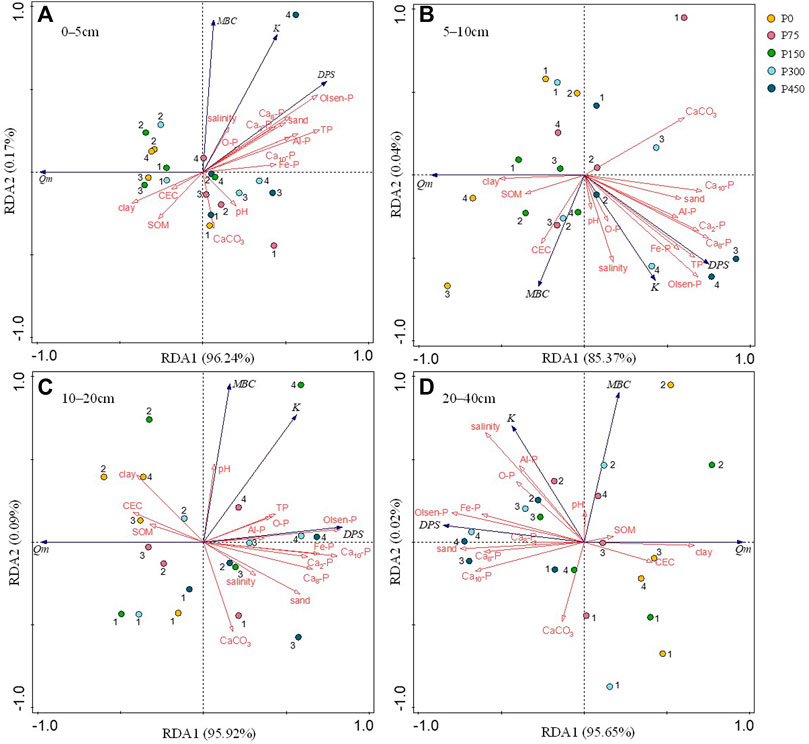
FIGURE 6. Redundancy analysis showing the correlations between the adsorption–desorption parameters and soil physicochemical properties of different treatments under 4-year fertilization. (A) 0–5 cm, (B) 5–10 cm, (C) 10–20 cm, and (D) 20–40 cm. 1, 2, 3, and 4 indicate 2016, 2017, 2018, and 2019, respectively.
Discussion
Effects of Different Fertilization Treatments on Inorganic Phosphorus Fractions
A 4-year continuous P fertilizer application resulted in the variation of the accumulation and distribution of P fractions and enhanced the contents of different forms of inorganic P, except under the P0 treatment (Figure 1). Compared with 2016, the total amount of inorganic P in the 0- to 40-cm soil layer of the P0 treatment in 2019 decreased by 8.2–10.8% (Figure 1). This is perhaps because of the lack of enough soil P available in the P0 treatment or the easy uptake of inorganic P by cotton (Guo et al., 2008). The proportions of Ca2-P and Ca8-P decreased over time, but the proportions of O-P and Ca10-P increased under the P0 treatment (Figure 2). This shows that Ca2-P and Ca8-P were easily used by cotton, whereas O-P and Ca10-P were less easily used and accumulated in soil, which is consistent with Zhang et al. (2021). Zhang et al. (2020) further clarified that after P fertilization ceased in a long-term cropland, plants mainly absorbed the available inorganic P and then moderate-activity P was desorbed to replenish the available P pool. The P fertilization enhanced the contents of inorganic P fractions, which increased with an increasing P application level under moderate P application treatments (P75, P150, and P300). Compared with the P0 treatment, the average content of Ca2-P, Ca8-P, and Al-P under moderate P application treatments (P75, P150, and P300) increased to 97.9, 76.8, and 52.6%, respectively (Figure 1). The possible reason is that the moderate P application activates soil biological characteristics, so as to promote the transformation of insoluble P to medium stable P and active P (Jiao et al., 2020). Excessive P fertilizer application (P450) may reduce the Ca2-P, Ca8-P, and Al-P contents compared with moderate application (Figure 1), which illustrates that excessive P fertilizer application was not the best way to improve the availability of soil inorganic P. By contrast, the P450 treatment increased the proportion of low available P (Ca10-P, O-P) compared with the P300 treatment. This increase was similar to that shown by Mahmood et al. (2020), which indicates that excessive P application can alter soil P availability and limit plant growth by compacting soil and fixation of P into different forms.
In addition, P accumulated in soil was mainly converted into Ca8-P, followed by Ca2-P, whereas the proportion of Ca10-P in total inorganic P decreased over the 4 years of P application. The proportion of other inorganic P forms (Al-P, Fe-P, and O-P) remained relatively stable over time. As the first available P source of soil, the Ca2-P content was low, and the increase in its proportion was consistent with the increase in the Olsen-P content over time (Supplementary Table S7). Previous studies have shown that an excess of available P in soil could promote the transformation of Ca2-P to Ca8-P, which reveals that the application of P fertilizer mainly added the moderate-activity P sources of soil (Li W. W. et al., 2020; Li C. L. et al., 2020). However, the proportions of Al-P and Fe-P did not increase significantly over time, maybe owing to their low contribution to total inorganic P or the differences in soil types (Sugihara et al., 2012). The O-P and Ca10-P forms represented the main potential P sources. The lowered proportion of Ca10-P over time may be due to the transformation of Ca10-P to Ca8-P. Li C. L. et al. (2020) observed that P fertilization for 32 years led to the transformation of Ca2-P, Fe-P, O-P, and Ca10-P into Ca8-P in a Mollisol of Northeast China under single spring maize cropping. The proportion of O-P is relatively stable with years in the current study, which may be because its inherent stability made it less affected by fertilization (Wang et al., 2020).
The change characteristics of inorganic P in the soil profile were in line with those shown by Pavinato et al. (2009); the inorganic P mainly accumulated in the uppermost soil layer, with a lower inorganic P level in deeper soil (Figure 1). In this study, Ca2-P, Ca8-P, and Al-P in the 0- to 10-cm soil layer of the five treatments were significantly higher than those in the 20- to 40-cm soil layer. However, the Fe-P, O-P, and Ca10-P had no significant differences in each soil layer in the five treatments (Figure 1). The movement of inorganic P fractions in the soil profile indicated that the movement of P in soil was affected by the form of inorganic P and soil characteristics (Keter and Ahn, 2010). Compared with the P accumulation in deeper soil (60–70-cm depth) observed by previous long-term studies (Rubaek et al., 2013; Wang et al., 2015), the P accumulation depth was shallower in the 4-year experiment (20–40 cm), owing to the shorter period of fertilization.
Effects of Different Fertilization Treatments on Phosphorus Adsorption and Desorption
The continuous application of P fertilizer profoundly affected the characteristics of P adsorption and desorption. The accumulation and transformation of soil P under the P application treatments distinctly decreased the P adsorption capacities, but those of the soil without P application were higher, as confirmed by the decreases in Qm and the increases in DPS as the P application rate increased. The decreasing P adsorption capacity in P-fertilized soils suggests that part of the excessive phosphate was occupying the adsorption sites, hence blocking further reaction. In the present study, the variation characteristics of soil P adsorption in 2016 were slightly different from those in other years, indicating that the adsorption capacity first increased and then decreased as the P application rate increased. This may be because the equilibrium concentration of adsorption–desorption reactions could be higher and more sensitive to the surrounding environment under the lower P content in the P0 and P75 treatments in 2016 (Supplementary Tables S7, S8) (Zhao et al., 2019). Correspondingly, the P fixation ability was relatively poorer in the P0 and P75 treatments, showing a lower adsorption capacity and a greater desorption capacity. Of these, P75 treatment had a lower Qm and a larger DPS. By contrast, the P150, P300, and P450 treatments had a higher Qm and a smaller KL value, which was similar to the findings of Shahabifar et al. (2019) for the adsorption parameters of calcareous soils with different inherent P levels. The discrepancy of the relationship between the soil P adsorption capacity and P application rate in 2016 (the first year of the experiment) and other years implies that the effect of different P application rates on soil P adsorption properties was also affected by the continuous P application. The organic acids secreted by plant roots will restrict the number of soil adsorption sites and complex with iron and aluminum ions, so as to inhibit the absorption of phosphorus by soil (Zhang X. et al., 2018). As the P content and cotton root distribution in deep soil were low (Prakash et al., 2017; Zhang Y. et al., 2018), the soil P adsorption capacity increased progressively toward the deep soil.
In a previous study, the soil P adsorption capacity increased in the unfertilized control but decreased in P application treatments in response to 6-year fertilization (Yan et al., 2018). Similarly, the interannual variation of the adsorption characteristics of different P application treatments in the current study mainly manifested in the continuous consumption of P in the cotton field under the P0 treatment, along with the increase in Qm and the decrease in DPS over time. In addition, Qm in the P75 treatment increased gradually over the 4-year study period. Moreover, P accumulated progressively in the P150, P300, and P450 treatments, along with the decrease in Qm and the increase in DPS over time. The differences of the soil P content and adsorption capacity among different P application treatments peaked in 2019 (the fourth year of the experiment), indicating a lower adsorption capacity under the higher P application amount. Moreover, the change in the DPS value with P application years showed that P fertilization over an extended period could significantly enhance the ability of soil to release the absorbed P to the environment. In 2019, the DPS value of surface soil under the P450 treatment was 26%, which was 8-fold than in 2016, and reached the critical value of soil P loss of 25% (Schoumans and Groenendijk, 2000). An increased DPS value in soil has resulted in P transport to the deeper soil (Rubaek et al., 2013), confirmed by the P accumulation in soil at all depths (Figure 1; Supplementary Tables S7, S8).
The desorption of P in soil is a reversible process, which is directly related to the reuse of adsorbed P and the improvement of P availability in soil (Heidari et al., 2017). The P desorption capacity of the cotton field soil decreased with the increase in adsorption capacity, coinciding with the results of Jalali and Peikam (2013) and Bai et al. (2017). For 4 consecutive years, the amount and rate of soil P desorption increased with the increase in the P application rate. The soil that absorbed a low proportion of applied P during the adsorption reaction tended to release a higher proportion during the desorption reaction (Bhattacharyya et al., 2015). Because soil capacity is full in the soil with higher P application and cannot uptake or adsorb more P, the desorption increases compared with adsorption. In the soil profile of the cotton field, the desorption capacity of soil P gradually decreased with the increase in soil depth. This supports the findings of Yan et al. (2018), wherein the desorption capacity of the 0- to 30-cm soil layer was about 1.5 times that of the 30- to 60-cm soil layer in P-fertilized calcareous soil. The stronger P desorption capacity of surface soil provided more P for cotton growth, whereas the desorption capacity of deep soil was weak, which reduced P leaching down the soil profile. Under a consecutive 4-year P fertilization, the P desorption capacity of the P150, P300, and P450 treatments increased over time, whereas it decreased in the P0 and P75 treatments. This was contrary to the change in P adsorption parameters and the soil P level under 4 years of P addition. The difference of P the level between different P application treatments increased further over time. Under the higher P application treatments, the soil P pools tended to be saturated and the limited adsorption sites of soil were occupied (Guo et al., 2008; Bhattacharyya et al., 2015; Wang et al., 2019). Therefore, the effect on the higher P adsorption–desorption capacity and desorption rate was different between years. Combined with the adsorption–desorption properties of soil and cotton yield among different P application treatments (Supplementary Figure S2), the optimal P fertilizer application for the cotton field was 75–150 kg P2O5 ha−1 year−1.
Response of Phosphorus Adsorption–Desorption Characteristics to Soil Properties Under Different Fertilization Treatments
A 4-year continuous P fertilizer application significantly affected some of the soil physicochemical properties and had subsequent impacts on soil adsorption–desorption characteristics. The RDA showed that the major factors affecting soil adsorption–desorption varied with soil depth, and the contribution of each factor to adsorption–desorption was unequal. Different P application levels led to significant differences in the soil P nutrient profit and loss, and the extreme range of TP and Olsen-P reached 0.33 g kg−1 and 72.19 mg kg−1 in the 0- to 5-cm soil layer in 2019, respectively. Moreover, there was an inverse relationship between the P content and adsorption capacity. Accordingly, the cotton field soil with accumulated P had a reduced P adsorption capacity, and soil properties contributed to this reduction. The Olsen-P played a key role in P adsorption in the soil profile, except for the 5- to 10-cm soil layer, and the TP, Ca8-P, and Ca10-P contents were the major parameters controlling P adsorption in the 0- to 5-, 5- to 10-, and 10- to 20-cm soil layers, respectively.
The P adsorption was also controlled by CaCO3, but this effect is not dominant in soils with a low P content (Freeman and Rowell, 1981). As shown in the current study, CaCO3 was negatively correlated with Qm and positively correlated with DPS, especially at the 5–10 cm soil depth (Figure 6B). The high content of CaCO3 in soil with the main inorganic P form of Ca-P contributed to the increase in DPS (Wang et al., 2019). The Qm value exhibited the reverse trend of DPS, indicating that CaCO3 reduced Qm in the 5- to 10-cm soil layer, which supports the findings of Bai et al. (2017) but is contrary to the general results (Leytem and Mikkelsen, 2005; Prakash et al., 2017). However, the Ca-P content in deep soil was reduced, and the effect on DPS decreased. Correspondingly, the effect of CaCO3 on adsorption parameters in the deeper soil layer was not significant, which might also be because the binding capacity of clay with P by adsorption exceeded that of CaCO3 with P in deep soil at low P contents (Figure 6C).
Cotton cultivation led to a gradual loss of fine particles by the influence of P fertilization, and the clay content of soil decreased with the increase in the P application level (Supplementary Table S11). Our findings suggest that the clay content was positively correlated with Qm, especially in the 10- to 20-cm soil layer (Figure 6C). In general, the majority of the adsorbed P was found on illite under P application in neutral and alkaline soils (Devau et al., 2011). Illite is a common clay mineral in the soil of northern China, which may have resulted in the contribution of clay to P adsorption. It may also be attributed to the specific surface area and CEC of clay minerals (Gérard, 2016). The clay accumulated on the surface layer of cotton field in this study (Supplementary Table S11); however, it had a reduced adsorption capacity compared with the deep layer (Figure 3; Supplementary Table S2). It may be affected by the P content. This indicates that clay was not the leading factor affecting the variance of soil adsorption in the soil profile.
It is also worth noting that there was a facilitation of P adsorption by CEC in the cotton field, especially in the 10- to 20-cm soil layer (Figure 6C), which is consistent with the result of Gonzalez-Pradas et al. (1994). The effects of CEC on P adsorption are related not only to the clay mineral type but also to the SOM content (Messmer et al., 2014). In the current study, SOM was not the main factor of P adsorption (Figure 6) as it primarily came from the returned stubble and root of cotton, and its content is low (Supplementary Table S5). Perhaps because of the influence of SOM, the effect of CEC on P absorption was not significant in other soil layers, except in the 10- to 20-cm soil layer.
Although the salinity was not the main factor affecting P adsorption and desorption, a higher salinity of soil was found to inhibit the P adsorption (Figure 6), which supports the previous research results (Zhang and Huang, 2011; Flower et al., 2017). Because of the periodic irrigation during the cotton growth period, the salinity of surface soil was transported downward with water, and the larger salinity of deep soil caused a clearer inhibition of P adsorption. Furthermore, an increase in pH could cause a slight inhibition of P adsorption in surface soil, and a lower P adsorption capacity was observed because of the competition for adsorption sites between OH− and HPO42− at pH > 7.0 (Jalali and Matin, 2015; Bai et al., 2017). However, there was no significant correlation in other soil layers, which may have been related to the minimal change of pH or the interaction with other factors.
Conclusion
A 4-year gradient P fertilizer application to a cotton field resulted in varying degrees of P accumulation in soil compared with unfertilized soil. A large proportion of P added as fertilizer was adsorbed and remained predominantly in the Ca8-P form and then may transform into the Ca2-P form, especially in the surface layer. The accumulation and transformation of P in fertilized soil altered the P adsorption–desorption capacity, and combined with the cotton yield, the optimal P fertilizer application was 75–150 kg P2O5 ha−1 year−1. The soil P content (e.g., TP, Olsen-P, Ca8-P, and Ca10-P), CaCO3, clay, and CEC were regarded as the primary soil attributes affecting P dynamics in soil. Further analysis is needed for improved insights into the detailed P dynamics in the soil of cotton fields with further corroboration by long-term experiments.
Data Availability Statement
The original contributions presented in the study are included in the article/Supplementary Material, and further inquiries can be directed to the corresponding author.
Author Contributions
XT contributed to the conception of the study, experiment, data analyses, and wrote the manuscript; ML performed the experiment; JS contributed to the revision of the manuscript; ZC performed the data analyses; GF helped perform the analysis with constructive discussions; and BC contributed significantly to the analysis and manuscript preparation.
Funding
This work was supported by the National Natural Science Foundation of China (No. 31960629), the Major Scientific and Technological Program of Xinjiang in China (No. 2020A01002-3), and the Tianshan Cedar Program of Xinjiang in China (No. 2020XS05).
Conflict of Interest
The author BC is employed by Xinjiang Huier Agriculture Company Ltd.
The remaining authors declare that the research was conducted in the absence of any commercial or financial relationships that could be construed as a potential conflict of interest.
Publisher’s Note
All claims expressed in this article are solely those of the authors and do not necessarily represent those of their affiliated organizations, or those of the publisher, the editors, and the reviewers. Any product that may be evaluated in this article, or claim that may be made by its manufacturer, is not guaranteed or endorsed by the publisher.
Acknowledgments
The authors thank Alex Boon, from Liwen Bianji, Edanz Editing China (www.liwenbianji.cn/ac), for editing the English text of a draft of this manuscript.
Supplementary Material
The Supplementary Material for this article can be found online at: https://www.frontiersin.org/articles/10.3389/fenvs.2022.874902/full#supplementary-material
Abbreviations
P, phosphorus; CaCO3, calcium carbonate; CEC, cation exchange capacity; O-P, occluded P; SOM, soil organic matter; TP, total phosphorus; MBC, maximum buffer capacity; DPS, degree of phosphorus saturation; RDA, redundancy analysis.
References
Bai, J., Ye, X., Jia, J., Zhang, G., Zhao, Q., Cui, B., et al. (2017). Phosphorus Sorption-Desorption and Effects of Temperature, pH and Salinity on Phosphorus Sorption in Marsh Soils from Coastal Wetlands with Different Flooding Conditions. Chemosphere 188, 677–688. doi:10.1016/j.chemosphere.2017.08.117
Bera, R., Seal, A., Bhattacharyya, P., Mukhopadhyay, K., and Giri, R. (2006). Phosphate Sorption Desorption Characteristics of Some Ferruginous Soils of Tropical Region in Eastern India. Environ. Geol. 51 (3), 399–407. doi:10.1007/s00254-006-0335-9
Bhattacharyya, P., Nayak, A. K., Shahid, M., Tripathi, R., Mohanty, S., Kumar, A., et al. (2015). Effects of 42-year Long-Term Fertilizer Management on Soil Phosphorus Availability, Fractionation, Adsorption-Desorption Isotherm and Plant Uptake in Flooded Tropical rice. Crop J. 3 (5), 387–395. doi:10.1016/j.cj.2015.03.009
Carreira, J. A., Viñegla, B., and Lajtha, K. (2006). Secondary CaCO3 and Precipitation of P-Ca Compounds Control the Retention of Soil P in Arid Ecosystems. J. Arid Environ. 64 (3), 460–473. doi:10.1016/j.jaridenv.2005.06.003
Chen, W., Jin, M., Ferré, T. P. A., Liu, Y., Huang, J., and Xian, Y. (2020). Soil Conditions Affect Cotton Root Distribution and Cotton Yield under Mulched Drip Irrigation. Field Crops Res. 249, 107743–107752. doi:10.1016/j.fcr.2020.107743
Devau, N., Hinsinger, P., Le Cadre, E., Colomb, B., and Gérard, F. (2011). Fertilization and pH Effects on Processes and Mechanisms Controlling Dissolved Inorganic Phosphorus in Soils. Geochim. Cosmochim. Acta 75 (10), 2980–2996. doi:10.1016/j.gca.2011.02.034
Dou, Z., Ramberg, C. F., Toth, J. D., Wang, Y., Sharpley, A. N., Boyd, S. E., et al. (2009). Phosphorus Speciation and Sorption-Desorption Characteristics in Heavily Manured Soils. Soil Sci. Soc. Am. J. 73, 93–101. doi:10.2136/sssaj2007.0416
Flower, H., Rains, M., Lewis, D., Zhang, J.-Z., and Price, R. (2017). Saltwater Intrusion as Potential Driver of Phosphorus Release from limestone Bedrock in a Coastal Aquifer. Estuarine, Coastal Shelf Sci. 184, 166–176. doi:10.1016/j.ecss.2016.11.013
Freeman, J. S., and Rowell, D. L. (1981). The Adsorption and Precipitation of Phosphate onto Calcite. J. Soil Sci. 32, 75–84. doi:10.1111/j.1365-2389.1981.tb01687.x
Gérard, F. (2016). Clay Minerals, Iron/aluminum Oxides, and Their Contribution to Phosphate Sorption in Soils-A Myth Revisited. Geoderma 262, 213–226. doi:10.1016/j.geoderma.2015.08.036
Gonzalez-Pradas, E., Socias-Viciana, M., Villafranca-Sanchez, M., and Fernandez-Perez, M. (1994). Ionic Strength and pH Effects on Sorption of Phosphate by Calcareous Soils from Spain. Fresen. Environ. Bull. 3 (2), 69–74.
Guo, S.-L., Dang, T.-H., and Hao, M.-D. (2008). Phosphorus Changes and Sorption Characteristics in a Calcareous Soil under Long-Term Fertilization. Pedosphere 18 (2), 248–256. doi:10.1016/s1002-0160(08)60014-4
Heidari, S., Reyhanitabar, A., and Oustan, S. (2017). Kinetics of Phosphorus Desorption from Calcareous Soils Using DGT Technique. Geoderma 305, 275–280. doi:10.1016/j.geoderma.2017.06.012
Jalali, M., and Hemati Matin, N. (2015). Sorption of Phosphorus in Calcareous Paddy Soils of Iran: Effects of Soil/solution Ratio and pH. Environ. Earth Sci. 73 (5), 2047–2059. doi:10.1007/s12665-014-3555-4
Jalali, M., and Peikam, E. N. (2013). Phosphorus Sorption-Desorption Behaviour of River Bed Sediments in the Abshineh River, Hamedan, Iran, Related to Their Composition. Environ. Monit. Assess. 185 (1), 537–552. doi:10.1007/s10661-012-2573-5
Jiang, B., and Gu, Y. (1989). A Suggested Fractionation Scheme of Inorganic Phosphorus in Calcareous Soils. Fertilizer Res. 20 (3), 159–165. doi:10.1007/bf01054551
Jiao, Y. P., Qi, P., Wang, X. J., Yao, Y. M., Wu, J., Cai, L. Q., et al. (2020). Effects of Nitrogen and Phosphorus Fertilization on Inorganic Phosphorus Forms of Typical farmland Soil in the Dry Farming Area of the Loess Plateau. J. Plant Nutr. Fertili. 26 (8), 1459–1472. (in Chinese). doi:10.11674/zwyf.20063
Keter, J. K. A., and Ahn, P. M. (2010). Profile Characteristics, and Form and Surface Activity of Inorganic Phosphorus in a Deep Red Kenya Coffee Soil (Nitosol). Eur. J. Soil Sci. 37 (1), 89–91. doi:10.1111/j.1365-2389.1986.tb00010.x
Leytem, A. B., and Mikkelsen, R. L. (2005). The Nature of Phosphorus in Calcareous Soils. Better Crop 89 (2), 11–13.
Li, W. W., Teng, Y. M., and Li, J. (2020a). Soil Phosphorus Fraction and Transformation under Different Production Modes of Greenhouse Vegetables. Soils 52 (2), 271–278. (in Chinese). doi:10.13758/j.cnki.tr.2020.02.007
Li, C. L., Zhang, P., Zhang, J. J., Zhu, P., and Wang, L. C. (2020b). Forms, Transformations, and Availability of Phosphorus after 32 Years of Manure and mineral Fertilization in a Mollisol under Continuous maize Cropping. Arch. Agron. Soil Sci. 67 (9), 1256–1271. doi:10.1080/03650340.2020.1787385
Lv, H., and Yang, H. (2019). Effects of Long-Term Fertilization on the Form of Inorganic Phosphorus and the Characteristic of Adsorption and Desorption in Black Soil. Commun. Soil Sci. Plant Anal. 50 (6), 763–771. doi:10.1080/00103624.2019.1589485
MacDonald, G. K., Bennett, E. M., Potter, P. A., and Ramankutty, N. (2011). Agronomic Phosphorus Imbalances across the World's Croplands. Proc. Natl. Acad. Sci. U.S.A. 108 (7), 3086–3091. doi:10.1073/pnas.1010808108
Mahmood, M., Tian, Y., Ma, Q. X., Ahmed, W., Mehmood, S., Hui, X. L., et al. (2020). Changes in Phosphorus Fractions and its Availability Status in Relation to Long Term P Fertilization in Loess Plateau of China. Agronomy 10 (11), 1–16. doi:10.3390/agronomy10111818
Mai, W., Xue, X., Feng, G., Yang, R., and Tian, C. (2018). Can Optimization of Phosphorus Input lead to High Productivity and High Phosphorus Use Efficiency of Cotton through Maximization of Root/mycorrhizal Efficiency in Phosphorus Acquisition? Field Crops Res. 216, 100–108. doi:10.1016/j.fcr.2017.11.017
McDowell, R. W. (2012). Minimising Phosphorus Losses from the Soil Matrix. Curr. Opin. Biotechnol. 23 (6), 860–865. doi:10.1016/j.copbio.2012.03.006
McLaughlin, M. J., McBeth, T. M., Smernik, R., Stacey, S. P., Ajiboye, B., and Guppy, C. (2011). The Chemical Nature of P Accumulation in Agricultural Soils-Implications for Fertiliser Management and Design: an Australian Perspective. Plant Soil 349 (1-2), 69–87. doi:10.1007/s11104-011-0907-7
Messmer, T., Elsenbeer, H., and Wilcke, W. (2014). High Exchangeable Calcium Concentrations in Soils on Barro Colorado Island, Panama. Geoderma 217-218, 212–224. doi:10.1016/j.geoderma.2013.10.021
Murphy, J., and Riley, J. P. (1962). A Modified Single Solution Method for the Determination of Phosphate in Natural Waters. Anal. Chim. Acta 27, 31–36. doi:10.1016/s0003-2670(00)88444-5
Pavinato, P. S., Merlin, A., and Rosolem, C. A. (2009). Phosphorus Fractions in Brazilian Cerrado Soils as Affected by Tillage. Soil Tillage Res. 105 (1), 149–155. doi:10.1016/j.still.2009.07.001
Prakash, D., Benbi, D. K., and Saroa, G. S. (2017). Clay, Organic Carbon, Available P and Calcium Carbonate Effects on Phosphorus Release and Sorption-Desorption Kinetics in Alluvial Soils. Commun. Soil Sci. Plant Anal. 48 (1), 92–106. doi:10.1080/00103624.2016.1253724
Rubaek, G. H., Kristensen, K., Olesen, S. E., Ostergaard, H. S., and Heckrath, G. (2013). Phosphorus Accumulation and Spatial Distribution in Agricultural Soils in Denmark. Geoderma 209, 241–250. doi:10.1016/j.geoderma.2013.06.022
Schoumans, O. F., and Groenendijk, P. (2000). Modeling Soil Phosphorus Levels and Phosphorus Leaching from Agricultural Land in the Netherlands. J. Environ. Qual. 29 (1), 111–116. doi:10.2134/jeq2000.00472425002900010014x
Shahabifar, J., Panahpour, E., Moshiri, F., Gholami, A., and Mostashari, M. (2019). The Quantity/intensity Relation Is Affected by Chemical and Organic P Fertilization in Calcareous Soils. Ecotoxicol. Environ. Saf. 172, 144–151. doi:10.1016/j.ecoenv.2019.01.058
Sugihara, S., Funakawa, S., Nishigaki, T., Kilasara, M., and Kosaki, T. (2012). Dynamics of Fractionated P and P Budget in Soil under Different Land Management in Two Tanzanian Croplands with Contrasting Soil Textures. Agric. Ecosyst. Environ. 162, 101–107. doi:10.1016/j.agee.2012.07.019
Sun, T., Deng, L., Fei, K., Zhang, L., and Fan, X. (2020). Characteristics of Phosphorus Adsorption and Desorption in Erosive Weathered Granite Area and Effects of Soil Properties. Environ. Sci. Pollut. Res. 27 (23), 28780–28793. doi:10.1007/s11356-020-08867-1
Wang, R., Guo, S., Li, N., Li, R., Zhang, Y., Jiang, J., et al. (2015). Phosphorus Accumulation and Sorption in Calcareous Soil under Long-Term Fertilization. PLoS One 10 (8), e0135160–14. doi:10.1371/journal.pone.0135160
Wang, Q., Zhan, X. Y., Zhang, S. X., Peng, C., Gao, H. J., Zhang, X. Z., et al. (2019). Phosphorus Adsorption and Desorption Characteristics and its Response to Soil Properties of Black Soil under Long-Term Different Fertilization. Sci. Agricult. Sin. 52 (21), 3866–3877. (in Chinese). doi:10.3864/j.issn.0578-1752.2019.21.015
Wang, F., Li, Q. H., Lin, C., and He, C. M. (2020). Yellow-mud Paddy Soil Productivity and Phosphorus Fractions under Long-Term Different Phosphorus Supply Levels in Southern China. Chin. J. Eco-Agricul. 28 (7), 960–968. (in Chinese). doi:10.13930/j.cnki.cjea.200059
Yan, X., Wei, Z., Hong, Q., Lu, Z., and Wu, J. (2017). Phosphorus Fractions and Sorption Characteristics in a Subtropical Paddy Soil as Influenced by Fertilizer Sources. Geoderma 295, 80–85. doi:10.1016/j.geoderma.2017.02.012
Yan, Z., Chen, S., Dari, B., Sihi, D., and Chen, Q. (2018). Phosphorus Transformation Response to Soil Properties Changes Induced by Manure Application in a Calcareous Soil. Geoderma 322, 163–171. doi:10.1016/j.geoderma.2018.02.035
Yang, X., Chen, X., and Yang, X. (2019). Effect of Organic Matter on Phosphorus Adsorption and Desorption in a Black Soil from Northeast China. Soil Tillage Res. 187, 85–91. doi:10.1016/j.still.2018.11.016
Yang, S., Zhao, Y., and Wang, J. (2020). Function and Application of the Eutrema Salsugineum PHT1;1 Gene in Phosphate Deficiency Stress. Plant Biol. J. 22 (6), 1133–1139. doi:10.1111/plb.13169
Zhang, J.-Z., and Huang, X.-L. (2011). Effect of Temperature and Salinity on Phosphate Sorption on marine Sediments. Environ. Sci. Technol. 45 (16), 6831–6837. doi:10.1021/es200867p
Zhang, Y., Huang, S., Guo, D., Zhang, S., Song, X., Yue, K., et al. (2018a). Phosphorus Adsorption and Desorption Characteristics of Different Textural Fluvo-Aquic Soils under Long-Term Fertilization. J. Soils Sediments 19 (3), 1306–1318. doi:10.1007/s11368-018-2122-0
Zhang, X., Gu, H. Y., and Chen, X. W. (2018b). Effects of Selective Cutting Disturbance on Soil Phosphorus Adsorption and Desorption in a Korean pine and Broad-Leaved Mixed forest in the Xiaoxing'an Mountains, China., (in Chinese). Ying Yong Sheng Tai Xue Bao 29 (1), 11–17. Available at: http://www.cjae.net/CN/Y2018/V29/I1/11. doi:10.13287/j.1001-9332.201801.004
Zhang, T. Q., Zheng, Z. M., Drury, C. F., Hu, Q. C., and Tan, C. S. (2020). Legacy Phosphorus after 45 Years with Consistent Cropping Systems and Fertilization Compared to Native Soils. Front. Earth Sci. 8, 183–193. doi:10.3389/feart.2020.00183
Zhang, W., Zhang, Y., An, Y., and Chen, X. (2021). Phosphorus Fractionation Related to Environmental Risks Resulting from Intensive Vegetable Cropping and Fertilization in a Subtropical Region. Environ. Pollut. 269, 116098–116107. doi:10.1016/j.envpol.2020.116098
Zhao, S. C., Zhang, Z. Y., Xia, J. H., Yang, J., Sheng, L. T., Tang, D., et al. (2019). Phosphorus Adsorption Characteristics of Riparian Soils Surrounding Poyang Lake, (in Chinese). Resour. Env. Yangtz. 28 (1), 166–174. Available at: http://yangtzebasin.whlib.ac.cn/CN/10.11870/cjlyzyyhj201901017. doi:10.11870/cjlyzyyhj201901017
Keywords: adsorption–desorption, inorganic P fractions, P application rate, cotton field, gray desert soil
Citation: Tang X, Liu M, Sheng J, Chai Z, Feng G and Chen B (2022) Inorganic Phosphorus Transformation and Phosphorus Adsorption–Desorption Properties of Soil in a Cotton Field Under Mulched Drip Irrigation in Xinjiang: A Four-Year Field Assessment. Front. Environ. Sci. 10:874902. doi: 10.3389/fenvs.2022.874902
Received: 13 February 2022; Accepted: 11 April 2022;
Published: 16 May 2022.
Edited by:
Dionisios Gasparatos, Agricultural University of Athens, GreeceReviewed by:
Maryam Foroughi, University of Maryland, United StatesJuan Fernando Hirzel, Instituto de Investigaciones Agropecuarias, Chile
Copyright © 2022 Tang, Liu, Sheng, Chai, Feng and Chen. This is an open-access article distributed under the terms of the Creative Commons Attribution License (CC BY). The use, distribution or reproduction in other forums is permitted, provided the original author(s) and the copyright owner(s) are credited and that the original publication in this journal is cited, in accordance with accepted academic practice. No use, distribution or reproduction is permitted which does not comply with these terms.
*Correspondence: Bolang Chen, chenwang200910@sina.com